- 1Transplant and Autoimmunity Group, Research Institute Marques de Valdecilla (IDIVAL), Santander, Spain
- 2Clinical and Translational Research in Digestive Pathology Group, Research Institute Marques de Valdecilla (IDIVAL), Santander, Spain
- 3Immunology Department, Marques de Valdecilla University Hospital, Santander, Spain
- 4Gastroenterology and Hepatology Department, Marques de Valdecilla University Hospital, Santander, Spain
The coronavirus infectious disease 2019 (COVID-19) pandemic has hit the world, affecting health, medical care, economies and our society as a whole. Furthermore, COVID-19 pandemic joins the increasing prevalence of metabolic syndrome in western countries. Patients suffering from obesity, type II diabetes mellitus, cardiac involvement and metabolic associated fatty liver disease (MAFLD) have enhanced risk of suffering severe COVID-19 and mortality. Importantly, up to 25% of the population in western countries is susceptible of suffering from both MAFLD and COVID-19, while none approved treatment is currently available for any of them. Moreover, it is well known that exacerbated innate immune responses are key in the development of the most severe stages of MAFLD and COVID-19. In this review, we focus on the role of the immune system in the establishment and progression of MAFLD and discuss its potential implication in the development of severe COVID-19 in MAFLD patients. As a result, we hope to clarify their common pathology, but also uncover new potential therapeutic targets and prognostic biomarkers for further research.
Introduction
The coronavirus infectious disease 2019 (COVID-19) pandemic caused by the severe acute respiratory syndrome coronavirus 2 (SARS-CoV-2) has emerged as a global challenge, affecting health and medical care but also economies and society. In an unprecedented way, researchers have unraveled its main clinical and epidemiological features, its pathogenesis and the mayor ways of transmission in record time. Knowledge and improved diagnostics and treatments are increasing day by day. It is not the aim of this review to summarize all these data, available elsewhere (1, 2), but we should highlight that SARS-CoV-2 infection is determined by the interaction of its spike protein with angiotensin converting enzyme 2 (ACE2) and further needs of transmembrane protease serine 2 (TMPRSS2) to initiate fusion and cell infection (3), although other receptors and proteases have been also involved (3–9). From here on, COVID-19 pathogenesis could evolve in several ways resulting in a wide range of symptoms and severity - from fully asymptomatic to death – (1). Host immune response is key in the course of the disease: immunodeficiency states can facilitate a more aggressive course with progression to severe COVID-19 characterized by a deregulated immune response resulting in the so-called “cytokine storm” (10) and complement-induced coagulopathy (2). Moreover, accumulating data are pointing out that COVID-19 is not just a respiratory disease, but a multi-organ dysfunction (11), in which a “bradykinin storm” starting in the lungs may have a pivotal role (12).
Regarding the digestive system, it is noteworthy that both adult and pediatric COVID-19 patients reported gastrointestinal symptoms including diarrhea, vomiting and abdominal pain during course of the disease. The gut symptoms correlate with markers of liver damage (13). Liver injury in patients with COVID-19 is frequent, although mild in nature, with a hepatocellular rather than cholestatic pattern (14). However, severe COVID-19 is accompanied by higher serum transaminases levels (15). In addition to the respiratory system, the gastrointestinal tract is a major infection site of SARS-CoV-2, as ACE2 is highly expressed in proximal and distal enterocytes (16) and viral nucleocapsid protein has been visualized in the cytoplasm of gastric, duodenal, and rectum glandular epithelial cells from a COVID-19 patient (17). Hepatocytes also express detectable amounts of both ACE2 and TMPRSS2, thus they are susceptible of SARS-CoV-2 infection (18). Furthermore, the liver is constantly expose to foreign antigens entering the bloodstream from the gut and keeps a fine balance between the activation of the immune cells for the detection and clearance of pathogens and tolerance towards non-damaging antigens. Due to this reason, the liver has special immune functions and contains the largest number of permanent macrophages, the well-known Kupffer cells (KC). Among their functions, KC are in charge of the clearance of senescent neutrophils and other activated host cells, thus limiting the potential of these cells to produce inflammatory mediators (19).
Older age, male sex, type II diabetes mellitus (DM2) and obesity are mayor risk factors for the development of critically ill COVID-19 (20). Most patients suffering from metabolic associated fatty liver disease - MAFLD, previously known as non-alcoholic fatty liver disease (21) – have all these characteristics (22, 23). Although full description of the mechanisms accounting for DM2 and obesity implication in COVID-19 development have been discussed already (24, 25), Figure 1 summarizes main contributions of MAFLD, obesity, DM2 and COVID-19 to the overall pathogenesis observed in severe COVID-19 patients. MAFLD includes a spectrum of liver disease defined by an excessive accumulation of fat in hepatocytes, ranging from hepatic steatosis to non-alcoholic steatohepatitis (NASH), liver cirrhosis, and hepatocellular carcinoma (HCC). Whereas steatosis is reversible, later stages cannot be cured and current treatment is limited to lifestyle interventions. Disease progression is characterized by increasing lymphocyte infiltration and inflammation in the liver together with fibrosis and reduced liver function. Recently, new factors contributing to MAFLD have been described, such as gut microbiome alterations, changes in intestinal permeability and bacterial antigen translocation (26, 27). MAFLD prevalence is increasing world-wide together with the pandemic of obesity and metabolic syndrome, affecting already 25% of the adult population globally (23). Moreover, it has been shown that liver fat content is determinant of higher risk of severe COVID-19 in obese patients (28) and the risk of obesity to COVID-19 severity is greater in those with MAFLD than in obese patients without MAFLD (29). Increasing evidence is supporting that MAFLD patients are at risk of developing severe COVID-19 (30–32), even in the absence of its common comorbidities (33) and especially in younger patients (34). Patients with MAFLD had a higher risk of disease progression, higher likelihood of abnormal liver function and longer viral shedding time compared to patients without MAFLD (30). Meta-analysis further confirmed that a high percentage of patients with COVID-19 had MAFLD, and MAFLD increased the risk of disease progression among patients with COVID-19 (35, 36). Liver fibrosis by itself has emerged as a risk factor for severe COVID-19 illness (32, 37) and patients with cirrhosis+COVID-19 had a higher mortality rate compared with those with COVID-19 alone (38, 39). Therefore, we want to focus attention on the high percentage of the population who will suffer from both MAFLD and severe COVID-19 without treatment for any of the diseases and the need to prioritize vaccination of these patients. This review is aimed at summarizing current knowledge about the role of the immune system in the establishment and progression of MAFLD and to discuss its potential implication in the development of severe COVID-19 in MAFLD patients. As a result, we hope to clarify their common pathology, but also uncover new potential therapeutic targets and prognostic biomarkers for further research.
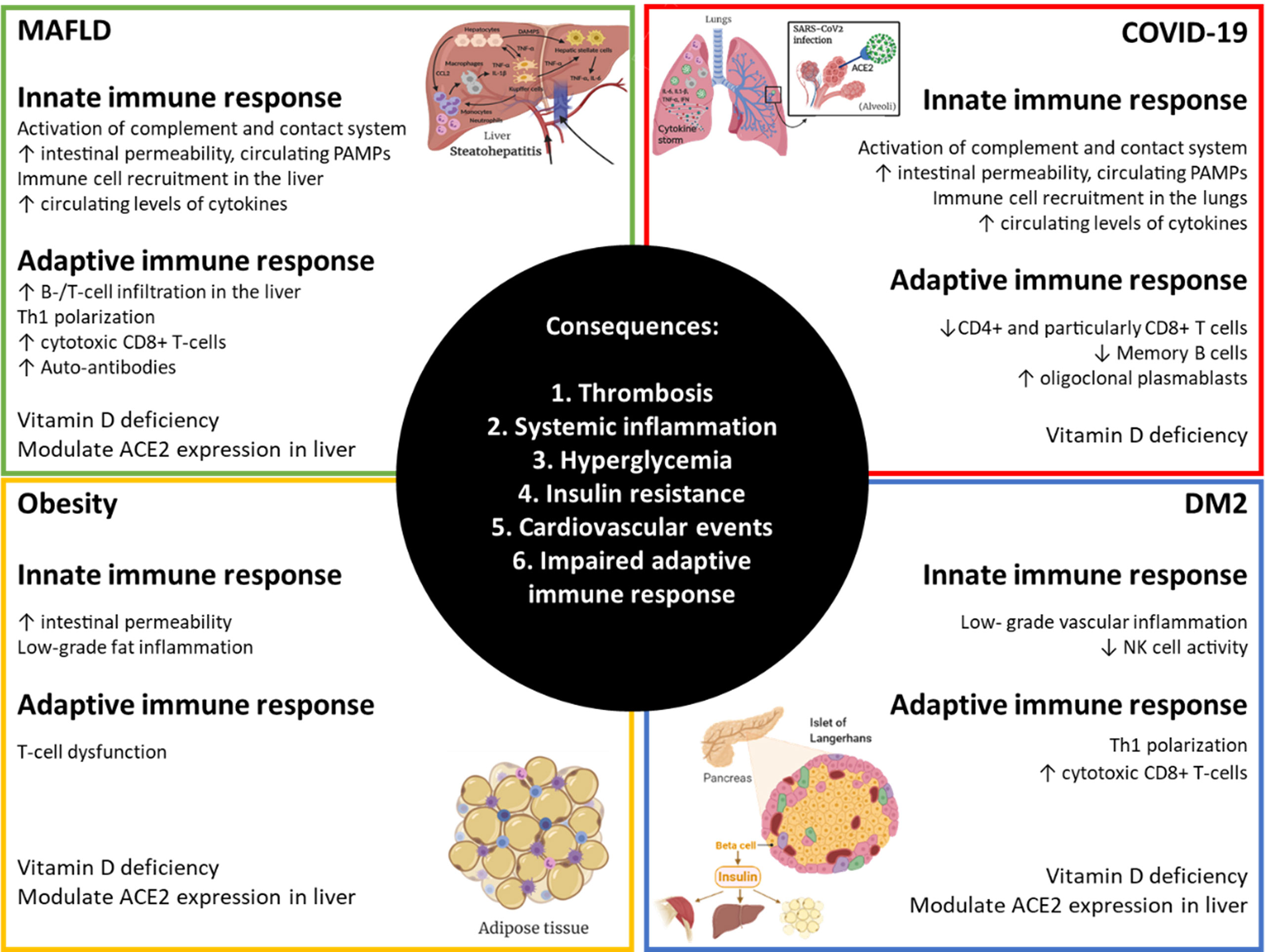
Figure 1 Alterations in immunity that amplify responses to SARS-CoV-2 in MAFLD, obese and DM2 patients. Obesity, diabetes mellitus type 2 (DM2) and metabolic associated fatty liver disease (MAFLD) are very common comorbidities. Each of them has broad effects on the immune system resulting in impairments in normal homeostasis that can lead to more severe COVID-19. Created with BioRender.com.
Alterations of the Innate Immune Response
Role of Innate Immune Response in MAFLD
There are many components of the innate immune response implicated in the MAFLD pathogenesis and development. Inflammation is an important factor to look out for, due to its capacity to go further and develop HCC, among other tumors (40, 41). Due to the relevant role of innate immunity in MAFLD, in the following sections we will discuss the implication of its different components in MAFLD progression.
Complement and Contact Systems
The complement system is activated, among other stimuli, after liver injury, contributing to the development of NASH and HCC (42). Thus, this liver damage reduces the majority of soluble complement proteins levels which lead to an increase of activation markers, and finally, inflammation. Moreover, some complement proteins, like C3 and C4, can act as acute-phase reactants, increasing the systemic inflammation response by 50% (43).
The synthesis of C3 is clearly enhanced in liver pathogenesis (44) and C1q has also been involved in HCC development (45). Other complement proteins that accumulate around hepatocytes with steatosis, such as iC3b, C3d, C4d and C5b-9, are related to some proinflammatory signals such as neutrophils recruitment and cytokine release (46). Therefore, it can be concluded that the inhibition of a permanently activated complement system may ameliorate the liver damage in NASH disease (47).
The contact system is composed of coagulation factors XI (FXI) and XII (FXII), plasma prekallikrein, and high molecular weight kininogen. The final product of the contact system activation cascade is bradykinin, which has vasodilator and pro-inflammatory properties (48). Because of the majority of coagulation factors are formed in the liver and MAFLD has important metabolic implications, a study showed MAFLD patients seem to have increased coagulation factors levels. It suggested a link between the liver fat content and insulin resistance which may be related to FIX and FXI activities. The increase of FXII in murine models could trigger FIX and FXI, which favors thrombosis appearance and propagation in MAFLD (49).
Pattern Recognition Receptors (PRR)
The Toll-Like Receptor family (TLR) is an important and well characterized class of cell surface or intracellular PRRs, which are highly expressed in many liver cells under metabolic stress, such as KC, hepatic stellate cells, biliary epithelial cells and sinusoidal endothelial cells (40, 41). Some members of the TLR family (TLR2, TLR4, TLR5, TLR6, TLR7 and TLR9) have been associated to MAFLD pathogenesis. The TLR signal triggers inflammatory pathways in fat tissue and liver, activating transcription factors such as NF-κB and IRFs (Interferon Regulatory Factors) and also several inflammatory cytokines (40, 50, 51). TLR4 is remarkably associated to MAFLD. It binds to the Myeloid Differentiation factor 2 protein (MD-2), and this association confers responsiveness to LPS (52). Actually, both animal models and clinical studies have noticed increased levels of circulating LPS in MAFLD due to the intrinsic endotoxemia caused by factors such as intestinal microbiota, intestinal permeability and high fat and/or sugar diet (HFD) (53–55). This last factor could explain the reason why free fatty acids regulate TLR4 positively in presence of high glucose levels (56). In addition, the TLR4-LPS pathway has also been implicated in MAFLD progression to HCC (57). In HFD, gut microbiota is enriched with gram positive bacteria (58, 59). Taking into account that TLR2 recognizes peptidoglycan, a gram positive bacterial component, its blockade in animal models has also a protective effect in developing insulin resistance, closely related to MAFLD pathogenesis. Actually, some studies have shown that mice with TLR2 deficiency express decreased proinflammatory cytokines by unchaining the inflammasome in KC (50). TLR2 usually forms heterodimers with TLR6, another extracellular receptor increased in NASH patient’s hepatocytes. This heterodimer is also present in lobular inflammation. Therefore, TLR6 has been proposed as a potential biomarker in the development of NASH in MAFLD obese patients (60). TLR7 has also been associated with liver fibrosis (61), although its role in MAFLD is not completely elucidated. According to a study, the presence of TLR7 or its agonists can avoid experimental MAFLD development. An autophagy activation marker seems to be regulated by TLR7 and this process can improve steatosis in MAFLD by stimulating lipid degradation. TLR7 stimulation could be a potential therapeutic target to prevent the consequences of MAFLD, but as it is mentioned before, further investigation is required to clarify TLR7 function in this disease (51). Finally, it should be said that TLR9 is the only intracellular receptor involved in MAFLD pathogenesis. Animal model studies have shown that its activation results in IL-1β production so, along with TLR2 and TLR4, it is strongly involved in NASH and liver fibrosis (50).
The NOD-Like Receptors (NLRs) can form inflammasomes and lead to cell death. NOD-, LRR- and pyrin domain-containing protein 3 (NLRP3) is an intracellular protein complex which plays a key role in the innate immune system triggering several inflammatory components. It has been demonstrated that the lack of NLRP3 inflammasome reduces hepatocyte pyroptosis and, in consequence, inflammation and fibrosis in animal models with NASH. Also, some studies in humans and murine models with liver damage have shown increased levels of NLRP3, caspase 1, IL-1β and IL-18. These facts could play a part in terms of NASH pathogenesis (40, 62).
Innate Immune Cells
Signals stimulate several cellular receptors which can activate different cells types in the liver. Around 15% of them are KC (40). After activation, KC can polarize into two phenotipically different forms or also express both at the same time: one more pro-inflammatory (M1) and other mostly characterized by its immunoregulatory properties (M2). Some studies have shown in mice that a HFD benefits the presence of M1 phenotype and the inflammatory response, while the up-regulation of the peroxisome proliferator-activated receptor gamma (PPAR-γ) induces the M1 phenotype polarization to a M2 immunoregulatory phenotype. This fact could prevent the progression of MAFLD disease (63). LPS and other bacterial products activate KC recognition by TLRs and trigger M1 phenotype, which produces several pro-inflammatory cytokines (TNF-α, IL-1β, IL-12), chemokines (CCL2 and CCL5) and damage-associated molecular patterns (DAMPs). These DAMPs promote liver damage through KC activation by TLR pathway, repeating this inflammation process and leading to hepatocyte injury. The accumulation of other products like several free fatty acids, oxidized lipoproteins and other lipids is a very important fact to take into account in experimental MAFLD/NASH pathogenesis because of its involvement in KC activation, causing a bigger response to LPS and therefore, disease progression (40).
Alteration in antigen presenting cells, like dendritic cells (DC), is also relevant in NASH, but its role in this disease is complex and not entirely defined. This could be due to the fact that the two subtypes of conventional DC have opposite roles in terms of NASH activity. A recent study showed that, in patients, cDC2 were positively associated with NASH, and both HLA-DR+CD123-CD11c+CD141+ cDC1 and HLA-DR+CD123+ plasmacytoid DC were inversely correlated with NASH and glucose levels (64).
Natural Killer (NK) cells have a controversial function in NASH pathogenesis: some studies link its activation by different cytokines and ligands to MAFLD/NASH, while others show a reduction in its cytotoxic activity in NASH (65, 66). Additionally, NK T cells (NKT) include two distinct subtypes of cells. Thus, type I NKT is activated by lipid accumulation and may play a pro-inflammatory role in MAFLD, while type II NKT could have an opposite function protecting against liver damage (67, 68). Actually, another study performed on patients showed that the level of liver NKT cells was positively associated with disease stage (69).
These apparently opposite effects of innate immune cells could be due to the plasticity of the immune response and it highlights the need to evaluate changes in phenotype and function longitudinally during liver injury.
Cytokines and Other Immune Cells Inflammatory Products
Studies in animal models have shown the involvement of several cytokines in MAFLD, including IL-1β (70), IL-6 (71), TNF-α (71–73) and IFN-α (74). These results are further supported by clinical findings, although some controversy is still present. IL-1β and IL-6 levels were significantly higher in patients with NASH compared with MAFLD and control group, but this study failed in detecting increased levels of TNF-α (75). Serum IL-6 levels were increased in patients with advanced fibrosis compared to patients with mild/no fibrosis (76) and they predict the development of DM2 in women (77). TNF mRNA expression was found increased in hepatic and adipose tissue of NASH patients (78). For instance, TNF-α, among others, featured a clear correlation with transaminase levels and histological severity of MAFLD patients and has been proposed as biomarker of disease progression (79). In contrast, early studies in humans exploring TNF-α blockade as a therapeutic target in metabolic diseases did not show beneficial effects. However, those trials were not well conducted and the clinical designs had some drawbacks such as dosing, duration or presence of confounding factors, among others (80). A recent study has found that levels of circulating pro-inflammatory cytokines were variable in MAFLD patients with or without obesity, but when patients were distributed by the presence of circulating bacterial antigens, a statistically significant increase was observed in serum TNF-α and IL-6 levels in MAFLD patients (26).
Several nuclear transcription factors and some intracellular signaling pathways are involved in MAFLD pathogenesis, but Nuclear factor-kappa B (NF-κB) and c-Jun N-terminal kinase (JNK) are especially remarkable in NASH pro-inflammatory pathways (81, 82). NF-κB is activated by TLRs and triggers the transduction of IL-1β, IL-2, IL-6 and TNF-α (83). JNK overactivation is highly involved in the development of MAFLD and the subsequent liver damage (84). Furthermore, the activation of these pathways links MAFLD and extra-hepatic comorbidities such as insulin resistance and cardiovascular disease (85, 86).
Innate Immunity and COVID-19 Severity in MAFLD Patients
At this point in the research on COVID-19, it is clear that dysregulated and excessive innate immune responses towards SARS-CoV-2 cause immune damage to the human body. Siddiqi et al. elegantly defined COVID-19 pathogenesis in three phases: early infection, pulmonary phase, and hyperinflammation phase (87). The third and most severe phase is defined by a cytokine storm, which results from a sudden acute increase in circulating levels of different pro-inflammatory cytokines and other related proteins including IL-1β, IL-7, IL-8, IL-9, IL-10, FGF, G-CSF, GM-CSF, IFN-γ, IP-10, MCP-1, MIP-1A, MIP1-B, PDGF, TNF-α, VEGF, C-reactive protein, ferritin and D-dimer (87, 88). MAFLD is associated with chronic, low-grade inflammation in the liver that causes systemic effects, detectable by alterations in circulating immune cells and humoral factors (85). Thus, this precondition would ease the progression of COVID-19 into its severe manifestations, as described hereafter.
Complement and Contact Systems
As we already mentioned, the complement system is crucial to trigger an innate immune response to several microorganisms (89) and thus to COVID-19. Actually, some soluble complement proteins, such as C3a and C5a, have proinflammatory functions and they are in charge of immune cell recruitment which can contribute to lung damage in COVID-19 pathogenesis. Although the complement action is not entirely clear about its protection or pathogenicity, a recent study proved the efficacy of a monoclonal antibody against C5/C5a, called eculizumab, in order to ameliorate the pulmonary dysfunction due to COVID-19 (90). Additionally, it has been noticed increased levels of C5a in bronchioalveolar fluid of COVID-19 patients with the most severe symptoms, pointing out the role of this protein in the enhanced inflammation developed in severe COVID-19 patients. Taking this into account, and due to the fact that C5a receptor 1 (C5aR1) levels are also increased in lung neutrophils of severe COVID-19 patients, a study showed anti-C5aR1 therapeutic monoclonal antibodies can fight against C5a action and avoid the infiltration of human myeloid cells in damaged organs, preventing the inflammation developed in severe COVID-19 patients (91). Another study also proved that C3 knockout mice showed lower levels of inflammatory immune innate cells, such as neutrophils and monocytes, leading to reduced SARS-CoV pulmonary injury and suggesting that this virus may activate the complement system (92). As mentioned before, liver injury induces complement activation markers in MAFLD, and due to the fact that this liver damage pattern is also present in COVID-19 (93), MAFLD patients coexisting with COVID-19 infection could have an increased complement function exacerbating the inflammation. The contact system is also an important part of the innate immune defense against viruses. Actually, it has been shown that SARS-CoV-2 penetrates the cell via ACE2. Thus, it has been suggested a target therapy consisting of ACE2 direct activation, which avoid SARS-CoV-2 protein S binding to ACE2 (94–96). Moreover, ACE2 has the capacity of degrading des-Arg bradykinin, which is the ligand of the bradykinin receptor type 1 (B1). Once activated, B1 signaling leads to pulmonary angioedema. SARS-CoV-2 infection impairs ACE2 function; therefore, des-Arg bradykinin is accumulated, producing pulmonary angioedema in COVID-19 patients (97). B1 blocking is also a potential strategy to ameliorate pulmonary complications in COVID-19. The complement system, as well as the contact system, seems to be important player in the progression of COVID-19 and, as we mentioned before, their function is altered in MAFLD patients. Although eculizumab is the only approved treatment for humans that inhibits complement cascade at the moment (96), anti-C5aR1 monoclonal antibodies blocking this receptor are a promising and more specific treatment in severe COVID-19 patients due to the fact that C5a is a chemoattractant factor that facilitates the adherence of leukocytes to the endothelium (91).
Pattern Recognition Receptors (PRR)
One of the first mechanisms linking COVID-19 severity and MAFLD affects the most basic elements of innate immunity: physical barriers. Several authors suggest that the increased risk observed in MAFLD patients is driven by SARS-CoV-2 infection of the gut, which exacerbates an existing state of intestinal permeability and mucosal inflammation, increasing the transmission of pathogen-associated molecular patterns to the liver and affecting systemic immune response (98–101). Supporting data is preliminary, but some authors have already shown that COVID-19 patients have altered fecal microbiota (102–105) and plasma markers of gut leakage and inflammasome activation are increased in COVID-19 patients, especially in those with cardiac involvement (106).
Some evidence also pointed out at TLR signaling as possible impaired mechanisms in severe COVID-19. On one hand, bioinformatics studies have shown that SARS-CoV-2 genome possess multiple single-stranded RNA sequences probably recognized by TLR7 and 8 (107). A case-series article identified four young men from two families carrying rare and inactivating mutations in TLR7 who suffered from severe COVID-19 in the absence of common comorbitidies (108). Although these mutations are unlikely to be an explanation for severe COVID-19 in the general population, other mechanisms accounting for TLR7 signaling impairment could result in a similar situation. Accordingly, some authors hypothesize that chronic stimulation of TLR7 by intrinsic substrates could lead to a desensitization of TLR7 signalling. Therefore, the immune response in those patients will be delayed upon viral infection, but when resensitization finally occurs, it leads to an overwhelming TLR7 response (109). As mentioned before, TLR7 seems to perform a protective role in liver injury (61) and MAFLD (51), but it has been also described that liver injury can promote pulmonary inflammation through the activation of TLR7/8 in alveolar macrophages. Based in human samples and animal models, authors found that injured hepatocytes release miRNA-122, which is preferentially transported to the lungs where it triggers TLR7/8 signaling eliciting macrophage inflammatory responses (110). On the other hand, TLR3 and TLR4 knock-out mice have shown its relevance in preventing SARS-CoV lethal infection, in which TRIF adaptor protein has a central role (111). In this sense, a small study analyzed PBMCs transcriptomics in patients with COVID-19 and observed that the expression of TLR4 and downstream signaling molecules were significantly upregulated. These authors also showed that SARS-CoV-2 proteins increased the expression of the TLR4 ligand S100A8/A9 in PBMCs in vitro, proposing that SARS-CoV-2 infection can product a feed-forward loop through TLR4 activation that sustained the inflammation in COVID-19 patients (112). As mention before, MAFLD patients have increased intestinal permeability, resulting in increased circulating levels of LPS (113). In conclusion, patients suffering from MAFLD could suffer a chronic stimulation of TLR7/8 and TLR4 in the alveolar macrophages, which make them more vulnerable to severe COVID-19.
Finally, SARS-CoV-2 is able to induce NRPL3 inflammasome (114–116), which is also a major contributor to hepatocyte death in NASH. Recently, it has been shown that the magnitude and course of NRPL3 activation has an important role in the clinical outcome of COVID-19 patients during their hospitalization period. NRPL3 presence in PBMCs of these patients, together with high levels of casp1p20, among others, support this statement and suggest inflammasome can act not only as a marker of severity, but also prognosis and even as a therapeutic target (117).
Innate Immune Cells
The innate immune system appears to have an opposite role to adaptive responses in SARS-COV2 infections; several innate immune cells sometimes contribute to the progression of the disease (118). Actually, lung inflammation caused by COVID-19 can be aggravated because of macrophage activation syndrome and its production of several inflammatory cytokines (IL-6, IL-7, TNF), chemokines (CCL2, CCL3, CXCL10) and the soluble form of α-chain of the IL-2 receptor (119, 120). In COVID-19 patients, hepatocellular liver damage is apparently frequent, and knowing that liver macrophages are able to produce a great variety of cytokines, it could be suggested a leaning towards M1 macrophages polarization of these immune cells thus contributing to the development of COVID-19 in MAFLD (93). Not only macrophages take part in COVID-19 pathogenesis, other immune innate cells such as monocytes and neutrophils are involved, and their levels in COVID-19 patients were found increased (121). Severe COVID-19 patients showed high levels of two essential monocyte recruitment chemokines: CCL2 and CCL7 (122). In another study of COVID-19 patients who needed ICU hospitalization, a remarkable CD14+CD16+ monocyte levels that also produce IL-6 were noticed (120). The inflammatory environment induced by these cells cause an excessive inflammation which could be even more dangerous than the virus infection itself (121). Additionally, the sex differences in COVID-19 patients are an interesting fact because they could be related to hormonal dependency: some immune innate cells such as neutrophils, macrophages, DC and NKs depend on estrogen and testosterone to mature and differentiate, without forgetting they can be modulated by individual variability and genetic background (123). Interestingly, a recent published study reported that MAFLD could also have sex specific preferences: several metabolic pathways and other inflammatory processes in the liver are regulated by estrogen receptors (124).
Cytokines and Other Immune Cells Inflammatory Products
As already mentioned, cytokines emerged as key players in severe COVID-19, correlating directly with lung injury and multiorgan failure. Actually, serum IL-6 level has been widely accepted as prognostic marker in COVID-19 as its elevation is the most frequently reported (88). For this reason, tocilizumab, a humanized anti-IL-6-receptor monoclonal antibody, has been proposed as a treatment for severe COVID-19 and used as an emergency and compassionate treatment (125, 126). It is currently under evaluation in clinical trials, with promising preliminary results (127, 128). Several clinical trials are also evaluating the efficacy of IL-1β inhibitors, such as anakinra and canakinumab, in preventing COVID-19 pneumonia and its associated cytokine storm; but, for now, the only evidence came from CAN-COVID interim analysis, in which canakinumab has failed to meet primary endpoints (129). Nonetheless, the cytokine storm involves lots of different pro-inflammatory cytokines; therefore, general immunosuppression could be even more effective. At this regard, corticosteroids have also been evaluated for the treatment of severe COVID-19 and, although earlier meta-analysis conclude with negative results (130), CoDEX clinical trial showed increased ventilator-free days in treated patients (131) and the WHO REACT Working Group further support lower 28-day all-cause mortality in critically ill COVID-19 patients receiving systemic corticosteroids (132). An important issue in the context of cytokine inhibition is to determine the right timing for treatments. For instance, IFN has been recommended as first-line antiviral in SARS-CoV-2 infection, although evidence is weak for now (133). Taking into consideration that MAFLD patients have increased basal levels of circulating cytokines, these patients may benefit for early intervention with immunosuppressive therapy. However, liver functions may be compromised in MAFLD patients and, accordingly, any therapeutic intervention should consider that drug metabolism could be impaired and further result in liver injury (134, 135). On the other hand, as mentioned previously, the immune response is dynamic and it is possible that many of the failed anti-cytokine therapies in COVID-19 may relate to the time of introduction of the treatment in the course of the disease.
Figure 2 summarizes the main concepts discussed in this section.
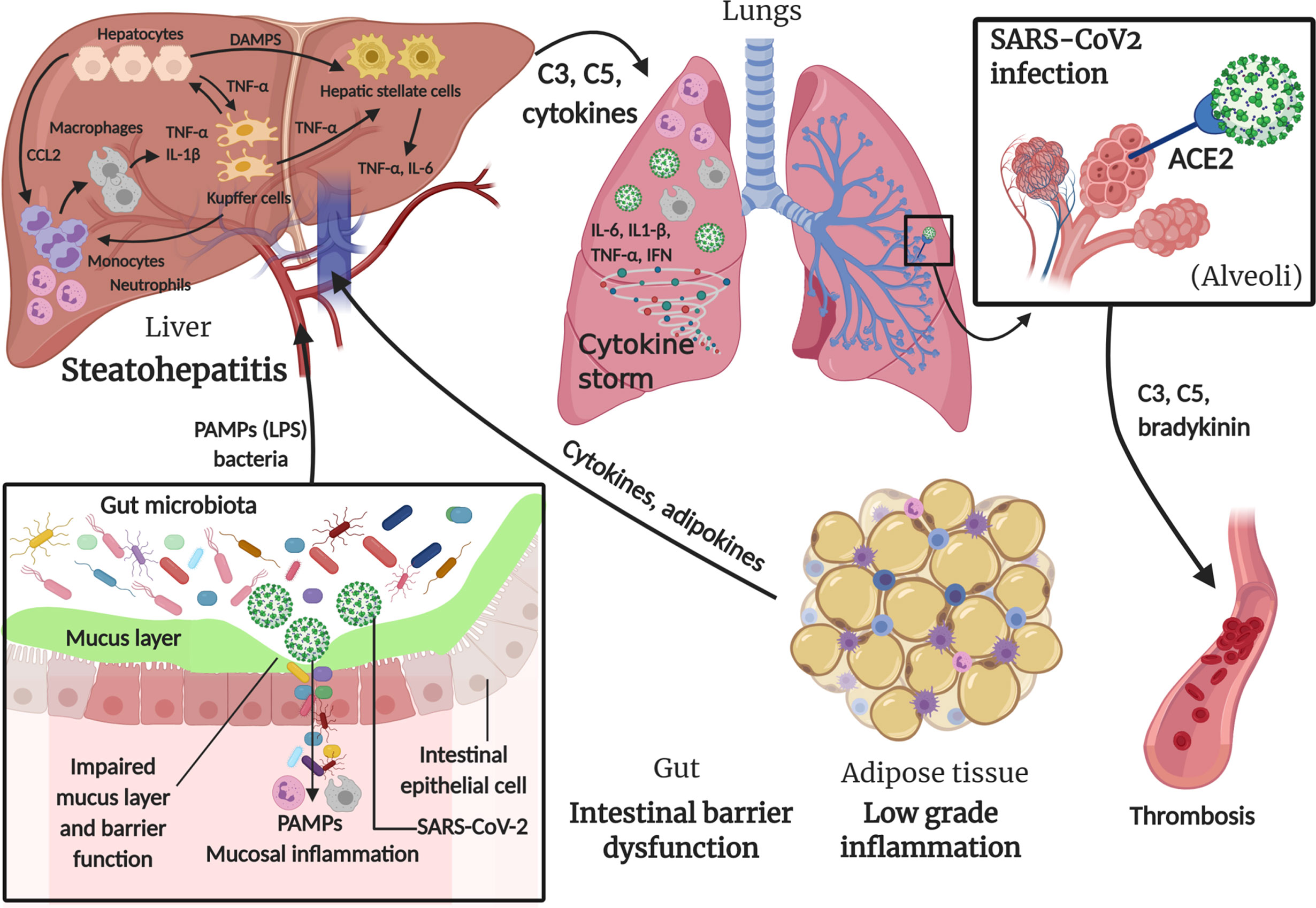
Figure 2 Involvement of innate immune system in MAFLD and SARS-CoV-2 infection. In metabolic associated fatty liver disease (MAFLD), intestinal barrier dysfunction caused by intrinsic endotoxemia due to alterations in gut microbiota, adipose tissue low grade inflammation and the excess of fat in hepatocytes, lead to a series of events triggering the innate immune response in order to restore the perturbed environment. SARS-CoV-2 infection also contributes to intestinal mucosal inflammation, in addition to main lung inflammation caused by the virus entry through angiotensin-converting enzyme 2 (ACE2) in alveoli, which can also lead to thrombosis through a “bradykinin storm”. All these triggers lead to a massive “cytokine storm” with multiorgan effect. Created with BioRender.com.
Alterations of the Adaptive Immune Response
Role of Adaptive Immune Responses and Innate-Adaptive Interplay in MAFLD
Although the implication of innate immune response and inflammation in MAFLD progression seems clear, recent findings have also uncovered a role of adaptive immune responses. As stated before, lipid accumulation in hepatocytes is an early finding in MAFLD and ROS generation is considered one of the main drivers of the diseases. In this sense, oxidized phospholipids and reactive aldehydes generated during lipid peroxidation form antigenic adducts with cellular macromolecules known as oxidative stress derived epitopes (OSEs) (136). These epitopes trigger both humoral and cellular adaptive immune responses. Much of the role of adaptive immunity in MAFLD is supported by the results obtained from different experimental models, including obesity related MAFLD (mice under different types of HFD) and NASH development in lean individuals (such as mice in methionine and choline deficient diet or similar diets, MCD). In this sense, comparison between mice living in specific pathogen-free (SPF) conditions with those housed on non-SPF showed that the latter had higher memory and effector T cells both under normal and HFD. These results were also observed in the liver: more than 95 and 85% of CD4+ and CD8+ T cells, respectively, expressed the effector memory T cell phenotype in non-SPF mice on HFD, which was accompanied by severe steatosis, lobular inflammation, hepatocellular ballooning and destroyed lobule structure while only some SPF mice displayed a mild fat accumulation in the liver (137). On the other hand, mice following a HFD showed reduced antigen-specific humoral and cellular immune responses after receiving hepatitis B vaccine due to diminish antigen processing and presentation (138). Recently, it has been also reported that follicular T helper cells are impaired in patients with advanced liver cirrhosis due to increased IL-2 signaling (139). These results give an early notion that adaptive immunity activation directly impact on MAFLD severity and, at the same time, MAFLD and cirrhosis result in impaired adaptive immunity activation towards other antigens. In this sense, exploring the immune response to SARS-CoV-2 vaccination seems mandatory in MAFLD and comparison between obese and non-obese patients.
Elevated titers of IgG anti-OSEs such as malondialdehyde adduct with human serum albumin (MDA-HSA), arachidonic acid hydroperoxide adduct with human serum albumin (AAHP-HSA) and oxidized cardiolipin (Ox-CL) have been found in MAFLD patients compared with controls. Moreover, 29–39% of total MAFLD patients had anti-OSEs IgG titers above the 97.5th percentile in controls, which defines a positive titer. MAFLD patients with positive anti-MDA-HSA antibodies had a threefold higher risk of having advanced fibrosis or cirrhosis compared with patients whose antibody titers were within the control range (140). Similar results were observed in pediatric MAFLD patients; in this case, authors found that 63% of patients had circulating IgG against anti-MDA-HSA above the control threshold. At the histology, patients with elevated anti-MDA-HSA antibodies showed higher scores of lobular inflammation than subjects with antibodies within control range (141). In an independent and more recent study, humoral immunity against OSE was confirmed by measuring circulating IgG anti-MDA adducts. They found that 43% of MAFLD/NASH patients had titers of anti-OSE IgG above the control threshold. The prevalence of advanced fibrosis or cirrhosis was higher among the subjects with elevated anti-OSE IgG (142). In this line, studies with MCD fed mice showed that the extension of liver injury and lobular inflammation paralleled the development of anti-MDA IgG antibodies and CD4+ and CD8+ T-lymphocytes responsive to the same antigens. Besides, further treatment with MDA-adducted bovine serum albumin stimulated transaminase release, lobular inflammation, and the hepatic expression of proinflammatory cytokines in MCD-fed mice, involving liver recruitment of the Th1 cells (143). These studies point out the early implication of humoral immunity in the recruitment of immune cells to the liver in MAFLD and the sustained autoimmune response as a factor contributing to disease progression. On the contrary, in a smaller cohort, Hendrikx et al. described no differences in plasma IgG anti-OSEs titers in MAFLD patients and healthy controls. Besides, they found decreased IgM anti-OSEs titers in MAFLD patients, although only IgM titers towards the specific malondialdehydeacetaldehyde P1 mimotope remained significant after adjusting for total IgM levels. They also showed that IgM titers against P1 mimotope inversely correlate with markers of obesity, systemic inflammation and liver damage, gaining a protective role (144). Moreover, low anti-adipocyte IgG antibodies have been observed in MAFLD patients in comparison to controls, whereas anti-adipocyte IgM antibodies were increased and correlated with portal inflammation (145). Finally, autoimmune hepatitis and common autoantibodies are more prevalent in MAFLD patients than in the general population (146–149), although its relevance in disease progression is unclear (149, 150). Thus, humoral response in human MAFLD is still controversial and deserves further research.
Cellular adaptive responses have been also described in MAFLD patients. 63% of MAFLD/NASH patients showed CD20+ B-cell and CD3+ T-cell aggregates in liver biopsies as determined by immunostaining (151). B and T lymphocyte infiltration in the liver of experimental models of MAFLD has been widely described (152–157). Patients with high B-/T-cell infiltration had elevated anti-OSE IgG titers as well as higher scores of lobular inflammation and fibrosis than the subjects with low/mild infiltration. The number and size of lymphocyte aggregates positively correlated with circulating IFN-γ levels, lobular inflammation score and fibrosis staging (142). NASH patients showed increased serum B-cell Activating Factor (BAFF) (158). Although little is known about the role of B lymphocytes in MAFLD pathogenesis, in mice receiving the MCD diet, hepatic B2-lymphocytes significantly declined in parallel with the onset of steatohepatitis and increasing titers of circulating anti-OSE IgG, accompanied by increased markers of lymphocyte activation and plasmablasts and cells expansion. Selective depletion of B2-lymphocytes resulted in reduced liver plasma cell maturation, anti-OSE IgG production, impaired liver recruitment of T lymphocytes, ameliorated lobular inflammation score and the prevalence of necrotic foci without affecting the extension of steatosis upon the induction of NASH. B-cell responses in NASH were associated to the up-regulation in the liver of BAFF. Thus, BAFF neutralization also ameliorated histological scores for steatosis and lobular inflammation as well as ALT release, liver triglycerides and hepatic expression of pro-inflammatory mediators (142). Moreover, B lymphocytes seemed to infiltrate earlier in the liver of HFD mice than T cells (159).
On the contrary, T cell alterations both in peripheral blood and intrahepatic infiltrates from MAFLD patients have been widely described. In this sense, several lines of evidence suggest that NASH should be considered a Th1-polarized disease (64, 74, 160–164). Circulating CD4+ cells rose in adult NASH patients (160, 162), together with an increased proportion of memory CD45RO+ cells and decreased of naïve CD45RA+ (151, 160). Similarly, CD8+CD45RO+ subpopulation was increased while CD8+CD45RA+ declined (151, 160). In pediatric NASH, whereas CD45RO and CD45RA subpopulations were similarly distributed among circulating CD4+, CD8+CD45RO and CD8+CD45RA subpopulations were found simultaneously increased in patients, which refers to a greater pool of CD8 T cells undergoing activation (161). Moreover, a higher frequency of IFN-γ-producing Th1 lymphocytes was observed as well as CD8+ cells retaining a cytotoxic phenotype (160, 161). In liver biopsies, IFN-γ-producing CD8+ cells were also increased both in adult and pediatric NASH (64, 160, 161). CD8+ T lymphocytes localized within inflammatory foci in close proximity to steatotic and ballooned hepatocytes and showed associations with lobular inflammation, ballooning and hepatic genes related to cytotoxic and IFN-γ responses, T helper differentiation and TNF-α signaling, strongly suggesting the presence of a local cytotoxic response in the liver (64). Other authors have observed that also the frequency of IL-4+ Th2 cells among CD4+ T cells was significantly elevated in patients with MAFLD and NASH in comparison with controls (151, 162). Moreover, analysis of intrahepatic lymphocytes showed significantly higher frequencies of intrahepatic IL-17, IL-4, and IFN-γ-producing T cells compared with peripheral blood. The greatest difference between intrahepatic and peripheral T cells was seen for the frequency of IFN-γ + cells among CD4+ T cells both for MAFLD and NASH. In livers from MAFLD and NASH groups, up to 44% of CD4+ T cells expressed the activation marker HLA-DR, thus contributing actively to pathogenesis in situ. However, Th17 cells were more frequent in the liver of patients with NASH in comparison with hepatic tissue from patients with MAFLD, which could differentiate disease stage (162). Regarding regulatory T-cells (Tregs), MAFLD patients showed a significantly lower frequency of naïve Tregs (CD4+CD45RA+CD25++) among CD4+ T cells in peripheral blood in comparison with controls, while NASH patients had an even lower frequency of these cells. The opposite was true for activated Tregs (CD4+CD45RA-CD25+++), suggesting increased turnover/consumption of Tregs in patients as a result of increased activation of the naïve Tregs (162). Another study reported that Foxp3+ Tregs are increased in the liver of NASH patients and its frequency among T cells correlated to higher NASscore (165). Moreover, oral treatment with anti-CD3 monoclonal antibodies improved serum transaminases and fasting plasma glucose in a small cohort of NASH patients through increasing circulatory Tregs (166). Although evidence is still scarce, we can hypothesize that Tregs may get activated and infiltrate to the liver in MAFLD patients as a compensatory mechanism for the enhanced local immune response, which in the context of sustained liver damage could favor the development of liver cancer (167). On the contrary, studies in the liver of HFD fed mice have shown that percentages of CD4+IFN-γ+ Th1 cells and CD4+IL-17+ Th17 cells were increased remarkably, while CD4+CD25+Foxp3+ Treg cells were decreased significantly (168, 169), which parallels the imbalance in T cell activation in the mesenteric lymph node. Chemotaxis of CD4+ from mesenteric lymph nodes to the liver was demonstrated, thus linking gut immunity alterations and MAFLD (168, 170). In this sense, although lymphocyte infiltration in the liver is mainly associated with bad prognosis in MAFLD, several studies in experimental models have shown that CD4+ T cell depletion could increase the risk of HCC development (171–173). On the contrary, maintained CD8+ cytotoxic responses seem to favor carcinogenesis (156, 157).
On the other hand, conflicting results have been reported. For instance, no differences between control, MAFLD and NASH in B cell or T cell populations have been described (174). Other studies have shown a reduction of circulating CD8+ T cells in MAFLD patients in comparison to control healthy subjects (151) or between steatosis stages (175). Moreover, circulating Th2 cells were observed increased while Th1 cells, Th17 cells and Tregs cells had similar frequencies in MAFLD patients compared to healthy controls (151). These contradictory results could be due to the common comorbidities associated to MAFLD, namely DM2 and obesity, and its different representation in the cohorts. Thus, several authors have analyzed the different immune response in MAFLD, DM2 and obese patients. Regarding the humoral response, MAFLD patients diagnosed with diabetes or hyperlipidemia were found to have significantly lower levels of anti-adipocytes IgG antibodies when compared with MAFLD patients with none of the comorbidities. Furthermore, anti-adipocytes IgM correlated positively and significantly with body-mass index while the contrary was true for anti-adipocytes IgG (145). After multiplex determination of cytokines in obese patients, Vonghia et al. concluded that DM2 patients showed a disturbed Th1/Th2 balance towards Th1 polarization, but, at the intrahepatic level, a mixed Th1 and Th2 impairment occurred and Th2 response was common to DM2 and NASH. Moreover, patients with advanced fibrosis showed higher intrahepatic INF-γ and IL-1β, which can stimulate the cells towards a pro-inflammatory Th1 phenotype (176). Besides, other authors stated that the hepatic expression levels of several mediators of the immune response are modified in all morbidly obese patients, regardless steatosis or inflammation, while NASH appears preferentially associated with a better antigen presentation and a Th1 response, highlighting again the relevance of adaptive immunity in MAFLD progression (163). Finally, authors analyzed the association of differentially expressed genes and immune cell populations in a large cohort of obese patients showing common metabolic comorbidities. IL-10+ CD4 T lymphocytes and cytotoxic CD8 T were positively associated with lobular inflammation, ballooning and glucose levels, thus linking NASH activity and DM2. Th2 lymphocytes and Tregs were mostly negatively associated with NASH and glucose parameters (64).
Adaptive Immunity and COVID-19 Severity in MAFLD Patients
Lymphopenia is a common feature of severe COVID-19, characterized by drastically reduced absolute numbers of CD4+ and particularly CD8+ T cells which correlates with COVID-19 severity and associated mortality (177, 178). Levels of T cell surface molecules (CD4, CD8 and CD2), T cell migration stimulators (DDP4), TCR signaling kinases (ZAP70, LCK and FYN) and MHC class II molecules (HLA-DRA, HLA-DRB1, HLA-DRB4 and HLA-DRB5) were also significantly lower in patients with severe disease (179). Peripheral CD8+ T cells from patients with COVID-19 express high levels of exhaustion markers, including programmed cell death protein 1 (PD1) and T cell immunoglobulin mucin-3 (TIM3); of note, this expression pattern was more pronounced among patients who required intensive care than in patients with mild disease (180). Analyses of circulating B cells showed expansion of oligoclonal plasmablasts and reduced memory B cell frequencies in patients with severe COVID-19 compared with responses in patients with mild disease or healthy individuals (181, 182). Taking all together, severe COVID-19 patients show a global impairment in the adaptive immune response. As stated before, adaptive immune responses towards auto-antigens in NASH result in lymphocyte infiltration in the liver, with a pro-inflammatory Th1 phenotype and increased consumption of Tregs. These mean a basal increased exhaustion of the adaptive immune system in NASH patients in comparison with healthy people, which could lead to a worse adaptive response towards SARS-CoV-2 infection. Th1 phenotype is also observed in lungs during SARS-CoV-2 infection, as macrophage phagocytosis of infected alveolar epithelial cells stimulate T cells to secrete IFN-γ and favors alveoli inflammation, forming a detrimental positive loop for severe COVID-19 (134). Moreover, a preliminary study with a Chinese cohort of COVID-19 patients showed that patients with MAFLD and increased neutrophil-to-lymphocyte ratio on admission are at substantially higher risk of severe COVID-19, irrespective of age, sex and metabolic comorbidities, indicating that the inability to transit from innate immune responses to adaptive ones is determinant in the progression of COVID-19 in MAFLD patients (183). Nonetheless, evidence is still very scarce and mechanisms underlying the pathophysiological links between metabolic syndrome and COVID-19 are mainly unproven. Figure 3 summarizes the main concepts discussed in this section.
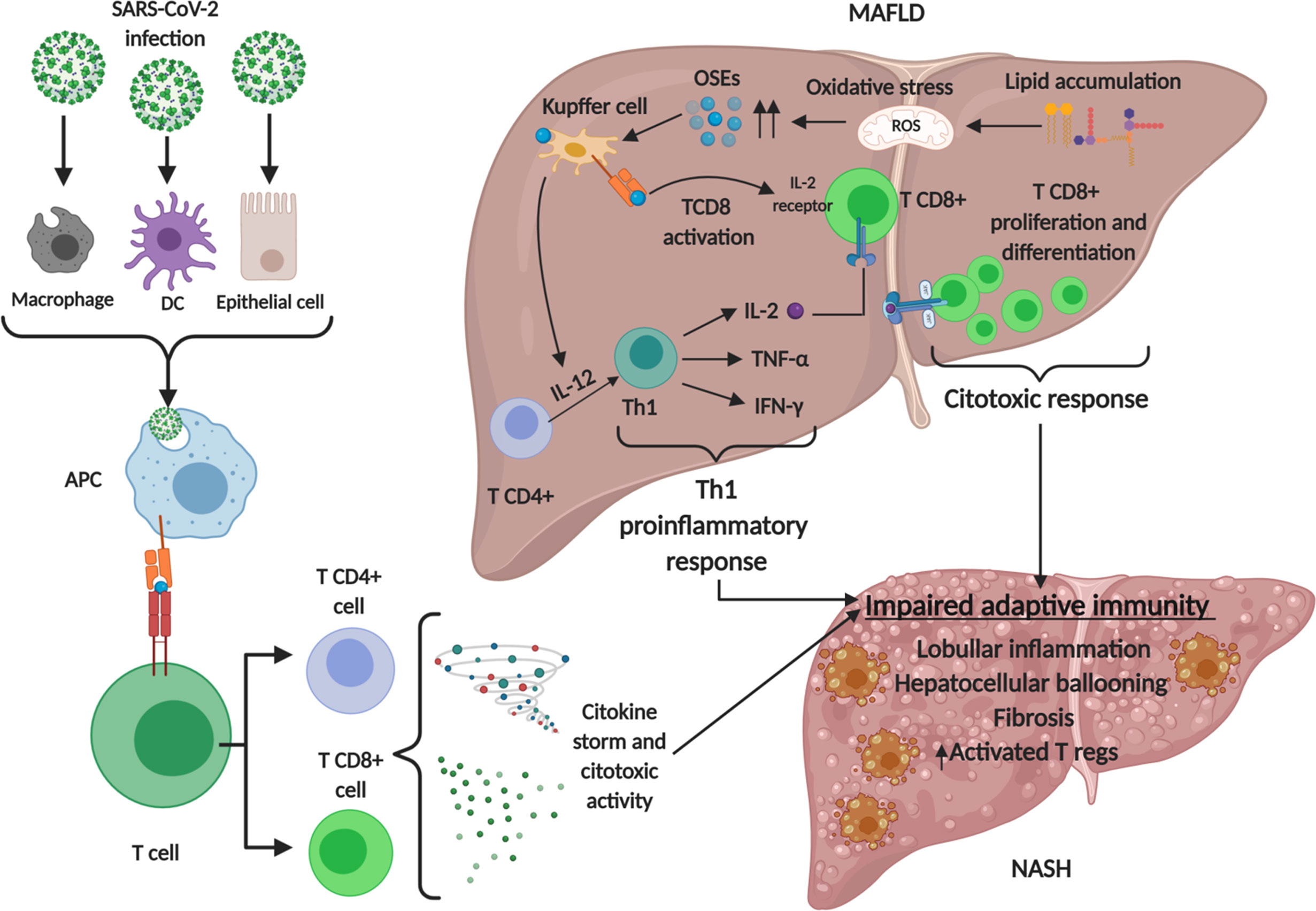
Figure 3 Involvement of adaptive immune system in MAFLD and SARS-CoV-2 infection. Lipid accumulation in metabolic associated fatty liver disease (MAFLD) causes oxidative stress and lipid peroxidation, generating oxidative stress derived epitopes (OSEs). These products lead to adaptive immune stimulating T cells toward Th1 proinflammatory phenotype and activating T CD8 cells which express interleukin 2 (IL-2) receptor to develop a cytotoxic response. These events, together with SARS-CoV-2 infection lead to an impaired adaptive immune response. Regulatory T cells (Tregs) infiltrate to the liver as a compensatory mechanism for the enhanced local immune response. Created with BioRender.com.
Other Factors Involved in MAFLD and COVID-19 Pathophysiology
Other factors could be responsible for the high incidence of severe COVID-19 in MAFLD patients. For instance, ACE2 is normally expressed in low amounts in cholangiocytes and hepatocytes, but it is increased in chronic liver damage and in experimental set-ups of diet-induced MAFLD, where it may exert anti-obesity and anti-inflammatory effects. Therefore, liver injury could lead to increased viral load and worsened effects of COVID-19 (184). In this line of research, SARS-CoV-2 entry factors are differently affected by DM2 and MAFLD in the liver of obese patients. While obese women with DM2 have lower expression of ACE2 and TMPRSS2 than obese normoglycemic women, obese men with NASH show markedly higher levels of these genes (18), which may explain the higher risk of severe COVID-19 in these patients (29). Very preliminary data suggest that liver injury in COVID-19 is more likely due to the exacerbated immune responses than for direct viral infection, but, to affirm this, postmortem liver biopsy was only performed in one patient (30).
Furthermore, it has been shown that COVID-19 severe patients also have downregulation of some classes of metabolites as well as dysfunctional metabolic processes, leading to a loss of important circulating nutrients. This fact, together with high cytokine levels and a proinflammatory environment, contribute to a possible hepatic dysfunction worsening the patient condition (185).
As mentioned before, the liver has special immune characteristics. Besides, its role as a secretory organ, particularly with respect the regulation of coagulation and hemostasis, makes it indispensable for intertissue communication. The steatotic and injured liver could therefore produce hepatokines that may alter the function of other systems, making MAFLD a multi-systemic disease. These molecules have been implicated in the development of increased adipocity, kidney injury, DM2 and cardiovascular disease (186). All these comorbidities are risk factors for severe COVID-19, and therefore evaluating the role of hepatokines in the progression of COVID-19 would be reasonable, but no attempts have been performed until day.
Conclusion
Increasing evidence is confirming an enhanced risk of severe COVID-19 in MAFLD patients, together with other common comorbidities of this disease such as DM2 and obesity. In this sense, a well-established paradigm in MAFLD pathogenesis is the chronic low-grade inflammation, which is the perfect niche for the development of a cytokine storm upon SARS-CoV-2 infection. As discussed along the text, TLR signaling might be sustained in MAFLD and COVID-19, leading to a hyperactivation of neutrophils and macrophages that produce large systemic levels of proinflammatory molecules. Not only regarding cytokines, the dysfunction of the innate immune response is key in both diseases, affecting the integrity of physical barriers, especially in the intestine; and the complement and contact systems, which are also responsible for the severe and long-lasting manifestations of COVID-19. Furthermore, the needed transition between innate and adaptive immune responses seems to be impaired in severe COVID-19. The dysregulation of adaptive responses is already present in MAFLD patients, who also have a proinflammatory T cell response and exhaustion of Tregs. Besides, humoral responses are activated towards auto-antigens in some cases. In this setting, proper adaptive immunity could not be expected.
Taking into consideration all these pathological mechanisms, several therapeutic approaches have been proposed. ACE2 direct activation has been considered to prevent SARS-CoV2 infection. Additionally, the efficacy of anakinra and canakinumab (IL-1β inhibitors) in preventing COVID-19 pneumonia and its associated cytokine storm is also being tested in several clinical trials; but, for now, canakinumab has failed to meet primary endpoints. To keep combating the inflammation, eculizumab is at the moment the only medication approved for humans to prevent the complement cascade in order to ameliorate the pulmonary dysfunction due to COVID-19, but also specific C5aR1 blockade is a very promising therapy to fight against severe COVID-19. General immunosuppression, through inhibition of NF-κB or treatment with corticoids reduces the cytokine storm, helping to diminish the inflammatory environment created by both MAFLD and COVID-19 diseases.
Last of all, immune independent mechanisms can also account for the increased risk of severe COVID-19 in MAFLD patients, but current studies are very limited.
Author Contributions
Conceptualization, JC and ML-H. Writing—original draft preparation, PL and MA-P. Writing—review and editing, DS, MA-L, JC, and ML-H. All authors contributed to the article and approved the submitted version.
Funding
This research received funding by the ISCIII (COV20/0170 and PI19/01509) and Cantabria Goverment (2020 UIC22-PUB-0019) to ML-H.
Conflict of Interest
The authors declare that the research was conducted in the absence of any commercial or financial relationships that could be construed as a potential conflict of interest.
References
1. Machhi J, Herskovitz J, Senan AM, Dutta D, Nath B, Oleynikov MD, et al. The Natural History, Pathobiology, and Clinical Manifestations of SARS-CoV-2 Infections. J Neuroimmune Pharmacol (2020) 15(3):359–86. doi: 10.1007/s11481-020-09944-5
2. Perico L, Benigni A, Casiraghi F, Ng LFP, Renia L, Remuzzi G. Immunity, endothelial injury and complement-induced coagulopathy in COVID-19. Nat Rev Nephrol (2021) 17:46–64. doi: 10.1038/s41581-020-00357-4
3. Hoffmann M, Kleine-Weber H, Schroeder S, Krüger N, Herrler T, Erichsen S, et al. SARS-CoV-2 Cell Entry Depends on ACE2 and TMPRSS2 and Is Blocked by a Clinically Proven Protease Inhibitor. Cell (2020) 181(2):271–80.e8. doi: 10.1016/j.cell.2020.02.052
4. Amraie R, Napoleon MA, Yin W, Berrigan J, Suder E, Zhao G, et al. CD209L/L-SIGN and CD209/DC-SIGN act as receptors for SARS-CoV-2 and are differentially expressed in lung and kidney epithelial and endothelial cells. bioRxiv (2020). doi: 10.1101/2020.06.22.165803
5. Zang R, Gomez Castro MF, McCune BT, Zeng Q, Rothlauf PW, Sonnek NM, et al. TMPRSS2 and TMPRSS4 promote SARS-CoV-2 infection of human small intestinal enterocytes. Sci Immunol (2020) 5(47):eabc3582. doi: 10.1126/sciimmunol.abc3582
6. Ou X, Liu Y, Lei X, Li P, Mi D, Ren L, et al. Characterization of spike glycoprotein of SARS-CoV-2 on virus entry and its immune cross-reactivity with SARS-CoV. Nat Commun (2020) 11(1):1620. doi: 10.1038/s41467-020-15562-9
7. Simmons G, Gosalia DN, Rennekamp AJ, Reeves JD, Diamond SL, Bates P. Inhibitors of cathepsin L prevent severe acute respiratory syndrome coronavirus entry. Proc Natl Acad Sci U S A (2005) 102(33):11876–81. doi: 10.1073/pnas.0505577102
8. Cantuti-Castelvetri L, Ojha R, Pedro LD, Djannatian M, Franz J, Kuivanen S, et al. Neuropilin-1 facilitates SARS-CoV-2 cell entry and infectivity. Science (2020) 370(6518):856–60. doi: 10.1126/science.abd2985
9. Matsuyama S, Nao N, Shirato K, Kawase M, Saito S, Takayama I, et al. Enhanced isolation of SARS-CoV-2 by TMPRSS2-expressing cells. Proc Natl Acad Sci U S A (2020) 117(13):7001–3. doi: 10.1073/pnas.2002589117
10. Ye Q, Wang B, Mao J. The pathogenesis and treatment of the `Cytokine Storm’ in COVID-19. J Infect (2020) 80(6):607–13. doi: 10.1016/j.jinf.2020.03.037
11. Wu T, Zuo Z, Kang S, Jiang L, Luo X, Xia Z, et al. Multi-organ Dysfunction in Patients with COVID-19: A Systematic Review and Meta-analysis. Aging Dis (2020) 11(4):874–94. doi: 10.14336/AD.2020.0520
12. Garvin MR, Alvarez C, Miller JI, Prates ET, Walker AM, Amos BK, et al. A mechanistic model and therapeutic interventions for COVID-19 involving a RAS-mediated bradykinin storm. Elife (2020) 9:e59177. doi: 10.7554/eLife.59177
13. Pan L, Mu M, Yang P, Sun Y, Wang R, Yan J, et al. Clinical Characteristics of COVID-19 Patients With Digestive Symptoms in Hubei, China: A Descriptive, Cross-Sectional, Multicenter Study. Am J Gastroenterol (2020) 115(5):766–73. doi: 10.14309/ajg.0000000000000620
14. Xie H, Zhao J, Lian N, Lin S, Xie Q, Zhuo H. Clinical characteristics of non-ICU hospitalized patients with coronavirus disease 2019 and liver injury: A retrospective study. Liver Int (2020) 40(6):1321–6. doi: 10.1111/liv.14449
15. Xu L, Liu J, Lu M, Yang D, Zheng X. Liver injury during highly pathogenic human coronavirus infections. Liver Int (2020) 40(5):998–1004. doi: 10.1111/liv.14435
16. Liang W, Feng Z, Rao S, Xiao C, Xue X, Lin Z, et al. Diarrhoea may be underestimated: a missing link in 2019 novel coronavirus. Gut (2020) 69(6):1141–3. doi: 10.1136/gutjnl-2020-320832
17. Xiao F, Tang M, Zheng X, Liu Y, Li X, Shan H. Evidence for Gastrointestinal Infection of SARS-CoV-2. Gastroenterology (2020) 158(6):1831–3.e3. doi: 10.1053/j.gastro.2020.02.055
18. Fondevila MF, Mercado-Gómez M, Rodríguez A, Gonzalez-Rellan MJ, Iruzubieta P, Valentí V, et al. Obese patients with NASH have increased hepatic expression of SARS-CoV-2 critical entry points. J Hepatol (2020) 73(3):717–18. doi: 10.1016/j.jhep.2020.09.027
19. Kubes P, Jenne C. Immune Responses in the Liver. Annu Rev Immunol (2018) 36:247–77. doi: 10.1146/annurev-immunol-051116-052415
20. Maffetone PB, Laursen PB. The Perfect Storm: Coronavirus (Covid-19) Pandemic Meets Overfat Pandemic. Front Public Health (2020) 8:135. doi: 10.3389/fpubh.2020.00135
21. Eslam M, Sanyal AJ, George J, Panel IC. MAFLD: A Consensus-Driven Proposed Nomenclature for Metabolic Associated Fatty Liver Disease. Gastroenterology (2020) 158(7):1999–2014.e1. doi: 10.1053/j.gastro.2019.11.312
22. Eslam M, Newsome PN, Sarin SK, Anstee QM, Targher G, Romero-Gomez M, et al. A new definition for metabolic dysfunction-associated fatty liver disease: An international expert consensus statement. J Hepatol (2020) 73(1):202–9. doi: 10.1016/j.jhep.2020.07.045
23. O’Hara J, Finnegan A, Dhillon H, Ruiz-Casas L, Pedra G, Franks B, et al. Cost of non-alcoholic steatohepatitis in Europe and the USA: The GAIN study. JHEP Rep (2020) 2(5):100142. doi: 10.1016/j.jhepr.2020.100142
24. O’Rourke RW, Lumeng CN. Pathways to Severe COVID-19 for People with Obesity. Obesity (Silver Spring) (2020). doi: 10.1002/oby.23099
25. Lim S, Bae JH, Kwon HS, Nauck MA. COVID-19 and diabetes mellitus: from pathophysiology to clinical management. Nat Rev Endocrinol (2021) 17(1):11–30. doi: 10.1038/s41574-020-00435-4
26. Gómez-Hurtado I, Gallego-Durán R, Zapater P, Ampuero J, Aller R, Crespo J, et al. Bacterial antigen translocation and age as BMI-independent contributing factors on systemic inflammation in NAFLD patients. Liver Int (2020) 40(9):2182–93. doi: 10.1111/liv.14571
27. Sookoian S, Salatino A, Castaño GO, Landa MS, Fijalkowky C, Garaycoechea M, et al. Intrahepatic bacterial metataxonomic signature in non-alcoholic fatty liver disease. Gut (2020) 69(8):1483–91. doi: 10.1136/gutjnl-2019-318811
28. Roca-Fernandez A, Dennis A, Nicolls R, McGonigle J, Kelly M, Banerjee R. High Liver Fat Associates With Higher Risk Of Developing Symptomatic COVID-19 Infection - Initial UK Biobank Observations. medRxiv (2020). 2020.06.04.20122457. doi: 10.1101/2020.06.04.20122457
29. Zheng KI, Gao F, Wang XB, Sun QF, Pan KH, Wang TY, et al. Letter to the Editor: Obesity as a risk factor for greater severity of COVID-19 in patients with metabolic associated fatty liver disease. Metabolism (2020) 108:154244. doi: 10.1016/j.metabol.2020.154244
30. Ji D, Qin E, Xu J, Zhang D, Cheng G, Wang Y, et al. Non-alcoholic fatty liver diseases in patients with COVID-19: A retrospective study. J Hepatol (2020) 73(2):451–3. doi: 10.1016/j.jhep.2020.03.044
31. Zhou YJ, Zheng KI, Wang XB, Sun QF, Pan KH, Wang TY, et al. Metabolic-associated fatty liver disease is associated with severity of COVID-19. Liver Int (2020) 40(9):2160–3. doi: 10.1111/liv.14575
32. Targher G, Mantovani A, Byrne CD, Wang XB, Yan HD, Sun QF, et al. Risk of severe illness from COVID-19 in patients with metabolic dysfunction-associated fatty liver disease and increased fibrosis scores. Gut (2020) 69(8):1545–7. doi: 10.1136/gutjnl-2020-321611
33. Gao F, Zheng KI, Wang XB, Yan HD, Sun QF, Pan KH, et al. Metabolic associated fatty liver disease increases coronavirus disease 2019 disease severity in nondiabetic patients. J Gastroenterol Hepatol (2020) 36(1):204–7. doi: 10.1111/jgh.15112
34. Zhou YJ, Zheng KI, Wang XB, Yan HD, Sun QF, Pan KH, et al. Younger patients with MAFLD are at increased risk of severe COVID-19 illness: A multicenter preliminary analysis. J Hepatol (2020) 73(3):719–21. doi: 10.1016/j.jhep.2020.04.027
35. Pan L, Huang P, Xie X, Xu J, Guo D, Jiang Y. Metabolic associated fatty liver disease increases the severity of COVID-19: A meta-analysis. Dig Liver Dis (2021) 53(2):153–7. doi: 10.1016/j.dld.2020.09.007
36. Sharma P, Kumar A. Metabolic dysfunction associated fatty liver disease increases risk of severe Covid-19. Diabetes Metab Syndr (2020) 14(5):825–7. doi: 10.1016/j.dsx.2020.06.013
37. Taylor RS, Taylor RJ, Bayliss S, Hagström H, Nasr P, Schattenberg JM, et al. Association Between Fibrosis Stage and Outcomes of Patients With Nonalcoholic Fatty Liver Disease: A Systematic Review and Meta-Analysis. Gastroenterology (2020) 158(6):1611–25.e12. doi: 10.1053/j.gastro.2020.01.043
38. Bajaj JS, Garcia-Tsao G, Biggins SW, Kamath PS, Wong F, McGeorge S, et al. Comparison of mortality risk in patients with cirrhosis and COVID-19 compared with patients with cirrhosis alone and COVID-19 alone: multicentre matched cohort. Gut (2021) 70(3):531–6. doi: 10.1136/gutjnl-2020-322118
39. Marjot T, Moon AM, Cook JA, Abd-Elsalam S, Aloman C, Armstrong MJ, et al. Outcomes following SARS-CoV-2 infection in patients with chronic liver disease: an international registry study. J Hepatol (2021) 74(3):567–77. doi: 10.1016/j.jhep.2020.09.024
40. Arrese M, Cabrera D, Kalergis AM, Feldstein AE. Innate Immunity and Inflammation in NAFLD/NASH. Dig Dis Sci (2016) 61(5):1294–303. doi: 10.1007/s10620-016-4049-x
41. Cai J, Zhang XJ, Li H. Role of Innate Immune Signaling in Non-Alcoholic Fatty Liver Disease. Trends Endocrinol Metab (2018) 29(10):712–22. doi: 10.1016/j.tem.2018.08.003
42. N Rezae, A Aghamohammadi, LD Notarangelo. (eds.). Primary Immunodeficiency Diseases. Berlin, Heidelberg: Springer (2017).
43. Gabay C, Kushner I. Acute-phase proteins and other systemic responses to inflammation. N Engl J Med (1999) 340(6):448–54. doi: 10.1056/NEJM199902113400607
44. Jia Q, Li C, Xia Y, Zhang Q, Wu H, Du H, et al. Association between complement C3 and prevalence of fatty liver disease in an adult population: a cross-sectional study from the Tianjin Chronic Low-Grade Systemic Inflammation and Health (TCLSIHealth) cohort study. PloS One (2015) 10(4):e0122026. doi: 10.1371/journal.pone.0122026
45. Lee JH, Poudel B, Ki HH, Nepali S, Lee YM, Shin JS, et al. Complement C1q stimulates the progression of hepatocellular tumor through the activation of discoidin domain receptor 1. Sci Rep (2018) 8(1):4908. doi: 10.1038/s41598-018-23240-6
46. Rensen SS, Slaats Y, Driessen A, Peutz-Kootstra CJ, Nijhuis J, Steffensen R, et al. Activation of the complement system in human nonalcoholic fatty liver disease. Hepatology (2009) 50(6):1809–17. doi: 10.1002/hep.23228
47. Segers FM, Verdam FJ, de Jonge C, Boonen B, Driessen A, Shiri-Sverdlov R, et al. Complement alternative pathway activation in human nonalcoholic steatohepatitis. PloS One (2014) 9(10):e110053. doi: 10.1371/journal.pone.0110053
48. Nicola H. The role of contact system in septic shock: the next target? An overview of the current evidence. J Intensive Care (2017) 5:31. doi: 10.1186/s40560-017-0228-x
49. Kotronen A, Joutsi-Korhonen L, Sevastianova K, Bergholm R, Hakkarainen A, Pietiläinen KH, et al. Increased coagulation factor VIII, IX, XI and XII activities in non-alcoholic fatty liver disease. Liver Int (2011) 31(2):176–83. doi: 10.1111/j.1478-3231.2010.02375.x
50. Arias-Loste MT, Fábrega E, López-Hoyos M, Crespo J. The Crosstalk between Hypoxia and Innate Immunity in the Development of Obesity-Related Nonalcoholic Fatty Liver Disease. BioMed Res Int (2015) 2015:319745. doi: 10.1155/2015/319745
51. Kim S, Park S, Kim B, Kwon J. Toll-like receptor 7 affects the pathogenesis of non-alcoholic fatty liver disease. Sci Rep (2016) 6:27849. doi: 10.1038/srep27849
52. Akira S, Uematsu S, Takeuchi O. Pathogen recognition and innate immunity. Cell (2006) 124(4):783–801. doi: 10.1016/j.cell.2006.02.015
53. Creely SJ, McTernan PG, Kusminski CM, Fisher F, Da Silva NF, Khanolkar M, et al. Lipopolysaccharide activates an innate immune system response in human adipose tissue in obesity and type 2 diabetes. Am J Physiol Endocrinol Metab (2007) 292(3):E740–7. doi: 10.1152/ajpendo.00302.2006
54. Douhara A, Moriya K, Yoshiji H, Noguchi R, Namisaki T, Kitade M, et al. Reduction of endotoxin attenuates liver fibrosis through suppression of hepatic stellate cell activation and remission of intestinal permeability in a rat non-alcoholic steatohepatitis model. Mol Med Rep (2015) 11(3):1693–700. doi: 10.3892/mmr.2014.2995
55. Pendyala S, Walker JM, Holt PR. A high-fat diet is associated with endotoxemia that originates from the gut. Gastroenterology (2012) 142(5):1100–.e2. doi: 10.1053/j.gastro.2012.01.034
56. Dasu MR, Jialal I. Free fatty acids in the presence of high glucose amplify monocyte inflammation via Toll-like receptors. Am J Physiol Endocrinol Metab (2011) 300(1):E145–54. doi: 10.1152/ajpendo.00490.2010
57. Dapito DH, Mencin A, Gwak GY, Pradere JP, Jang MK, Mederacke I, et al. Promotion of hepatocellular carcinoma by the intestinal microbiota and TLR4. Cancer Cell (2012) 21(4):504–16. doi: 10.1016/j.ccr.2012.02.007
58. Taira R, Yamaguchi S, Shimizu K, Nakamura K, Ayabe T, Taira T. Bacterial cell wall components regulate adipokine secretion from visceral adipocytes. J Clin Biochem Nutr (2015) 56(2):149–54. doi: 10.3164/jcbn.14-74
59. Raman M, Ahmed I, Gillevet PM, Probert CS, Ratcliffe NM, Smith S, et al. Fecal microbiome and volatile organic compound metabolome in obese humans with nonalcoholic fatty liver disease. Clin Gastroenterol Hepatol (2013) 11(7):868–75.e1-3. doi: 10.1016/j.cgh.2013.02.015
60. Arias-Loste MT, Iruzubieta P, Puente Á, Ramos D, Santa Cruz C, Estébanez Á, et al. Increased Expression Profile and Functionality of TLR6 in Peripheral Blood Mononuclear Cells and Hepatocytes of Morbidly Obese Patients with Non-Alcoholic Fatty Liver Disease. Int J Mol Sci (2016) 17(11):1878. doi: 10.3390/ijms17111878
61. Roh YS, Park S, Kim JW, Lim CW, Seki E, Kim B. Toll-like receptor 7-mediated type I interferon signaling prevents cholestasis- and hepatotoxin-induced liver fibrosis. Hepatology (2014) 60(1):237–49. doi: 10.1002/hep.26981
62. Wan X, Xu C, Yu C, Li Y. Role of NLRP3 Inflammasome in the Progression of NAFLD to NASH. Can J Gastroenterol Hepatol (2016) 2016:6489012. doi: 10.1155/2016/6489012
63. Luo W, Xu Q, Wang Q, Wu H, Hua J. Effect of modulation of PPAR-γ activity on Kupffer cells M1/M2 polarization in the development of non-alcoholic fatty liver disease. Sci Rep (2017) 7:44612. doi: 10.1038/srep44612
64. Haas JT, Vonghia L, Mogilenko DA, Verrijken A, Molendi-Coste O, Fleury S, et al. Transcriptional Network Analysis Implicates Altered Hepatic Immune Function in NASH development and resolution. Nat Metab (2019) 1(6):604–14. doi: 10.1038/s42255-019-0076-1
65. Ganz M, Szabo G. Immune and inflammatory pathways in NASH. Hepatol Int (2013) 7 Suppl 2:771–81. doi: 10.1007/s12072-013-9468-6
66. Tian Z, Chen Y, Gao B. Natural killer cells in liver disease. Hepatology (2013) 57(4):1654–62. doi: 10.1002/hep.26115
67. Kumar V. NKT-cell subsets: promoters and protectors in inflammatory liver disease. J Hepatol (2013) 59(3):618–20. doi: 10.1016/j.jhep.2013.02.032
68. Martin-Murphy BV, You Q, Wang H, De La Houssaye BA, Reilly TP, Friedman JE, et al. Mice lacking natural killer T cells are more susceptible to metabolic alterations following high fat diet feeding. PloS One (2014) 9(1):e80949. doi: 10.1371/journal.pone.0080949
69. Tajiri K, Shimizu Y, Tsuneyama K, Sugiyama T. Role of liver-infiltrating CD3+CD56+ natural killer T cells in the pathogenesis of nonalcoholic fatty liver disease. Eur J Gastroenterol Hepatol (2009) 21(6):673–80. doi: 10.1097/MEG.0b013e32831bc3d6
70. Mridha AR, Wree A, Robertson AAB, Yeh MM, Johnson CD, Van Rooyen DM, et al. NLRP3 inflammasome blockade reduces liver inflammation and fibrosis in experimental NASH in mice. J Hepatol (2017) 66(5):1037–46. doi: 10.1016/j.jhep.2017.01.022
71. Henning JR, Graffeo CS, Rehman A, Fallon NC, Zambirinis CP, Ochi A, et al. Dendritic cells limit fibroinflammatory injury in nonalcoholic steatohepatitis in mice. Hepatology (2013) 58(2):589–602. doi: 10.1002/hep.26267
72. Talukdar S, Oh DY, Bandyopadhyay G, Li D, Xu J, McNelis J, et al. Neutrophils mediate insulin resistance in mice fed a high-fat diet through secreted elastase. Nat Med (2012) 18(9):1407–12. doi: 10.1038/nm.2885
73. Miura K, Yang L, van Rooijen N, Brenner DA, Ohnishi H, Seki E. Toll-like receptor 2 and palmitic acid cooperatively contribute to the development of nonalcoholic steatohepatitis through inflammasome activation in mice. Hepatology (2013) 57(2):577–89. doi: 10.1002/hep.26081
74. Luo XY, Takahara T, Kawai K, Fujino M, Sugiyama T, Tsuneyama K, et al. IFN-γ deficiency attenuates hepatic inflammation and fibrosis in a steatohepatitis model induced by a methionine- and choline-deficient high-fat diet. Am J Physiol Gastrointest Liver Physiol (2013) 305(12):G891–9. doi: 10.1152/ajpgi.00193.2013
75. Hadinia A, Doustimotlagh AH, Goodarzi HR, Arya A, Jafarinia M. Circulating Levels of Pro-inflammatory Cytokines in Patients with Nonalcoholic Fatty Liver Disease and Non-Alcoholic Steatohepatitis. Iran J Immunol (2019) 16(4):327–33. doi: 10.22034/IJI.2019.80284
76. Kar S, Paglialunga S, Jaycox SH, Islam R, Paredes AH. Assay validation and clinical performance of chronic inflammatory and chemokine biomarkers of NASH fibrosis. PloS One (2019) 14(7):e0217263. doi: 10.1371/journal.pone.0217263
77. Pradhan AD, Manson JE, Rifai N, Buring JE, Ridker PM. C-reactive protein, interleukin 6, and risk of developing type 2 diabetes mellitus. JAMA (2001) 286(3):327–34. doi: 10.1001/jama.286.3.327
78. Crespo J, Cayón A, Fernández-Gil P, Hernández-Guerra M, Mayorga M, Domínguez-Díez A, et al. Gene expression of tumor necrosis factor alpha and TNF-receptors, p55 and p75, in nonalcoholic steatohepatitis patients. Hepatology (2001) 34(6):1158–63. doi: 10.1053/jhep.2001.29628
79. du Plessis J, Korf H, van Pelt J, Windmolders P, Vander Elst I, Verrijken A, et al. Pro-Inflammatory Cytokines but Not Endotoxin-Related Parameters Associate with Disease Severity in Patients with NAFLD. PloS One (2016) 11(12):e0166048. doi: 10.1371/journal.pone.0166048
80. Ofei F, Hurel S, Newkirk J, Sopwith M, Taylor R. Effects of an engineered human anti-TNF-alpha antibody (CDP571) on insulin sensitivity and glycemic control in patients with NIDDM. Diabetes (1996) 45(7):881–5. doi: 10.2337/diabetes.45.7.881
81. Yu J, Ip E, Dela Peña A, Hou JY, Sesha J, Pera N, et al. COX-2 induction in mice with experimental nutritional steatohepatitis: Role as pro-inflammatory mediator. Hepatology (2006) 43(4):826–36. doi: 10.1002/hep.21108
82. Brenner DA, Seki E, Taura K, Kisseleva T, Deminicis S, Iwaisako K, et al. Non-alcoholic steatohepatitis-induced fibrosis: Toll-like receptors, reactive oxygen species and Jun N-terminal kinase. Hepatol Res (2011) 41(7):683–6. doi: 10.1111/j.1872-034X.2011.00814.x
83. Dev A, Iyer S, Razani B, Cheng G. NF-κB and innate immunity. Curr Top Microbiol Immunol (2011) 349:115–43. doi: 10.1007/82_2010_102
84. Czaja MJ. JNK regulation of hepatic manifestations of the metabolic syndrome. Trends Endocrinol Metab (2010) 21(12):707–13. doi: 10.1016/j.tem.2010.08.010
85. Gehrke N, Schattenberg JM. Metabolic Inflammation-A Role for Hepatic Inflammatory Pathways as Drivers of Comorbidities in Nonalcoholic Fatty Liver Disease? Gastroenterology (2020) 158(7):1929–47.e6. doi: 10.1053/j.gastro.2020.02.020
86. Francque SM, van der Graaff D, Kwanten WJ. Non-alcoholic fatty liver disease and cardiovascular risk: Pathophysiological mechanisms and implications. J Hepatol (2016) 65(2):425–43. doi: 10.1016/j.jhep.2016.04.005
87. Siddiqi HK, Mehra MR. COVID-19 illness in native and immunosuppressed states: A clinical-therapeutic staging proposal. J Heart Lung Transplant (2020) 39(5):405–7. doi: 10.1016/j.healun.2020.03.012
88. Ragab D, Salah Eldin H, Taeimah M, Khattab R, Salem R. The COVID-19 Cytokine Storm; What We Know So Far. Front Immunol (2020) 11:1446. doi: 10.3389/fimmu.2020.01446
89. Li G, Fan Y, Lai Y, Han T, Li Z, Zhou P, et al. Coronavirus infections and immune responses. J Med Virol (2020) 92(4):424–32. doi: 10.1002/jmv.25685
90. Campbell CM, Kahwash R. Will Complement Inhibition Be the New Target in Treating COVID-19-Related Systemic Thrombosis? Circulation (2020) 141(22):1739–41. doi: 10.1161/CIRCULATIONAHA.120.047419
91. Carvelli J, Demaria O, Vély F, Batista L, Chouaki Benmansour N, Fares J, et al. Association of COVID-19 inflammation with activation of the C5a-C5aR1 axis. Nature (2020) 588:146–50. doi: 10.1038/s41586-020-2600-6
92. Gralinski LE, Sheahan TP, Morrison TE, Menachery VD, Jensen K, Leist SR, et al. Complement Activation Contributes to Severe Acute Respiratory Syndrome Coronavirus Pathogenesis. mBio (2018) 9(5):e01753–18. doi: 10.1128/mBio.01753-18
93. Lefere S, Tacke F. Macrophages in obesity and non-alcoholic fatty liver disease: Crosstalk with metabolism. JHEP Rep (2019) 1(1):30–43. doi: 10.1016/j.jhepr.2019.02.004
94. Rodríguez-Puertas R. ACE2 activators for the treatment of COVID 19 patients. J Med Virol (2020) 92(10):1701–2. doi: 10.1002/jmv.25992
95. Li W, Moore MJ, Vasilieva N, Sui J, Wong SK, Berne MA, et al. Angiotensin-converting enzyme 2 is a functional receptor for the SARS coronavirus. Nature (2003) 426(6965):450–4. doi: 10.1038/nature02145
96. Maglakelidze N, Manto KM, Craig TJ. A Review: Does Complement or the Contact System Have a Role in Protection or Pathogenesis of COVID-19? Pulm Ther (2020) 6(2):169–76. doi: 10.1007/s41030-020-00118-5
97. Milewska A, Falkowski K, Kalinska M, Bielecka E, Naskalska A, Mak P, et al. Kallikrein 13: a new player in coronaviral infections. bioRxiv (2020). 2020.03.01.971499. doi: 10.1101/2020.03.01.971499
98. Mönkemüller K, Fry L, Rickes S. COVID-19, coronavirus, SARS-CoV-2 and the small bowel. Rev Esp Enferm Dig (2020) 112(5):383–8. doi: 10.17235/reed.2020.7137/2020
99. Assante G, Williams R, Youngson NA. Is the increased risk for MAFLD patients to develop severe COVID-19 linked to perturbation of the gut-liver axis? J Hepatol (2021) 74:469–90. doi: 10.1016/j.jhep.2020.05.051
100. Cardinale V, Capurso G, Ianiro G, Gasbarrini A, Arcidiacono PG, Alvaro D. Intestinal permeability changes with bacterial translocation as key events modulating systemic host immune response to SARS-CoV-2: A working hypothesis. Dig Liver Dis (2020) 52(12):1383–9. doi: 10.1016/j.dld.2020.09.009
101. Belančić A. Gut microbiome dysbiosis and endotoxemia - Additional pathophysiological explanation for increased COVID-19 severity in obesity. Obes Med (2020) 20:100302. doi: 10.1016/j.obmed.2020.100302
102. Zuo T, Zhang F, Lui GCY, Yeoh YK, Li AYL, Zhan H, et al. Alterations in Gut Microbiota of Patients With COVID-19 During Time of Hospitalization. Gastroenterology (2020) 159(3):944–55.e8. doi: 10.1053/j.gastro.2020.05.048
103. Gu S, Chen Y, Wu Z, Gao H, Lv L, Guo F, et al. Alterations of the Gut Microbiota in Patients with COVID-19 or H1N1 Influenza. Clin Infect Dis (2020). doi: 10.1093/cid/ciaa709
104. Zuo T, Liu Q, Zhang F, Lui GC, Tso EY, Yeoh YK, et al. Depicting SARS-CoV-2 faecal viral activity in association with gut microbiota composition in patients with COVID-19. Gut (2020) 70:276–84. doi: 10.1136/gutjnl-2020-322294
105. Zuo T, Zhan H, Zhang F, Liu Q, Tso EYK, Lui GCY, et al. Alterations in Fecal Fungal Microbiome of Patients With COVID-19 During Time of Hospitalization until Discharge. Gastroenterology (2020) 159(4):1302–10.e5. doi: 10.1053/j.gastro.2020.06.048
106. Hoel H, Heggelund L, Reikvam DH, Stiksrud B, Ueland T, Michelsen AE, et al. Elevated markers of gut leakage and inflammasome activation in COVID-19 patients with cardiac involvement. J Intern Med (2020) 5. doi: 10.1111/joim.13178
107. Moreno-Eutimio MA, López-Macías C, Pastelin-Palacios R. Bioinformatic analysis and identification of single-stranded RNA sequences recognized by TLR7/8 in the SARS-CoV-2, SARS-CoV, and MERS-CoV genomes. Microbes Infect (2020) 22(4-5):226–9. doi: 10.1016/j.micinf.2020.04.009
108. van der Made CI, Simons A, Schuurs-Hoeijmakers J, van den Heuvel G, Mantere T, Kersten S, et al. Presence of Genetic Variants Among Young Men With Severe COVID-19. JAMA (2020) 324(7):663–73. doi: 10.1001/jama.2020.13719
109. Englmeier L. A theory on SARS-COV-2 susceptibility: reduced TLR7-activity as a mechanistic link between men, obese and elderly. J Biol Regul Homeost Agents (2020) 34(3):1125–9. doi: 10.23812/20-221-L-49
110. Wang Y, Liang H, Jin F, Yan X, Xu G, Hu H, et al. Injured liver-released miRNA-122 elicits acute pulmonary inflammation via activating alveolar macrophage TLR7 signaling pathway. Proc Natl Acad Sci U S A (2019) 116(13):6162–71. doi: 10.1073/pnas.1814139116
111. Totura AL, Whitmore A, Agnihothram S, Schäfer A, Katze MG, Heise MT, et al. Toll-Like Receptor 3 Signaling via TRIF Contributes to a Protective Innate Immune Response to Severe Acute Respiratory Syndrome Coronavirus Infection. mBio (2015) 6(3):e00638–15. doi: 10.1128/mBio.00638-15
112. Sohn KM, Lee SG, Kim HJ, Cheon S, Jeong H, Lee J, et al. COVID-19 Patients Upregulate Toll-like Receptor 4-mediated Inflammatory Signaling That Mimics Bacterial Sepsis. J Korean Med Sci (2020) 35(38):e343. doi: 10.3346/jkms.2020.35.e343
113. Carpino G, Del Ben M, Pastori D, Carnevale R, Baratta F, Overi D, et al. Increased Liver Localization of Lipopolysaccharides in Human and Experimental NAFLD. Hepatology (2020) 72(2):470–85. doi: 10.1002/hep.31056
114. Shi CS, Nabar NR, Huang NN, Kehrl JH. SARS-Coronavirus Open Reading Frame-8b triggers intracellular stress pathways and activates NLRP3 inflammasomes. Cell Death Discov (2019) 5:101. doi: 10.1038/s41420-019-0181-7
115. Nieto-Torres JL, Verdiá-Báguena C, Jimenez-Guardeño JM, Regla-Nava JA, Castaño-Rodriguez C, Fernandez-Delgado R, et al. Severe acute respiratory syndrome coronavirus E protein transports calcium ions and activates the NLRP3 inflammasome. Virology (2015) 485:330–9. doi: 10.1016/j.virol.2015.08.010
116. Siu KL, Yuen KS, Castaño-Rodriguez C, Ye ZW, Yeung ML, Fung SY, et al. Severe acute respiratory syndrome coronavirus ORF3a protein activates the NLRP3 inflammasome by promoting TRAF3-dependent ubiquitination of ASC. FASEB J (2019) 33(8):8865–77. doi: 10.1096/fj.201802418R
117. Rodrigues TS, de Sá KSG, Ishimoto AY, Becerra A, Oliveira S, Almeida L, et al. Inflammasomes are activated in response to SARS-CoV-2 infection and are associated with COVID-19 severity in patients. J Exp Med (2021) 218(3):e20201707. doi: 10.1084/jem.20201707
118. Paces J, Strizova Z, Smrz D, Cerny J. COVID-19 and the immune system. Physiol Res (2020) 69(3):379–88. doi: 10.33549/physiolres.934492
119. McGonagle D, O’Donnell JS, Sharif K, Emery P, Bridgewood C. Immune mechanisms of pulmonary intravascular coagulopathy in COVID-19 pneumonia. Lancet Rheumatol (2020) 2(7):e437–e45. doi: 10.1016/S2665-9913(20)30121-1
120. Merad M, Martin JC. Pathological inflammation in patients with COVID-19: a key role for monocytes and macrophages. Nat Rev Immunol (2020) 20(6):355–62. doi: 10.1038/s41577-020-0331-4
121. Yao X, Ye F, Zhang M, Cui C, Huang B, Niu P, et al. In Vitro Antiviral Activity and Projection of Optimized Dosing Design of Hydroxychloroquine for the Treatment of Severe Acute Respiratory Syndrome Coronavirus 2 (SARS-CoV-2). Clin Infect Dis (2020) 71(15):732–9. doi: 10.1093/cid/ciaa237
122. Zhou Z, Ren L, Zhang L, Zhong J, Xiao Y, Jia Z, et al. Heightened Innate Immune Responses in the Respiratory Tract of COVID-19 Patients. Cell Host Microbe (2020) 27(6):883–90.e2. doi: 10.1016/j.chom.2020.04.017
123. Jaillon S, Berthenet K, Garlanda C. Sexual Dimorphism in Innate Immunity. Clin Rev Allergy Immunol (2019) 56(3):308–21. doi: 10.1007/s12016-017-8648-x
124. Della Torre S. Non-alcoholic Fatty Liver Disease as a Canonical Example of Metabolic Inflammatory-Based Liver Disease Showing a Sex-Specific Prevalence: Relevance of Estrogen Signaling. Front Endocrinol (Lausanne) (2020) 11:572490. doi: 10.3389/fendo.2020.572490
125. Price CC, Altice FL, Shyr Y, Koff A, Pischel L, Goshua G, et al. Tocilizumab Treatment for Cytokine Release Syndrome in Hospitalized Patients With Coronavirus Disease 2019: Survival and Clinical Outcomes. Chest (2020) 158(4):1397–408. doi: 10.1016/j.chest.2020.06.006
126. Morrison AR, Johnson JM, Griebe KM, Jones MC, Stine JJ, Hencken LN, et al. Clinical characteristics and predictors of survival in adults with coronavirus disease 2019 receiving tocilizumab. J Autoimmun (2020) 114:102512. doi: 10.1016/j.jaut.2020.102512
127. Perrone F, Piccirillo MC, Ascierto PA, Salvarani C, Parrella R, Marata AM, et al. Tocilizumab for patients with COVID-19 pneumonia. The single-arm TOCIVID-19 prospective trial. J Transl Med (2020) 18(1):405. doi: 10.1186/s12967-020-02573-9
128. Ramiro S, Mostard RLM, Magro-Checa C, van Dongen CMP, Dormans T, Buijs J, et al. Historically controlled comparison of glucocorticoids with or without tocilizumab versus supportive care only in patients with COVID-19-associated cytokine storm syndrome: results of the CHIC study. Ann Rheum Dis (2020) 79(9):1143–51. doi: 10.1136/annrheumdis-2020-219534
129. Novartis. Novartis provides update on CAN-COVID trial in hospitalized patients with COVID-19 pneumonia and cytokine release syndrome (CRS) Basel: Novartis. (2020). Available at: https://www.novartis.com/news/media-releases/novartis-provides-update-can-covid-trial-hospitalized-patients-covid-19-pneumonia-and-cytokine-release-syndrome-crs, [Press release].
130. Pei L, Zhang S, Huang L, Geng X, Ma L, Jiang W, et al. Antiviral agents, glucocorticoids, antibiotics, and intravenous immunoglobulin in 1142 patients with coronavirus disease 2019: a systematic review and meta-analysis. Pol Arch Intern Med (2020) 130(9):726–33. doi: 10.20452/pamw.15543
131. Tomazini BM, Maia IS, Cavalcanti AB, Berwanger O, Rosa RG, Veiga VC, et al. Effect of Dexamethasone on Days Alive and Ventilator-Free in Patients With Moderate or Severe Acute Respiratory Distress Syndrome and COVID-19: The CoDEX Randomized Clinical Trial. JAMA (2020) 324(13):1307–16. doi: 10.1001/jama.2020.17021
132. Sterne JAC, Murthy S, Diaz JV, Slutsky AS, Villar J, Angus DC, et al. Association Between Administration of Systemic Corticosteroids and Mortality Among Critically Ill Patients With COVID-19: A Meta-analysis. JAMA (2020) 324(13):1330–41. doi: 10.1001/jama.2020.17023
133. Yu C, Kang L, Chen J, Zang N. Evaluation of safety, efficacy, tolerability, and treatment-related outcomes of type I interferons for human coronaviruses (HCoVs) infection in clinical practice: An updated critical systematic review and meta-analysis. Int Immunopharmacol (2020) 86:106740. doi: 10.1016/j.intimp.2020.106740
134. Fisher CD, Lickteig AJ, Augustine LM, Ranger-Moore J, Jackson JP, Ferguson SS, et al. Hepatic cytochrome P450 enzyme alterations in humans with progressive stages of nonalcoholic fatty liver disease. Drug Metab Dispos (2009) 37(10):2087–94. doi: 10.1124/dmd.109.027466
135. Naik A, Belič A, Zanger UM, Rozman D. Molecular Interactions between NAFLD and Xenobiotic Metabolism. Front Genet (2013) 4:2. doi: 10.3389/fgene.2013.00002
136. Papac-Milicevic N, Busch CJ, Binder CJ. Malondialdehyde Epitopes as Targets of Immunity and the Implications for Atherosclerosis. Adv Immunol (2016) 131:1–59. doi: 10.1016/bs.ai.2016.02.001
137. Sbierski-Kind J, Kath J, Brachs S, Streitz M, von Herrath MG, Kühl AA, et al. Distinct Housing Conditions Reveal a Major Impact of Adaptive Immunity on the Course of Obesity-Induced Type 2 Diabetes. Front Immunol (2018) 9:1069. doi: 10.3389/fimmu.2018.01069
138. Miyake T, Akbar SM, Yoshida O, Chen S, Hiasa Y, Matsuura B, et al. Impaired dendritic cell functions disrupt antigen-specific adaptive immune responses in mice with nonalcoholic fatty liver disease. J Gastroenterol (2010) 45(8):859–67. doi: 10.1007/s00535-010-0218-4
139. Basho K, Zoldan K, Schultheiss M, Bettinger D, Globig AM, Bengsch B, et al. IL-2 contributes to cirrhosis-associated immune dysfunction by impairing follicular T helper cells in advanced cirrhosis. J Hepatol (2021) 74(3):649–60. doi: 10.1016/j.jhep.2020.10.012
140. Albano E, Mottaran E, Vidali M, Reale E, Saksena S, Occhino G, et al. Immune response towards lipid peroxidation products as a predictor of progression of non-alcoholic fatty liver disease to advanced fibrosis. Gut (2005) 54(7):987–93. doi: 10.1136/gut.2004.057968
141. Nobili V, Parola M, Alisi A, Marra F, Piemonte F, Mombello C, et al. Oxidative stress parameters in paediatric non-alcoholic fatty liver disease. Int J Mol Med (2010) 26(4):471–6. doi: 10.3892/ijmm_00000487
142. Bruzzì S, Sutti S, Giudici G, Burlone ME, Ramavath NN, Toscani A, et al. B2-Lymphocyte responses to oxidative stress-derived antigens contribute to the evolution of nonalcoholic fatty liver disease (NAFLD). Free Radic Biol Med (2018) 124:249–59. doi: 10.1016/j.freeradbiomed.2018.06.015
143. Sutti S, Jindal A, Locatelli I, Vacchiano M, Gigliotti L, Bozzola C, et al. Adaptive immune responses triggered by oxidative stress contribute to hepatic inflammation in NASH. Hepatology (2014) 59(3):886–97. doi: 10.1002/hep.26749
144. Hendrikx T, Watzenböck ML, Walenbergh SM, Amir S, Gruber S, Kozma MO, et al. Low levels of IgM antibodies recognizing oxidation-specific epitopes are associated with human non-alcoholic fatty liver disease. BMC Med (2016) 14(1):107. doi: 10.1186/s12916-016-0652-0
145. Karrar A, Stepanova M, Alaparthi L, Lingam S, Younoszai Z, Zheng L, et al. Anti-adipocyte antibody response in patients with non-alcoholic fatty liver disease. J Gastroenterol Hepatol (2015) 30(5):900–8. doi: 10.1111/jgh.12856
146. Loria P, Lonardo A, Leonardi F, Fontana C, Carulli L, Verrone AM, et al. Non-organ-specific autoantibodies in nonalcoholic fatty liver disease: prevalence and correlates. Dig Dis Sci (2003) 48(11):2173–81. doi: 10.1023/B:DDAS.0000004522.36120.08
147. Adams LA, Lindor KD, Angulo P. The prevalence of autoantibodies and autoimmune hepatitis in patients with nonalcoholic Fatty liver disease. Am J Gastroenterol (2004) 99(7):1316–20. doi: 10.1111/j.1572-0241.2004.30444.x
148. Vuppalanchi R, Gould RJ, Wilson LA, Unalp-Arida A, Cummings OW, Chalasani N, et al. Clinical significance of serum autoantibodies in patients with NAFLD: results from the nonalcoholic steatohepatitis clinical research network. Hepatol Int (2012) 6(1):379–85. doi: 10.1007/s12072-011-9277-8
149. Cotler SJ, Kanji K, Keshavarzian A, Jensen DM, Jakate S. Prevalence and significance of autoantibodies in patients with non-alcoholic steatohepatitis. J Clin Gastroenterol (2004) 38(9):801–4. doi: 10.1097/01.mcg.0000139072.38580.a0
150. Niwa H, Sasaki M, Haratake J, Kasai T, Katayanagi K, Kurumaya H, et al. Clinicopathological significance of antinuclear antibodies in non-alcoholic steatohepatitis. Hepatol Res (2007) 37(11):923–31. doi: 10.1111/j.1872-034X.2007.00150.x
151. Diedrich T, Kummer S, Galante A, Drolz A, Schlicker V, Lohse AW, et al. Characterization of the immune cell landscape of patients with NAFLD. PloS One (2020) 15(3):e0230307. doi: 10.1371/journal.pone.0230307
152. Sutti S, Albano E. Adaptive immunity: an emerging player in the progression of NAFLD. Nat Rev Gastroenterol Hepatol (2020) 17(2):81–92. doi: 10.1038/s41575-019-0210-2
153. Ni Y, Nagashimada M, Zhuge F, Zhan L, Nagata N, Tsutsui A, et al. Astaxanthin prevents and reverses diet-induced insulin resistance and steatohepatitis in mice: A comparison with vitamin E. Sci Rep (2015) 5:17192. doi: 10.1038/srep17192
154. Inzaugarat ME, De Matteo E, Baz P, Lucero D, García CC, Gonzalez Ballerga E, et al. New evidence for the therapeutic potential of curcumin to treat nonalcoholic fatty liver disease in humans. PloS One (2017) 12(3):e0172900. doi: 10.1371/journal.pone.0172900
155. Alchera E, Rolla S, Imarisio C, Bardina V, Valente G, Novelli F, et al. Adenosine A2a receptor stimulation blocks development of nonalcoholic steatohepatitis in mice by multilevel inhibition of signals that cause immunolipotoxicity. Transl Res (2017) 182:75–87. doi: 10.1016/j.trsl.2016.11.009
156. Wolf MJ, Adili A, Piotrowitz K, Abdullah Z, Boege Y, Stemmer K, et al. Metabolic activation of intrahepatic CD8+ T cells and NKT cells causes nonalcoholic steatohepatitis and liver cancer via cross-talk with hepatocytes. Cancer Cell (2014) 26(4):549–64. doi: 10.1016/j.ccell.2014.09.003
157. Nishida T, Tsuneyama K, Fujimoto M, Nomoto K, Hayashi S, Miwa S, et al. Spontaneous onset of nonalcoholic steatohepatitis and hepatocellular carcinoma in a mouse model of metabolic syndrome. Lab Invest (2013) 93(2):230–41. doi: 10.1038/labinvest.2012.155
158. Miyake T, Abe M, Tokumoto Y, Hirooka M, Furukawa S, Kumagi T, et al. B cell-activating factor is associated with the histological severity of nonalcoholic fatty liver disease. Hepatol Int (2013) 7(2):539–47. doi: 10.1007/s12072-012-9345-8
159. Wu Z, Xu J, Tan J, Song Y, Liu L, Zhang F, et al. Mesenteric adipose tissue B lymphocytes promote local and hepatic inflammation in non-alcoholic fatty liver disease mice. J Cell Mol Med (2019) 23(5):3375–85. doi: 10.1111/jcmm.14232
160. Inzaugarat ME, Ferreyra Solari NE, Billordo LA, Abecasis R, Gadano AC, Cherñavsky AC. Altered phenotype and functionality of circulating immune cells characterize adult patients with nonalcoholic steatohepatitis. J Clin Immunol (2011) 31(6):1120–30. doi: 10.1007/s10875-011-9571-1
161. Ferreyra Solari NE, Inzaugarat ME, Baz P, De Matteo E, Lezama C, Galoppo M, et al. The role of innate cells is coupled to a Th1-polarized immune response in pediatric nonalcoholic steatohepatitis. J Clin Immunol (2012) 32(3):611–21. doi: 10.1007/s10875-011-9635-2
162. Rau M, Schilling AK, Meertens J, Hering I, Weiss J, Jurowich C, et al. Progression from Nonalcoholic Fatty Liver to Nonalcoholic Steatohepatitis Is Marked by a Higher Frequency of Th17 Cells in the Liver and an Increased Th17/Resting Regulatory T Cell Ratio in Peripheral Blood and in the Liver. J Immunol (2016) 196(1):97–105. doi: 10.4049/jimmunol.1501175
163. Bertola A, Bonnafous S, Anty R, Patouraux S, Saint-Paul MC, Iannelli A, et al. Hepatic expression patterns of inflammatory and immune response genes associated with obesity and NASH in morbidly obese patients. PloS One (2010) 5(10):e13577. doi: 10.1371/journal.pone.0013577
164. Li Z, Soloski MJ, Diehl AM. Dietary factors alter hepatic innate immune system in mice with nonalcoholic fatty liver disease. Hepatology (2005) 42(4):880–5. doi: 10.1002/hep.20826
165. Söderberg C, Marmur J, Eckes K, Glaumann H, Sällberg M, Frelin L, et al. Microvesicular fat, inter cellular adhesion molecule-1 and regulatory T-lymphocytes are of importance for the inflammatory process in livers with non-alcoholic steatohepatitis. APMIS (2011) 119(7):412–20. doi: 10.1111/j.1600-0463.2011.02746.x
166. Lalazar G, Mizrahi M, Turgeman I, Adar T, Ben Ya’acov A, Shabat Y, et al. Oral Administration of OKT3 MAb to Patients with NASH, Promotes Regulatory T-cell Induction, and Alleviates Insulin Resistance: Results of a Phase IIa Blinded Placebo-Controlled Trial. J Clin Immunol (2015) 35(4):399–407. doi: 10.1007/s10875-015-0160-6
167. Świderska M, Jaroszewicz J, Stawicka A, Parfieniuk-Kowerda A, Chabowski A, Flisiak R. The interplay between Th17 and T-regulatory responses as well as adipokines in the progression of non-alcoholic fatty liver disease. Clin Exp Hepatol (2017) 3(3):127–34. doi: 10.5114/ceh.2017.68466
168. Su L, Wu Z, Chi Y, Song Y, Xu J, Tan J, et al. Mesenteric lymph node CD4. Cell Immunol (2019) 337:33–41. doi: 10.1016/j.cellimm.2019.01.005
169. He B, Wu L, Xie W, Shao Y, Jiang J, Zhao Z, et al. The imbalance of Th17/Treg cells is involved in the progression of nonalcoholic fatty liver disease in mice. BMC Immunol (2017) 18(1):33. doi: 10.1186/s12865-017-0215-y
170. Hu Y, Zhang H, Li J, Cong X, Chen Y, He G, et al. Gut-derived lymphocyte recruitment to liver and induce liver injury in non-alcoholic fatty liver disease mouse model. J Gastroenterol Hepatol (2016) 31(3):676–84. doi: 10.1111/jgh.13183
171. Ma C, Kesarwala AH, Eggert T, Medina-Echeverz J, Kleiner DE, Jin P, et al. NAFLD causes selective CD4(+) T lymphocyte loss and promotes hepatocarcinogenesis. Nature (2016) 531(7593):253–7. doi: 10.1038/nature16969
172. Brown ZJ, Fu Q, Ma C, Kruhlak M, Zhang H, Luo J, et al. Carnitine palmitoyltransferase gene upregulation by linoleic acid induces CD4. Cell Death Dis (2018) 9(6):620. doi: 10.1038/s41419-018-0687-6
173. Coia H, Ma N, Hou Y, Permaul E, Berry DL, Cruz MI, et al. Theaphenon E prevents fatty liver disease and increases CD4+ T cell survival in mice fed a high-fat diet. Clin Nutr (2021) 40(1):110–9. doi: 10.1016/j.clnu.2020.04.033
174. Stiglund N, Strand K, Cornillet M, Stål P, Thorell A, Zimmer CL, et al. Retained NK Cell Phenotype and Functionality in Non-alcoholic Fatty Liver Disease. Front Immunol (2019) 10:1255. doi: 10.3389/fimmu.2019.01255
175. Adler M, Taylor S, Okebugwu K, Yee H, Fielding C, Fielding G, et al. Intrahepatic natural killer T cell populations are increased in human hepatic steatosis. World J Gastroenterol (2011) 17(13):1725–31. doi: 10.3748/wjg.v17.i13.1725
176. Vonghia L, Magrone T, Verrijken A, Michielsen P, Van Gaal L, Jirillo E, et al. Peripheral and Hepatic Vein Cytokine Levels in Correlation with Non-Alcoholic Fatty Liver Disease (NAFLD)-Related Metabolic, Histological, and Haemodynamic Features. PloS One (2015) 10(11):e0143380. doi: 10.1371/journal.pone.0143380
177. Tan L, Kang X, Ji X, Li G, Wang Q, Li Y, et al. Validation of Predictors of Disease Severity and Outcomes in COVID-19 Patients: A Descriptive and Retrospective Study. Med (N Y) (2020) 1(1):128–38.e3. doi: 10.1016/j.medj.2020.05.002
178. Zheng M, Gao Y, Wang G, Song G, Liu S, Sun D, et al. Functional exhaustion of antiviral lymphocytes in COVID-19 patients. Cell Mol Immunol (2020) 17(5):533–5. doi: 10.1038/s41423-020-0402-2
179. Li J, Guo M, Tian X, Wang X, Yang X, Wu P, et al. Virus-Host Interactome and Proteomic Survey Reveal Potential Virulence Factors Influencing SARS-CoV-2 Pathogenesis. Med (N Y) (2021) 2(1):99–112.e7. doi: 10.1016/j.medj.2020.07.002
180. Diao B, Wang C, Tan Y, Chen X, Liu Y, Ning L, et al. Reduction and Functional Exhaustion of T Cells in Patients With Coronavirus Disease 2019 (COVID-19). Front Immunol (2020) 11:827. doi: 10.3389/fimmu.2020.00827
181. Mathew D, Giles JR, Baxter AE, Oldridge DA, Greenplate AR, Wu JE, et al. Deep immune profiling of COVID-19 patients reveals distinct immunotypes with therapeutic implications. Science (2020) 369(6508):eabc8511. doi: 10.1126/science.abc8511
182. Wilk AJ, Rustagi A, Zhao NQ, Roque J, Martínez-Colón GJ, McKechnie JL, et al. A single-cell atlas of the peripheral immune response in patients with severe COVID-19. Nat Med (2020) 26(7):1070–6. doi: 10.1038/s41591-020-0944-y
183. Targher G, Mantovani A, Byrne CD, Wang XB, Yan HD, Sun QF, et al. Detrimental effects of metabolic dysfunction-associated fatty liver disease and increased neutrophil-to-lymphocyte ratio on severity of COVID-19. Diabetes Metab (2020) 46(6):505–7. doi: 10.1016/j.diabet.2020.06.001
184. Prins GH, Olinga P. Potential implications of COVID-19 in non-alcoholic fatty liver disease. Liver Int (2020) 40(10):2568. doi: 10.1111/liv.14484
185. Su Y, Chen D, Yuan D, Lausted C, Choi J, Dai CL, et al. Multi-Omics Resolves a Sharp Disease-State Shift between Mild and Moderate COVID-19. Cell (2020) 183(6):1479–95.e20. doi: 10.1016/j.cell.2020.10.037
Keywords: MAFLD, NASH, COVID-19, SARS-CoV-2, innate immunity, adaptive immunity
Citation: Lamadrid P, Alonso-Peña M, San Segundo D, Arias-Loste M, Crespo J and Lopez-Hoyos M (2021) Innate and Adaptive Immunity Alterations in Metabolic Associated Fatty Liver Disease and Its Implication in COVID-19 Severity. Front. Immunol. 12:651728. doi: 10.3389/fimmu.2021.651728
Received: 10 January 2021; Accepted: 08 March 2021;
Published: 30 March 2021.
Edited by:
Hai Huang, The Ohio State University, United StatesReviewed by:
Tomas Meroño, Universitat de Barcelona, SpainKa Man Law, University of California, Los Angeles, United States
Copyright © 2021 Lamadrid, Alonso-Peña, San Segundo, Arias-Loste, Crespo and Lopez-Hoyos. This is an open-access article distributed under the terms of the Creative Commons Attribution License (CC BY). The use, distribution or reproduction in other forums is permitted, provided the original author(s) and the copyright owner(s) are credited and that the original publication in this journal is cited, in accordance with accepted academic practice. No use, distribution or reproduction is permitted which does not comply with these terms.
*Correspondence: Marcos Lopez-Hoyos, bWFyY29zLmxvcGV6QHNjc2FsdWQuZXM=
†These authors have contributed equally to this work