- 1Department of Biology, Lomonosov Moscow State University, Moscow, Russia
- 2Department of Immunology, Shemyakin and Ovchinnikov Institute of Bioorganic Chemistry RAS, Moscow, Russia
- 3National Research Center for Hematology, Moscow, Russia
Immunotherapy harnessing the host immune system for tumor destruction revolutionized oncology research and advanced treatment strategies for lymphoma patients. Lymphoma is a heterogeneous group of cancer, where the central roles in pathogenesis play immune evasion and dysregulation of multiple signaling pathways. Immunotherapy-based approaches such as engineered T cells (CAR T), immune checkpoint modulators and NK cell-based therapies are now in the frontline of lymphoma research. Even though emerging immunotherapies showed promising results in treating lymphoma patients, low efficacy and on-target/off-tumor toxicity are of a major concern. To address that issue it is suggested to look into the emerging role of heat shock proteins. Heat shock proteins (HSPs) showed to be highly expressed in lymphoma cells. HSPs are known for their abilities to modulate immune responses and inhibit apoptosis, which made their successful entry into cancer clinical trials. Here, we explore the role of HSPs in Hodgkin and Non-Hodgkin lymphoma and their involvement in CAR T therapy, checkpoint blockade and NK cell- based therapies. Understanding the role of HSPs in lymphoma pathogenesis and the ways how HSPs may enhance anti-tumor responses, may help in the development of more effective, specific and safe immunotherapy.
Introduction
Lymphoma is a heterogeneous cancer divided into two major types such as Hodgkin lymphoma (HL) and Non-Hodgkin lymphoma (NHL) (1, 2). In oncology research, management of lymphoma stands out as the choice of treatment is largely based on the results obtained from prospective clinical trials (1). Standard treatment regimen includes chemotherapy and radiation therapy for the treatment of HL and chemotherapy combined with anti-CD20 antibodies for NHL patients, reaching the cure rate of 80-90% (1, 2). Even though the response rate is high, treatment-related toxicity such as induction of second malignancy and cardiotoxicity is of a major concern (1). Following initial treatment, 10-30% of lymphoma patients develop refractory or recurrent (r/r) disease which is treated with high-dose chemotherapy followed by an autologous hematopoietic stem cell transplantation (ASCT) (1, 2). The overall goal of current and emerging treatments for HL and NHL is to cure disease and minimize treatment-related toxicity (1, 2). Current treatments for lymphoma patients are summarized in Table 1. Recently approved treatments for r/r HL and NHL subtypes include anti-CD30 antibody-drug conjugate brentuximab vedotin, PD1 inhibitors (pembrolizumab and nivolumab), Bruton’s tyrosine kinase inhibitors (ibrutinib and acalabrutinib), phosphoinositide 3-kinase γ and/or δ inhibitors (idelalisib, copanlisib and duvelisib) and CD19 chimeric antigen receptor (CAR) T cell therapy (tisagenlecleucel and axicabtagene ciloleucel) (Table 1) (1, 2, 29).
Heat shock proteins (HSPs) are molecular chaperones highly expressed in various types of cancer. HSPs are classified into several families such as HSP110, HSP90, HSP70, HSP40, chaperonins and HSPB (30). HSPs are largely known for their role in blocking apoptosis which was further translated into development of HSP inhibitors (31–49). Along this line, Kamal and colleagues showed that HSP90 inhibitor 17-allylamino-17-demethoxy-geldanamycin (17-AAG) selectively targets cancer cells (50). In light of the reported, several HSP90 inhibitors such as alvespimycin (NCT01126502), luminespib (NCT01485536), PU-H71 (NCT01581541) and SNX-5422 (NCT02914327) currently are assessed in clinical trials for the treatment of lymphoma patients. Furthermore, HSPs showed to be potent immune system activators through the induction of cytotoxic CD8+T cell response (51–56). Several HSP-based vaccines have been evaluated in clinical trials (57–61). Specifically, the efficacy and safety of HSPPC-96 vaccine, which is an autologous gp96 heat shock protein-peptide complex vaccine, was assessed in patients with indolent non-Hodgkin lymphoma (62). Inspired by the ability of HSPs to induce immune responses, Li and colleagues developed a novel nanovaccine that mimics HSPs, so it can be used to stimulate anti-tumor immune responses (55). Additionally, numerous studies have assessed extracellular HSPs derived from various liquid biopsies (serum, plasma, urine, plasma/serum/urine-derived exosomes) as potential cancer biomarkers [reviewed in (31)] (63–71).
Novel emerging immunotherapy approaches involve CAR T cell therapy, checkpoint inhibitors and NK cell-based therapies that are aimed at improving effectiveness in treating of lymphoma patients. In this review, we focus on the role of HSP family in Hodgkin and Non-Hodgkin lymphoma. Since the mechanism of apoptosis and immune modulation are the key features in lymphoma pathogenesis, it is of particular interest to explore the contribution of HSPs in this process. We explain how the understanding of the cross-talk between tumor microenvironment (TME) and malignant cells and the role of HSPs in this process may help to improve emerging treatments for lymphoma patients.
HSPs in Hodgkin Lymphoma
Hodgkin lymphoma (HL) is a B cell lymphoma divided into classic HL (cHL) which accounts for the majority of the cases and nodular lymphocyte-predominant HL (NLPHL) (1). Histologically, cHL is classified into four types such as nodular sclerosis HL (NSHL), mixed cellularity HL (MCHL), lymphocyte-rich HL (LRHL) and lymphocyte-depleted HL (LDHL) (1).
cHL is characterized by the presence of malignant Hodgkin and Reed-Sternberg (HRS) cells that constitute minor population (~1%) of the tumor mass (72). The majority of infiltrate surrounding HRS cells is represented by different types of non-malignant immune cells such as dendritic cells, macrophages, lymphocytes, mast cells, neutrophils, eosinophils and fibroblasts which form tumor microenvironment (TME) (1, 72). Even though, HRS cells are germinal center (GC)- derived B cells, they resemble immunophenotype that does not associate with any known cells of hematopoietic origin (Figure 1) (74). Specifically, HRS cells rarely express typical B-cell lineage markers such as CD19, CD20, CD22, CD79, CD79B and instead express markers of dendritic cells (CD83), myeloid markers (CD15) and T cell markers (CD2, CD3, CD4) (1, 74, 75, 81). Additionally, members of tumor necrosis factor family namely CD30 and CD40 are expressed on HRS cells (75). It is interesting to point out that cHL TME is composed of variable cellularity that is different in each cHL subtype (1). As an example, NSHL is rich in fibroblast-like cells and fibrosis, MCHL is composed of B cells, T cells, neutrophils, histiocytes, plasma cells and mast cells, LRHL is characterized by HRS cells surrounded by mantle zone B cells and histiocytes whereas LDHL predominantly consists of CD4+T cells, histiocytes and fibrosis (1).
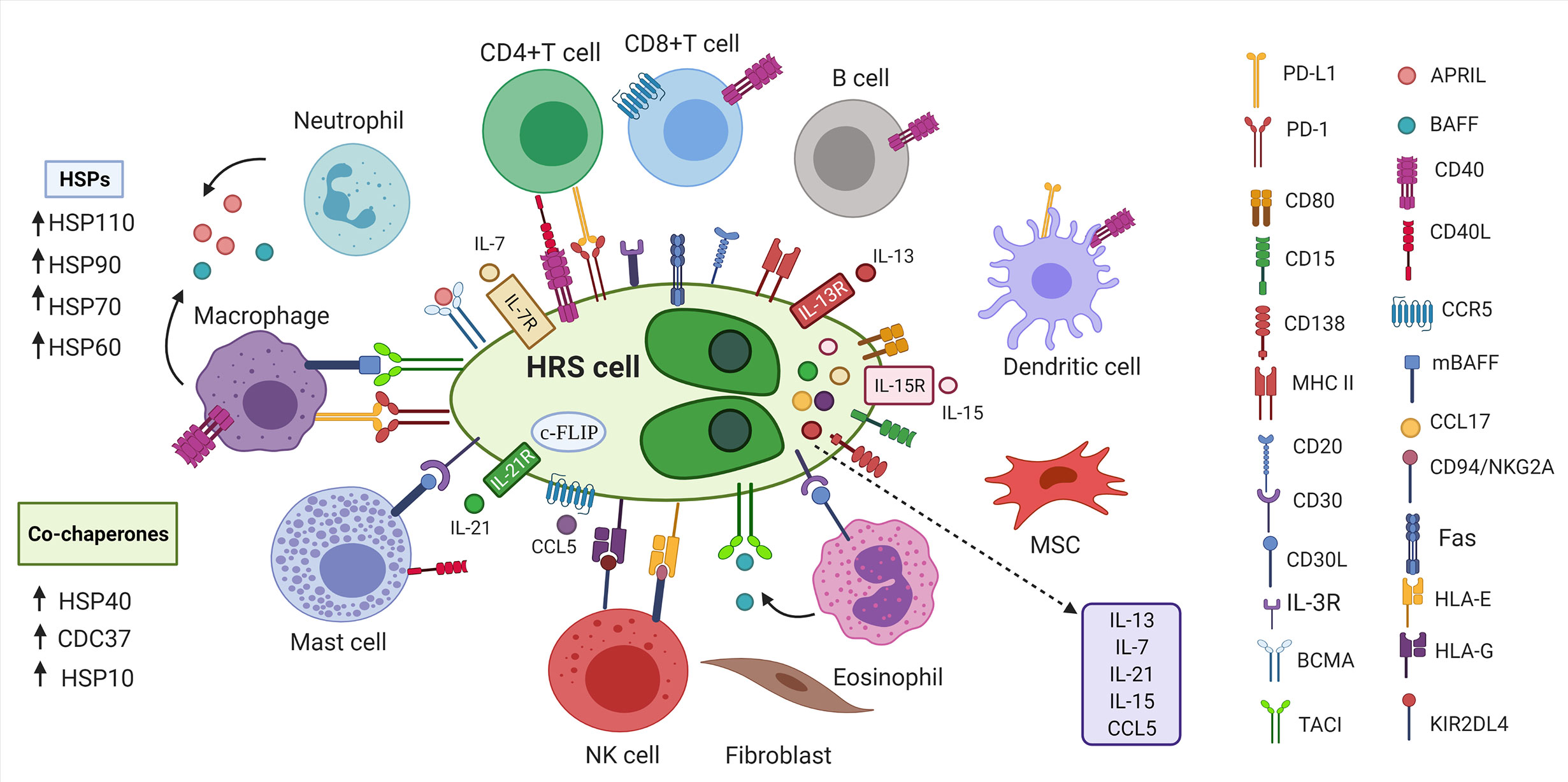
Figure 1 Tumor microenvironment in cHL. HRS cells are surrounded by non-malignant immune and stromal cells. Inflammatory cells secrete cytokines, tumor necrosis family members (CD40L, CD30L) and other molecules (APRIL, BAFF) that bind to the proteins on the surface of HRS cells to promote growth and survival of HRS cells (1, 73). HRS cells express various markers of B cells, T cells, myeloid markers, markers of dendritic cells (74). HRS cells express PD-L1 to escape anti-tumor responses (75). HRS cells express Fas, but avoid FasL-mediated apoptosis by overexpressing c-FLIP (75–77). HRS cells express FasL leading to apoptosis of Fas-expressing NK cells (76, 78). HSP chaperones and their corresponding co-chaperones are highly expressed in HRS cells, which further contribute to immunosuppressive TME (79, 80). HRS, Hodgkin and Reed-Sternberg cells; BCMA, B cell maturation antigen; APRIL, a proliferation-inducing ligand; BAFF, B-cell activating factor;PD-L1, programmed death ligand 1; PD-1, programmed death 1; CD30L, CD30 ligand; CD40L, CD40 ligand; CCL5, CC-chemokine ligand 5; IL-3R, interleukin-3 receptor; TACI, transmembrane activator and calcium-modulator and cyclophilin ligand interactor; HSP, Heat shock protein; MSC, mesenchymal stromal cells; mBAFF, membrane-bound B-cell activating factor; MHC II, Major histocompatibility complex class II; HLA-E/G, Human leukocyte antigen- E/G; KIR2DL4, killer cell immunoglobulin-like receptor family member; c-FLIP, cellular FLICE-inhibitory protein; cHL, classic Hodgkin lymphoma; NK cells, natural killer cells.
Nuclear factor- kappa B (NF-kB) is constitutively activated in HRS cells (1, 82). Engagement of CD40, CD30, RANK and BCMA/TACI with their cognate ligands showed to activate NF-kB leading to increased production of IL-6, IL-8, CCL5, IFNγ and IL-13 (75). During activation process, the IKK complex, which is composed of two kinase subunits IKKα, IKKβ and a regulatory subunit IKKγ, phosphorylates IkBα, an inhibitor of NF-kB, allowing NF-kB translocation to the nucleus and activation of genes responsible for B-cell proliferation and survival (73). Notably, treatment with HSP90 inhibitor geldanamycin resulted in inactivation of NF-kB and IKK activity in HRS cell lines (83). HSP90 showed to stabilize subunits of IKK complex (IKKα and IKKβ) and protect them from proteasomal degradation (83).
Another important signaling cascade which is constitutively activated in HRS cells is Janus kinase (JAK)-signal transducer and activator of transcription (STAT) (1). Activation of JAK-STAT pathway leads to hyperphosphorylation of STAT proteins (1). In particular, STAT3, STAT6 and STAT5 showed to be constitutively phosphorylated in cHL cell lines (84–86). Intriguingly, Schoof and coworkers reported that pharmacological blocking of HSP90 inhibited the phosphorylation of STAT1, STAT3, STAT5 and STAT6 in cHL cell lines (84). Overall, HSP90-targeting agents may be a promising strategies in cHL where deregulated NF-kB and JAK-STAT signaling pathways play a major role in cHL pathogenesis.
Phosphatidylinositol 3-kinase (PI3K)-serine/threonine protein kinase (AKT) pathway is also constitutively activated in HRS cells as a result of activation of multiple receptor tyrosine kinases (RTKs) (1, 87). RTKs such as platelet-derived growth factor receptor A (PDGFRA), discoidin domain-containing receptor 2(DDR2),tyrosine kinase receptor A (TRKA) and TRKB showed to be aberrantly expressed in HRS cells of HL patients, while no expression of these RTKs was observed in normal B cells or B-cell NHL cells (87). Furthermore, aberrant expression of mitogen-activated protein kinase (MAPK)/ERK has been reported in HL (88).In light of the reported, HSP90 inhibitor 17-AAG showed to deplete AKT and inhibit extracellular signal-regulated kinase (ERK) phosphorylation, leading to growth arrest and apoptosis in HL cell lines (89) (88). This was further supported by the finding that HSP90 inhibitor celastrol induced anti-tumor effects in HRS cells by downregulating RAS, ERK1/2 and c-Fos (90). In another experiment, inhibition of HSP90 by geldanamycin induced apoptosis in HRS cells with wild-type IkBα in p53-independent manner (91). Taken together, these observations suggest that targeting AKT, NF-kB and MAPK/ERK pathways with HSP90 inhibitors may prove effective in HL treatment.
In addition to unique immunophenotype and multiple deregulated signaling pathways, HRS cells express high level of HSPs. Hsu and colleagues assessed HSP expression of formalin-fixed, paraffin-embedded tissues derived from patients with different cHL subtypes (80). High cytoplasmic expression of HSP90 and HSP60 in HRS cells was found in NSHL, MCHL, LRHL and LDHL (80). By contrast, no cytosolic HSP27 expression was found in HRS cells in LRHL and low expression in LDLH while 20% of patients with NSHL and MCHL showed strong HSP27 expression (80). Later, Santon and co-workers used tissue microarray to analyze immunohistochemical expression of HSPs in HRS cells of cHL patients (79). More than 90 percent of cHL patients in HRS cells showed high cytoplasmic expression of HSP60, HSP10, HSP90, and CDC37, nuclear HSF1 whereas HSP110 showed to be highly expressed in nucleus and cytoplasm of HRS cells (79). Positive cytoplasmic staining of HSP70 and cytoplasmic/nuclear expression of HSP40 was observed in 78% of cHL patients whereas 54% had positive cytoplasmic expression of HSP27 (79). Expression of HSP90 and HSP70 positively correlated with expression of their co-chaperones CDC37 and HSP40, respectively (79). Furthermore, expression of HSP40 positively correlated with p53, caspase 9 and cellular FLICE-inhibitory protein (c-FLIP) whereas HSP70 expression correlated with caspase 3 (79). In another study, high cytoplasmic expression of HSP60 was observed in HRS cells in 100% of NSHL and MCHL cases (92).
HSPs in Non-Hodgkin Lymphoma
Non-Hodgkin lymphoma (NHL) is comprised of B-cell lymphoma, accounting for the majority of the NHL lymphoma subtypes, while other NHLs include T-cell lymphoma and NK-cell lymphoma (93). NHL is classified into indolent (slow-growing) and aggressive (fast-growing) lymphoma. The most common indolent lymphoma is follicular lymphoma (FL), while other slow-growing lymphoma subtypes include marginal zone lymphoma (MZL), chronic lymphocytic leukemia (CLL)/small lymphocytic lymphoma (SLL) and lymphoplasmacytic lymphoma (94). The most common aggressive NHL subtype is represented by diffuse large B-cell lymphoma (DLBCL), while other aggressive lymphoma subtypes include mantle cell lymphoma (MCL), Burkitt lymphoma (BL) and primary effusion lymphoma (94).
Lymphoma cells largely depend on microenvironment for their growth and survival (95). Continuous signaling from B cell receptor (BCR), immune and stromal cells are required to support proliferation activity and survival of lymphoma cells. BCR is required for B cell survival and the loss of BCR results in B cell death (95, 96). BCR activation by self-antigens showed to be a driving force in various NHL subtypes (97–99). Moreover, some subtypes of DLBCL carry genetic mutations that activate BCR signaling, including mutation in CD79B and CARD11, where the latter mutation leads to constitutive activation of NF-kB in activated B cell-like (ABC) subtype of DLBCL (95, 97, 100–102). Notably, Walter and colleagues demonstrated that HSP90 and its client protein spleen tyrosine kinase (SYK) are required for tonic BCR signaling in BL lymphoma, suggesting potential use of HSP90 as potential target for BL lymphoma treatment (103, 104). Additionally, HSP90 showed to stabilize BCR kinases such as Bruton tyrosine kinase (BTK), SYK, LYN and AKT in chronic lymphocytic leukemia cells (105). Recent studies have added more insight into the role of HSP90 in BCR signaling in NHL subtypes. Jacobson and colleagues reported that HSP90 inhibition led to the complete loss of BTK and IKKα and downstream loss of phosphorylated ERK1/2 in mantle cell lymphoma cell lines (106).Moreover, HSP90 inhibitor showed to downregulate BTK in cells expressing BTK C481S mutation, which was found to be associated with resistance to BTK inhibitor ibrutinib in MCL and CLL patients (106–108). Importantly, Cerchietti and colleagues showed that HSP90 interacts with B-cell lymphoma-6 (Bcl-6) which was further supported by the finding that HSP90 inhibitor PU-H71 selectively killed Bcl-6-dependent DLBCL cells (109). Subsequently, Goldstein and co-workers used PU-H71 and tumor-enriched HSP90 (teHSP90) complexes derived from DLBCL cell lines to show that LYN, SYK, BTK and phospholipase C γ 2(PLCγ2) are dependent on teHSP90 (110). Furthermore, treatment with PU-H71 showed to disrupt BCR signaling, calcium influx and NF-kB activity, resulting in cell growth inhibition (110). Additionally, PU-H71 in combination with ibrutinib led to the killing of lymphoma cells, suggesting that combinatorial therapeutic approach may be more effective in NHL patients (110).
In addition to continuous BCR signaling, lymphoma cells require additional signals to survive. Early experiments in establishing NHL cell lines showed that FL cells require signals from T cells for CD40-mediated interaction and IL-4 stimulation for sustained proliferation of lymphoma cells (95, 111–113). Furthermore, in NHL subtypes myeloid cells secrete high level of B cell- activating factor (BAFF) and a proliferation inducing ligand (APRIL) that are critical for survival and differentiation of B cells (73, 95, 114–117). In addition to the signals provided by immune cells, the cross-talk between stromal cells and FL cells plays important role for the growth of FL B cells [reviewed in (118)].
Members of HSP family showed to be highly expressed in NHL subtypes. Valbuena and colleagues reported moderate-to-strong cytoplasmic expression of HSP90 in 100% of cases of BL,61% of FL patients, 59% of DLBCL, 38% of nodal MZL and 33% of cases with SLL/CLL and 30% of lymphoplasmacytic lymphoma (119). Weak cytoplasmic expression of HSP90 was observed in 43% of cases with extranodal marginal zone B-cell lymphoma of mucosa-associated lymphoid tissue (119). Patients with T-cell lymphoma showed moderate/strong cytoplasmic expression of HSP90 (119). HSP60 also showed to be highly expressed in DLBCL and high-grade FL whereas no HSP60 was detected in low-grade FL (92). NK/T-cell lymphomas showed positive cytoplasmic expression of HSP60 (92).
Recent studies have emphasized the role of HSP110 in aggressive subtypes of B-cell NHLs such as DLBCL and BL (120). Zappasodi and colleagues demonstrated that inactivation of HSP105/HSPH1 leads to downregulation of c-Myc and Bcl-6 (120).Mechanistically, HSP105 showed to interact with c-Myc and Bcl-6 in nucleus in primary human DLBCL and BL cells, suggesting that HSP105 may function as a chaperone for both c-Myc and Bcl-6 (120).Additionally, higher expression of HSP105 was found in DLBCL expressing c-Myc compared to c-Myc low/negative counterparts (120). In light of the reported, Boudesco and co-workers showed that overexpression of HSP110 resulted in upregulation of NF-kB, whereas silencing of HSP110 donwregulated NF-kB, suggesting that there is an interplay between HSP110 and NF-kB (121). Mechanistically, HSP110 showed to stabilize myeloid differentiation factor 88 (MyD88), leading to chronic NF-kB activation in ABC-DLBCL (121). Therefore, targeting HSP110 may be a promising strategy for the treatment of B-cell NHLs.
Phase II clinical trial was conducted to assess the safety and efficacy of HSP90 inhibitor AUY922 in patients with r/r DLBCL and peripheral T-cell lymphoma (PTCL) (92). Overall, 14 patients with DLBCL and 6 with PTCL were enrolled, 1 patient with DLBCL reached complete response (CR) and 1 patient with PTCL achieved partial response (122). Treatment-related adverse effects included fatigue, visual disturbance that was fully reversible and anemia (122). Authors concluded that HSP90 inhibitors may be a good target in some cases, though, combination with chemotherapeutic agents and histone deacetylase (HDAC) inhibitors may be used to improve anti-tumor activity (122). Several studies assessing combination of HSP90 inhibitors with chemotherapeutic drugs such as fludarabine, doxorubicin, cytarabine, melphalan, or HDAC inhibitors demonstrated promising results in hematological malignancies (122–126). Taken together, NHL subtypes have high expression of specific HSP members, however, use of combinatorial approach in NHL patients warrants further investigation.
HSPs and Emerging Lymphoma Immunotherapy
HSPs and CAR T
CAR T therapy involves ex vivo expansion and genetic modification of an autologous (self) or allogeneic (donor) T cells that specifically identify and eliminate cognate target ligand (127, 128). CAR consists of antigen-recognition domain represented by a single-chain variable fragment (scFv), hinge, transmembrane and intracellular signaling domains (127). Majority of CARs contain CD3ζ which is critical for T cell receptor (TCR) signaling (129). Due to low CAR T cell activity and persistence, second generation CARs have been developed that integrated co-stimulatory domains derived from CD28 or 4-1BB into the CAR design (128, 129). Importantly, CAR T cells that contain CD28 domains differentiate into effector memory T cells whereas 4-1BB-domain CAR T cells differentiate into central memory T cells (128, 130).
CAR T showed to be effective in the treatment of B-cell malignancies (129). In 2017 the first CAR T immunotherapy tisagenlecleucel (CD19-specific 4-1BB-CAR) was approved by FDA for the treatment of r/r B-cell acute lymphoblastic leukemia (B-ALL) (128). Later, in 2018, axicabtagene ciloucel (CD19-specific CD28-CAR) was approved for the treatment of r/r DLBCL (93). Nevertheless, the main challenge in CAR T therapy now is to find an antigen universally expressed on tumor cells that can be targeted by CAR T. Since HRS cells almost exclusively express CD30, CD30 was proposed as an attractive target for the CAR T therapy. Up till now only 3 studies assessed the efficacy and safety of CD30 CAR T immunotherapy for the treatment of Hodgkin lymphoma (129). Recently, Ramos and colleagues have conducted two phase I/II clinical trials where autologous CD30 CAR Ts were administered to patients with r/r Hodgkin lymphoma after lymphodepletion with fludarabine in combination with either bendamustine or cyclophosphamide (131). The overall response rate (ORR) for 32 patients was 72%, 19 (59%) of which achieved a complete remission (131). It is encouraging that no neurotoxicity was observed. Cytokine release syndrome (CRS) and skin rash that occurred in 10 and 20 patients, respectively, were found to be associated with cyclophosphamide rather than with bendamustine and both spontaneously resolved (131). In another study, Wang and colleagues designed anti-CD30 CAR and conducted a pilot study in patients with r/r Hodgkin lymphoma (132). After lymphodepletion, patients were infused with anti-CD30 CAR Ts. A total of 9 patients received CD30 CAR T infusion, 3 achieved CR, six experienced CRS from which 4 were low-grade and no neurotoxicity was observed (132). Authors also demonstrated promising results in combination therapy where CD30 CAR T treatment was combined with anti-PD-1 antibody (132). Notably, Watanabe and colleagues showed that CD30 induces expression of HSP90α and HSP90β in cHL by activating heat shock factor 1 (HSF1) (133). Since CD30 and HSP90 are overexpressed in cHL, future studies should explore the effect of anti-CD30 CAR T therapy on HSP90 (133).
The use of CARs against HSP70 was proposed by Smith and colleagues (134). Their invention particularly aims to target membrane-bound form of HSP70, so that specifically HSP70-surface positive tumor cells can be killed (134). Similar to the Smith group, Claffey and co-workers identified heavy chain antibody (HCAb2) that selectively targets HSP90 on malignant cells (135). Based on their findings, authors described an antibody that specifically binds to the cell surface HSP90β isoform (135, 136). Therefore, targeting surface expression of HSPs can be a new promising strategy for the development of more efficient CAR T therapy (Figure 2).
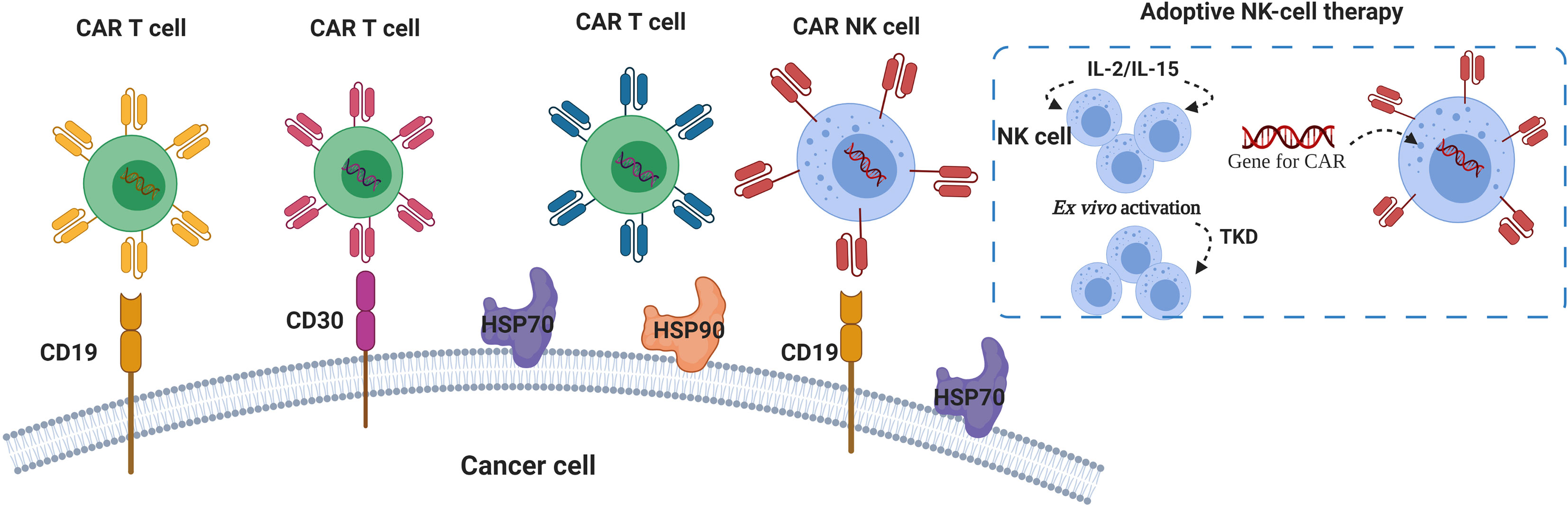
Figure 2 HSPs and CAR T/NK cells in lymphoma immunotherapy. Chimeric Antigens Receptor (CAR) targeting CD19 and CD30 showed promising results in patients with r/r lymphoma (93, 129, 131, 132). Autologous NK cells pre-activated with cytokines (IL-2, IL-15) and 14-mer HSP70-derived peptide (TKD) can be used for ex vivo activation of NK cells for the adoptive transfer therapy (137–140). Anti- HSP70 and HSP90 CARs were proposed for specific targeting of membrane-bound forms of HSP70 and HSP90 (134, 136).
Due to on-target/off-tumor toxicity that associated with the use of CAR T therapy, several investigators proposed that the activity of CAR T cells can be controlled by thermal regulation with the use of HSP-based promoters (pHSP) (141, 142). Shapiro and colleagues used genetically engineered circuits pHSP-CAR to induce CAR expression in T cells in response to thermal stimuli (142). Studies assessing the use of pHSP for thermal control of CAR T highlighted that, despite their names, HSP members respond to various cellular stresses such as heat, hypoxia, radiation, heavy metal toxicity and cytokines (142–144). Therefore, the choice of HSP-based promoter should largely depend on the context in which these promoters will be used (142).
Several investigators demonstrated that HSP90 is crucial for the functional activity of T lymphocytes. For example, Bae and coworkers demonstrated that HSP90 inhibition downregulates the expression of CD3, CD4, CD8 as well as CD28, CD40L and αβ receptors and cripples T cell proliferation and interferon-γ (IFN-γ) secretion (145). In another study, pharmacological blocking of HSP90 resulted in decreased expression of Linker for activation of T cells (LAT) in activated T cells (146). Therefore, taking into account that HSP90 is important for the T cell function and phenotype, and that CAR T-containing CD28 and CD3 domains may affect intracellular and extracellular HSP90 expression, further studies should address the effect of CAR T on HSP90 expression.
HSPs and NK Cell-Based Immunotherapy
The immunosuppressive TME specifically inhibits functional activity and proliferation of NK cells (76). Several investigators reported deficiency in the number of NK cells in the biopsies of cHL patients (76, 147). Moreover, lower number of circulating NK cells were detected in peripheral blood of cHL patients (76, 148, 149). The main goal of NK-based immunotherapy in HL is to reactivate NK cells (150). Boll and colleagues demonstrated that HSP90 inhibitor called BIIB021 combined with doxorubicin and gemcitabine selectively killed Hodgkin lymphoma cells by inhibiting NF-kB activation (150). Moreover, HSP90 inhibition resulted in upregulation of NKG2D ligands such as MHC class I chain-related A (MICA), MICB and ULBP2 on HL cells, making HL cells susceptible to NK-mediated killing (150). In line with that, Fionda and colleagues demonstrated that HSP90 inhibition upregulates MICA and MICB and leads to increased NK cell degranulation in myeloma cell lines (151).
Contrary to T cells, NK cells do not recognize an antigen in the form of MHC-peptide complex, but rather sense the absence of self-MHC class I on tumor cells. HSP90 and HSP70 chaperones showed to be crucial for antigen presentation by MHC I molecule. Binder and colleagues reported that peptide antigens bound to HSPs, such as HSP90, HSP70, gp96, were presented 100 fold more efficiently by MHC I compared to free peptides (152, 153). Callahan and co-workers demonstrated that HSP90 inhibition disrupts the loading of peptides on MHC I (153). Furthermore, Kunizawa and Shastri showed that TCP-1 ring complex (TRiC/CCT) chaperonin is required for the expression of peptide-loaded MHC I on the cell surface (154). Later the same team found that inhibition of HSP90α or co-chaperone carboxyl terminus of Hsc70-interacting protein (CHIP) reduced presentation of peptide-bound MHC I on the cell surface (155).
Several investigators proposed the use of engineered NK cells as promising strategy for adoptive cell therapy in hematological malignancies (156). NK cells that are used in adoptive transfer can be allogeneic, autologous or immortalized such as NK-92 (156, 157). For research studies, NK cells can be isolated from peripheral blood or differentiated from stem cells, ex vivo expanded, activated with cytokines (IL-2, IL-15) and co-cultured with γ-irradiated feeder cells. It is important to point out that NK cells derived from peripheral blood mononuclear cells (PBMC) differ from NK cells differentiated from stem cells (156). For example, NK cells derived from cord blood showed no expression of CD57, high expression of NKG2A, lower expression of killer-immunoglobulin-like receptors (KIRs) and lower secretion of interferon-γ (156, 158). Several studies showed that NK cells can also be activated by HSP70 protein or 14-mer HSP70-derived peptide (TKD) in combination with IL-2 or IL-15 (Figure 2) (137–139). Multhoff and colleagues demonstrated that aggressive tumors have high expression of membrane-bound HSP70 (mHSP70) and that radio and/or- chemotherapy further increases surface expression of HSP70 (159–161). Furthermore, they reported that NK cells pre-activated with TKD and IL-2 recognize mHSP70 on tumor cells (137). Translating this to clinical trial, Multhoff et al. showed that four cycles of adoptive transfer of autologous NK cells pre-stimulated with TKD and IL-2 was well-tolerated and resulted in increase in the number of NK cells in peripheral blood of patients with mHSP70-positive non-small cell lung cancer (NSCLC) following radiochemotherapy in phase II clinical trial (140). Earlier, same research team demonstrated that NK cells activated with IL-2 and TKD and combined with anti-PD-1 antibody increased cytolytic activity of NK cells toward cancer cells and delayed tumor growth in vivo (162).
NK cells that express tumor-specific CARs showed to be efficiently applied in B cell malignancies (156). Currently, six CAR-NK therapies (CD19-CAR NK, CD22-CAR NK, CD19/CD22, CD7-CAR NK,CD19-t-haNK) are assessed in clinical trials for the treatment of lymphoma patients (156). Liu and colleagues used NK cells from the cord blood (CB) for incorporation of genes for CAR-CD19, IL-15 cytokine and caspase 9 as safety switch (iC9/CAR.19/IL15) to efficiently kill CD19-positive leukemia/lymphoma cells lines (156, 163). Same research group further assessed administration of HLA-mismatched iC9/CAR.19/IL15-transduced CB-NK cells in Phase I/II clinical trial to patients with r/r CD19- positive NHL and chronic lymphocytic leukemia (CLL) (164). Overall, 11 patients were administrated with anti-CD19-CAR NK cells, 8 patients (73%) achieved clinical response from which 4 patients with lymphoma and 3 patients with CLL had a complete remission (164). Notably, no neurotoxicity, CRS or increase in IL-6 were observed, which are frequently associated with the administration of CAR T therapies (156, 164).
HSPs and Immune Checkpoints
Immunotherapy in the form of checkpoint modulation has advanced cancer research (165). In 2011, the first monoclonal antibody targeting immune checkpoint cytotoxic T-lymphocyte antigen-4 (CTLA-4) called ipilimumab received FDA approval. Later, monoclonal antibodies that target programmed death 1 (PD-1) such as pembrolizumab and nivolumab, and antibodies against PD-L1 such as atezolizumab and durvalumab were developed (165). Novel combinatorial approaches currently emerge, where the use of immune checkpoint modulators in combination with other anti-cancer therapies may further improve clinical response (166).
Taking into account that a major challenge in immunotherapy is a loss of tumor-associated antigens, HSP90 inhibitors were proposed as complementary approach to checkpoint inhibitors for cancer treatment (167). Rao and colleagues reported that inhibition of HSP90 resulted in proteasome-dependent degradation of its client oncoprotein EphA2 and, hence, increased tumor recognition by EphA2-specific CD8+T lymphocytes (168, 169). Haggerty and colleagues demonstrated that HSP90 inhibitors showed to upregulate the expression of tumor antigens such as Melan-A/MART-1, TPR-2, gp100 and enhance T cell recognition in melanoma cell lines (170). HSP90 client protein nucleophosmin-anaplastic lymphoma kinase (NPM/ALK) showed to induce PD-L1 via STAT3 activation in T cell lymphoma (171). Notably, administration of anti-PD-L1 antibody in combination with HSP90 inhibitor ganetespib showed significantly higher anti-tumor activity than when treated with anti-PD-L1 alone in syngeneic mouse models of melanoma and colon carcinoma (167). Furthermore, Mbofung and colleagues demonstrated that ganetespib in combination with anti-CTLA4 and anti-PD-1 antibodies improved survival and anti-tumor response in mice bearing MC38/gp100 tumors (172). Authors also showed that combinatorial treatment of HSP90 inhibitors and checkpoint blockade therapy decreased number of T regulatory cells and increased the expression of CXCL9, CXCL10 and IFN-γ (172). Combinatorial treatment also increased the number of CD8+T cells producing granzyme A and granzyme B, suggesting that combination of anti-CTLA4 and HSP90 inhibition enhances cytotoxic activity of CD8+T cells (172).
CD47 is an innate immune checkpoint highly expressed on the surface of tumor cells (93, 173). CD47 forms complex with signal regulatory protein α (SIRPα), which is expressed on phagocytic cells such as monocytes, macrophages and dendritic cells (93). CD47/SIRPα sends ‘don’t eat me” signals to innate immune cells, thus, inhibiting phagocytosis (174). Chao and colleagues reported that CD47 was overexpressed on samples derived from patients with various NHL subtypes (DLBCL,B-CLL, MCL, FL, MZL, pre-B ALL) and high CD47 expression correlated with poor clinical prognosis in NHL patients (173, 175). Blocking of CD47/SIRPα with anti-CD47 monoclonal antibody resulted in phagocytosis of acute myeloid leukemia cells (173). Combination of anti-CD47 monoclonal antibody (Hu5F9-G4) and rituximab showed promising results in patients with r/r DLBCL and FL in phase I clinical trial (176). Interestingly, Cook et al. demonstrated that inhibition of glucose-regulated protein-78 (GRP78), a member of HSP70 family, downregulated CD47 expression in tumor cells, leading to enhanced macrophage infiltration (177). Moreover, co-expression of CD47 and GRP78 showed to associate with poor survival in breast cancer patients (177). HSP90 also showed to play a role in CD47 regulation as inactivation of Myc, a client protein of HSP90, resulted in reduced expression of CD47 and PD-L1 (178, 179). It is interesting to note that HSP90 inhibitor PU-H71 induced apoptosis and inhibited tumor growth in patient-derived xenograft model of MCL via downregulating Myc (179, 180). Therefore, further studies are required to understand the HSP90-MYC-CD47/PD-L1 relationship and the role of GRP78 in CD47 regulation for the development of more specific and effective CD47-based therapies.
Discussion
Lymphoma represents a unique group of cancer derived from major effector cells of an immune system such as B cells, T and NK cells (81, 93). Immunotherapy-based approaches showed encouraging results for patients with HL and NHL, however, severe toxicity and low efficacy profiles restrict the use of immunotherapy in lymphoma patients (2, 81). To overcome these limitations, various therapeutic strategies are developed that include the use of HSPs.
HSPs belong to evolutionally conserved family of chaperones that assist client proteins in folding, trafficking, degradation and showed to be involved in the most stages of cancer development (56, 181–184). High expression of HSPs on the surface correlates with the aggressiveness and resistance to therapy in many types of cancer (185, 186). Furthermore, HSPs are largely known for their critical role in regulating cell death mechanisms and immune responses (187–189).
Lymphoma cells create a complex and unique immune-modulatory tumor microenvironment, where inflammatory and stromal cells provide essential signals for growth, proliferation and survival of tumor cells (75, 95, 118). One of the major hallmark of lymphoma is represented by the deregulated critical signaling pathways including NF-kB, JAK-STAT, BCR signaling, PI3K/AKT, MAPK/ERK and apoptosis signaling pathways (82, 85–88, 190).Noticeably, specific members of HSP families, in particular, HSP90 showed to interfere with all these signaling cascades (83, 89, 103). Based on positive results from preclinical studies, several researchers proposed the use of HSP90 inhibitors for the treatment of lymphoma (122). However, result from phase II clinical trial of HSP90 inhibitor revealed low efficacy, but durable response, and acceptable toxicity profile in patients with r/r NHL (122). Taking into account critical role of HSP90 in lymphoma pathogenesis, further studies should be performed to assess the role of HSP90 in different subtypes of HL and NHL lymphomas.
For effective development of HSP-based therapy in lymphoma, it is critical to bear in mind that different HSP members reside in different cellular compartments where they perform specific functions (30, 191, 192). For example, mitochondrial HSP90 homolog known as tumor necrosis factor receptor-associated protein 1 (TRAP1) is involved in mitochondrial bioenergetics while endoplasmic reticulum (ER) HSP90 member referred to as glucose-regulated protein 94 (GRP94/gp96/Endoplasmin/HSP90B1) is critical for the unfolded protein response (193–196). It also appears that cell has some form of a balance of HSP distribution across compartments and cancer seems to impair this equilibrium, leading to the translocation of HSPs, which further reflects their functions (197–199). For example, surface expression of GRP94 showed to increase tumor immunogenicity and stabilize plasma membrane HER2 in breast cancer cells (200–202). So whether HSP-based immunotherapy also affects distribution of HSPs in HL and NHL, shifting HSPs from their primary locations is not yet clear and requires further investigation.
It is also important to point out that HSPs in extracellular milieu exist in several forms either secreted or membrane-bound and each form has distinct function (203–208). For example, dying tumor cells secrete HSP70s that serve as damage-associated molecular patters (DAMPs) and showed to elicit strong T cell response which with long-term exposure leads to the induction of immune tolerance and tumor growth (209–212). Conversely, viable tumor cells export HSP70 in exosomes which showed to activate myeloid-derived suppressor cells (MDSCs) and macrophages for the production of IL-6 and TNF-α, respectively (213, 214). Additionally, HSP70 on the surface of tumor cells serves as a recognition structure for NK cells (199, 215). In light of the reported, extracellular HSP70 activates T regulatory cells leading to the downregulation of interferon-γ and TNF-α section and upregulation of IL-10 and transforming growth factor-β (TGF-β) production (216). Along this line, interaction of extracellular HSP70 with antigen-presenting cells resulted in activation of NF-kB, leading to the production of TNF-α, IL-1β, IL-6 and IL-12 (56, 217–220).Figueiredo and colleagues reported that soluble HSP70 alone or in combination with IL-2 resulted in increased production of IFN-γ by T cells (221). Furthermore, stimulation of T cells with HSP70 and IL-2 or IL-7/IL-12/IL-15 resulted in upregulation of Granzyme B in CD4+T cells in target-independent manner, suggesting that extracellular HSP70 can induce target-independent cytotoxicity in T- helper cells (221). In light of the reported, extracellular HSP110 induce pro-inflammatory phenotype in macrophages (222). Along this line, HSP27-positive tumor –derived exosomes enhance immunosuppressive activity of MDSCs whereas soluble HSP27 induces tolerogenic phenotype in macrophages (223, 224). Furthermore, extracellular HSP27 inhibits differentiation of monocytes to DCs (225). Therefore, taking into account immunologic role of extracellular HSPs, it is important to study their functions in lymphoma pathogenesis and further monitor expression of HSPs in different stages and subtypes of HL and NHL.
Despite their extracellular roles in tumor immunology, HSP members have also distinct intracellular immunologic functions. For example, ER HSP90 homolog GRP94 plays important role in immunosuppressive activity of T regulatory cells, in lymphopoiesis of T and B cells, in production of proinflammatory cytokines by tumor-associated macrophages, in the regulation of platelet GPIbα subunit of GPIb-IX-V complex and maturation of dendritic cells (195, 201, 202, 226–231). Along this line, mitochondrial HSP70 homolog GRP75/mtHSP70/mortalin/HSPA9 showed to interact with complement C9 and protect tumor cell from complement-dependent cytotoxicity (232–234).Furthermore, HSPs regulate an important component of innate immune response- the Nod-like receptor protein-3 (NLRP3) inflammasome (235, 236). Therefore, taking into account that central role in lymphoma pathogenesis play immune evasion mechanisms, intracellular immunologic roles of HSPs should also be considered for the development of more effective and safe HSP-based immunotherapy for the HL and NHL treatment.
HSP chaperones also showed to interact with each other. For example, mortalin interacts with HSP60 and HSP90 whereas GRP94 interacts with binding immunoglobulin protein (BiP/GRP78/HSPA5) (234, 237–239). Furthermore, individual homologs may have specific client networks. For example, ER HSP90 member GRP94 has client network that does not overlap with client network of cytosolic HSP90 homologs (195, 240, 241). Conversely, members from different HSP families may have overlapping client network. For example, HSP110 and HSP90 showed to stabilize c-Myc and Bcl-6 (109, 120, 179). Therefore, it is critical to note that blocking HSP member may further affect its co-chaperones, its client network and other HSP chaperones. Therefore, further studies should explore what happens on the level of individual HSP members in different subtypes of HL and NHL lymphoma and whether specific blocking of a particular homolog and, hence, its client and co-chaperone network, will be more advantageous for the lymphoma treatment.
HSP90 showed to affect CD3 and CD28, thus, the effect of HSP90 on CAR- containing CD3 and CD28-derived domains requires further investigation (133). From the other hand, HSP70-derived peptide TKD has been used for ex vivo activation of NK cells for adoptive transfer therapy and, since no severe toxicity was observed for NK-based immunotherapy, this strategy may be exploited for the development of lymphoma immunotherapy (140). In light of the reported, two research teams proposed to target membrane-bound HSP70 and HSP90 isoforms on tumors by CARs (134, 136).
Evidently, HSP90 via its client network (NPM/ALK and Myc) showed to be involved in the regulation of immune checkpoints such as PD-L1 and CD47 whereas HSP70 ER member GRP78 showed to be co-expressed with CD47 (171, 177–179). Since combination of HSP90 inhibitors with either anti-PD-1, anti-PD-L1 or anti-CTLA-4 antibodies showed anti-tumor effect in mouse models, combinatorial approaches of using HSP90 inhibitors and checkpoint inhibitors or HSP70 inhibitors coupled with anti-CD47 antibodies may further improve anti-tumor response (167, 172).
Another strategy to improve therapy responses involves the use of biomarkers that can predict clinical outcome. Large body of evidence suggests that extracellular HSPs can be used as predictive, prognostic and diagnostic biomarkers of cancer (31, 63, 64, 242–244). Further studies should be performed to assess expression of HSPs in extracellular milieu in their potential to predict clinical response in patients with HL and NHL. Recently, Dunphy and colleagues have conducted phase I clinical trial to test the safety and feasibility of administering 124I-PU-H71 radiologic agent followed by positron emission tomography (PET) to detect HSP90 within epichaperome complex in various types of tumors, thus supporting further development of HSP90-based targeted- therapeutics (245).
Taken together, members of HSP family may be exploited for the development of more efficacious treatment, though, further studies are required to understand the effect of HSP expression in various lymphoma subtypes and their use in the development of T/NK-based immunotherapies and combination approaches. Moreover, the role of HSPs as biomarker to predict clinical outcome in lymphoma patients warrants further investigation.
Conclusion
Lymphoma is a heterogeneous group of cancer, derived from immune cells and characterized into two major subtypes such as Hodgkin and Non-Hodgkin lymphoma. HSP members in particular HSP90, HSP60 and HSP70 are highly expressed in most subtypes of HL and NHL lymphoma. Evidently, HSPs play a major role in hallmarks of lymphoma pathogenesis including their involvement in immune evasion and dysregulation of key signaling cascades. Exploiting HSPs in immunotherapy-based approaches and as biomarkers for the lymphoma therapy may prove effective, however, requires further investigation.
Author Contributions
ZA: conceptualization and manuscript writing. YM: manuscript editing. AS: Resources. All authors contributed to the article and approved the submitted version.
Funding
This work was funded by RFBR, project number 20-315-90081. YM was supported by the RAKFOND grant 2020-02.
Conflict of Interest
The authors declare that the research was conducted in the absence of any commercial or financial relationships that could be construed as a potential conflict of interest.
Acknowledgments
The figures were created with BioRender.com.
References
1. Connors JM, Cozen W, Steidl C, Carbone A, Hoppe RT, Flechtner H-H, et al. Hodgkin lymphoma. Nat Rev Dis Primers (2020) 6(1):61. doi: 10.1038/s41572-020-0189-6
2. Andrew DZ, Leo IG, Jeremy SA, Ranjana HA, Nancy LB, Paolo FC, et al. NCCN Guidelines Insights: B-Cell Lymphomas, Version 3.2019. J Natl Compr Cancer Netw J Natl Compr Canc Netw (2019) 17(6):650–61. doi: 10.6004/jnccn.2019.0029
3. National Comprehensive Cancer Network. NCCN Clinical Practice Guidelines. Hodgkin lymphoma (2019). NCCN. Available at: https://www.nccn.org/professionals/physician_gls/default.aspx#site (Accessed 21 February 2021).
4. Eichenauer DA, Engert A, André M, Federico M, Illidge T, Hutchings M, et al. Hodgkin’s lymphoma: ESMO Clinical Practice Guidelines for diagnosis, treatment and follow-up. Ann Oncol (2014) 25:iii70–5. doi: 10.1093/annonc/mdu181
5. Kuruvilla J, Keating A, Crump M. How I treat relapsed and refractory Hodgkin lymphoma. Blood (2011) 117(16):4208–17. doi: 10.1182/blood-2010-09-288373
6. Linch DC, Goldstone AH, McMillan A, Chopra R, Vaughan Hudson G, Winfield D, et al. Dose intensification with autologous bone-marrow transplantation in relapsed and resistant Hodgkin’s disease: results of a BNLI randomised trial. Lancet (1993) 341(8852):1051–4. doi: 10.1016/0140-6736(93)92411-L
7. Schmitz N, Pfistner B, Sextro M, Sieber M, Carella AM, Haenel M, et al. Aggressive conventional chemotherapy compared with high-dose chemotherapy with autologous haemopoietic stem-cell transplantation for relapsed chemosensitive Hodgkin’s disease: a randomised trial. Lancet (2002) 359(9323):2065–71. doi: 10.1016/S0140-6736(02)08938-9
8. Advani RH, Hoppe RT. How I treat nodular lymphocyte predominant Hodgkin lymphoma. Blood (2013) 122(26):4182–8. doi: 10.1182/blood-2013-07-453241
9. U.S.Food and Drug Administration. FDA expands approval of Adcetris for first-line treatment of Stage III or IV classical Hodgkin lymphoma in combination with chemotherapy (2018). Available at: https://www.fda.gov/news-events/press-announcements/fda-expands-approval-adcetris-first-line-treatment-stage-iii-or-iv-classical-hodgkin-lymphoma (Accessed 21 February 2021).
10. U. S. Food and Drug Administration. Nivolumab (Opdivo) for Hodgkin Lymphoma (2016). Available at: https://www.fda.gov/drugs/resources-information-approved-drugs/nivolumab-opdivo-hodgkin-lymphoma (Accessed 21 Feb 2021).
11. U.S. Food and Drug Administration. FDA extends approval of pembrolizumab for classical Hodgkin lymphoma (2020). Available at: https://www.fda.gov/drugs/drug-approvals-and-databases/fda-extends-approval-pembrolizumab-classical-hodgkin-lymphoma (Accessed 21 February 2021).
12. U.S. Food and Drug Administration. FDA approves first-line treatment for peripheral T-cell lymphoma under new review pilot (2018). Available at: https://www.fda.gov/news-events/press-announcements/fda-approves-first-line-treatment-peripheral-t-cell-lymphoma-under-new-review-pilot (Accessed 21 February 2021).
13. U.S. Food and Drug Administration. FDA approves ibrutinib plus rituximab for chronic lymphocytic leukemia (2020). Available at: https://www.fda.gov/drugs/drug-approvals-and-databases/fda-approves-ibrutinib-plus-rituximab-chronic-lymphocytic-leukemia (Accessed 21 February 2021).
14. Shanafelt TD, Wang XV, Kay NE, Hanson CA, O’Brien S, Barrientos J, et al. Ibrutinib–Rituximab or Chemoimmunotherapy for Chronic Lymphocytic Leukemia. New Engl J Med (2019) 381(5):432–43. doi: 10.1056/NEJMoa1817073
15. U.S. Food and Drug Administration. Project Orbis: FDA approves acalabrutinib for CLL and SLL (2019). Available at: https://www.fda.gov/drugs/resources-information-approved-drugs/project-orbis-fda-approves-acalabrutinib-cll-and-sll (Accessed 21 February 2021).
16. Sharman JP, Egyed M, Jurczak W, Skarbnik A, Pagel JM, Flinn IW, et al. Acalabrutinib with or without obinutuzumab versus chlorambucil and obinutuzumab for treatment-naive chronic lymphocytic leukaemia (ELEVATE-TN): a randomised, controlled, phase 3 trial. Lancet (2020) 395(10232):1278–91. doi: 10.1016/S0140-6736(20)30262-2
17. Ghia P, Pluta A, Wach M, Lysak D, Kozak T, Simkovic M, et al. ASCEND: Phase III, Randomized Trial of Acalabrutinib Versus Idelalisib Plus Rituximab or Bendamustine Plus Rituximab in Relapsed or Refractory Chronic Lymphocytic Leukemia. J Clin Oncol (2020) 38(25):2849–61. doi: 10.1200/JCO.19.03355
18. U.S. Food and Drug Administration. FDA grants accelerated approval to acalabrutinib for mantle cell lymphoma (2017). Available at: https://www.fda.gov/drugs/resources-information-approved-drugs/fda-grants-accelerated-approval-acalabrutinib-mantle-cell-lymphoma (Accessed 21 February 2021).
19. Wang M, Rule S, Zinzani PL, Goy A, Casasnovas O, Smith SD, et al. Durable response with single-agent acalabrutinib in patients with relapsed or refractory mantle cell lymphoma. Leukemia (2019) 33(11):2762–6. doi: 10.1038/s41375-019-0575-9
20. Wang M, Rule S, Zinzani PL, Goy A, Casasnovas O, Smith SD, et al. Acalabrutinib in relapsed or refractory mantle cell lymphoma (ACE-LY-004): a single-arm, multicentre, phase 2 trial. Lancet (2018) 391(10121):659–67. doi: 10.1016/S0140-6736(17)33108-2
21. Miller BW, Przepiorka D, de Claro RA, Lee K, Nie L, Simpson N, et al. FDA Approval: Idelalisib Monotherapy for the Treatment of Patients with Follicular Lymphoma and Small Lymphocytic Lymphoma. Clin Cancer Res (2015) 21(7):1525. doi: 10.1158/1078-0432.CCR-14-2522
22. U.S.Food and Drug Administration. FDA grants accelerated approval to copanlisib for relapsed follicular lymphoma (2017). Available at: https://www.fda.gov/drugs/resources-information-approved-drugs/fda-grants-accelerated-approval-copanlisib-relapsed-follicular-lymphoma (Accessed 21 February 2021).
23. U.S.Food and Drug Administration. duvelisib (COPIKTRA, Verastem, Inc.) for adult patients with relapsed or refractory chronic lymphocytic leukemia (CLL) or small lymphocytic lymphoma (SLL) (2018). Available at: https://www.fda.gov/drugs/resources-information-approved-drugs/duvelisib-copiktra-verastem-inc-adult-patients-relapsed-or-refractory-chronic-lymphocytic-leukemia (Accessed 21 February 2021).
24. U.S. Food and Drug Administration. FDA approves pembrolizumab for treatment of relapsed or refractory PMBCL (2018). Available at: https://www.fda.gov/drugs/resources-information-approved-drugs/fda-approves-pembrolizumab-treatment-relapsed-or-refractory-pmbcl (Accessed 21 Februrary 2021).
25. Zinzani PL, Thieblemont C, Melnichenko V, Bouabdallah K, Walewski J, Majlis A, et al. Efficacy and Safety of Pembrolizumab in Relapsed/Refractory Primary Mediastinal Large B-Cell Lymphoma (rrPMBCL): Updated Analysis of the Keynote-170 Phase 2 Trial. Blood (2017) 130 (Supplement1):2833–3. doi: 10.1182/blood.V130.Suppl_1.2833.2833
26. U.S.Food and Drug Administration. FDA approves tisagenlecleucel for adults with relapsed or refractory large B-cell lymphoma (2018). Available at: https://www.fda.gov/drugs/resources-information-approved-drugs/fda-approves-tisagenlecleucel-adults-relapsed-or-refractory-large-b-cell-lymphoma (Accessed 21 February 2021).
27. Locke FL, Ghobadi A, Jacobson CA, Miklos DB, Lekakis LJ, Oluwole OO, et al. Long-term safety and activity of axicabtagene ciloleucel in refractory large B-cell lymphoma (ZUMA-1): a single-arm, multicentre, phase 1-2 trial. Lancet Oncol (2019) 20(1):31–42. doi: 10.1016/S1470-2045(18)30864-7
28. U.S.Food and Drug Administration. FDA approves axicabtagene ciloleucel for large B-cell lymphoma (2017). Available at: https://www.fda.gov/drugs/resources-information-approved-drugs/fda-approves-axicabtagene-ciloleucel-large-b-cell-lymphoma (Accessed 21 February 2021).
29. Chaudhari K, Rizvi S, Syed B. Non-Hodgkin lymphoma therapy landscape. Nat Rev Drug Discovery (2019) 18:663–4. doi: 10.1038/d41573-019-00051-6
30. Kampinga HH, Hageman J, Vos MJ, Kubota H, Tanguay RM, Bruford EA, et al. Guidelines for the nomenclature of the human heat shock proteins. Cell Stress Chaperones (2009) 14(1):105–11. doi: 10.1007/s12192-008-0068-7
31. Albakova Z, Siam MKS, Sacitharan PK, Ziganshin RH, Ryazantsev DY, Sapozhnikov AM. Extracellular heat shock proteins and cancer: New perspectives. Trans Oncol (2021) 14(2):100995. doi: 10.1016/j.tranon.2020.100995
32. Antonoff MB, Chugh R, Skube SJ, Dudeja V, Borja-Cacho D, Clawson KA, et al. Role of Hsp-70 in triptolide-mediated cell death of neuroblastoma. J Surg Res (2010) 163(1):72–8. doi: 10.1016/j.jss.2010.04.047
33. Braunstein MJ, Scott SS, Scott CM, Behrman S, Walter P, Wipf P, et al. Antimyeloma Effects of the Heat Shock Protein 70 Molecular Chaperone Inhibitor MAL3-101. J Oncol (2011) 2011:232037. doi: 10.1155/2011/232037
34. Ernst K, Liebscher M, Mathea S, Granzhan A, Schmid J, Popoff MR, et al. A novel Hsp70 inhibitor prevents cell intoxication with the actin ADP-ribosylating Clostridium perfringens iota toxin. Sci Rep (2016) 6(1):20301. doi: 10.1038/srep20301
35. Fewell SW, Smith CM, Lyon MA, Dumitrescu TP, Wipf P, Day BW, et al. Small molecule modulators of endogenous and co-chaperone-stimulated Hsp70 ATPase activity. J Biol Chem (2004) 279(49):51131–40. doi: 10.1074/jbc.M404857200
36. Howe MK, Bodoor K, Carlson DA, Hughes PF, Alwarawrah Y, Loiselle DR, et al. Identification of an allosteric small-molecule inhibitor selective for the inducible form of heat shock protein 70. Chem Biol (2014) 21(12):1648–59. doi: 10.1016/j.chembiol.2014.10.016
37. Hung C-M, Su Y-H, Lin H-Y, Lin J-N, Liu L-C, Ho C-T, et al. Demethoxycurcumin Modulates Prostate Cancer Cell Proliferation via AMPK-Induced Down-regulation of HSP70 and EGFR. J Agric Food Chem (2012) 60(34):8427–34. doi: 10.1021/jf302754w
38. Huryn DM, Brodsky JL, Brummond KM, Chambers PG, Eyer B, Ireland AW, et al. Chemical methodology as a source of small-molecule checkpoint inhibitors and heat shock protein 70 (Hsp70) modulators. Proc Natl Acad Sci U.S.A. (2011) 108(17):6757–62. doi: 10.1073/pnas.1015251108
39. Jacobson BA, Chen EZ, Tang S, Belgum HS, McCauley JA, Evenson KA, et al. Triptolide and its prodrug minnelide suppress Hsp70 and inhibit in vivo growth in a xenograft model of mesothelioma. Genes Cancer (2015) 6(3-4):144–52. doi: 10.18632/genesandcancer.55
40. Jung JH, Lee JO, Kim JH, Lee SK, You GY, Park SH, et al. Quercetin suppresses HeLa cell viability via AMPK-induced HSP70 and EGFR down-regulation. J Cell Physiol (2010) 223(2):408–14. doi: 10.1002/jcp.22049
41. Ko S-K, Kim J, Na Deuk C, Park S, Park S-H, Hyun Ji Y, et al. A Small Molecule Inhibitor of ATPase Activity of HSP70 Induces Apoptosis and Has Antitumor Activities. Chem Biol (2015) 22(3):391–403. doi: 10.1016/j.chembiol.2015.02.004
42. Leu JI, Pimkina J, Frank A, Murphy ME, George DL. A small molecule inhibitor of inducible heat shock protein 70. Mol Cell (2009) 36(1):15–27. doi: 10.1016/j.molcel.2009.09.023
43. Phillips PA, Sangwan V, Borja-Cacho D, Dudeja V, Vickers SM, Saluja AK. Myricetin induces pancreatic cancer cell death via the induction of apoptosis and inhibition of the phosphatidylinositol 3-kinase (PI3K) signaling pathway. Cancer Lett (2011) 308(2):181–8. doi: 10.1016/j.canlet.2011.05.002
44. Rérole A-L, Gobbo J, De Thonel A, Schmitt E, Pais de Barros JP, Hammann A, et al. Peptides and Aptamers Targeting HSP70: A Novel Approach for Anticancer Chemotherapy. Cancer Res (2011) 71(2):484. doi: 10.1158/0008-5472.CAN-10-1443
45. Rodina A, Patel PD, Kang Y, Patel Y, Baaklini I, Wong MJ, et al. Identification of an allosteric pocket on human hsp70 reveals a mode of inhibition of this therapeutically important protein. Chem Biol (2013) 20(12):1469–80. doi: 10.1016/j.chembiol.2013.10.008
46. Rousalova I, Banerjee S, Sangwan V, Evenson K, McCauley JA, Kratzke R, et al. Minnelide: a novel therapeutic that promotes apoptosis in non-small cell lung carcinoma in vivo. PloS One (2013) 8(10):e77411. doi: 10.1371/journal.pone.0077411
47. Schmitt E, Maingret L, Puig PE, Rerole AL, Ghiringhelli F, Hammann A, et al. Heat shock protein 70 neutralization exerts potent antitumor effects in animal models of colon cancer and melanoma. Cancer Res (2006) 66(8):4191–7. doi: 10.1158/0008-5472.Can-05-3778
48. Williams DR, Ko S-K, Park S, Lee M-R, Shin I. An Apoptosis-Inducing Small Molecule That Binds to Heat Shock Protein 70. Angewandte Chemie Int Edition (2008) 47(39):7466–9. doi: 10.1002/anie.200802801
49. Speranza G, Anderson L, Chen AP, Do K, Eugeni M, Weil M, et al. First-in-human study of the epichaperome inhibitor PU-H71: clinical results and metabolic profile. Invest New Drugs (2018) 36(2):230–9. doi: 10.1007/s10637-017-0495-3
50. Kamal A, Thao L, Sensintaffar J, Zhang L, Boehm MF, Fritz LC, et al. A high-affinity conformation of Hsp90 confers tumour selectivity on Hsp90 inhibitors. Nature (2003) 425(6956):407–10. doi: 10.1038/nature01913
51. Udono H, Srivastava PK. Comparison of tumor-specific immunogenicities of stress-induced proteins gp96, hsp90, and hsp70. J Immunol (1994) 152(11):5398.
52. Srivastava P. Interaction of heat shock proteins with peptides and antigen presenting cells: chaperoning of the innate and adaptive immune responses. Annu Rev Immunol (2002) 20:395–425. doi: 10.1146/annurev.immunol.20.100301.064801
53. Blachere NE, Li Z, Chandawarkar RY, Suto R, Jaikaria NS, Basu S, et al. Heat shock protein-peptide complexes, reconstituted in vitro, elicit peptide-specific cytotoxic T lymphocyte response and tumor immunity. J Exp Med (1997) 186(8):1315–22. doi: 10.1084/jem.186.8.1315
54. Weng D, Calderwood SK, Gong J. Preparation of a heat-shock protein 70-based vaccine from DC-tumor fusion cells. Methods Mol Biol (2011) 787:255–65. doi: 10.1007/978-1-61779-295-3_19
55. Li X, Cai X, Zhang Z, Ding Y, Ma R, Huang F, et al. Mimetic Heat Shock Protein Mediated Immune Process to Enhance Cancer Immunotherapy. Nano Lett (2020) 20(6):4454–63. doi: 10.1021/acs.nanolett.0c01230
56. Albakova Z, Armeev GA, Kanevskiy LM, Kovalenko EI, Sapozhnikov AM. HSP70 Multi-Functionality in Cancer. Cells (2020) 9:(3). doi: 10.3390/cells9030587
57. Milani V, Stangl S, Issels R, Gehrmann M, Wagner B, Hube K, et al. Anti-tumor activity of patient-derived NK cells after cell-based immunotherapy–a case report. J Transl Med (2009) 7:50. doi: 10.1186/1479-5876-7-50
58. Krause SW, Gastpar R, Andreesen R, Gross C, Ullrich H, Thonigs G, et al. Treatment of colon and lung cancer patients with ex vivo heat shock protein 70-peptide-activated, autologous natural killer cells: a clinical phase i trial. Clin Cancer Res (2004) 10(11):3699–707. doi: 10.1158/1078-0432.Ccr-03-0683
59. Maeda Y, Yoshimura K, Matsui H, Shindo Y, Tamesa T, Tokumitsu Y, et al. Dendritic cells transfected with heat-shock protein 70 messenger RNA for patients with hepatitis C virus-related hepatocellular carcinoma: a phase 1 dose escalation clinical trial. Cancer Immunol Immunother (2015) 64(8):1047–56. doi: 10.1007/s00262-015-1709-1
60. Kokowski K, Stangl S, Seier S, Hildebrandt M, Vaupel P, Multhoff G. Radiochemotherapy combined with NK cell transfer followed by second-line PD-1 inhibition in a patient with NSCLC stage IIIb inducing long-term tumor control: a case study. Strahlenther Onkol (2019) 195(4):352–61. doi: 10.1007/s00066-019-01434-9
61. Specht HM, Ahrens N, Blankenstein C, Duell T, Fietkau R, Gaipl US, et al. Heat Shock Protein 70 (Hsp70) Peptide Activated Natural Killer (NK) Cells for the Treatment of Patients with Non-Small Cell Lung Cancer (NSCLC) after Radiochemotherapy (RCTx) - From Preclinical Studies to a Clinical Phase II Trial. Front Immunol (2015) 6:162. doi: 10.3389/fimmu.2015.00162
62. Oki Y, McLaughlin P, Fayad LE, Pro B, Mansfield PF, Clayman GL, et al. Experience with heat shock protein-peptide complex 96 vaccine therapy in patients with indolent non-Hodgkin lymphoma. Cancer (2007) 109(1):77–83. doi: 10.1002/cncr.22389
63. Balázs M, Zsolt H, László G, Gabriella G, Lilla T, Gyula O, et al. Serum Heat Shock Protein 70, as a Potential Biomarker for Small Cell Lung Cancer. Pathol Oncol Res (2017) 23(2):377–83. doi: 10.1007/s12253-016-0118-x
64. Chanteloup G, Cordonnier M, Isambert N, Bertaut A, Hervieu A, Hennequin A, et al. Monitoring HSP70 exosomes in cancer patients’ follow up: a clinical prospective pilot study. J Extracell Vesicles (2020) 9(1):1766192–1766192. doi: 10.1080/20013078.2020.1766192
65. Gehrmann M, Specht HM, Bayer C, Brandstetter M, Chizzali B, Duma M, et al. Hsp70–a biomarker for tumor detection and monitoring of outcome of radiation therapy in patients with squamous cell carcinoma of the head and neck. Radiat Oncol (London England) (2014) 9:131–1. doi: 10.1186/1748-717X-9-131
66. Rong B, Zhao C, Liu H, Ming Z, Cai X, Gao W, et al. Erratum: Identification and verification of Hsp90-beta as a potential serum biomarker for lung cancer. Am J Cancer Res (2016) 6(6):1460–0.
67. Rong B, Zhao C, Liu H, Ming Z, Cai X, Gao W, et al. Identification and verification of Hsp90-beta as a potential serum biomarker for lung cancer. Am J Cancer Res (2014) 4(6):874–85.
68. Tas F, Bilgin E, Erturk K, Duranyildiz D. Clinical Significance of Circulating Serum Cellular Heat Shock Protein 90 (HSP90) Level in Patients with Cutaneous Malignant Melanoma. Asian Pac J Cancer Prev (2017) 18(3):599–601. doi: 10.22034/APJCP.2017.18.3.599
69. Wyciszkiewicz A, Kalinowska-Łyszczarz A, Nowakowski B, Kaźmierczak K, Osztynowicz K, Michalak S. Expression of small heat shock proteins in exosomes from patients with gynecologic cancers. Sci Rep (2019) 9(1):9817. doi: 10.1038/s41598-019-46221-9
70. Bodzek P, Partyka R, Damasiewicz-Bodzek A. Antibodies against Hsp60 and Hsp65 in the sera of women with ovarian cancer. J Ovarian Res (2014) 7:30–0. doi: 10.1186/1757-2215-7-30
71. Hallal S, Russell BP, Wei H, Lee MYT, Toon CW, Sy J, et al. Extracellular Vesicles from Neurosurgical Aspirates Identifies Chaperonin Containing TCP1 Subunit 6A as a Potential Glioblastoma Biomarker with Prognostic Significance. PROTEOMICS (2019) 19(1-2):1800157. doi: 10.1002/pmic.201800157
72. Steidl C. The ecosystem of classical Hodgkin lymphoma. Blood (2017) 130(22):2360–1. doi: 10.1182/blood-2017-10-807172
73. Chiu A, Xu W, He B, Dillon SR, Gross JA, Sievers E, et al. Hodgkin lymphoma cells express TACI and BCMA receptors and generate survival and proliferation signals in response to BAFF and APRIL. Blood (2007) 109(2):729–39. doi: 10.1182/blood-2006-04-015958
74. Küppers R, Hansmann M-L. The Hodgkin and Reed/Sternberg cell. Int J Biochem Cell Biol (2005) 37(3):511–7. doi: 10.1016/j.biocel.2003.10.025
75. Aldinucci D, Celegato M, Casagrande N. Microenvironmental interactions in classical Hodgkin lymphoma and their role in promoting tumor growth, immune escape and drug resistance. Cancer Lett (2016) 380(1):243–52. doi: 10.1016/j.canlet.2015.10.007
76. Chiu J, Ernst DM, Keating A. Acquired Natural Killer Cell Dysfunction in the Tumor Microenvironment of Classic Hodgkin Lymphoma. Front Immunol (2018) 9:267. doi: 10.3389/fimmu.2018.00267
77. Metkar SS, Naresh KN, Redkar AA, Soman CS, Advani SH, Nadkarni JJ. Expression of Fas and Fas Ligand in Hodgkin’s Disease. Leukemia Lymphoma (1999) 33(5-6):521–30. doi: 10.3109/10428199909058456
78. Verbeke CS, Wenthe U, Grobholz R, Zentgraf H. Fas Ligand Expression in Hodgkin Lymphoma. Am J Surg Pathol (2001) 25:(3). doi: 10.1097/00000478-200103000-00014
79. Santón A, García-Cosío M, Cristóbal E, Pascual A, Muriel A, García-Laraña J. Expression of heat shock proteins in classical Hodgkin lymphoma: correlation with apoptotic pathways and prognostic significance. Histopathology (2011) 58(7):1072–80. doi: 10.1111/j.1365-2559.2011.03803.x
80. Hsu P-L, Hsu S-M. Abundance of Heat Shock Proteins (hsp89, hsp60, and hsp27) in Malignant Cells of Hodgkin's Disease. Cancer Res (1998) 58(23):5507.
81. Küppers R, Engert A, Hansmann M-L. Hodgkin lymphoma. J Clin Invest (2012) 122(10):3439–47. doi: 10.1172/JCI61245
82. Bargou RC, Emmerich F, Krappmann D, Bommert K, Mapara MY, Arnold W, et al. Constitutive nuclear factor-kappaB-RelA activation is required for proliferation and survival of Hodgkin’s disease tumor cells. J Clin Invest (1997) 100(12):2961–9. doi: 10.1172/JCI119849
83. Broemer M, Krappmann D, Scheidereit C. Requirement of Hsp90 activity for IκB kinase (IKK) biosynthesis and for constitutive and inducible IKK and NF-κB activation. Oncogene (2004) 23(31):5378–86. doi: 10.1038/sj.onc.1207705
84. Schoof N, von Bonin F, Trümper L, Kube D. HSP90 is essential for Jak-STAT signaling in classical Hodgkin lymphoma cells. Cell Commun Signal (2009) 7(1):17. doi: 10.1186/1478-811X-7-17
85. Holtick U, Vockerodt M, Pinkert D, Schoof N, Stürzenhofecker B, Kussebi N, et al. STAT3 is essential for Hodgkin lymphoma cell proliferation and is a target of tyrphostin AG17 which confers sensitization for apoptosis. Leukemia (2005) 19(6):936–44. doi: 10.1038/sj.leu.2403750
86. Skinnider BF, Elia AJ, Gascoyne RD, Patterson B, Trumper L, Kapp U, et al. Signal transducer and activator of transcription 6 is frequently activated in Hodgkin and Reed-Sternberg cells of Hodgkin lymphoma. Blood (2002) 99(2):618–26. doi: 10.1182/blood.V99.2.618
87. Renné C, Willenbrock K, K̈ppers R, Hansmann M-L, Bräuninger A. Autocrine- and paracrine-activated receptor tyrosine kinases in classic Hodgkin lymphoma. Blood (2005) 105(10):4051–9. doi: 10.1182/blood-2004-10-4008
88. Zheng B, Fiumara P, Li YV, Georgakis G, Snell V, Younes M, et al. MEK/ERK pathway is aberrantly active in Hodgkin disease: a signaling pathway shared by CD30, CD40, and RANK that regulates cell proliferation and survival. Blood (2003) 102(3):1019–27. doi: 10.1182/blood-2002-11-3507
89. Georgakis GV, Li Y, Rassidakis GZ, Martinez-Valdez H, Medeiros LJ, Younes A. Inhibition of Heat Shock Protein 90 Function by 17-Allylamino-17-Demethoxy-Geldanamycin in Hodgkin's Lymphoma Cells Down-Regulates Akt Kinase, Dephosphorylates Extracellular Signal–Regulated Kinase, and Induces Cell Cycle Arrest and Cell Death. Clin Cancer Res (2006) 12(2):584. doi: 10.1158/1078-0432.CCR-05-1194
90. Segges P, Corrêa S, Du Rocher B, Vera-Lozada G, Krsticevic F, Arce D, et al. Targeting Hodgkin and Reed–Sternberg Cells with an Inhibitor of Heat-Shock Protein 90: Molecular Pathways of Response and Potential Mechanisms of Resistance. Int J Mol Sci (2018) 19(3):836. doi: 10.3390/ijms19030836
91. Janz M, Stühmer T, Vassilev LT, Bargou RC. Pharmacologic activation of p53-dependent and p53-independent apoptotic pathways in Hodgkin/Reed-Sternberg cells. Leukemia (2007) 21(4):772–9. doi: 10.1038/sj.leu.2404565
92. Jin H, Yoshino T, Jin Z, Oka T, Kobayashi K, Yamasaki R, et al. Expression of Heat Shock Protein 60 in Normal and Neoplastic Human Lymphoid Tissues. J Clin Exp Hematopathol (2002) 42:25–32. doi: 10.3960/jslrt.42.25
93. Wang L, Qin W, Huo Y-J, Li X, Shi Q, Rasko JEJ, et al. Advances in targeted therapy for malignant lymphoma. Signal Transduct Target Ther (2020) 5(1):15. doi: 10.1038/s41392-020-0113-2
94. Armengol M, Santos JC, Fernández-Serrano M, Profitós-Pelejà N, Ribeiro ML, Roué G. Immune-Checkpoint Inhibitors in B-Cell Lymphoma. Cancers (Basel) (2021) 13(2):214. doi: 10.3390/cancers13020214
95. Scott DW, Gascoyne RD. The tumour microenvironment in B cell lymphomas. Nat Rev Cancer (2014) 14(8):517–34. doi: 10.1038/nrc3774
96. Lam K-P, Kühn R, Rajewsky K. In Vivo Ablation of Surface Immunoglobulin on Mature B Cells by Inducible Gene Targeting Results in Rapid Cell Death. Cell (1997) 90(6):1073–83. doi: 10.1016/S0092-8674(00)80373-6
97. Myklebust JH, Brody J, Kohrt HE, Kolstad A, Czerwinski DK, Wälchli S, et al. Distinct patterns of B-cell receptor signaling in non-Hodgkin lymphomas identified by single-cell profiling. Blood (2017) 129(6):759–70. doi: 10.1182/blood-2016-05-718494
98. Cha S-C, Qin H, Kannan S, Rawal S, Watkins LS, Baio FE, et al. Nonstereotyped lymphoma B cell receptors recognize vimentin as a shared autoantigen. J Immunol (Baltimore Md 1950) (2013) 190(9):4887–98. doi: 10.4049/jimmunol.1300179
99. Young RM, Wu T, Schmitz R, Dawood M, Xiao W, Phelan JD, et al. Survival of human lymphoma cells requires B-cell receptor engagement by self-antigens. Proc Natl Acad Sci U S A (2015) 112(44):13447–54. doi: 10.1073/pnas.1514944112
100. Compagno M, Lim WK, Grunn A, Nandula SV, Brahmachary M, Shen Q, et al. Mutations of multiple genes cause deregulation of NF-kappaB in diffuse large B-cell lymphoma. Nature (2009) 459(7247):717–21. doi: 10.1038/nature07968
101. Lenz G, Davis RE, Ngo VN, Lam L, George TC, Wright GW, et al. Oncogenic <em<CARD11</em< Mutations in Human Diffuse Large B Cell Lymphoma. Science (2008) 319(5870):1676. doi: 10.1126/science.1153629
102. Davis RE, Ngo VN, Lenz G, Tolar P, Young RM, Romesser PB, et al. Chronic active B-cell-receptor signalling in diffuse large B-cell lymphoma. Nature (2010) 463(7277):88–92. doi: 10.1038/nature08638
103. Walter R, Pan K-T, Doebele C, Comoglio F, Tomska K, Bohnenberger H, et al. HSP90 promotes Burkitt lymphoma cell survival by maintaining tonic B-cell receptor signaling. Blood (2017) 129(5):598–608. doi: 10.1182/blood-2016-06-721423
104. ten Hacken E, Burger JA. HSP90, a chaperone that can make you SYK. Blood (2017) 129(5):542–4. doi: 10.1182/blood-2016-12-753152
105. Guo A, Lu P, Lee J, Zhen C, Chiosis G, Wang YL. HSP90 stabilizes B-cell receptor kinases in a multi-client interactome: PU-H71 induces CLL apoptosis in a cytoprotective microenvironment. Oncogene (2017) 36(24):3441–9. doi: 10.1038/onc.2016.494
106. Jacobson C, Kopp N, Layer JV, Redd RA, Tschuri S, Haebe S, et al. HSP90 inhibition overcomes ibrutinib resistance in mantle cell lymphoma. Blood (2016) 128(21):2517–26. doi: 10.1182/blood-2016-04-711176
107. Woyach JA, Furman RR, Liu T-M, Ozer HG, Zapatka M, Ruppert AS, et al. Resistance Mechanisms for the Bruton’s Tyrosine Kinase Inhibitor Ibrutinib. New Engl J Med (2014) 370(24):2286–94. doi: 10.1056/NEJMoa1400029
108. Furman RR, Cheng S, Lu P, Setty M, Perez AR, Guo A, et al. Ibrutinib resistance in chronic lymphocytic leukemia. N Engl J Med (2014) 370(24):2352–4. doi: 10.1056/NEJMc1402716
109. Cerchietti LC, Lopes EC, Yang SN, Hatzi K, Bunting KL, Tsikitas LA, et al. A purine scaffold Hsp90 inhibitor destabilizes BCL-6 and has specific antitumor activity in BCL-6-dependent B cell lymphomas. Nat Med (2009) 15(12):1369–76. doi: 10.1038/nm.2059
110. Goldstein RL, Yang SN, Taldone T, Chang B, Gerecitano J, Elenitoba-Johnson K, et al. Pharmacoproteomics identifies combinatorial therapy targets for diffuse large B cell lymphoma. J Clin Invest (2015) 125(12):4559–71. doi: 10.1172/JCI80714
111. Umetsu DT, Esserman L, Donlon TA, DeKruyff RH, Levy R. Induction of proliferation of human follicular (B type) lymphoma cells by cognate interaction with CD4+ T cell clones. J Immunol (1990) 144(7):2550.
112. Calvo KR, Dabir B, Kovach A, Devor C, Bandle R, Bond A, et al. IL-4 protein expression and basal activation of Erk in vivo in follicular lymphoma. Blood (2008) 112(9):3818–26. doi: 10.1182/blood-2008-02-138933
113. Johnson PWM, Watt SM, Betts DR, Davies D, Jordan S, Norton AJ, et al. Isolated Follicular Lymphoma Cells Are Resistant to Apoptosis and Can Be Grown In Vitro in the CD40/Stromal Cell System. Blood (1993) 82(6):1848–57. doi: 10.1182/blood.V82.6.1848.1848
114. Munari F, Lonardi S, Cassatella MA, Doglioni C, Cangi MG, Amedei A, et al. Tumor-associated macrophages as major source of APRIL in gastric MALT lymphoma. Blood (2011) 117(24):6612–6. doi: 10.1182/blood-2010-06-293266
115. Schwaller J, Schneider P, Mhawech-Fauceglia P, McKee T, Myit S, Matthes T, et al. Neutrophil-derived APRIL concentrated in tumor lesions by proteoglycans correlates with human B-cell lymphoma aggressiveness. Blood (2006) 109(1):331–8. doi: 10.1182/blood-2006-02-001800
116. Schiemann B, Gommerman JL, Vora K, Cachero TG, Shulga-Morskaya S, Dobles M, et al. An Essential Role for BAFF in the Normal Development of B Cells Through a BCMA-Independent Pathway. Science (2001) 293(5537):2111. doi: 10.1126/science.1061964
117. Ogden CA, Pound JD, Batth BK, Owens S, Johannessen I, Wood K, et al. Enhanced Apoptotic Cell Clearance Capacity and B Cell Survival Factor Production by IL-10-Activated Macrophages: Implications for Burkitt’s Lymphoma. J Immunol (2005) 174(5):3015. doi: 10.4049/jimmunol.174.5.3015
118. Mourcin F, Pangault C, Amin-Ali R, Amé-Thomas P, Tarte K. Stromal cell contribution to human follicular lymphoma pathogenesis. Front Immunol (2012) 3:280. doi: 10.3389/fimmu.2012.00280
119. Valbuena JR, Rassidakis GZ, Lin P, Atwell C, Georgakis GV, Younes A, et al. Expression of heat-shock protein-90 in non-Hodgkin’s lymphomas. Modern Pathol (2005) 18(10):1343–9. doi: 10.1038/modpathol.3800459
120. Zappasodi R, Ruggiero G, Guarnotta C, Tortoreto M, Tringali C, Cavanè A, et al. HSPH1 inhibition downregulates Bcl-6 and c-Myc and hampers the growth of human aggressive B-cell non-Hodgkin lymphoma. Blood (2015) 125(11):1768–71. doi: 10.1182/blood-2014-07-590034
121. Boudesco C, Verhoeyen E, Martin L, Chassagne-Clement C, Salmi L, Mhaidly R, et al. HSP110 sustains chronic NF-κB signaling in activated B-cell diffuse large B-cell lymphoma through MyD88 stabilization. Blood (2018) 132(5):510–20. doi: 10.1182/blood-2017-12-819706
122. Yasuhiro O, Anas Y, Javier K, Felipe S, Loretta N, Fredrick H, et al. Experience with HSP90 inhibitor AUY922 in patients with relapsed or refractory non-Hodgkin lymphoma. Haematologica (2015) 100(7):e272–4. doi: 10.3324/haematol.2015.126557
123. Walsby EJ, Lazenby M, Pepper CJ, Knapper S, Burnett AK. The HSP90 inhibitor NVP-AUY922-AG inhibits the PI3K and IKK signalling pathways and synergizes with cytarabine in acute myeloid leukaemia cells. Br J Haematol (2013) 161(1):57–67. doi: 10.1111/bjh.12215
124. Best OG, Mulligan SP. Heat shock protein-90 inhibitor, NVP-AUY922, is effective in combination with fludarabine against chronic lymphocytic leukemia cells cultured on CD40L-stromal layer and inhibits their activated/proliferative phenotype. Leukemia Lymphoma (2012) 53(11):2314–20. doi: 10.3109/10428194.2012.698278
125. Kaiser M, Lamottke B, Mieth M, Jensen MR, Quadt C, Garcia-Echeverria C, et al. Synergistic action of the novel HSP90 inhibitor NVP-AUY922 with histone deacetylase inhibitors, melphalan, or doxorubicin in multiple myeloma. Eur J Haematol (2010) 84(4):337–44. doi: 10.1111/j.1600-0609.2009.01403.x
126. Rao R, Fiskus W, Yang Y, Lee P, Joshi R, Fernandez P, et al. HDAC6 inhibition enhances 17-AAG–mediated abrogation of hsp90 chaperone function in human leukemia cells. Blood (2008) 112(5):1886–93. doi: 10.1182/blood-2008-03-143644
127. Chavez JC, Bachmeier C, Kharfan-Dabaja MA. CAR T-cell therapy for B-cell lymphomas: clinical trial results of available products. Ther Adv Hematol (2019) 10:2040620719841581–2040620719841581. doi: 10.1177/2040620719841581
128. Rafiq S, Hackett CS, Brentjens RJ. Engineering strategies to overcome the current roadblocks in CAR T cell therapy. Nat Rev Clin Oncol (2020) 17(3):147–67. doi: 10.1038/s41571-019-0297-y
129. Halim L, Maher J. CAR T-cell immunotherapy of B-cell malignancy: the story so far. Ther Adv Vaccines Immunother (2020) 8:2515135520927164–2515135520927164. doi: 10.1177/2515135520927164
130. Kawalekar OU, O’Connor RS, Fraietta JA, Guo L, McGettigan SE, Posey AD Jr, et al. Distinct Signaling of Coreceptors Regulates Specific Metabolism Pathways and Impacts Memory Development in CAR T Cells. Immunity (2016) 44(2):380–90. doi: 10.1016/j.immuni.2016.01.021
131. Ramos CA, Grover NS, Beaven AW, Lulla PD, Wu M-F, Ivanova A, et al. Anti-CD30 CAR-T Cell Therapy in Relapsed and Refractory Hodgkin Lymphoma. J Clin Oncol (2020) 38(32):3794–804. doi: 10.1200/jco.20.01342
132. Wang D, Zeng C, Xu B, Xu JH, Wang J, Jiang LJ, et al. Anti-CD30 chimeric antigen receptor T cell therapy for relapsed/refractory CD30+ lymphoma patients. Blood Cancer J (2020) 10(1):8. doi: 10.1038/s41408-020-0274-9
133. Watanabe M, Nakano K, Kadin ME, Higashihara M, Watanabe T, Horie R. CD30 Induces Heat Shock Protein 90 and Signal Integration in Classic Hodgkin Lymphoma Cells. Am J Pathol (2017) 187(1):163–75. doi: 10.1016/j.ajpath.2016.09.007
134. Smith J VJ, Juillerat A, Duchateau P, Sasu BJ, Rajpal A. Anti-hsp70 specific chimeric antigen receptors (CARs) for cancer immunotherapy. U.S. Patent and Trademark Office (2016), US Patent. Available at: https://patentscope.wipo.int/search/en/detail.jsf?docId=US209520457
135. Devarakonda CV, Kita D, Phoenix KN, Claffey KP. Patient-derived heavy chain antibody targets cell surface HSP90 on breast tumors. BMC Cancer (2015) 15:614–4. doi: 10.1186/s12885-015-1608-z
136. Claffey KP, Devarakonda C, Kita D. ANTIBODY AND ANTIGEN-BINDING FRAGMENT COMPOSITIONS TARGETING CELL SURFACE ANTIGENS IN TUMORS AND METHODS OF USE THEREOF. U.S. Patent and Trademark Office. (2019) Available at: https://patentscope.wipo.int/search/en/detail.jsf?docId=US278926324&docAn=16533937
137. Multhoff G, Pfister K, Gehrmann M, Hantschel M, Gross C, Hafner M, et al. A 14-mer Hsp70 peptide stimulates natural killer (NK) cell activity. Cell Stress Chaperones (2001) 6(4):337–44. doi: 10.1379/1466-1268(2001)006<0337:AMHPSN>2.0.CO;2
138. Hromadnikova I, Li S, Kotlabova K, Dickinson AM. Influence of In Vitro IL-2 or IL-15 Alone or in Combination with Hsp 70 Derived 14-Mer Peptide (TKD) on the Expression of NK Cell Activatory and Inhibitory Receptors on Peripheral Blood T Cells, B Cells and NKT Cells. PloS One (2016) 11(3):e0151535–e0151535. doi: 10.1371/journal.pone.0151535
139. Stangl S, Gross C, Pockley AG, Asea AA, Multhoff G. Influence of Hsp70 and HLA-E on the killing of leukemic blasts by cytokine/Hsp70 peptide-activated human natural killer (NK) cells. Cell Stress Chaperones (2008) 13(2):221–30. doi: 10.1007/s12192-007-0008-y
140. Multhoff G, Seier S, Stangl S, Sievert W, Shevtsov M, Werner C, et al. Targeted Natural Killer Cell–Based Adoptive Immunotherapy for the Treatment of Patients with NSCLC after Radiochemotherapy: A Randomized Phase II Clinical Trial. Clin Cancer Res (2020) 26(20):5368. doi: 10.1158/1078-0432.CCR-20-1141
141. Gamboa L, Zamat AH, Kwong GA. Synthetic immunity by remote control. Theranostics (2020) 10(8):3652–67. doi: 10.7150/thno.41305
142. Abedi MH, Lee J, Piraner DI, Shapiro MG. Thermal Control of Engineered T-cells. ACS Synthetic Biol (2020) 9(8):1941–50. doi: 10.1021/acssynbio.0c00238
143. Kregel KC. Invited Review: Heat shock proteins: modifying factors in physiological stress responses and acquired thermotolerance. J Appl Physiol (2002) 92(5):2177–86. doi: 10.1152/japplphysiol.01267.2001
144. Morimoto RI. Regulation of the heat shock transcriptional response: cross talk between a family of heat shock factors, molecular chaperones, and negative regulators. Genes Dev (1998) 12(24):3788–96. doi: 10.1101/gad.12.24.3788
145. Bae J, Munshi A, Li C, Samur M, Prabhala R, Mitsiades C, et al. Heat shock protein 90 is critical for regulation of phenotype and functional activity of human T lymphocytes and NK cells. J Immunol (Baltimore Md 1950) (2013) 190(3):1360–71. doi: 10.4049/jimmunol.1200593
146. Hayashi K, Kamikawa Y. HSP90 is crucial for regulation of LAT expression in activated T cells. Mol Immunol (2011) 48(6):941–6. doi: 10.1016/j.molimm.2010.12.014
147. Gattringer G, Greil R, Radaszkiewicz T, Huber H. In situ quantification of T-cell subsets, NK-like cells and macrophages in hodgkin’s disease: Quantity and quality of infiltration density depends on histopathological subtypes. Blut (1986) 53(1):49–58. doi: 10.1007/BF00320582
148. Ayoub J-P, Palmer JL, Huh Y, Cabanillas F, Younes A. Therapeutic and Prognostic Implications of Peripheral Blood Lymphopenia in Patients with Hodgkin’s Disease. Leukemia Lymphoma (1999) 34(5-6):519–27. doi: 10.3109/10428199909058479
149. Konjević G, Jurišić V, Banićević B, Spužić I. The difference in NK-cell activity between patients with non-Hodgkin’s lymphomas and Hodgkin’s disease. Br J Haematol (1999) 104(1):144–51. doi: 10.1046/j.1365-2141.1999.01129.x
150. Böll B, Eltaib F, Reiners KS, von Tresckow B, Tawadros S, Simhadri VR, et al. Heat Shock Protein 90 Inhibitor BIIB021 (CNF2024) Depletes NF-κB and Sensitizes Hodgkin’s Lymphoma Cells for Natural Killer Cell–Mediated Cytotoxicity. Clin Cancer Res (2009) 15(16):5108–16. doi: 10.1158/1078-0432.Ccr-09-0213
151. Fionda C, Soriani A, Malgarini G, Iannitto ML, Santoni A, Cippitelli M. Heat Shock Protein-90 Inhibitors Increase MHC Class I-Related Chain A and B Ligand Expression on Multiple Myeloma Cells and Their Ability to Trigger NK Cell Degranulation. J Immunol (2009) 183(7):4385. doi: 10.4049/jimmunol.0901797
152. Binder RJ, Blachere NE, Srivastava PK. Heat Shock Protein-chaperoned Peptides but Not Free Peptides Introduced into the Cytosol Are Presented Efficiently by Major Histocompatibility Complex I Molecules*. J Biol Chem (2001) 276(20):17163–71. doi: 10.1074/jbc.M011547200
153. Callahan MK, Garg M, Srivastava PK. Heat-shock protein 90 associates with N-terminal extended peptides and is required for direct and indirect antigen presentation. Proc Natl Acad Sci U States America (2008) 105(5):1662–7. doi: 10.1073/pnas.0711365105
154. Kunisawa J, Shastri N. The Group II Chaperonin TRiC Protects Proteolytic Intermediates from Degradation in the MHC Class I Antigen Processing Pathway. Mol Cell (2003) 12(3):565–76. doi: 10.1016/j.molcel.2003.08.009
155. Kunisawa J, Shastri N. Hsp90α Chaperones Large C-Terminally Extended Proteolytic Intermediates in the MHC Class I Antigen Processing Pathway. Immunity (2006) 24(5):523–34. doi: 10.1016/j.immuni.2006.03.015
156. Franks SE, Wolfson B, Hodge JW. Natural Born Killers: NK Cells in Cancer Therapy. Cancers (Basel) (2020) 12:(8). doi: 10.3390/cancers12082131
157. Gong JH, Maki G, Klingemann HG. Characterization of a human cell line (NK-92) with phenotypical and functional characteristics of activated natural killer cells. Leukemia (1994) 8(4):652–8.
158. Luevano M, Daryouzeh M, Alnabhan R, Querol S, Khakoo S, Madrigal A, et al. The unique profile of cord blood natural killer cells balances incomplete maturation and effective killing function upon activation. Hum Immunol (2012) 73(3):248–57. doi: 10.1016/j.humimm.2011.12.015
159. Gehrmann M, Marienhagen J, Eichholtz-Wirth H, Fritz E, Ellwart J, Jäättelä M, et al. Dual function of membrane-bound heat shock protein 70 (Hsp70), Bag-4, and Hsp40: protection against radiation-induced effects and target structure for natural killer cells. Cell Death Different (2005) 12(1):38–51. doi: 10.1038/sj.cdd.4401510
160. Multhoff G, Botzler C, Wiesnet M, Müller E, Meier T, Wilmanns W, et al. A stress-inducible 72-kDa heat-shock protein (HSP72) is expressed on the surface of human tumor cells, but not on normal cells. Int J Cancer (1995) 61(2):272–9. doi: 10.1002/ijc.2910610222
161. Botzler C, Schmidt J, Luz A, Jennen L, Issels R, Multhoff G. Differential Hsp70 plasma-membrane expression on primary human tumors and metastases in mice with severe combined immunodeficiency. Int J Cancer (1998) 77(6):942–8. doi: 10.1002/(SICI)1097-0215(19980911)77:6<942::AID-IJC25>3.0.CO;2-1
162. Shevtsov M, Pitkin E, Ischenko A, Stangl S, Khachatryan W, Galibin O, et al. Ex vivo Hsp70-Activated NK Cells in Combination With PD-1 Inhibition Significantly Increase Overall Survival in Preclinical Models of Glioblastoma and Lung Cancer. Front Immunol (2019) 10:454. doi: 10.3389/fimmu.2019.00454
163. Liu E, Tong Y, Dotti G, Shaim H, Savoldo B, Mukherjee M, et al. Cord blood NK cells engineered to express IL-15 and a CD19-targeted CAR show long-term persistence and potent antitumor activity. Leukemia (2018) 32(2):520–31. doi: 10.1038/leu.2017.226
164. Liu E, Marin D, Banerjee P, Macapinlac HA, Thompson P, Basar R, et al. Use of CAR-Transduced Natural Killer Cells in CD19-Positive Lymphoid Tumors. N Engl J Med (2020) 382(6):545–53. doi: 10.1056/NEJMoa1910607
165. Robert C. A decade of immune-checkpoint inhibitors in cancer therapy. Nat Commun (2020) 11(1):3801. doi: 10.1038/s41467-020-17670-y
166. Proia DA, Kaufmann GF. Targeting Heat-Shock Protein 90 (HSP90) as a Complementary Strategy to Immune Checkpoint Blockade for Cancer Therapy. Cancer Immunol Res (2015) 3(6):583–9. doi: 10.1158/2326-6066.Cir-15-0057
167. Proia DA, Kaufmann GF. Targeting Heat-Shock Protein 90 (HSP90) as a Complementary Strategy to Immune Checkpoint Blockade for Cancer Therapy. Cancer Immunol Res (2015) 3(6):583. doi: 10.1158/2326-6066.CIR-15-0057
168. Kryeziu K, Bruun J, Guren TK, Sveen A, Lothe RA. Combination therapies with HSP90 inhibitors against colorectal cancer. Biochim Biophys Acta (BBA) - Rev Cancer (2019) 1871(2):240–7. doi: 10.1016/j.bbcan.2019.01.002
169. Rao A, Taylor JL, Chi-Sabins N, Kawabe M, Gooding WE, Storkus WJ. Combination therapy with HSP90 inhibitor 17-DMAG reconditions the tumor microenvironment to improve recruitment of therapeutic T cells. Cancer Res (2012) 72(13):3196–206. doi: 10.1158/0008-5472.CAN-12-0538
170. Haggerty TJ, Dunn IS, Rose LB, Newton EE, Pandolfi F, Kurnick JT. Heat Shock Protein-90 Inhibitors Enhance Antigen Expression on Melanomas and Increase T Cell Recognition of Tumor Cells. PloS One (2014) 9(12):e114506. doi: 10.1371/journal.pone.0114506
171. Marzec M, Zhang Q, Goradia A, Raghunath PN, Liu X, Paessler M, et al. Oncogenic kinase NPM/ALK induces through STAT3 expression of immunosuppressive protein CD274 (PD-L1, B7-H1). Proc Natl Acad Sci U States America (2008) 105(52):20852–7. doi: 10.1073/pnas.0810958105
172. Mbofung RM, McKenzie JA, Malu S, Zhang M, Peng W, Liu C, et al. HSP90 inhibition enhances cancer immunotherapy by upregulating interferon response genes. Nat Commun (2017) 8(1):451–1. doi: 10.1038/s41467-017-00449-z
173. Majeti R, Chao MP, Alizadeh AA, Pang WW, Jaiswal S, Gibbs KD Jr, et al. CD47 is an adverse prognostic factor and therapeutic antibody target on human acute myeloid leukemia stem cells. Cell (2009) 138(2):286–99. doi: 10.1016/j.cell.2009.05.045
174. McCracken MN, Cha AC, Weissman IL. Molecular Pathways: Activating T Cells after Cancer Cell Phagocytosis from Blockade of CD47 “Don’t Eat Me” Signals. Clin Cancer Res an Off J Am Assoc Cancer Res (2015) 21(16):3597–601. doi: 10.1158/1078-0432.CCR-14-2520
175. Chao MP, Alizadeh AA, Tang C, Myklebust JH, Varghese B, Gill S, et al. Anti-CD47 antibody synergizes with rituximab to promote phagocytosis and eradicate non-Hodgkin lymphoma. Cell (2010) 142(5):699–713. doi: 10.1016/j.cell.2010.07.044
176. Advani R, Flinn I, Popplewell L, Forero A, Bartlett NL, Ghosh N, et al. CD47 Blockade by Hu5F9-G4 and Rituximab in Non-Hodgkin’s Lymphoma. New Engl J Med (2018) 379(18):1711–21. doi: 10.1056/NEJMoa1807315
177. Cook KL, Soto-Pantoja DR. “UPRegulation” of CD47 by the endoplasmic reticulum stress pathway controls anti-tumor immune responses. Biomark Res (2017) 5(1):26. doi: 10.1186/s40364-017-0105-8
178. Casey SC, Tong L, Li Y, Do R, Walz S, Fitzgerald KN, et al. MYC regulates the antitumor immune response through CD47 and PD-L1. Sci (New York NY) (2016) 352(6282):227–31. doi: 10.1126/science.aac9935
179. Lee J, Zhang LL, Wu W, Guo H, Li Y, Sukhanova M, et al. Activation of MYC, a bona fide client of HSP90, contributes to intrinsic ibrutinib resistance in mantle cell lymphoma. Blood Adv (2018) 2(16):2039–51. doi: 10.1182/bloodadvances.2018016048
180. Zhang L, Nomie K, Zhang H, Bell T, Pham L, Kadri S, et al. B-Cell Lymphoma Patient-Derived Xenograft Models Enable Drug Discovery and Are a Platform for Personalized Therapy. Clin Cancer Res an Off J Am Assoc Cancer Res (2017) 23(15):4212–23. doi: 10.1158/1078-0432.CCR-16-2703
181. Pearl LH, Prodromou C. Structure and Mechanism of the Hsp90 Molecular Chaperone Machinery. Annu Rev Biochem (2006) 75(1):271–94. doi: 10.1146/annurev.biochem.75.103004.142738
182. Kaul SC, Deocaris CC, Wadhwa R. Three faces of mortalin: A housekeeper, guardian and killer. Exp Gerontol (2007) 42(4):263–74. doi: 10.1016/j.exger.2006.10.020
183. Kang BH, Plescia J, Dohi T, Rosa J, Doxsey SJ, Altieri DC. Regulation of Tumor Cell Mitochondrial Homeostasis by an Organelle-Specific Hsp90 Chaperone Network. Cell (2007) 131(2):257–70. doi: 10.1016/j.cell.2007.08.028
184. Young JC, Hoogenraad NJ, Hartl FU. Molecular Chaperones Hsp90 and Hsp70 Deliver Preproteins to the Mitochondrial Import Receptor Tom70. Cell (2003) 112(1):41–50. doi: 10.1016/S0092-8674(02)01250-3
185. Balogi Z, Multhoff G, Jensen TK, Lloyd-Evans E, Yamashima T, Jäättelä M, et al. Hsp70 interactions with membrane lipids regulate cellular functions in health and disease. Prog Lipid Res (2019) 74:18–30. doi: 10.1016/j.plipres.2019.01.004
186. Juhasz K, Lipp A-M, Nimmervoll B, Sonnleitner A, Hesse J, Haselgruebler T, et al. The Complex Function of Hsp70 in Metastatic Cancer. Cancers (Basel) (2014) 6(1):42–66. doi: 10.3390/cancers6010042
187. Mosser DD, Caron AW, Bourget L, Denis-Larose C, Massie B. Role of the human heat shock protein hsp70 in protection against stress-induced apoptosis. Mol Cell Biol (1997) 17(9):5317–27. doi: 10.1128/mcb.17.9.5317
188. Calderwood SK, Gong J, Murshid A. Extracellular HSPs: The Complicated Roles of Extracellular HSPs in Immunity. Front Immunol (2016) 7:159. doi: 10.3389/fimmu.2016.00159
189. van Eden W, Jansen MAA, Ludwig I, van Kooten P, van der Zee R, Broere F. The Enigma of Heat Shock Proteins in Immune Tolerance. Front Immunol (2017) 8:1599. doi: 10.3389/fimmu.2017.01599
190. Sun R-F, Yu Q-Q, Young KH. Critically dysregulated signaling pathways and clinical utility of the pathway biomarkers in lymphoid malignancies. Chronic Dis Transl Med (2018) 4(1):29–44. doi: 10.1016/j.cdtm.2018.02.001
191. Johnson JL. Evolution and function of diverse Hsp90 homologs and cochaperone proteins. Biochim Biophys Acta (BBA) - Mol Cell Res (2012) 1823(3):607–13. doi: 10.1016/j.bbamcr.2011.09.020
192. Southworth DR, Agard DA. Species-dependent ensembles of conserved conformational states define the Hsp90 chaperone ATPase cycle. Mol Cell (2008) 32(5):631–40. doi: 10.1016/j.molcel.2008.10.024
193. Sciacovelli M, Guzzo G, Morello V, Frezza C, Zheng L, Nannini N, et al. The mitochondrial chaperone TRAP1 promotes neoplastic growth by inhibiting succinate dehydrogenase. Cell Metab (2013) 17(6):988–99. doi: 10.1016/j.cmet.2013.04.019
194. Matassa DS, Agliarulo I, Avolio R, Landriscina M, Esposito F. TRAP1 Regulation of Cancer Metabolism: Dual Role as Oncogene or Tumor Suppressor. Genes (Basel) (2018) 9(4):195. doi: 10.3390/genes9040195
195. Marzec M, Eletto D, Argon Y. GRP94: An HSP90-like protein specialized for protein folding and quality control in the endoplasmic reticulum. Biochim Biophys Acta (2012) 1823(3):774–87. doi: 10.1016/j.bbamcr.2011.10.013
196. Brewer JW, Diehl JA. PERK mediates cell-cycle exit during the mammalian unfolded protein response. Proc Natl Acad Sci U S A (2000) 97(23):12625–30. doi: 10.1073/pnas.220247197
197. Melendez K, Wallen ES, Edwards BS, Mobarak CD, Bear DG, Moseley PL. Heat shock protein 70 and glycoprotein 96 are differentially expressed on the surface of malignant and nonmalignant breast cells. Cell Stress Chaperones (2006) 11(4):334–42. doi: 10.1379/csc-187.1
198. Ryu J, Kaul Z, Yoon AR, Liu Y, Yaguchi T, Na Y, et al. Identification and functional characterization of nuclear mortalin in human carcinogenesis. J Biol Chem (2014) 289(36):24832–44. doi: 10.1074/jbc.M114.565929
199. Multhoff G, Botzler C, Wiesnet M, Muller E, Meier T, Wilmanns W, et al. A stress-inducible 72-kDa heat-shock protein (HSP72) is expressed on the surface of human tumor cells, but not on normal cells. Int J Cancer (1995) 61(2):272–9. doi: 10.1002/ijc.2910610222
200. Patel PD, Yan P, Seidler PM, Patel HJ, Sun W, Yang C, et al. Paralog-selective Hsp90 inhibitors define tumor-specific regulation of HER2. Nat Chem Biol (2013) 9(11):677–84. doi: 10.1038/nchembio.1335
201. Wu BX, Hong F, Zhang Y, Ansa-Addo E, Li Z. Chapter Seven - GRP94/gp96 in Cancer: Biology, Structure, Immunology, and Drug Development. In: Isaacs J, Whitesell L, editors. Advances in Cancer Research, vol. 129. SANDIEGO, USA: Elsevier Academic Press (2016). p. 165–90. doi: 10.1016/bs.acr.2015.09.001
202. Zheng H, Dai J, Stoilova D, Li Z. Cell Surface Targeting of Heat Shock Protein gp96 Induces Dendritic Cell Maturation and Antitumor Immunity. J Immunol (2001) 167(12):6731. doi: 10.4049/jimmunol.167.12.6731
203. Tang X, Chang C, Guo J, Lincoln V, Liang C, Chen M, et al. Tumour-Secreted Hsp90α on External Surface of Exosomes Mediates Tumour - Stromal Cell Communication via Autocrine and Paracrine Mechanisms. Sci Rep (2019) 9(1):15108. doi: 10.1038/s41598-019-51704-w
204. Ono K, Eguchi T, Sogawa C, Calderwood SK, Futagawa J, Kasai T, et al. HSP-enriched properties of extracellular vesicles involve survival of metastatic oral cancer cells. J Cell Biochem (2018) 119(9):7350–62. doi: 10.1002/jcb.27039
205. Noonan EJ, Place RF, Rasoulpour RJ, Giardina C, Hightower LE. Cell number-dependent regulation of Hsp70B′ expression: Evidence of an extracellular regulator. J Cell Physiol (2007) 210(1):201–11. doi: 10.1002/jcp.20875
206. Eguchi T, Sogawa C, Okusha Y, Uchibe K, Iinuma R, Ono K, et al. Organoids with cancer stem cell-like properties secrete exosomes and HSP90 in a 3D nanoenvironment. PloS One (2018) 13(2):e0191109–e0191109. doi: 10.1371/journal.pone.0191109
207. Caruso Bavisotto C, Cappello F, Macario AJL, Conway de Macario E, Logozzi M, Fais S, et al. Exosomal HSP60: a potentially useful biomarker for diagnosis, assessing prognosis, and monitoring response to treatment. Expert Rev Mol Diagnostics (2017) 17(9):815–22. doi: 10.1080/14737159.2017.1356230
208. Gunther S, Ostheimer C, Stangl S, Specht HM, Mozes P, Jesinghaus M, et al. Correlation of Hsp70 Serum Levels with Gross Tumor Volume and Composition of Lymphocyte Subpopulations in Patients with Squamous Cell and Adeno Non-Small Cell Lung Cancer. Front Immunol (2015) 6:556:556. doi: 10.3389/fimmu.2015.00556
209. Rothammer A, Sage EK, Werner C, Combs SE, Multhoff G. Increased heat shock protein 70 (Hsp70) serum levels and low NK cell counts after radiotherapy - potential markers for predicting breast cancer recurrence? Radiat Oncol (2019) 14(1):78. doi: 10.1186/s13014-019-1286-0
210. Hulina A, Grdić Rajković M, Jakšić Despot D, Jelić D, Dojder A, Čepelak I, et al. Extracellular Hsp70 induces inflammation and modulates LPS/LTA-stimulated inflammatory response in THP-1 cells. Cell Stress Chaperones (2018) 23(3):373–84. doi: 10.1007/s12192-017-0847-0
211. Breloer M, Fleischer B, Bonin AV. In Vivo and In Vitro Activation of T Cells After Administration of Ag-Negative Heat Shock Proteins. J Immunol (1999) 162(6):3141.
212. Daniels GA, Sanchez-Perez L, Diaz RM, Kottke T, Thompson J, Lai M, et al. A simple method to cure established tumors by inflammatory killing of normal cells. Nat Biotechnol (2004) 22(9):1125–32. doi: 10.1038/nbt1007
213. Chalmin F, Ladoire S, Mignot G, Vincent J, Bruchard M, Remy-Martin JP, et al. Membrane-associated Hsp72 from tumor-derived exosomes mediates STAT3-dependent immunosuppressive function of mouse and human myeloid-derived suppressor cells. J Clin Invest (2010) 120(2):457–71. doi: 10.1172/jci40483
214. Vega VL, Rodríguez-Silva M, Frey T, Gehrmann M, Diaz JC, Steinem C, et al. Hsp70 Translocates into the Plasma Membrane after Stress and Is Released into the Extracellular Environment in a Membrane-Associated Form that Activates Macrophages. J Immunol (2008) 180(6):4299. doi: 10.4049/jimmunol.180.6.4299
215. Multhoff G, Botzler C, Jennen L, Schmidt J, Ellwart J, Issels R. Heat shock protein 72 on tumor cells: a recognition structure for natural killer cells. J Immunol (1997) 158(9):4341.
216. Wachstein J, Tischer S, Figueiredo C, Limbourg A, Falk C, Immenschuh S, et al. HSP70 enhances immunosuppressive function of CD4(+)CD25(+)FoxP3(+) T regulatory cells and cytotoxicity in CD4(+)CD25(-) T cells. PloS One (2012) 7(12):e51747–7. doi: 10.1371/journal.pone.0051747
217. Asea A, Kraeft SK, Kurt-Jones EA, Stevenson MA, Chen LB, Finberg RW, et al. HSP70 stimulates cytokine production through a CD14-dependant pathway, demonstrating its dual role as a chaperone and cytokine. Nat Med (2000) 6(4):435–42. doi: 10.1038/74697
218. Asea A, Rehli M, Kabingu E, Boch JA, Bare O, Auron PE, et al. Novel signal transduction pathway utilized by extracellular HSP70: role of toll-like receptor (TLR) 2 and TLR4. J Biol Chem (2002) 277(17):15028–34. doi: 10.1074/jbc.M200497200
219. Asea A. Initiation of the Immune Response by Extracellular Hsp72: Chaperokine Activity of Hsp72. Curr Immunol Rev (2006) 2(3):209–15. doi: 10.2174/157339506778018514
221. Figueiredo C, Wittmann M, Wang D, Dressel R, Seltsam A, Blasczyk R, et al. Heat shock protein 70 (HSP70) induces cytotoxicity of T-helper cells. Blood (2009) 113(13):3008–16. doi: 10.1182/blood-2008-06-162727
222. Berthenet K, Boudesco C, Collura A, Svrcek M, Richaud S, Hammann A, et al. Extracellular HSP110 skews macrophage polarization in colorectal cancer. Oncoimmunology (2016) 5(7):e1170264–e1170264. doi: 10.1080/2162402X.2016.1170264
223. Banerjee S, Lin C-FL, Skinner KA, Schiffhauer LM, Peacock J, Hicks DG, et al. Heat Shock Protein 27 Differentiates Tolerogenic Macrophages That May Support Human Breast Cancer Progression. Cancer Res (2011) 71(2):318. doi: 10.1158/0008-5472.CAN-10-1778
224. Chalmin F, Ladoire S, Mignot G, Vincent J, Bruchard M, Remy-Martin J-P, et al. Membrane-associated Hsp72 from tumor-derived exosomes mediates STAT3-dependent immunosuppressive function of mouse and human myeloid-derived suppressor cells. J Clin Invest (2010) 120(2):457–71. doi: 10.1172/JCI40483
225. Laudanski K, De A, Miller-Graziano C. Exogenous heat shock protein 27 uniquely blocks differentiation of monocytes to dendritic cells. Eur J Immunol (2007) 37(10):2812–24. doi: 10.1002/eji.200636993
226. Staron M, Wu S, Hong F, Stojanovic A, Du X, Bona R, et al. Heat-shock protein gp96/grp94 is an essential chaperone for the platelet glycoprotein Ib-IX-V complex. Blood (2011) 117(26):7136–44. doi: 10.1182/blood-2011-01-330464
227. Zhang Y, Ansa-Addo E, Li Z. GP96: safeguarding Treg. Oncotarget (2015) 6(24):19936–7. doi: 10.18632/oncotarget.4582
228. Morales C, Rachidi S, Hong F, Sun S, Ouyang X, Wallace C, et al. Immune Chaperone gp96 Drives the Contributions of Macrophages to Inflammatory Colon Tumorigenesis. Cancer Res (2014) 74(2):446. doi: 10.1158/0008-5472.CAN-13-1677
229. Li R, Emsley J. The organizing principle of the platelet glycoprotein Ib–IX–V complex. J Thromb Haemostasis (2013) 11(4):605–14. doi: 10.1111/jth.12144
230. Wiest DL, Burkhardt JK, Hester S, Hortsch M, Meyer DI, Argon Y. Membrane biogenesis during B cell differentiation: most endoplasmic reticulum proteins are expressed coordinately. J Cell Biol (1990) 110(5):1501–11. doi: 10.1083/jcb.110.5.1501
231. Gass JN, Gifford NM, Brewer JW. Activation of an Unfolded Protein Response during Differentiation of Antibody-secreting B Cells *. J Biol Chem (2002) 277(50):49047–54. doi: 10.1074/jbc.M205011200
232. Pilzer D, Fishelson Z. Mortalin/GRP75 promotes release of membrane vesicles from immune attacked cells and protection from complement-mediated lysis. Int Immunol (2005) 17(9):1239–48. doi: 10.1093/intimm/dxh300
233. Pilzer D, Saar M, Koya K, Fishelson Z. Mortalin inhibitors sensitize K562 leukemia cells to complement-dependent cytotoxicity. Int J Cancer (2010) 126(6):1428–35. doi: 10.1002/ijc.24888
234. Rozenberg P, Ziporen L, Gancz D, Saar-Ray M, Fishelson Z. Cooperation between Hsp90 and mortalin/GRP75 in resistance to cell death induced by complement C5b-9. Cell Death Dis (2018) 9(2):150. doi: 10.1038/s41419-017-0240-z
235. Martine P, Rébé C. Heat Shock Proteins and Inflammasomes. Int J Mol Sci (2019) 20(18):4508. doi: 10.3390/ijms20184508
236. Martine P, Chevriaux A, Derangère V, Apetoh L, Garrido C, Ghiringhelli F, et al. HSP70 is a negative regulator of NLRP3 inflammasome activation. Cell Death Dis (2019) 10(4):256–6. doi: 10.1038/s41419-019-1491-7
237. Yaguchi T, Aida S, Kaul SC, Wadhwa R. Involvement of Mortalin in Cellular Senescence from the Perspective of its Mitochondrial Import, Chaperone, and Oxidative Stress Management Functions. Ann New York Acad Sci (2007) 1100(1):306–11. doi: 10.1196/annals.1395.032
238. Wadhwa R, Takano S, Kaur K, Aida S, Yaguchi T, Kaul Z, et al. Identification and characterization of molecular interactions between mortalin/mtHsp70 and HSP60. Biochem J (2005) 391(Pt 2):185–90. doi: 10.1042/bj20050861
239. Custer CD, Kaul SC, Wadhwa R. On the Brotherhood of the Mitochondrial Chaperones Mortalin and Heat Shock Protein 60. Cell Stress Chaperones (2006) 11(2):116–28. doi: 10.1379/CSC-144R.1
240. Goetz MP, Toft DO, Ames MM, Erlichman C. The Hsp90 chaperone complex as a novel target for cancer therapy. Ann Oncol (2003) 14(8):1169–76. doi: 10.1093/annonc/mdg316
241. Zhang H, Chung D, Yang Y-C, Neely L, Tsurumoto S, Fan J, et al. Identification of new biomarkers for clinical trials of Hsp90 inhibitors. Mol Cancer Ther (2006) 5(5):1256. doi: 10.1158/1535-7163.MCT-05-0537
242. Zhao M, Ding JX, Zeng K, Zhao J, Shen F, Yin YX, et al. Heat shock protein 27: a potential biomarker of peritoneal metastasis in epithelial ovarian cancer? Tumor Biol (2014) 35(2):1051–6. doi: 10.1007/s13277-013-1139-7
243. Suzuki K, Ito Y, Wakai K, Kawado M, Hashimoto S, Seki N, et al. Serum Heat Shock Protein 70 Levels and Lung Cancer Risk: A Case-Control Study Nested in a Large Cohort Study. Cancer Epidemiol Biomarkers & Prev (2006) 15(9):1733. doi: 10.1158/1055-9965.EPI-06-0005
244. Gunaldi M, Afsar CU, Okuturlar Y, Gedikbasi A, Kocoglu H, Kural A, et al. Elevated Serum Levels of Heat Shock Protein 70 Are Associated with Breast Cancer. Tohoku J Exp Med (2015) 236(2):97–102. doi: 10.1620/tjem.236.97
Keywords: heat shock proteins, lymphoma, CAR T, CAR NK, checkpoint inhibitors
Citation: Albakova Z, Mangasarova Y and Sapozhnikov A (2021) Heat Shock Proteins in Lymphoma Immunotherapy. Front. Immunol. 12:660085. doi: 10.3389/fimmu.2021.660085
Received: 28 January 2021; Accepted: 26 February 2021;
Published: 18 March 2021.
Edited by:
Jérôme Paggetti, Luxembourg Institute of Health, LuxembourgReviewed by:
Gabriele Multhoff, Technical University of Munich, GermanyCarmen Garrido, Institut National de la Santé et de la Recherche Médicale (INSERM), France
Copyright © 2021 Albakova, Mangasarova and Sapozhnikov. This is an open-access article distributed under the terms of the Creative Commons Attribution License (CC BY). The use, distribution or reproduction in other forums is permitted, provided the original author(s) and the copyright owner(s) are credited and that the original publication in this journal is cited, in accordance with accepted academic practice. No use, distribution or reproduction is permitted which does not comply with these terms.
*Correspondence: Zarema Albakova, emFyZW1hLmFsYmFrb3ZhMTRAZ21haWwuY29t