- 1Division of Pulmonary Medicine, Cincinnati Children's Hospital Medical Center, Cincinnati, OH, United States
- 2Department of Pediatrics, College of Medicine, University of Cincinnati, Cincinnati, OH, United States
- 3Division of Pulmonary, Critical Care and Sleep Medicine, University of Cincinnati, Cincinnati, OH, United States
- 4Division of Biomedical Informatics, Cincinnati Children's Hospital Medical Center, Cincinnati, OH, United States
Idiopathic Pulmonary Fibrosis (IPF) is a severe fibrotic lung disease characterized by excessive collagen deposition and progressive decline in lung function. Th2 T cell-derived cytokines including IL-4 and IL-13 have been shown to contribute to inflammation and fibrotic remodeling in multiple tissues. Interleukin-31 (IL-31) is a newly identified cytokine that is predominantly produced by CD4 Th2 T cells, but its signaling receptor IL-31RA is primarily expressed by non-hematopoietic cells. However, the potential role of the IL-31-IL31RA axis in pulmonary inflammation and fibrosis has remained largely unknown. To determine the role of IL-31RA deficiency in pulmonary fibrosis, wildtype, and IL-31RA knockout mice were treated with bleomycin and measured changes in collagen deposition and lung function. Notably, the loss of IL-31 signaling attenuated collagen deposition and lung function decline during bleomycin-induced pulmonary fibrosis. The total lung transcriptome analysis showed a significant reduction in fibrosis-associated gene transcripts including extracellular matrix and epithelial cell-associated gene networks. Furthermore, the lungs of human IPF showed an elevated expression of IL-31 when compared to healthy subjects. In support, the percentage of IL-31 producing CD4+ T cells was greater in the lungs and PBMCs from IPF patients compared to healthy controls. Our findings suggest a pathogenic role for IL-31/IL-31RA signaling during bleomycin-induced pulmonary fibrosis. Thus, therapeutic targeting the IL-31-IL-31RA axis may prevent collagen deposition, improve lung function, and have therapeutic potential in pulmonary fibrosis.
Introduction
Pulmonary fibrosis is a chronic heterogeneous lung disease characterized by an uncontrolled injury and repair process in the lung parenchyma. This process includes excessive deposition of collagen and extracellular matrix (ECM) protein components in the distal areas of the lung (1, 2). Patients with severe fibrotic lung diseases including idiopathic pulmonary fibrosis (IPF) develop irreversible decline of lung function characterized by a decrease in forced expiratory volume in one second (FEV1) and forced vital capacity (FVC) in part due to the progressive accumulation of ECM producing myofibroblasts. Currently, the United States Food and Drug Administration (FDA) approved therapies to treat IPF, Nintedanib or Pirfenidone, slow the rate of FVC decline but do not reverse ongoing fibrosis (3, 4). While the etiology of IPF is not well established, multiple pathways underlying the pathogenesis of pulmonary fibrosis have been identified (2, 5). The development of fibrotic lesions, specifically, is regulated by signaling pathways that are driven by multiple pro-fibrotic growth factors, including TGF-β, TGF-α, CTGF, and cytokines such as IL-1β, IL-17, IL-4, and IL-13 (6–8). Th2 cytokines IL-4 and IL-13 have been found to play a major role in the pathophysiology of fibrotic diseases and are considered potential therapeutic targets (8). IL-13 signaling can induce activation of fibroblasts and macrophages to produce ECM and constitutive factors such as proline, which are required for the biogenesis of collagen. IL-4 and IL-13 signal through a type II IL-4 receptor alpha (IL-4Rα); this receptor is expressed in multiple cell types, including macrophages, epithelial cells, and fibroblasts (9).
Interleukin-31 (IL-31) is a recently described cytokine in the gp130/IL-6 cytokine family and is mainly produced by type 2 helper T cells. It plays a major role in the pathogenesis of chronic inflammatory diseases including atopic dermatitis (AD) or eczema (10–12). IL-31 signaling is mediated through the heterodimeric receptor composed of the IL-31 receptor A (IL-31RA) and the Oncostatin M receptor β (OSMR β). IL-31RA is expressed by various cells including immune cells, epithelial cells, and fibroblasts; these cells can secrete pro-inflammatory cytokines and chemokines following stimulation by IL-31 (11, 13–16). The role of IL-31 in the development of inflammatory diseases including AD has been described and potential therapeutic agents have been generated to target IL-31/IL-31RA signaling (10, 11, 17, 18). Recent studies have shown elevated levels of IL-31 in patients with AD or asthma and these levels have been correlated with disease severity (19, 20). Importantly, a recent published study suggests a significant increase in IL-31 levels in plasma, fibrotic skin, and lung lesions of patients with scleroderma compared to healthy controls (21). Similar findings have been reported during bleomycin-induced pulmonary fibrosis and also virus-induced liver fibrosis (22, 23). In fibrotic diseases, several of IL-6 family cytokines including IL6 and OSM have been shown to induce pulmonary fibrosis, but the contribution of IL-31/IL-31RA signaling in lung fibrosis has remained unexplored (20, 24). Our previous work has depicted the effector mechanism of IL-4/IL-13 signaling in the pathogenesis of pulmonary fibrosis and the upregulation of IL-31RA via the IL-4Rα/STAT6 signaling axis (8, 25). However, the role of IL-31/IL-31RA signaling in the pathogenesis of pulmonary fibrosis has not been definitively determined.
The current study aimed to determine the role of IL-31/IL-31RA signaling in the pathogenesis of pulmonary fibrosis using a bleomycin-induced lung fibrosis model and IL-31RA deficient mice. We observed a significant decrease in collagen deposition and the expression of profibrotic genes in the lungs of IL-31RA deficient mice compared to wildtype mice treated with bleomycin. Notably, the loss of IL-31RA signaling was sufficient to attenuate worsening lung function. Further, we observed increased expression of IL-31 in the lung tissue and CD4-positive T cells of IPF patients compared to healthy subjects.
Materials and Methods
Mice
IL31RA knockout (IL-31RA−/−) mice and their littermate wildtype mice of C57BL/6 background were used in this study to investigate the role of IL-31RA signaling in the development of pulmonary fibrosis. IL-31 RA KO mice used in this study have been previously described by Dillon and colleagues (11). In brief, the mouse IL31RA gene contains 14 exons covering roughly 70Kb of sequence. The construct designed to knockout the IL31RA gene via homologous recombination deletes exons 4, 5, and 6 and the intervening introns (3400bp). The deletion of exons 4-6 of IL-31RA deletes the cytokine-binding domain 2 region and IL-31RA knockout mice are identified by PCR genotyping. The published studies have shown that these mice display impaired IL-31 signaling and pathology in vivo (11, 25, 26). Both male and female mice at 10–18 weeks of age were used for all of the experiments and were housed in the Cincinnati Children's Hospital Medical Center animal facility under conditions approved by the American Association for the Accreditation of Laboratory Animal Care. All mice were maintained under aseptic conditions and received sterile food and water. The experiments were approved by the Institutional Animal Care and Use Committee.
Bleomycin-Induced Pulmonary Fibrosis Model
IL-31RA knockout mice and littermate wildtype mice were intradermally injected with bleomycin to induce lung fibrosis as previously described (8, 27). Briefly, mice were injected with saline or bleomycin (6 U/kg of body weight) in 50 μl saline, once a day and during 5 consecutive days per week, for a total of 4 weeks, to induce pulmonary fibrosis. Mice were euthanized on day 28 and lung samples were collected to assess fibrosis using biochemical and molecular methods.
Histology and Lung Hydroxyproline
The lungs were fixed with 10% neutral formalin and paraffin embedded. The five-micron thick lung sections were prepared and stained with Masson's Trichome to evaluate the deposition of collagen in lung tissues, a key feature of the fibrotic process. The deposition of collagen was assessed by measuring hydroxyproline levels in lung lysates using a colorimetric detection assay as previously described (28). In brief, the right lung lobes were collected, weighed, and hydrolyzed in 4 ml of 6 N HCl overnight at 110°C. Hydrolyzed samples were neutralized with 1 N NaOH. For colorization, chloramine-T (0.05 M) and the aldehyde-perchloric acid reagent were added and samples were placed in a hot water bath at 60°C for 25 min. The concentration of hydroxyproline in the samples was determined using the standard curve. The hydroxyproline level was normalized to the lung weight and expressed in comparison to the level measured in the saline-treated wildtype control group (8).
Lung Function Measurements
Murine lung function was measured using a computerized FlexiVent system (Flexiware version 7.5, SCIREQ, Montreal Canada) as previously described (29–31). Briefly, mice were anesthetized with Ketamine/Xylazine and an incision was performed on the anterior area of the neck to expose the trachea. The trachea was then cannulated with a 20G cannula and the mouse was connected to the FlexiVent system. Following deep inflation, three consecutive measures were collected for each mouse and the average value was used to measure resistance, elastance, and compliance.
Immunohistochemistry
De-identified explanted lung tissue specimens collected from distal areas of lung were acquired using research protocol approved by Cincinnati Children's Hospital Medical Center institutional review board (IRB # 2017-6850). Formalin-fixed and paraffin-embedded human lung tissue sections of IPF (n = 6) and healthy (n = 5) controls were immunostained with antibodies against IL-31 as previously described (32, 33). Briefly, rabbit anti-human IL-31 monoclonal antibody (R&D Systems) was used as the primary antibody (1:25 dilution). Goat anti-rabbit Ig was conjugated with a peroxidase enzyme to form a brown precipitate in the presence of hydrogen peroxide and DAB. Nuclei were stained in blue using Hematoxylin counterstain as described in previous reports (27, 34). The staining of the lung section using control IgG showed no detectable immunostaining (data not shown). All images were obtained using a Leica DM2700 M bright-field microscope. High-magnification images (X40) were captured with a 3CCD color video camera. The number of IL-31-positive cells and total cells was counted using MetaMorph imaging software and expressed as the percentage of IL-31-positive cells in total cells in the field.
RNA Preparation and Real-Time PCR
Lungs were homogenized using Qiagen tissue homogenizer and beads (Qiagen), and total RNA was extracted from lung tissues using RNeasy mini kit (Qiagen Science, Valencia, CA) as previously described (10, 25). Lung RNA samples were reverse transcribed into cDNA using Superscript III (Invitrogen), and the relative transcript expression of select genes was measured using SYBR Green PCR Master Mix (Applied Biosystems, Foster City, CA) and the CFX384 Touch Real-Time PCR detection system (Bio-Rad, Hercules, CA) as previously described (35). Gene transcripts were normalized to the housekeeping gene transcripts such as hypoxanthine-guanine phosphoribosyltransferase (HPRT) or β actin and were expressed as the relative fold-induction change compared to the gene expression level of the control wildtype mice treated with saline solution. Data was analyzed with Bio-Rad CFX maestro software version 4.2(Bio-Rad Laboratories, Hercules, CA). The list of primers used for real-time PCR to analyze the relative expression of the genes is included in Supplementary Table 1.
Whole Lung Transcriptome Shotgun Sequencing (RNAseq)
Total lung RNA samples were obtained from IL-31RA−/− and wildtype mice treated with bleomycin as described above. Four samples were prepared from each experimental group and subjected to RNA sequencing using an Illumina HiSeq-1000 Sequencer (Illumina, San Diego, CA), as described previously (36). A comparative analysis between groups was realized to identify genes with differential changes in expression following treatment with bleomycin or saline. Genes with statistically significant changes were selected based on a p-value cut-off of 0.05 and differential expression (defined as a 1.5-fold increase or decrease in expression). Heatmaps of genes with differential expression change were generated to highlight clusters of genes up-or downregulated in IL-31RA−/− and wildtype mice treated with bleomycin (Supplementary Figure 1). Functional enrichment analyses were performed using the ToppFun application of the ToppGene Suite (37). The IL-31RA-dependent genes were also compared with differentially expressed genes (DEGs) from IPF patients (GSE53845) to identify common genes between IL-31RA regulated genes and IPF DEGs as described previously (38, 39).
In vitro Epithelial Cell Treatments
Human bronchial epithelial BEAS-2B cells were obtained from the American type culture collection (Manassas, VA) and cultured in DMEM medium supplemented with 10% fetal bovine serum (FBS) at 37 and 5% CO2. Primary normal Human bronchial epithelial cells (HBEC) were obtained from the CCHMC Cystic Fibrosis Model Systems Core and cultured as previously described (40, 41). The use of de-identified HBEC was approved by CCHMC institutional review board protocol (IRB # 2017-6850). HBECs were grown at an air-liquid interface (ALI) on trans well inserts for at least 21 days at 370C and 5% CO2. To assess the effects of IL-31, media was changed to low serum media (1%FBS) for 16 hrs. Under low serum conditions, cells were stimulated with media supplemented with increasing doses (0, 100, 250, or 500 ng/ml) of recombinant IL-31(R&D Systems) for 16 hours. Total RNA was extracted from the cultured cells using the Qiagen RNA shredder and the RNeasy mini kit (Qiagen). cDNA was prepared and applied for real-time RT-PCR to measure gene transcript levels as described above. Gene expression was normalized to the housekeeping gene human β-actin.
Flow Cytometry
Peripheral blood mononuclear cells (PBMC) were isolated from peripheral venous blood of IPF patients (n = 7) and healthy control donors (n = 8) using Ficoll-paque density gradient-based separation with centrifugation (GE Healthcare, Uppsala, Sweden). The collection and use of PBMCs from IPF patients and healthy controls was approved by University of Cincinnati and Cincinnati Children's Hospital Medical Center institutional review board approved protocols (IRB # CR08 2013-8157 and Supplementary Table 2). Red blood cells were lysed using an ACK lysis buffer and cell viability was confirmed with trypan blue. Isolated PBMCs were frozen with DMSO and stored in liquid nitrogen until used. To measure the production of IL-31 and IL-4, PBMCs were thawed from liquid nitrogen and plated in a 96-well round-bottom plate (1 x 106 cells/well) in RPMI 1640 medium supplemented with L-glutamine, antibiotics (penicillin/streptomycin), and 10% heat-inactivated fetal bovine serum (FBS). Cells then were allowed to rest overnight. PBMCs were stimulated with phorbol 12-myristate 13-acetate (PMA) 10 ng/ml with Ionomycin (1 μg/ml), and Golgi stop (1:1500) for 5 h to induce cytokine production. Control samples were cultured with PMA and ionomycin for the same period. Cells were then stained with Zombie UV(BioLegend) for 30 min at room temperature in the dark to allow for discrimination between living and dead cells. Non-specific binding of fluorochromes was prevented by incubating cells with Human BD Fc Block™ (BD Biosciences), followed by surface marker staining with anti-CD3-V500 (UCHT1) and anti-CD4-APC(RPA-T4) (Bio Legend). Cells were then fixed and permeabilized using Cytofix/Cytoperm (BD Biosciences) and stained with antibodies against IL-31- PE (U26-947) (BD Biosciences) and IL-4 -PE-Cy7 (MP4-25D2) (BioLegend). Data was acquired using LSR Fortessa flow cytometer and analyzed using FlowJo 10 (FlowJo, Tree Star). Flow cytometry gating strategy was used to exclude dead cells, doublets and analyzed for IL-31-producing T cells in total live cells (Supplementary Figure 3).
Statistical Analysis
All data were analyzed using GraphPad PRISM 8. One-way ANOVA with Turkey's multiple comparison test was used to compare different experimental groups. Student's t-test was used to compare between two experimental groups. Quantitative data were presented as mean ± SEM, and p < 0.05 was considered statistically significant.
Results
The Loss of IL-31RA Signaling Attenuates Collagen Deposition and Lung Function Decline
To determine whether the loss of IL-31RA has any effect on pulmonary fibrosis, IL-31RA knockout mice and their littermate wildtype mice were treated intradermally with bleomycin (to induce pulmonary fibrosis) or treated with saline as a non-fibrosis control. To assess collagen deposition, the lung sections were stained with Masson Trichrome. There were no significant differences in collagen staining between wildtype and IL-31RA knockout mice treated with saline. A significant increase in collagen deposition was observed in the lungs of both wildtype mice and IL-31RA knockout mice treated with bleomycin (Figure 1A). However, there was a modest decrease in collagen staining in mice deficient for IL-31RA compared to wildtype mice treated with bleomycin (Figure 1A). To further evaluate collagen deposition, the level of hydroxyproline was quantified in the lungs of IL-31RA knockout mice and wildtype mice treated with bleomycin or saline. Both wildtype and IL-31RA knockout mice treated with bleomycin had increased expression of lung hydroxyproline compared with saline-treated mice. However, there was a modest but significant reduction in lung hydroxyproline levels in IL-31RA knockout mice compared to wildtype mice treated with bleomycin (Figure 1B). Our published studies have demonstrated that repetitive bleomycin-induced fibrotic remodeling decreases lung function (42, 43). Therefore, we assessed whether the loss of IL-31RA signaling has any effect on the decline in lung function following repetitive bleomycin treatment. Consistent with previous findings, there was an increase in resistance, and elastance, and a significant decrease in compliance in wildtype mice treated with bleomycin compared to saline-treated wildtype or IL-31RA knockout mice (Figures 1C–E). However, the decline in lung function was attenuated with the loss of IL-31RA signaling compared to wildtype mice treated with bleomycin (Figures 1C–E). These findings suggest that the loss of IL-31RA has a partial protective effect against collagen deposition, and the subsequent decline in lung function, during bleomycin-induced pulmonary fibrosis.
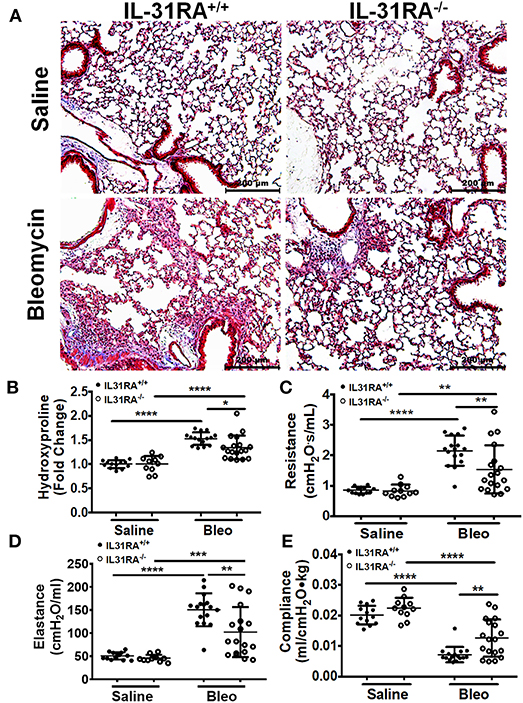
Figure 1. The loss of IL-31RA signaling attenuates collagen deposition and lung function decline during bleomycin-induced pulmonary fibrosis. Wildtype (IL-31RA+/+) and IL-31RA knockout (IL31RA−/−) mice were treated intradermally with bleomycin or saline for four weeks to induce pulmonary fibrosis. (A) Lung sections of saline or bleomycin-treated mice were stained with Masson Trichrome. Scale bar 200 μm. (B) Total lung hydroxyproline was compared between IL-31RA−/− and IL31RA+/+ mice treated with bleomycin or saline. (C–E) Lung function measurements including resistance, elastance and compliance were measured in all four groups using FlexiVent. Data are presented as means ± SEM. The above data is cumulative of two independent experiments (n = 12-19/group). Statistical analysis was performed using one-way ANOVA with Tukey's multiple comparisons test. *p < 0.05; **p < 0.01; ***p < 0.005 and ****p < 0.001.
The Role of IL-31RA Signaling in Fibrosis-Associated Gene Expression
To identify IL-31RA dependent gene expression and associated signaling pathways involved in bleomycin-induced pulmonary fibrosis, whole lung transcriptome analysis was performed using next-generation RNA-seq analysis in wildtype and IL-31RA knockout mice treated with bleomycin. The comparison of differentially expressed genes, with 1.5-fold up-or down-regulation in wildtype and IL31RA knockout lungs, highlighted two clusters of genes as illustrated in the heatmap (Figure 2). A total of 458 genes were differentially expressed in the lungs of IL-31RA knockout mice compared to wildtype mice treated with bleomycin. About 228 genes were up-regulated in IL-31RA knockout mice, and 230 genes were down-regulated, with the loss of IL-31RA signaling (Supplementary Table 3). Gene ontology enrichment analysis of down-regulated genes suggested that IL-31RA is involved in the expression of several ECM-associated genes (Col1a1, Col3a, Fn1, Arg1, Timp1, Mmp13, Ereg, and IL-6), Protein G coupled receptor Gα(i) signaling (Ccl28, Rgs5, Rgs4, and Rgs16), Epithelium-associated genes (Krt4, Krt5, Krt14, Krt20), Adenylate cyclase activity (Calcb, Calcr), and chemotaxis (Ccl28, Mcp1, IL6). Enrichment analysis of genes that were upregulated with the loss of IL-31RA suggested an increase in the expression of genes associated with the G protein-coupled receptor, and synaptic and calcium signaling.
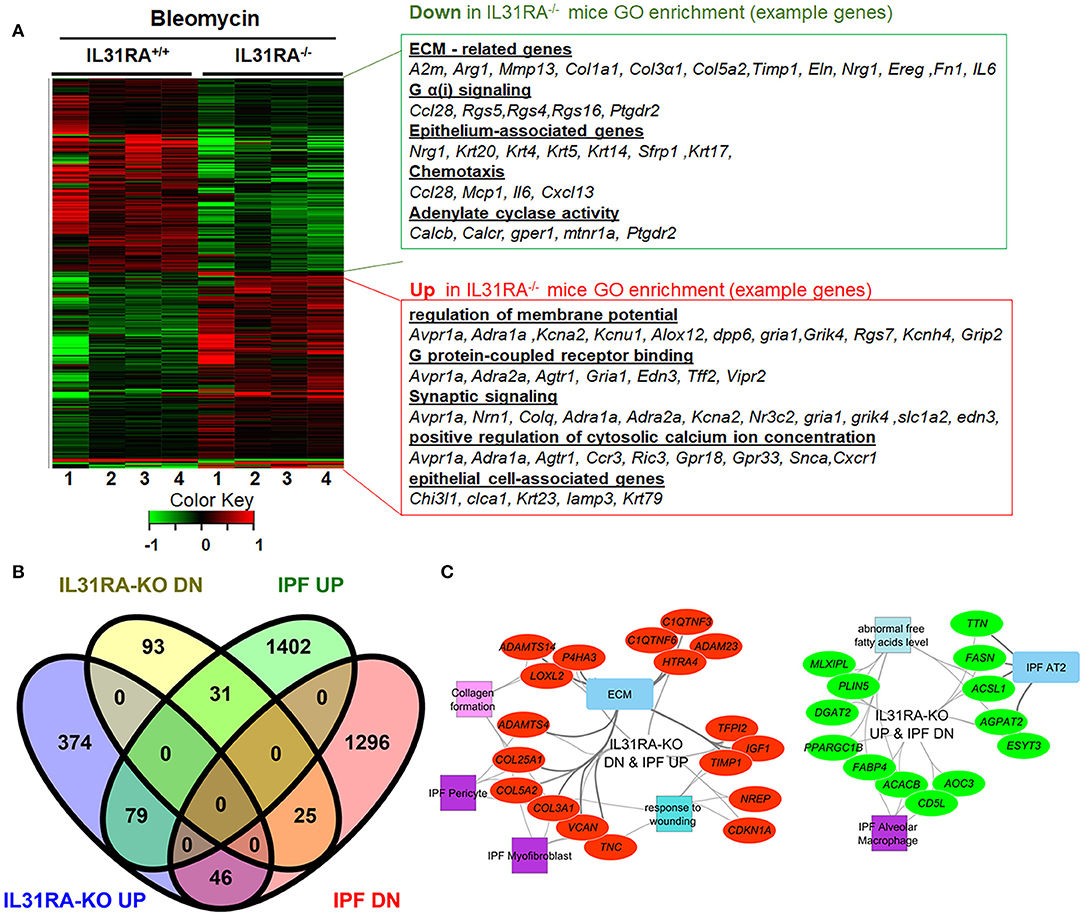
Figure 2. The IL-31-IL-31RA axis regulated gene networks during bleomycin-induced pulmonary fibrosis and IPF. Total lung RNA was isolated from bleomycin or saline-treated mice and performed RNA-seq analysis. (A) The heat map shows two clusters of differentially expressed genes that were up-or down-regulated by at least 1.5-fold upon bleomycin treatment of genetic knockdown of IL-31RA compared to wildtype mice. Gene Ontology Enrichment analysis for IL-31RA-dependent gene transcripts was performed using ToppFun. (B) Venn diagram of IL31RA-regulated genes compared with differentially expressed lung transcripts of IPF patients. (C) IL-31RA-driven gene networks that are activated in IPF were analyzed using ToppFun and visualized using Cytoscape. Red- and green-colored oval shapes represent genes that are IL31RA-regulated and up-or down-regulated, respectively in IPF lungs. Both square and rectangle shapes represent different enriched biological processes and cell types for the inversely correlated genes between the IL-31-IL-31RA axis and IPF.
Next, to identify the IL-31RA-dependent genes that are also dysregulated and relevant to IPF pathogenesis, differentially expressed genes in IPF lungs were obtained and compared to IL-31RA-dependent genes (Figure 2B). In total, 181 IPF differentially expressed genes were identified that were also dysregulated with the loss of IL-31RA (Figure 2B). This IL-31RA dependent gene list includes 31 genes that were up-regulated, and 46 down-regulated genes, in IPF lungs (Figure 2B and Supplementary Figure 1). We performed an enrichment analysis of these negatively correlated genes using the ToppFun application and visualized the gene networks using Cytoscape. As shown in Figure 2C, our gene functional enrichment analysis further supports alterations in fibroblast- and epithelial cell-associated genes and also a decrease in multiple collagen gene transcripts.
The Loss of IL-31RA Signaling Attenuates the Expression of Pro-fibrotic Genes
To validate the findings using RNA-seq, fibrosis-associated gene transcripts were quantified in the total lung RNA of IL-31RA knockout and wildtype mice treated with bleomycin or saline. The major ECM gene transcripts including Col1α, Col3α, and Fn1 were upregulated in lungs of wildtype mice treated with bleomycin compared to wildtype mice treated with saline. This increase in ECM gene expression was significantly attenuated in IL-31RA knockout mice compared to wildtype mice treated with bleomycin (Figure 3A). Similarly, genes associated with ECM production and remodeling were significantly decreased with the loss of IL-31RA; these genes included MMP13 and TIMP1, as well as IL-6 which increased in wildtype mice during bleomycin-induced pulmonary fibrosis (Figure 3B). The total lung RNA-seq analysis also suggests altered expression of several epithelial cell-associated genes with the loss of IL-31RA during bleomycin-induced pulmonary fibrosis (Figure 2). To validate the differential expression of genes associated with epithelial cells, we measured transcript levels of several epithelium-associated genes including Krt5, and Krt14. As shown in Figure 4A, we observed a significant increase in the transcripts of Krt5, and Krt14 in wildtype mice treated with bleomycin compared to saline-treated wildtype mice. This increase in epithelial gene transcripts was attenuated with the loss of IL-31RA compared to wildtype mice treated with bleomycin (Figure 4A). These findings suggest a critical role for airway epithelium in the pathophysiology of IL-31RA-driven pulmonary fibrosis and decline in lung function. Therefore, we studied the effect of IL-31 on airway epithelial cells in the production of pro-fibrotic cytokines associated with pulmonary fibrosis. Bronchial epithelial cell line BEAS-2B, or primary bronchial epithelial cells, were stimulated with IL-31 cytokine and the transcript levels of Mcp1 and IL-6 were determined using RTPCR. The production of pro-fibrotic cytokines, including Mcp1 and IL-6, increased with IL-31 stimulation in both BEAS-2B and HBEC cells (Figures 4B,C). In addition, we observed a significant increase in the expression of IL-31RA with IL-31 stimulation in both BEAS-2B and HBEC cells (Supplementary Figure 3). We quantified the levels of IL-6 and MCP1 in lung lysates of wildtype and IL-31RA knockout mice treated with bleomycin using ELISA and observed a significant decrease in both IL-6 and MCP1 in IL31RA knockout mice compared to wildtype mice treated with bleomycin (Supplementary Figure 4). These findings suggest that IL-31-driven signaling increases the production of pro-fibrotic cytokines by epithelial cells in the pathogenesis of pulmonary fibrosis.
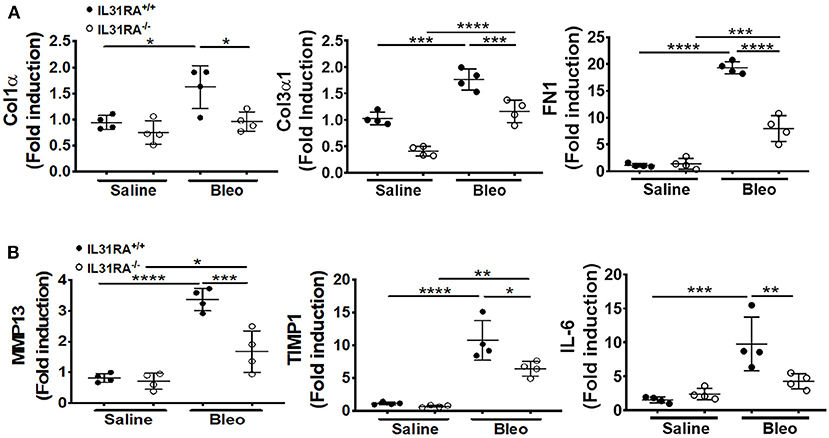
Figure 3. The loss of IL-31RA signaling attenuates the expression of fibrosis-associated genes during bleomycin-induced pulmonary fibrosis. Total lung RNA was isolated from wildtype and IL-31RA−/− mice treated with saline and bleomycin and fibrosis-associated genes quantified using real-time RTPCR. (A) Quantification of ECM gene transcripts including Col1α, Col3α1, and Fn1. (B) Quantification of gene transcripts involved in ECM remodeling and production including Mmp13, Timp1, and IL-6. Data presented as Mean ± SEM (n = 4/group). Statistical analysis was performed using one-way ANOVA with Tukey's multiple comparisons test *p < 0.05; **p < 0.01; ***p < 0.005; ****p < 0.001.
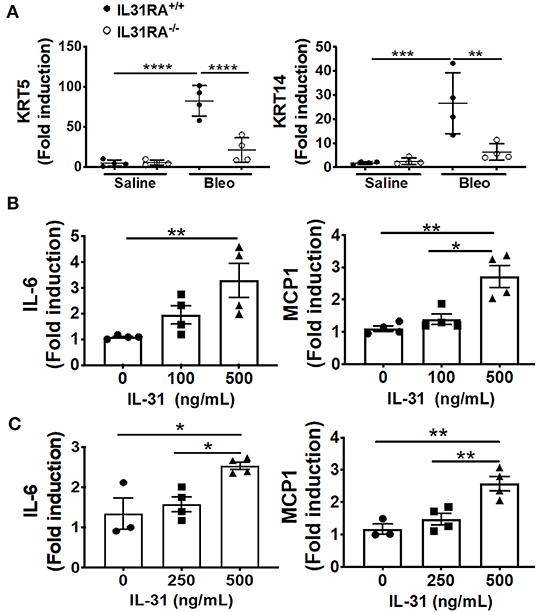
Figure 4. The loss of IL-31RA signaling alters the expression of epithelial cell-specific gene expression during bleomycin-induced pulmonary fibrosis. (A) Reduced expression of epithelial cell-specific genes including KRT5 and KRT14 in the lungs during bleomycin-induced pulmonary fibrosis. (B) Bronchial epithelial cell line BEAS-2B was stimulated with recombinant IL-31 for 24 h and the transcripts of MCP1 and IL-6 measured using RT-PCR. (C) primary normal human bronchial epithelial (HBEC) cells were stimulated with recombinant IL-31 for 24 h and the transcripts of MCP1 and IL-6 measured using RT-PCR. Data presented as Mean ± SEM (n = 4 mice/group). Statistical analysis was performed using one-way ANOVA with Tukey's multiple comparisons test *p < 0.05; **p < 0.01; ***p < 0.005; ****p < 0.001.
Increased Frequency of IL-31 Producing Cells in IPF Patients
To determine whether IL-31 is involved in human IPF, IL-31 staining was performed in the lung sections obtained from healthy controls and IPF patients. In contrast to normal lungs, we observed prominent immunostaining for IL-31 in cells populated in the thickened parenchymal areas of IPF lungs (Figure 5A). We quantified the number of IL-31-positive cells and total cells in lung images to assess the accumulation of IL-31-positive cells in IPF lungs compared to healthy lungs. There was a significant increase in the percent of IL-31-positive cells in the lungs from IPF patients compared with healthy controls (Figure 5B). Previous studies have described type 2 CD4 T cells as the main source of IL-31 production in chronic allergic diseases such as AD (11, 44). To determine the frequency of IL-31 producing CD4 T cells in IPF, PBMCs were stimulated with PMA-ionomycin or medium for 5 h and fixed. Cells were fluorescently stained with IL-31 and IL-4 and analyzed by flow cytometry with a gating strategy as illustrated in Supplementary Figure 2. Notably, the majority of IL-31 producing cells in PBMCs were T cells. CD4 T cells producing IL-31, or IL-4 and IL-31, were significantly increased in PBMCs of IPF patients compared to healthy controls (Figures 5C–E).
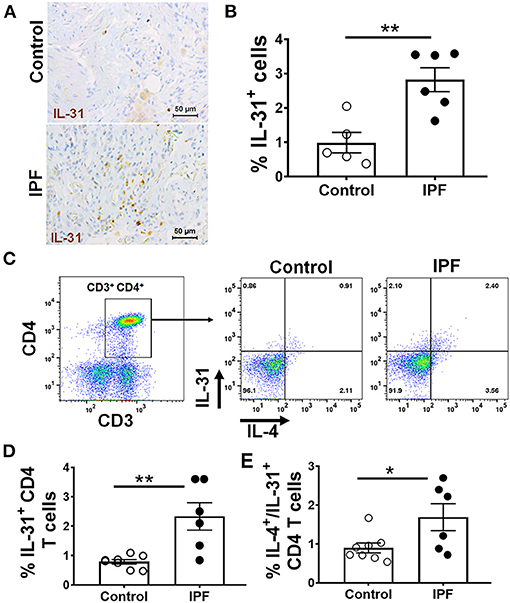
Figure 5. Increased staining and frequency of IL-31-positive CD4 T cells in IPF. Lung sections from IPF and non-IPF control subjects were probed to detect the expression of IL-31 by immunohistochemistry, (A) Representative images of IL-31 expression in non-IPF (top) and IPF (bottom) lung sections. (B) Percent of IL-31+ cells in total lung cells quantified in IHC stained lung sections. Data are shown as mean ± SEM., **p < 0.01. Peripheral blood mononuclear cells (PBMC) of IPF patients (n = 7) and healthy control subjects (n = 8) were isolated and stimulated with PMA and ionomycin, intracellular cytokine expression of IL-31 and IL-4 were assessed by flow cytometry. (C) Representative images of CD3 CD4 cells expressing IL-31 and IL-4. (D) Percent of IL-31 and (E) IL4/IL-31- producing CD4 T cells are shown. Data presented as mean ± SEM with n = 6-9, with *p < 0.05, **p < 0.01. Student t-test was used to compare the mean between groups.
Discussion
In this study, we have demonstrated that bleomycin-induced pulmonary fibrosis is dependent on IL-31RA-driven signaling. The loss of IL-31RA signaling was associated with a decrease in hydroxyproline and downregulation of several pro-fibrotic gene transcripts. In addition, our findings demonstrate that the loss of IL-31RA attenuates lung function decline as observed during bleomycin induced-pulmonary fibrosis. We also showed that IL-31-positive cells are increased in fibrotic lung lesions of IPF patients and IL-31 is predominantly produced by CD4 T cells. These findings illustrate the potential utility of targeting the IL-31/IL-31RA axis to treat IPF.
Previous studies have identified important roles for Th2 T cell-derived IL-4 and/or IL-13 in the development of pulmonary fibrosis and other fibrotic diseases. However, the role of IL-31/IL-31RA signaling in pulmonary fibrosis has remained unexplored. Our previous study along with other research studies have shown that the administration of exogenous IL-31, or overexpression of IL-31, in transgenic mice induces skin thickening and alters the skin barrier. These findings suggest that IL-31/IL-31RA signaling plays an integral role in inflammation and fibrosis (10, 11). Previous studies have suggested a potential role for IL-31-driven signaling in the development of fibrosis in various organs including the liver (23) and intestine (45). Shish and colleagues reported increased expression of IL-31RA on intestinal myofibroblasts following treatment with Tl1a, a TNF superfamily 15 protein, and established an association between IL-31RA signaling and colonic fibrosis (45). Here, we dissected the pro-fibrotic role of the IL-31/IL-31RA axis using a mouse model of bleomycin-induced pulmonary fibrosis. Our studies which were conducted using IL-31RA knockout mice revealed a significant decrease in hydroxyproline and subsequent improvement in lung function. In addition, whole lung transcriptome analysis revealed a decrease in the expression of ECM-related genes, which thus supports the role of IL-31/IL-31RA in lung fibrosis. Our findings align with a recent publication demonstrating that in scleroderma IL-31 contributes to skin fibrosis; in this study, a sub-cutaneous mini-pump was implanted in the skin to deliver IL-31 (21). We assessed changes in tissue inflammation using H&E staining of lung section from wildtype and IL-31RA knout-out mice treated with saline and bleomycin (Supplementary Figure 5). We observed limited or no changes in tissue inflammation between wildtype and IL-31RA knockout mice treated with bleomycin. Future studies are warranted to identify both qualitative and quantitative changes in inflammation in the absence of IL-31RA using alternative mouse models of airway inflammation. Together, these studies indicate that IL-31-driven signaling is a key factor driving pulmonary fibrosis. However, additional studies are needed to identify specific mechanisms by which IL-31 contributes to ECM production and decline in lung function during bleomycin-induced pulmonary fibrosis.
Previous studies have identified CD4 helper T cells as a source of IL-31 (11, 12) which is consistent with our findings. We demonstrated a significant increase in IL-31 and IL-4/IL-31-producing CD4+ T cells in PBMCs of IPF patients compared with control healthy subjects. The expression of IL-31 was significantly increased in lung tissues of IPF patients compared to control subjects. These findings may indicate that during pulmonary fibrosis development, the Th2 CD4+ T cell population contributes to the increased expression of IL-31 in fibrotic lung lesions; within these lung lesions, IL-31 interacts with the unique receptor IL-31RA that is expressed in stromal cells. Collectively, these observations make a compelling case that hematopoietic cell-derived IL-31 might play a role in the pathogenesis of pulmonary fibrosis by activating non-hematopoietic cells such as epithelial cells and mesenchymal cells. Indeed, stimulation of airway epithelial cells with IL-31 induced an increased production of MCP1 and IL-6 cytokines known to play a role in the development of pulmonary fibrosis (46). Recent studies have revealed that the expression of IL-31 was dependent of TGF-β1 signaling in different cell types and disease conditions including fibrosis and skin wound healing (22, 47, 48). However, TGF- β1 independent pathways might also regulate the expression of IL-31 on CD4+ T cells. A study by Hwang et al. has shown that JunB and the transcription factor Nuclear Factor of Activated T cells (NFAT1) proteins can regulate the expression of IL-31 on CD4+ T cells from AD murine model (49). However, further investigations are required to elucidate whether the expression of IL-31 by CD4+ T cells is also mediated by TGF-β1 signaling.
We applied the whole lung transcriptome through RNA seq analysis to identify IL-31/IL-31RA -dependent genes and pathways that might participate in IL-31-driven pulmonary fibrosis. Expression of genes associated with the ECM formation was significantly downregulated in IL-31RA−/− mice treated with bleomycin when compared to wildtype mice. Notably, genes associated with epithelial tissue integrity (Krt5 and Krt14), protein Gα signaling, as well as previously reported genes associated with lung function and remodeling (Arg1, Ccl28 and Rgs4) were down regulated in IL-31RA−/− fibrotic mice (50–52). Weathington et al. (53) have recently reported an association between increased expression of the genes KCNJ2 and Krt18 in the setting of asthma, suggesting a strong relationship between lung function and tissue remodeling. In support, genes related to membrane potential regulation, including genes for potassium channels Kcna2, Kcnh4, and Kcnu1, were upregulated in IL-31RA−/− mice compared to wildtype mice. These findings support a mechanistic pathway that mediates improved lung function in the absence of IL-31RA signaling. Additional investigation is needed to explore whether IL-31/IL-31RA signaling contributes to the regulation of potassium channel activity and whether altered keratin gene expression in epithelial cells could mediate collagen deposition and lung function.
In conclusion, our data indicates that genetic deletion of IL-31RA significantly attenuates collagen deposition and lung function decline induced during repetitive bleomycin-induced injury and pulmonary fibrosis. In recent years, several cytokines have been implicated in the pathogenesis of pulmonary fibrosis. While IL-31 has been identified in several inflammatory and remodeling diseases, mechanistic studies evaluating IL-31 producing cells in IPF, and the role of IL-31 in pulmonary fibrosis have been missing. Our results provide new evidence that supports the pathogenic role of IL-31RA-driven signaling in bleomycin-induced pulmonary fibrosis. The IL-31-IL31RA axis may serve as a novel therapeutic target for the treatment of pulmonary fibrosis.
Data Availability Statement
The datasets presented in this study can be found in online repositories. The names of the repository/repositories and accession number(s) can be found in the article/Supplementary Material.
Ethics Statement
The studies involving human participants were reviewed and approved by IRB. The patients/participants provided their written informed consent to participate in this study. The animal study was reviewed and approved by IACUC. Written informed consent was obtained from the individual(s) for the publication of any potentially identifiable images or data included in this article.
Author Contributions
SM conceived and designed the research. DY and SM performed the experiments and wrote the manuscript. AJ performed bioinformatic analysis and edited the manuscript. NG provided human IPF samples and edited the manuscript. VO analyzed data and edited the manuscript. All authors contributed to the article and approved the submitted version.
Funding
This work was supported by NIH [1R01 HL134801, 1R21 AI137309, and W81XWH-17-1-0666 (SM)].
Conflict of Interest
The authors declare that the research was conducted in the absence of any commercial or financial relationships that could be construed as a potential conflict of interest.
Acknowledgments
The authors thank Dr. Brijendra Singh, Dr. Edukulla Ramakrishna, and the veterinary services and pathology research core at Cincinnati Children's Hospital Medical Center for help in this study. We also thank Dr. Stacey R. Dillon (Bristol-Myers Squibb Company) for providing breeding pairs of IL-31RA knockout mice.
Supplementary Material
The Supplementary Material for this article can be found online at: https://www.frontiersin.org/articles/10.3389/fimmu.2021.645717/full#supplementary-material
References
1. Sgalla G, Iovene B, Calvello M, Ori M, Varone F, Richeldi L. Idiopathic pulmonary fibrosis: pathogenesis and management. Respir Res. (2018) 19:32. doi: 10.1186/s12931-018-0730-2
2. Wolters PJ, Collard HR, Jones KD. Pathogenesis of idiopathic pulmonary fibrosis. Annu Rev Pathol. (2014) 9:157–79. doi: 10.1146/annurev-pathol-012513-104706
3. Richeldi L, du Bois RM, Raghu G, Azuma A, Brown KK, Costabel U, et al. Efficacy and safety of nintedanib in idiopathic pulmonary fibrosis. N Engl J Med. (2014) 370:2071–82. doi: 10.1056/NEJMoa1402584
4. King TE Jr, Bradford WZ, Castro-Bernardini S, Fagan EA, Glaspole I, Glassberg MK, et al. A phase 3 trial of pirfenidone in patients with idiopathic pulmonary fibrosis. N Engl J Med. (2014) 370:2083–92. doi: 10.1056/NEJMoa1402582
5. Sontake V, Gajjala PR, Kasam RK, Madala SK. New therapeutics based on emerging concepts in pulmonary fibrosis. Expert Opin Ther Targets. (2019) 23:69–81. doi: 10.1080/14728222.2019.1552262
6. Wilson MS, Madala SK, Ramalingam TR, Gochuico BR, Rosas IO, Cheever AW, et al. Bleomycin and IL-1beta-mediated pulmonary fibrosis is IL-17A dependent. J Exp Med. (2010) 207:535–52. doi: 10.1084/jem.20092121
7. Kim KK, Sheppard D, Chapman HA. TGF-beta1 signaling and tissue fibrosis. Cold Spring Harb Perspect Biol. (2018) 10:22293. doi: 10.1101/cshperspect.a022293
8. Singh B, Kasam RK, Sontake V, Wynn TA, Madala SK. Repetitive intradermal bleomycin injections evoke T-helper cell 2 cytokine-driven pulmonary fibrosis. Am J Physiol Lung Cell Mol Physiol. (2017) 313:L796–L806. doi: 10.1152/ajplung.00184.2017
9. Gieseck RL 3rd, Wilson MS, Wynn TA. Type 2 immunity in tissue repair and fibrosis. Nat Rev Immunol. (2018) 18:62–76. doi: 10.1038/nri.2017.90
10. Singh B, Jegga AG, Shanmukhappa KS, Edukulla R, Khurana Hershey GH, Medvedovic M, et al. IL-31-driven skin remodeling involves epidermal cell proliferation and thickening that lead to impaired skin-barrier function. PLoS ONE. (2016) 11:e0161877. doi: 10.1371/journal.pone.0161877
11. Dillon SR, Sprecher C, Hammond A, Bilsborough J, Rosenfeld-Franklin M, Presnell SR, et al. Interleukin 31, a cytokine produced by activated T cells, induces dermatitis in mice. Nat Immunol. (2004) 5:752–60. doi: 10.1038/ni1084
12. Maier E, Werner D, Duschl A, Bohle B, Horejs-Hoeck J. Human Th2 but not Th9 cells release IL-31 in a STAT6/NF-kappaB-dependent way. J Immunol. (2014) 193:645–54. doi: 10.4049/jimmunol.1301836
13. Raap U, Gehring M, Kleiner S, Rudrich U, Eiz-Vesper B, Haas H, et al. Human basophils are a source of - and are differentially activated by - IL-31. Clin Exp Allergy. (2017) 47:499–508. doi: 10.1111/cea.12875
14. Kunsleben N, Rudrich U, Gehring M, Novak N, Kapp A, Raap U. IL-31 induces chemotaxis, calcium mobilization, release of reactive oxygen species, and CCL26 in eosinophils, which are capable to release IL-31. J Invest Dermatol. (2015) 135:1908–11. doi: 10.1038/jid.2015.106
15. Yagi Y, Andoh A, Nishida A, Shioya M, Nishimura T, Hashimoto T, et al. Interleukin-31 stimulates production of inflammatory mediators from human colonic subepithelial myofibroblasts. Int J Mol Med. (2007) 19:941–6. doi: 10.3892/ijmm.19.6.941
16. Ip WK, Wong CK, Li ML, Li PW, Cheung PF, Lam CW. Interleukin-31 induces cytokine and chemokine production from human bronchial epithelial cells through activation of mitogen-activated protein kinase signalling pathways: implications for the allergic response. Immunology. (2007) 122:532–41. doi: 10.1111/j.1365-2567.2007.02668.x
17. Cevikbas F, Wang XD, Akiyama T, Kempkes C, Savinko T, Antal A, et al. A sensory neuron-expressed IL-31 receptor mediates T helper cell-dependent itch: involvement of TRPV1 and TRPA1. J Allergy Clin Immun. (2014) 133:448–+. doi: 10.1016/j.jaci.2013.10.048
18. Oyama S, Kitamura H, Kuramochi T, Higuchi Y, Matsushita H, Suzuki T, et al. Cynomolgus monkey model of interleukin-31-induced scratching depicts blockade of human interleukin-31 receptor A by a humanized monoclonal antibody. Exp Dermatol. (2018) 27:14–21. doi: 10.1111/exd.13236
19. Psachoulia K, Chamberlain KA, Heo D, Davis SE, Paskus JD, Nanescu SE, et al. IL4I1 augments CNS remyelination and axonal protection by modulating T cell driven inflammation. Brain. (2016) 139:3121–36. doi: 10.1093/brain/aww254
20. Lin W, Zhou Q, Liu C, Ying M, Xu S. Increased plasma IL-17, IL-31, and IL-33 levels in chronic spontaneous urticaria. Sci Rep. (2017) 7:17797. doi: 10.1038/s41598-017-18187-z
21. Yaseen B, Lopez H, Taki Z, Zafar S, Rosario H, Abdi BA, et al. Interleukin-31 promotes pathogenic mechanisms underlying skin and lung fibrosis in scleroderma. Rheumatology. (2020) 59:2625–36. doi: 10.1093/rheumatology/keaa195
22. Shi K, Jiang J, Ma T, Xie J, Duan L, Chen R, et al. Pathogenesis pathways of idiopathic pulmonary fibrosis in bleomycin-induced lung injury model in mice. Respir Physiol Neurobiol. (2014) 190:113–7. doi: 10.1016/j.resp.2013.09.011
23. Ming D, Yu X, Guo R, Deng Y, Li J, Lin C, et al. Elevated TGF-beta1/IL-31 pathway is associated with the disease severity of hepatitis B virus-related liver cirrhosis. Viral Immunol. (2015) 28:209–16. doi: 10.1089/vim.2014.0142
24. Stawski L, Trojanowska M. Oncostatin M and its role in fibrosis. Connect Tissue Res. (2019) 60:40–49. doi: 10.1080/03008207.2018.1500558
25. Edukulla R, Singh B, Jegga AG, Sontake V, Dillon SR, Madala SK. Th2 cytokines augment IL-31/IL-31RA interactions via STAT6-dependent IL-31RA expression. J Biol Chem. (2015) 290:13510–20. doi: 10.1074/jbc.M114.622126
26. Bilsborough J, Mudri S, Chadwick E, Harder B, Dillon SR. IL-31 receptor (IL-31RA) knockout mice exhibit elevated responsiveness to oncostatin M. J Immunol. (2010) 185:6023–30. doi: 10.4049/jimmunol.0902769
27. Sontake V, Kasam RK, Sinner D, Korfhagen TR, Reddy GB, White ES, et al. Wilms' tumor 1 drives fibroproliferation and myofibroblast transformation in severe fibrotic lung disease. JCI Insight. (2018) 3:121252. doi: 10.1172/jci.insight.121252
28. Madala SK, Edukulla R, Davis KR, Schmidt S, Davidson C, Kitzmiller JA, et al. Resistin-like molecule alpha1 (Fizz1) recruits lung dendritic cells without causing pulmonary fibrosis. Resp Res. (2012) 13:51. doi: 10.1186/1465-9921-13-51
29. Madala SK, Schmidt S, Davidson C, Ikegami M, Wert S, Hardie WD. MEK-ERK pathway modulation ameliorates pulmonary fibrosis associated with epidermal growth factor receptor activation. Am J Respir Cell Mol Biol. (2012) 46:380–8. doi: 10.1165/rcmb.2011-0237OC
30. Madala SK, Edukulla R, Schmidt S, Davidson C, Ikegami M, Hardie WD. Bone marrow-derived stromal cells are invasive and hyperproliferative and alter transforming growth factor-alpha-induced pulmonary fibrosis. Am J Respir Cell Mol Biol. (2014) 50:777–86. doi: 10.1165/rcmb.2013-0042OC
31. Kasam RK, Ghandikota S, Soundararajan D, Reddy GB, Huang SK, Jegga AG, et al. Inhibition of Aurora Kinase B attenuates fibroblast activation and pulmonary fibrosis. EMBO Mol Med. (2020) 12:e12131. doi: 10.15252/emmm.202012131
32. Nobbe S, Dziunycz P, Muhleisen B, Bilsborough J, Dillon SR, French LE, et al. IL-31 expression by inflammatory cells is preferentially elevated in atopic dermatitis. Acta Derm Venereol. (2012) 92:24–8. doi: 10.2340/00015555-1191
33. Kasam RK, Gajjala PR, Jegga AG, Courtney JA, Randell SH, Kramer EL, et al. Fibrocyte accumulation in the lungs of cystic fibrosis patients. J Cyst Fibros. (2020) 19:815–22. doi: 10.1016/j.jcf.2020.06.011
34. Sontake V, Shanmukhappa SK, DiPasquale BA, Reddy GB, Medvedovic M, Hardie WD, et al. Fibrocytes regulate Wilms tumor 1-positive cell accumulation in severe fibrotic lung disease. J Immunol. (2015) 195:3978–91. doi: 10.4049/jimmunol.1500963
35. Yombo DJK, Mentink-Kane MM, Wilson MS, Wynn TA, Madala SK. Heat shock protein 70 is a positive regulator of airway inflammation and goblet cell hyperplasia in a mouse model of allergic airway inflammation. J Biol Chem. (2019) 294:15082–94. doi: 10.1074/jbc.RA119.009145
36. Madala SK, Edukulla R, Phatak M, Schmidt S, Davidson C, Acciani TH, et al. Dual targeting of MEK and PI3K pathways attenuates established and progressive pulmonary fibrosis. PLoS ONE. (2014) 9:e86536. doi: 10.1371/journal.pone.0086536
37. Chen J, Bardes EE, Aronow BJ, Jegga AG. ToppGene Suite for gene list enrichment analysis and candidate gene prioritization. Nucleic Acids Res. (2009) 37:W305–11. doi: 10.1093/nar/gkp427
38. Sontake V, Wang Y, Kasam RK, Sinner D, Reddy GB, Naren AP, et al. Hsp90 regulation of fibroblast activation in pulmonary fibrosis. JCI Insight. (2017) 2:e91454. doi: 10.1172/jci.insight.91454
39. DePianto DJ, Chandriani S, Abbas AR, Jia G, N'Diaye EN, Caplazi P, et al. Heterogeneous gene expression signatures correspond to distinct lung pathologies and biomarkers of disease severity in idiopathic pulmonary fibrosis. Thorax. (2015) 70:48–56. doi: 10.1136/thoraxjnl-2013-204596
40. Hudock KM, Collins MS, Imbrogno M, Snowball J, Kramer EL, Brewington JJ, et al. Neutrophil extracellular traps activate IL-8 and IL-1 expression in human bronchial epithelia. Am J Physiol Lung Cell Mol Physiol. (2020) 319:L137–47. doi: 10.1152/ajplung.00144.2019
41. Brewington JJ, Backstrom J, Feldman A, Kramer EL, Moncivaiz JD, Ostmann AJ, et al. Chronic beta2AR stimulation limits CFTR activation in human airway epithelia. JCI Insight. (2018) 3:93029. doi: 10.1172/jci.insight.93029
42. Ryu JH, Moua T, Daniels CE, Hartman TE, Yi ES, Utz JP, et al. Idiopathic pulmonary fibrosis: evolving concepts. Mayo Clin Proc. (2014) 89:1130–42. doi: 10.1016/j.mayocp.2014.03.016
43. Wynn TA, Ramalingam TR. Mechanisms of fibrosis: therapeutic translation for fibrotic disease. Nat Med. (2012) 18:1028–40. doi: 10.1038/nm.2807
44. Kim HJ, Zeidi M, Bonciani D, Pena SM, Tiao J, Sahu S, et al. Itch in dermatomyositis: the role of increased skin interleukin-31. Br J Dermatol. (2018) 179:669–78. doi: 10.1111/bjd.16498
45. Shih DQ, Zheng L, Zhang X, Zhang H, Kanazawa Y, Ichikawa R, et al. Inhibition of a novel fibrogenic factor Tl1a reverses established colonic fibrosis. Mucosal Immunol. (2014) 7:1492–503. doi: 10.1038/mi.2014.37
46. Ayaub EA, Dubey A, Imani J, Botelho F, Kolb MRJ, Richards CD, et al. Overexpression of OSM and IL-6 impacts the polarization of pro-fibrotic macrophages and the development of bleomycin-induced lung fibrosis. Sci Rep. (2017) 7:13281. doi: 10.1038/s41598-017-13511-z
47. Xu J, Zanvit P, Hu L, Tseng PY, Liu N, Wang F, et al. The cytokine TGF-beta induces interleukin-31 expression from dermal dendritic cells to activate sensory neurons and stimulate wound itching. Immunity. (2020) 53:371–83.e5. doi: 10.1016/j.immuni.2020.06.023
48. Jawa RS, Chattopadhyay S, Tracy E, Wang Y, Huntoon K, Dayton MT, et al. Regulated expression of the IL-31 receptor in bronchial and alveolar epithelial cells, pulmonary fibroblasts, pulmonary macrophages. J Interferon Cytokine Res. (2008) 28:207–19. doi: 10.1089/jir.2007.0057
49. Hwang JS, Kim GC, Park E, Kim JE, Chae CS, Hwang W, et al. NFAT1 and JunB cooperatively regulate IL-31 gene expression in CD4+ T cells in health and disease. J Immunol. (2015) 194:1963–74. doi: 10.4049/jimmunol.1401862
50. Wong GS, Redes JL, Balenga N, McCullough M, Fuentes N, Gokhale A, et al. RGS4 promotes allergen- and aspirin-associated airway hyperresponsiveness by inhibiting PGE2 biosynthesis. J Allergy Clin Immunol. (2020) 146:1152–64 e13. doi: 10.1016/j.jaci.2020.03.004
51. Huang J, Yue H, Jiang T, Gao J, Shi Y, Shi B, et al. IL-31 plays dual roles in lung inflammation in an OVA-induced murine asthma model. Biol Open. (2019) 8:36244. doi: 10.1242/bio.036244
52. Thomas MA, Buelow BJ, Nevins AM, Jones SE, Peterson FC, Gundry RL, et al. Structure-function analysis of CCL28 in the development of post-viral asthma. J Biol Chem. (2015) 290:4528–36. doi: 10.1074/jbc.M114.627786
Keywords: interleukin 31, interleukin 31 receptor alpha, lung, idiopathic pulmonary fibrosis, bleomycin IL-31 regulation of pulmonary fibrosis
Citation: Yombo DJK, Odayar V, Gupta N, Jegga AG and Madala SK (2021) The Protective Effects of IL-31RA Deficiency During Bleomycin-Induced Pulmonary Fibrosis. Front. Immunol. 12:645717. doi: 10.3389/fimmu.2021.645717
Received: 23 December 2020; Accepted: 22 February 2021;
Published: 19 March 2021.
Edited by:
Remo Castro Russo, Federal University of Minas Gerais, BrazilReviewed by:
Viswanathan Natarajan, University of Illinois at Chicago, United StatesBernahrd Ryffel, Centre National de la Recherche Scientifique (CNRS), France
Copyright © 2021 Yombo, Odayar, Gupta, Jegga and Madala. This is an open-access article distributed under the terms of the Creative Commons Attribution License (CC BY). The use, distribution or reproduction in other forums is permitted, provided the original author(s) and the copyright owner(s) are credited and that the original publication in this journal is cited, in accordance with accepted academic practice. No use, distribution or reproduction is permitted which does not comply with these terms.
*Correspondence: Satish K. Madala, c2F0aXNoLm1hZGFsYUBjY2htYy5vcmc=