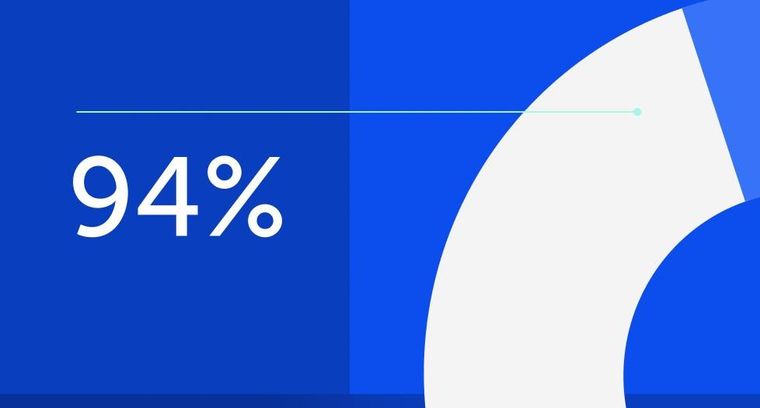
94% of researchers rate our articles as excellent or good
Learn more about the work of our research integrity team to safeguard the quality of each article we publish.
Find out more
ORIGINAL RESEARCH article
Front. Immunol., 30 September 2021
Sec. Vaccines and Molecular Therapeutics
Volume 12 - 2021 | https://doi.org/10.3389/fimmu.2021.645299
This article is part of the Research TopicResearch, Development and Clinical Trials for Peptides-Based VaccinesView all 12 articles
Advances in high-throughput sequencing have revolutionized the manner with which we can study T cell responses. We describe a woman who received a human papillomavirus (HPV) therapeutic vaccine called PepCan, and experienced complete resolution of her cervical high-grade squamous intraepithelial lesion. By performing bulk T cell receptor (TCR) β deep sequencing of peripheral blood mononuclear cells before and after 4 vaccinations, 70 putatively vaccine-specific clonotypes were identified for being significantly increased using a beta-binomial model. In order to verify the vaccine-specificity of these clonotypes, T cells with specificity to a region, HPV 16 E6 91-115, previously identified to be vaccine-induced using an interferon-γ enzyme-linked immunospot assay, were sorted and analyzed using single-cell RNA-seq and TCR sequencing. HPV specificity in 60 of the 70 clonotypes identified to be vaccine-specific was demonstrated. TCR β bulk sequencing of the cervical liquid-based cytology samples and cervical formalin-fixed paraffin-embedded samples before and after 4 vaccinations demonstrated the presence of these HPV-specific T cells in the cervix. Combining traditional and cutting-edge immunomonitoring techniques enabled us to demonstrate expansion of HPV-antigen specific T cells not only in the periphery but also in the cervix. Such an approach should be useful as a novel approach to assess vaccine-specific responses in various anatomical areas.
Human papillomavirus (HPV) is best known as the causative agent of cervical cancer, but it can also cause cancers at other mucosal sites including the anus, oropharynx, penis, vagina, and vulva. It is estimated that HPV is responsible for 42,700 cancers in the US each year (1), including more than 90% of anal and cervical cancers and about 70% of oropharyngeal, vaginal, and vulvar cancers (1). Incidences of HPV-associated anal and oropharyngeal cancers have increased notably, and although incidence of cervical cancer has stabilized after significant decreases over the past several decades (2), this remains the fourth most common cancer among women globally (3). The available prophylactic vaccines are effective for preventing HPV infections, but they cannot eliminate established infections; therapeutic vaccines could fill this need. Such vaccines would benefit young women (narrowly, those ≤24 years old), and broadly, any woman who plans to become pregnant (4) because increased incidence of preterm delivery (from 4.4% to 8.9%) is associated with surgical treatments (e.g., loop electrical excision procedure [LEEP]) for high-grade squamous intraepithelial lesion (HSIL) (4, 5). Furthermore, HPV infection has been shown to be associated with inability to conceive (6, 7), spontaneous abortion (8), and male infertility (9, 10). With the goal of circumventing these adverse obstetrics and reproductive outcomes as well as for treating cervical cancer, a variety of HPV therapeutic vaccines are in development including DNA-based (11), peptide-based (12, 13), and bacterial vector-based (14) delivery.
We evaluated the safety of an HPV therapeutic vaccine (PepCan) in a single-center, single-arm, dose-escalation Phase I clinical trial treating women with biopsy-proven HSILs (NCT01653249) (15, 16). PepCan consists of four current good manufacturing practice (cGMP)-grade peptides covering the human papillomavirus type 16 (HPV 16) E6 protein (amino acids 1-45, 46-80, 81-115, and 116-158) and Candida albicans skin test reagent (Candin®, Nielsen Biosciences, San Diego, CA). PepCan was shown to be safe, and resulted in a histological regression rate of 45% which is roughly double that of a historical placebo (22%) (17). In addition, circulating, peripheral T-helper type 1 (Th1) cells (p=0.0004) were increased, and the HPV 16 viral load was significantly decreased (p=0.008) (15).
Recent advances in high-throughput sequencing technology have enhanced our ability to appreciate how the T cell receptor (TCR) repertoire may reveal the role of T cells in immunotherapy for HPV-related diseases (18–20). The actual diversity present in a human body is estimated to be around 1013 unique TCRs (21). Next generation sequencing can facilitate the simultaneous analysis of millions of TCR sequences. Understanding the cytotoxic T cell repertoire, in parallel with observing clinical responses, would be insightful for revealing immune mechanisms behind immunotherapies for chronic infectious diseases or cancer (18, 22–25). However, the use of high-throughput sequencing technology alone can only identify putatively vaccine-specific T cells on a statistical basis, but it is not able to verify their specificity on an immunological basis. In this article, we utilize multiplexed PCR-based TCR sequencing using genomic DNA to characterize TCR repertoires in peripheral blood mononuclear cells (PBMCs), stimulated CD3+ T cells, cervical formalin-fixed paraffin-embedded (FFPE) tissues, and cervical liquid-based cytology (LBC) samples from one subject who was a histologic responder from the Phase I clinical trial mentioned above. In addition, single-cell RNA-seq and TCR sequencing approaches were utilized to reveal the TCR sequences of HPV-specific T cells with a specificity to the HPV 16 E6 91-115 amino acid region revealed by the enzyme-linked immunospot (ELISPOT) assay. We provide proof-of-principle that a traditional assay, such as ELISPOT, can be combined with a cutting-edge technology to better characterize the specificities of T cells generated by vaccination.
The subject, a 41-year old Caucasian woman, participated in a single-arm, open-label Phase I clinical trial of an HPV therapeutic vaccine, PepCan, for treating biopsy-proven cervical HSILs (Figure 1A) (15, 16). At study entry, she had cervical intraepithelial neoplasia 3 (CIN 3), and was positive for HPV types 16, 31, and 58. At study exit (12 weeks after vaccination series completion), her LEEP biopsy was benign but was noted to have marked lymphocytic infiltration. Furthermore, she was noted to have leukocytosis and lymphocytosis (Table 1), and was positive for HPV 40 at exit. ELISPOT assay showed CD3+ IFN-γ+ T cell responses specific to multiple regions of HPV 16 E6 and E7 protein before and after the vaccinations. The response to one region, HPV 16 E6 91-115, was significantly increased after 4 vaccinations (Figure 1B, p=0.023). Peripheral immune cell profiling showed an increased percentage of Th1 cells, but unchanged levels of Tregs and Th2 cells (Figure 1C). Her HLA types were HLA-A*24/A*30, B*15/B*51, C*01/C*03, DPB1*02/DPB1*02, DQB1*03/DQB1*06, and DRB1*11/DRB1*13.
Figure 1 The Phase I clinical trial design and routine immune monitoring assays. (A) Clinical trial design of the Phase I study. Vaccination (PepCan) visits were scheduled 3 weeks apart for patients who had biopsy-confirmed cervical high-grade squamous intraepithelial lesions (HSILs, i.e. CIN grade 2 or 3). Blood draws were performed pre-vaccination, and post-2 and post-4 vaccinations. Cervical local samples (LBC and FFPE) were collected pre-vaccination and post-4 vaccinations. FFPE samples were prepared from a pre-vaccination cervical biopsy and from loop electrical excision procedure (LEEP) biopsy post-4 vaccinations. (B) Immunogenic HPV16 E6 and E7 regions were determined for each vaccine phase using IFN-γ ELISPOT assay. In pre-vaccine phase, positive responses (i.e., at least twice the media control) were detected in the E6 16-40, E6 31-55, and E6 106-130 regions. Positive responses were seen in the E6 1-25, E6 106-130, E6 136-158, and E7 1-25 regions in the post-2 vaccination sample, and in the E6 31-55, E6 91-115, and E7 46-70 regions in the post-4 vaccination sample. The increase in the response to the HPV16 E6 91-115 regions was statistically significant (paired t-test, p=0.023) after 4 vaccinations. Phytohemagglutinin was used as a positive control (not shown). The y-axis represents mean spot forming units of triplicates per 1 x 106 CD3+ T cells, and error bars represent standard error of means. (C) The fluorescent cell sorter analysis of PBMCs revealed that the Th1 (CD4+Tbet+) level expressed as the percentage of CD4+ T cells increased after 4 vaccinations, but Treg (CD4+CD25+FoxP3+) and Th2 (CD4+GATA3) levels were minimally changed.
All samples examined (n=10: PBMCs and stimulated CD3+ T cell samples at pre-, post-2, and post-4 vaccinations; and FFPE and LBC samples at pre- and post-4 vaccinations) yielded sufficient quantities of DNA for bulk TCR sequencing. In total, 749,417 clonotypes, and 1,256,277 T cells were identified in these 10 samples (Table 2). The numbers of total T cells and clonotypes were higher in PBMCs than in stimulated CD3+ T cell samples (Figure 2). In cervical samples, the clonotypes and total T cells were detected in FFPE (pre- and post-4) and LBC (pre- and post-4). The productive clonality was increased after 4 vaccinations in PBMC, stimulated CD3+ T cell, and LBC samples, and the maximum productive frequencies at least doubled in all sample types (Figure 2). The T cell fraction was highest in stimulated CD3+ T cells, and lowest in LBCs. DNA per cell was similar among PBMCs, stimulated CD3+ T cells, and LBC (ranging from 0.0061 ng/cell to 0.011 ng/cell), but much higher in FFPE samples (0.714 ng/cell for pre-vaccination and 1.27 ng/cell for post-4 vaccinations).
Figure 2 T cell structures of PBMCs stimulated CD3+ T cells, LBC, and FFPE samples described with multiplexed PCR-based TCR sequencing using genomic DNA. The T cell structures of the 4 sample types (PBMCs, stimulated CD3+ T cells, LBC, and FFPE) are shown as the total number of T cells defined by nucleotide sequence, productive clonality (one minus normalized Shannon’s entropy for all productive rearrangements), fraction of T cells (the number of productive templates divided by the number of nucleated cells), number of clonotypes defined by nucleotide sequence, maximum productive frequency (the most frequent specific productive rearrangement among all productive rearrangements within a sample), and the quantity of DNA (ng) per nucleated cell. The number of nucleated cells were determined using amplification of reference gene primers.
The percentages of the top 15 most frequent clonotypes were significantly increased after 4 vaccinations in all sample types except for FFPE (Figure 3A). Venn diagrams of clonotypes detected in PBMCs, LBC, and FFPE at pre-vaccination and post-4 vaccinations revealed that some clonotypes can be detected in all sample types, reflecting the capacity of at least a subset of T cells to traffic to the cervix (Figure 3B). The presence of T cells in peripheral blood and cervix was shown. Both LBC and FFPE samples from the cervix were analyzed. However, the quality of DNA from FFPE may have been low as approximately one hundred fold larger quantity of DNA per nucleated cell was reported (Table 2). This may possibly have led to incomplete identification of TCRs because TCR DNA split in multiple fragments cannot be identified. So, the overlap between FFPE and peripheral blood may not be as reliable as an overlap between LBC and peripheral blood. A beta-binomial model, which accounts for variance due to random sampling from a highly diverse repertoire and time-dependent variance for identifying clinically relevant expansion of T cells (26), was used to identify putatively vaccine-specific TCRs using pre- and post-4 vaccination PBMC samples. Seventy putatively vaccine-specific TCRs were identified using the CDR3 nucleotide sequences (Supplementary Table 1). The numbers of such clonotypes and total T cells in pre- and post-4 vaccination FFPE (1 and 9 clonotypes, and 1 and 13 total T cells, respectively) and pre- and post-4 vaccination LBC [14 and 47 clonotypes (Figure 3C), and 33 and 1,523 total T cells respectively] showed that LBC may be more an informative sample type compared to FFPE because of a greater T cell abundance and better DNA quality.
Figure 3 Tracking of clonotypes in the peripheral blood and cervix. (A) Tracking of the top 15 clonotypes defined by nucleotide sequence are shown in productive frequency. The top 15 highest frequency clonotypes were significantly decreased after 2 vaccinations (Wilcoxon matched-pairs signed-ranks test, p=0.0012), but significantly increased after 4 vaccinations (p<0.0001) in PBMC samples calculated using the numbers of T cells. For stimulated CD3+ T cell samples, significant increases were seen between pre-vaccination and post-4 vaccinations (p=0.034) and between post-2 and post-4 vaccinations samples (p=0.0034). A significant increase was seen in LBC samples (p<0.0001) but not in FFPE samples. (B) Venn diagrams of clonotypes defined by nucleotides in PBMC, LBC, and FFPE samples pre-vaccination and post-4 vaccinations. Most clonotypes appear only in one sample type, but there are 17 TCRs present in PBMCs, LBC, and FFPE at the pre-vaccination visit and 72 TCRs at the post-4 vaccination visit. (C) Putatively vaccine-specific clonotypes in LBC samples before and after 4 vaccinations. Seventy putatively vaccine-specific clonotypes were identified through a comparison of post-4 PBMC and pre PBMC samples using the beta-binomial model (shown as red dots with and without black circular borders). Red dots with black circular borders represent these putatively vaccine-specific TCRs present in pre-vaccination LBC sample (n=15) and in post-4 vaccination LBC sample (n=57). Dark grey dots are not significantly different between pre-vaccination and post-4 vaccinations PBMC samples. Dark grey dots with black circular borders are not significantly increased but are present in the respective LBC sample. Light grey dots without black circular borders are not present in the respective LBC sample.
Of 8.5 x 106 peptide (three 15-mer peptides covering the HPV 16 E6 91-115 region)-stimulated and IFN-γ labeled cells from monocyte depleted post-4 vaccination PBMCs, 1.3 x 106 (15.3%) were positively sorted. For the TCR sequencing, the estimated number of cells was 12,240 with mean read pairs of 13,678 per cell. Most (10,246 of 12,240 or 83.7%) cells contained productive V-J spanning pairs. The TCR β amino acid sequences of the 4 clonotypes with a frequency of ≥5% among the IFN-γ positive cells are shown in Table 3.
The single-cell RNA-seq analysis revealed an estimated 15,114 total number of cells, 32,659 mean reads per cell, and 2,047 median number of genes per cell. After filtering and normalization, cells were clustered into 9 separated populations (Figure 4A). Notably, abundant expression of IFN-γ and tumor necrosis factor (TNF), but not interleukin-4 (IL-4), was detected in cluster #1, #2 and #3 within the CD8+ T-cell populations, as shown in violin and feature plots (Figures 4B, C). These results corroborate the importance of the role of the Th1 cells play as previously demonstrated using the fluorescent-activated cell sorter analysis (Figure 1C).
Figure 4 Single-cell gene expression profile of HPV 16 E6 91-115 specific T cells. (A) A UMAP plot showing 9 clusters based on gene expression profiles. (B) Violin plots showing CD3D, CD4, CD8A, IFN-γ, TNF, and IL-4 gene expression. (C) Feature plots showing CD3D, CD4, CD8A, IFN-γ, TNF, and IL-4 gene expression.
Using the TCR β CDR3 sequences of the 4 clonotypes specific for HPV 16 E6 91-115, their frequencies in PBMCs, LBC, and FFPE samples were determined using TCR β chain sequencing (Figure 5). All 4 clonotypes were detectable in PBMCs and LBC prior to vaccination, and their expansion after 4 vaccinations is shown. Only one T cell of clonotype 2 is detectable prior to vaccination in FFPE. All 4 clonotypes were detectable after 4 vaccinations, but only at 2 T cells for clonotypes 1, 3, and 4, and 1 T cell for clonotype 2. As much fewer cells were detected in FFPE, LBC was a better source for assessing T cell populations, at least in this subject. All 4 clonotypes were represented in the top 15 most frequent clonotypes for PBMCs, LBC, and stimulated CD3+ T cells, but only clonotype 1 was present in FFPE (Figure 3A). Of the 70 clonotypes identified to be putatively vaccine-specific using the beta-biomial model, 60 clonotypes were shown to be HPV 16 E6 91-115 specific (Supplementary Table 1). Clonotype 1 was the most abundant clonotype in PBMCs and LBC, and the second most abundant clonotype in stimulated CD3+ T cells.
Figure 5 Tracking HPV 16 E6 91-115 specific T cells in PBMC, LBC, and FFPE. The TCR Vα and Vβ sequences of HPV 16 E6 91-115 specific T cells were determined by sorting and sequencing such cells based on IFN-γ secretion upon peptide stimulation. The TCR Vβ CDR3 sequences of top 4 clonotypes (≥ 5% of IFN-γ secreting cells) are shown in Table 3. The frequencies of these clonotypes in PBMC, LBC, and FFPE at pre-vaccination (yellow), post-2 vaccinations (blue), and post-4 vaccinations (red) time points are shown. All 4 vaccine-specific clonotypes in PBMC and LBC increased in frequency after 4 vaccinations. On the other hand, data from FFPE were not as informative.
This was a proof-of-concept study to demonstrate the utility of TCR analyses using high-throughput sequencing technology in the context of HPV therapeutic vaccine trials. The earliest evidence of the link between HPV and cervical cancer was discovered in 1983 by Harald zur Hausen and his colleagues (27) to whom a Nobel Prize was later awarded. To date, over 200 HPV types have been described (28). HPV antigens are ideal targets for cancer immunotherapy because they are foreign. Various versions of investigational HPV therapeutic vaccines have been in clinical trials for about the last 30 years, but none has been approved by the United States Food and Drug Administration. Investigational HPV therapeutic vaccines have been tested for many indications including clearance of HPV 16 and/or 18 infection (29), HSIL regression (11, 15, 16), prevention of recurrence of squamous cell carcinoma of head and neck (HNC)(NCT03821272), treatment of advanced stage cervical cancer (13, 14), and treatment of advanced stage HNC (30). The assessment of vaccine efficacy depends on the indication being tested. For HPV 16 infection clearance, HPV-DNA typing was used (29), and biopsies were utilized to evaluate HSIL regression (11, 15, 16). Lack of recurrence within a 2 year period is being used for assessing prevention of recurrence (NCT03821272). Antitumor efficacy was examined using the numbers of patients with complete and partial response, tumor shrinkage, duration of response (13).
Unlike the HPV prophylactic vaccines which work by inducing production of neutralizing antibodies (31, 32), the HPV therapeutic vaccines are believed to cast their effects through stimulation of cell-mediated immunity, mainly T cells. Therefore, assessments of T cell immune response should be included in the endpoints of clinical trials. Such implementation varies widely among the clinical trials because T cell assays are technically challenging. In a Phase I clinical trial, Maciag et al. (14) examined the safety of Lm-LLO-E7 vaccine which is a live-attenuated Listeria monocytogenes engineered to secrete HPV 16 E7 protein fused with a Listeria monocytogenes protein listeriolysin. Fifteen patients with invasive carcinoma of the cervix were enrolled. In order to demonstrate immune responses, the investigators attempted to perform IFN-γ ELISPOT assay using pooled peptides, but most samples were not suitable due to low yield and viability after thawing. Of the 3 patients having a sufficient number of cells available to perform the assay, only one demonstrated an HPV-specific T cell response after vaccination. HPV 16 E7 short and long peptides were pooled before testing, so no information as to which portion of the protein contained immunogenic epitopes was obtained (14). In the GTL001 trial, van Damme et al. performed ex vivo IFN-γ ELISPOT assay with pooled HPV 16 E7 peptides or HPV 18 E7 peptides. GTL001 was made of recombinant HPV 16 and HPV 18 E7 proteins which were fused with catalytically inactive CyaA protein of Bordetella pertussis. A total of 47 women with HPV 16 or HPV 18 infection were studied in 4 cohorts. Overall, 18 of 31 patients (58.1%) who received any dose of GTL001 with imiquimod demonstrated positive ELISPOT results to either protein (29). Trimble et al. also tested immune responses using IFN-γ ELISPOT assay and intracellular cytokine staining for assessment of T cell immunity. Significantly higher responses were reported for patients with HSIL who received the VGX-3100 vaccine (synthetic DNA designed to express HPV 16 and 18 E6 and E7 proteins) compared to those who received placebo. As peptides were pooled for each protein tested (HPV 16 E6, HPV 16 E7, HPV 18 E6, and HPV 18 E7), information on which portion of the protein contained the immunogenic epitopes was not determined (11). In the clinical trial which treated advanced-stage HNC patients with ISA101 (a synthetic long-peptide HPV 16 vaccine) and nivolumab (an anti-PD-1 checkpoint inhibitor), the investigators performed IFN-γ ELISPOT assay for HPV 16 E6 and E7 again using peptide pools. Variable increases in the number of HPV-specific T cells were observed after vaccination in both responders and nonresponders, making the role of vaccine-induced T cells uncertain. Furthermore, the immune response did not correlate with efficacy endpoints (30). In addition to IFN-γ ELISPOT assay, Melief et al. performed lymphocyte stimulation test, intracellular cytokine staining, and cytometric bead arrays to assess immune responses for a clinical trial studying the effects of ISA101 vaccination during chemotherapy in patients with advanced, recurrent, or metastatic cervical cancer (13). In all 64 patients who received ISA101 vaccination, HPV 16 E6 and/or E7-specific T cell responses to one or more of 6 peptide pools (4 pools for HPV 16 E6 and 2 pools for HPV 16 7 protein) were demonstrated.
Our IFN-γ ELISPOT protocol distinguishes itself among others in that we tested for 10 HPV 16 E6 peptides pools and 6 HPV 16 E7 peptide pools (Figure 1B) (15, 16). Therefore, the locations of the antigenic epitopes can be narrowed down to 25 amino acid regions. This characteristic of our ELISPOT assay was key to identifying a significant response to the HPV 16 E6 91-115 region, and subsequent isolation of antigen-specific T cells based on IFN-γ secretion. In this clinical trial, 61% (19 of 31) of vaccine recipients demonstrated a new CD3+ T cell response in ELISPOT assay using peripheral blood sample to at least one region of the HPV 16 E6 protein which was not present prior to vaccination (15, 16). Furthermore, these increased responses were statistically significant in 42% (13 of 31) of the subjects when ELISPOT results after vaccination were compared to those prior to vaccination (15, 16). However, the presence of such peripheral T cell responses to HPV after vaccination did not correlate with cervical HSIL regression. Therefore, ELISPOT data alone may not be a good correlate of clinical response, and that they should be combined with advanced technologies such as TCR sequencing for more critical evaluation of local protection at the site of lesion.
It is intriguing that our prior work demonstrated the HPV 16 E6 91-115 region as one of areas in which significantly increased CD4+ T cell responses were demonstrated among regressors of cervical lesions compared to persistors (33). The single-cell RNA-seq data in the current work suggest that the HPV-specific T cells are CD8+ T cells. These data combined raises a possibility that the HPV 16 E6 91-115 region may be an epitope hotspot just like the HPV 16 E6 52-62 regions which we described to be a T cell eptiope hotspot containing both CD4 and CD8 epitopes (34).
TCRs are highly diverse heterodimers consisting of α and β chains in the majority of T cells. However, 1-5% of T cells express γδ chains (35). Similar to B cell receptors, the TCR chains contains a variable region responsible for antigen recognition, and a constant region. The variable region of the α and δ chains is encoded by recombined variable (V) and joining (J) genes. Additionally for the β and γ chains, diversity (D) genes are also recombined (i.e., VDJ recombination). Therefore, the β and γ chains are more diverse than the α and δ chains. The advent of high-throughput sequencing made it possible to probe into the complexity of such TCRs. In the current study, we employed TCR β chain deep sequencing using bulk DNA and single-cell RNA-based TCR analysis using mRNA. The former has the advantage of using DNA, which can be extracted from LBC and FFPE samples; therefore, live cells are not necessary. The latter was utilized to analyze IFN-γ secreting HPV 16 E6 91-115 specific T cells from monocyte-deprived PBMCs after 4 vaccinations. Information on TCR α and β sequences and their pairings was obtained, and the gene expression profiles of individual cells was examined. We demonstrated that using the information from a traditional IFN-γ ELISPOT assay in combination with TCR sequencing enables us to demonstrate the expansion of HPV-specific CD3+ T cells and their presence in the cervix. In addition to demonstrating the information on TCR α and β chain pairings, the single-cell RNA-based method has the advantage of yielding the entire sequences of the α and β chains. This would enable construction of the TCRs in viral vectors with which their specificities can be verified (36, 37). Furthermore, such engineered T cells can be used for immunotherapy as demonstrated by Draper and colleagues (38). They used T cells genetically engineered to express the TCR of HPV 16 E6 29-38 (TIHDIILECV) epitope restricted by HLA-A*02:01. These engineered T cells were shown to be cytotoxic to HPV 16-positive cervical and HNC cell lines (38). The limitation of our current study was that we only examined one subject in this proof-of-concept study. As the Phase II clinical trial of PepCan is ongoing (NCT02481414), additional analyses of Phase II participants would aid in determining the generalizability of the findings of this study. As the participants are being randomized in a blinded fashion to PepCan arm and adjuvant only arm, making comparisons between these two arms as well as between responders and non-responders would be possible.
This open-label single center dose-escalation Phase I clinical trial of PepCan was reported previously (15, 16). Subject 6 was selected for the current study because she was a vaccine responder, and sufficient amounts of her samples were available for further analyses. Briefly, subjects qualified for vaccination if they had biopsy-proven CIN 2 and/or CIN 3 (Figure 1). PepCan (subject 6 received 50 μg/peptide dose) was given 4 times 3 weeks apart, and LEEP was performed 12 weeks after the last vaccination. Cervical LBC samples (ThinPrep, Hologic, Marborough, MA) were collected for HPV typing before vaccination at the time of qualifying biopsy, and after 4 vaccinations at the time of LEEP. Blood was drawn before vaccination, after 2 vaccinations, and after 4 vaccinations to isolate PBMCs. Routine clinical laboratory tests (complete blood count, sodium, potassium, chloride, carbon dioxide, blood urea nitrogen, creatinine, aspartate transaminase, alanine transaminase, lactate dehydrogenase, γ-glutamyl transpeptidase, total bilirubin, and direct bilirubin) were performed. PBMCs were isolated using the ficoll density gradient method. Cells were stored in liquid nitrogen tanks while LBC samples were kept in - 80°C freezers. Cervical FFPE samples were stored at room temperature.
Research laboratory analyses performed (15, 16) as a part of the clinical trial included HPV typing (Linear Array HPV Genotyping Test, Roche Molecular Diagnostics, Pleasanton, CA), IFN-γ ELISPOT assay, fluorescent-activated cell sorter analysis of peripheral Th1, Th2, and Treg cells, and HLA class I and class II low-resolution typing (One Lambda, West Hills, CA). The Linear Array HPV Genotyping Test detects 37 individual HPV types (6, 11, 16, 18, 26, 31, 33, 35, 39, 40, 42, 45, 51, 52, 53, 54, 55, 56, 58, 59, 61, 62, 64, 66, 67, 68, 69, 70, 71, 72, 73, 81, 82, 83, 84, IS 39, and CP6108). For the ELISPOT assay, magnetically selected CD3+ T cells (Pan T Cell Isolation Kit, Miltenyi Biotec, Auburn CA) were stimulated with autologous monocyte-derived dendritic cells pulsed with HPV 16 E6 or E7 using recombinant vaccinia viruses (39) and recombinant GST fusion proteins (39) twice with a one-week duration for each stimulation (15, 16). The assay was performed in triplicates using overlapping HPV 16 E6 and E7 peptide pools covering HPV 16 E6 1-25, 16-40, 31-55, 45-70, 61-85, 76-100, 91-115, 106-130, 121-145, 136-158 and HPV 16 E7 16-40, 31-55, 46-70, 61-85, and 76-98 regions. Each peptide pool contained three peptides which were 15 amino acids in length with 10 amino acid overlap. PBMCs were stained for CD4+, CD25+, T-bet, GATA3, and Foxp3 (15, 16). The percentage of CD4+ cells positive for T-bet represented Th1 cells, those positive for GATA3 represented Th2 cells, and those positive for CD25+ and FoxP3 represented Tregs.
The TCR β CDR3 regions were PCR-amplified and sequenced (immunoSEQ, Adaptive Biotechnologies, Seattle, WA) (40) using genomic DNA from PBMCs (pre-, post-2, and post-4), CD3+ T cells stimulated with HPV 16 E6 and E7 expressed by recombinant vaccinia viruses and in a form of GST-proteins (pre-, post-2, and post-4), LBC (pre- and post-4), and FFPE (pre- and post-4). Using bias-controlled V and J gene primers, the rearranged V(D)J segments were amplified and sequenced. A clustering algorithm was used to correct for sequencing errors, and the CDR3 segments were annotated according to the International ImMunoGeneTicsCollaboration (41, 42) to identify the V, D, and J genes that contributed to each rearrangement. A mixture of synthetic TCR analogs was used in PCR to estimate the number of cells bearing each unique TCR sequence (43). “Detailed rearrangements”, “Track Rearrangements”, “Venn Diagram”, “Differential Abundance”, and “Scatterplot with Annotation” features of the immunoSeq analyzer (44) were used to analyze data.
In order to obtain TCR Vα and Vβ sequences of T cells specific for HPV 16 E6 91-115 (Figure 1B), such T cells were selected using a human IFN-γ Secretion Assay – Cell Enrichment and Detection Kit (Miltenyi Biotec) following the manufacturer’s instructions as previously described (34, 45–48). Post-4 vaccination PBMC sample cryopreserved after monocyte depletion (CD14 MicroBeads, Miltenyi Biotec) was thawed and cultured overnight in Yssel’s media (Gemini Bio Products, West Sacramento, CA) with 1% human serum and 1,200 IU/mL of recombinant human interleukin-2 (R&D Systems, Inc., Minneapolis, MN). As a positive control, healthy donor PBMCs mixed with 1% HPV 16 E6 52-61 (FAFRDLCIVY)-specific CD8+ T cell clone cells (46) were processed in the same manner. The cells were stimulated for 3 h with 10 μM each of peptides in RPMI1640 media plus 5% human serum: FAFRDLCIVY for the positive control, and the three 15-mer overlapping peptides covering the HPV 16 E6 91-115 region (91-105, YGTTLEQQYNKPLCD; 96-110, EQQYNKPLCDLLIRC; 101-115, KPLCDLLIRCINCQK; RS Synthesis, Louiville, KY; ≥ 70% purity) (49). IFN-γ secreting cells were labeled using the IFN-γ catch reagent and phycoerythrin (PE)-labeled IFN-γ detection antibody. The positive control sample and healthy donor PBMCs stained with mouse IgG1K isotype labeled with PE (eBiosciences) were used as a negative control to set the gate. The cells were sorted for IFN-γ positivity only using FACS Aria (BD Biosciences, Franklin Lakes, New Jersey).
A Next GEM Chip G was loaded with approximately 10,000 cells and Chromium Next GEM Single Cell 5’ Library Gel Bead Kit v1.1 reagent (10X Genomics, Pleasanton, CA). An emulsion was generated with the Chromium Controller (10X Genomics). Gene expression (GEX) libraries were prepared with the Chromium Single Cell 5’ Library Construction Kit and TCR libraries were prepared with the Chromium Single Cell V(D)J Enrichment Kit, Human T Cell (10X Genomics). A low-pass surveillance sequencing run of both libraries were performed on separate Illumina mid-output MiniSeq flow cells (GEX library Read1:26bp, Read2:91bp, TCR library Read1:150bp, Read2:150bp). Sequencing was scaled up on an Illumina NextSeq 500 with a high-output 150-cycle v2.5 kit for the GEX library and a mid-output 300-cycle v2.5 kit for the TCR library; both runs used identical read lengths as on the MiniSeq. Data was aggregated from both runs.
Sequencing data were first processed by a Cell Ranger pipeline (v3.1.0; 10X Genomics). Gene expression sequencing data were mapped to human reference (GRCh38-3.0.0) dataset. The raw single-cell data were processed by R package Seurat v. 3.2.2, by following the recommended steps and settings. The low-quality cells and doublets were filtered out by the following recommended setting: percentage of mitochondrial genes > 5%, number of detected genes < 200 and number of detected genes > 2500. The clustering was performed with the resolution setting at 0.4. The UMAP (Uniform Manifold Approximation and Projection) plot, violin plots and feature plots were also generated by Seurat (Figure 4).
TCR sequencing data were mapped to human TCR reference (GRCh38-alts-ensembl-3.1.0) dataset, and they were further analyzed by Loupe V(D)J Browser (v3.0.0; 10X Genomics). T cell clonotypes were defined based on TCR Vβ CDR3 nucleotide sequences after removing single cells containing only α chains and those containing two different TCR Vβ CDR3 nucleotide sequences (likely doublets). For calculating the frequencies of ≥ 5% clonotypes (Table 3), clonotypes with two or more single cells were included. Full-length TCRα β amino acid sequences were obtained by the Loupe V(D)J Browser.
A paired t-test was performed to assess the significant changing of spot forming units (i.e., IFN-γ secreting cells) before and after vaccination in ELISPOT assay. The number of T cells between study visits in PBMC, stimulated CD3+ T cells, LBC, and FFPE were compared using Wilcoxon matched-pairs signed-ranks test (GraphPad Instat 3, GraphPad Software, San Diego, CA). A p value < 0.05 was considered statistically significant.
The datasets presented in this study can be found in online repositories. The names of the repository/repositories and accession number(s) can be found below: https://doi.org/10.21417/TS2020HPV, immuneACCESS, and https://www.ncbi.nlm.nih.gov/geo/query/acc.cgi?=GSE184703, National Center for Biotechnology Information’s Gene Expression Omnibus (28).
The studies involving human participants were reviewed and approved by University of Arkansas for Medical Sciences Institutional Review Board. The patients/participants provided their written informed consent to participate in this study.
MN, DJ, ToS, and Y-CL developed the concepts and designed this project. TaS, SS, TE, OS, CQ, HC, and MN performed the experiments. TaS, BL, and MN wrote the manuscript, and all authors edited it. TaS, SS, HS, EP, and MN performed statistical and bioinformatics analyses. All authors contributed to the article and approved the submitted version.
This work was supported by the grant from the National Institutes of Health (R01CA143130, USA), Drs. Mae and Anderson Nettleship Endowed Chair of Oncologic Pathology (31005156, USA), and the Arkansas Biosciences Institute (the major component of the Tobacco Settlement Proceeds Act of 2000, AWD00053655, USA).
The contributions of BL to this study were completed in their entirety while he was affiliated with the University of Arkansas for Medical Sciences, and he now is employed by Adaptive Biotechnologies (Seattle, WA, USA). This manuscript was submitted for publication prior to his employment with Adaptive Biotechnologies.
MN is one of the inventors named in the patents and patent applications for the HPV therapeutic vaccine (PepCan).
The remaining authors declare that the research was conducted in the absence of any commercial or financial relationships that could be construed as a potential conflict of interest.
All claims expressed in this article are solely those of the authors and do not necessarily represent those of their affiliated organizations, or those of the publisher, the editors and the reviewers. Any product that may be evaluated in this article, or claim that may be made by its manufacturer, is not guaranteed or endorsed by the publisher.
The Supplementary Material for this article can be found online at: https://www.frontiersin.org/articles/10.3389/fimmu.2021.645299/full#supplementary-material
CIN 3, cervical intraepithelial neoplasia 3; cGMP, current good manufacturing practice; ELISPOT, enzyme-linked imunospot; FFPE, formalin-fixed paraffin-embedded; HNC, head and neck cancer; HPV, human papillomavirus; HPV 16, human papillomavirus type 16; HSIL, high-grade squamous intraepithelial lesion; IFN-γ, interferon-γ; IL-4, interleukin-4; LBC, liquid-based cytology; LEEP, loop electrical excision procedure; PBMCs, peripheral blood mononuclear cells; TCR, T cell receptor; Th1, T-helper type 1; TNF, tumor necrosis factor.
1. HPV-Associated Cancer Statistics Vol. 2019. Atlanta, GA: Centers for Disease Control and Prevention (2019).
3. de Martel C, Plummer M, Vignat J, Franceschi S. Worldwide Burden of Cancer Attributable to HPV by Site, Country and HPV Type. Int J Cancer (2017) 141:664–70. doi: 10.1002/ijc.30716
4. Massad LS, Einstein MH, Huh WK, Katki HA, Kinney WK, Shiffman M, et al. Updated Consensus Guidelines for the Management of Abnormal Cervical Cancer Screening Tests and Cancer Precursors. Obstet Gynecol (2013) 121:829–46. doi: 10.1097/AOG.0b013e3182883a34
5. Bruinsma FJ, Quinn MA. The Risk of Preterm Birth Following Treatment for Precancerous Changes in the Cervix: A Systematic Review and Meta-Analysis. BJOG (2011) 118:1031–41. doi: 10.1111/j.1471-0528.2011.02944.x
6. Spandorfer SD, Bongiovanni AM, Fasioulotis S, Resenwaks Z, Ledger WJ, Witkin SS, et al. Prevalence of Cervical Human Papillomavirus in Women Undergoing In Vitro Fertilization and Association With Outcome. Fertil Steril (2006) 86:765–7. doi: 10.1016/j.fertnstert.2006.01.051
7. Depuydt CE, Verstraete L, Berth M, Beert J, Bogers JP, Salembier G, et al. Human Papillomavirus Positivity in Women Undergoing Intrauterine Insemination Has a Negative Effect on Pregnancy Rates. Gynecol Obstet Invest (2016) 81:41–6. doi: 10.1159/000434749
8. Hermonat PL, Han L, Wendel PJ, Quirk JG, Stern S, Lowery CL, et al. Human Papillomavirus Is More Prevalent in First Trimester Spontaneously Aborted Products of Conception Compared to Elective Specimens. Virus Genes (1997) 14:13–7. doi: 10.1023/A:1007975005433
9. Garolla A, Engl B, Pizzol D, Ghezzi M, Bertoldo A, Bottacin A, et al. Spontaneous Fertility and In Vitro Fertilization Outcome: New Evidence of Human Papillomavirus Sperm Infection. Fertil Steril (2016) 105:65–72.e61. doi: 10.1016/j.fertnstert.2015.09.018
10. Garolla A, Pizzol D, Foresta C. The Role of Human Papillomavirus on Sperm Function. Curr Opin Obstet Gynecol (2011) 23:232–7. doi: 10.1097/GCO.0b013e328348a3a4
11. Trimble CL, Morrow MP, Kraynyak KA, Shen X, Dallas M, Yan J, et al. Safety, Efficacy, and Immunogenicity of VGX-3100, A Therapeutic Synthetic DNA Vaccine Targeting Human Papillomavirus 16 and 18 E6 and E7 Proteins for Cervical Intraepithelial Neoplasia 2/3: A Randomised, Double-Blind, Placebo-Controlled Phase 2b Trial. Lancet (2015) 386(10008):2078–88. doi: 10.1016/S0140-6736(15)00239-1
12. Kenter GG, Welters MJ, Valentijin AR, Lowik MJ, Berends-van der Meer DM, Vloon AP, et al. Vaccination Against HPV-16 Oncoproteins for Vulvar Intraepithelial Neoplasia. N Engl J Med (2009) 361:1838–47. doi: 10.1056/NEJMoa0810097
13. Melief CJM, Welters MJP, Vergote I, Kroep JR, Kenter GG, Ottevanger PB, et al. Strong Vaccine Responses During Chemotherapy Are Associated With Prolonged Cancer Survival. Sci Transl Med (2020) 12. doi: 10.1126/scitranslmed.aaz8235
14. Maciag PC, Radulovic S, Rothman J. The First Clinical Use of a Live-Attenuated Listeria Monocytogenes Vaccine: A Phase I Safety Study of Lm-LLO-E7 in Patients With Advanced Carcinoma of the Cervix. Vaccine (2009) 27:3975–83. doi: 10.1016/j.vaccine.2009.04.041
15. Coleman HN, Greenfield WW, Stratton SL, Vaughn R, Kieber A, Moerman-Herzog AM, et al. Human Papillomavirus Type 16 Viral Load Is Decreased Following a Therapeutic Vaccination. Cancer Immunol Immunother (2016) 65:563–73. doi: 10.1007/s00262-016-1821-x
16. Greenfield WW, Stratton SL, Myrick RS, Vaughn R, Donnalley LM, Coleman HN, et al. A Phase I Dose-Escalation Clinical Trial of a Peptide-Based Human Papillomavirus Therapeutic Vaccine With Skin Test Reagent as a Novel Vaccine Adjuvant for Treating Women With Biopsy-Proven Cervical Intraepithelial Neoplasia 2/3. Oncoimmunology (2015) 4:e1031439. doi: 10.1080/2162402X.2015.1031439
17. Nieminen P, Harper DM, Einstein MH, Garcia F, Donders G, Huh W, et al. Efficacy and Safety of RO5217990 Treatment in Patients With High Grade Cervical Intraepithelial Neoplasia (CIN2/3). In: 28th International Papillomavirus Conference. Puerto Rico (2012).
18. Cui JH, Lin KR, Yuan SH, Jin YB, Chen XP, Su XK, et al. TCR Repertoire as a Novel Indicator for Immune Monitoring and Prognosis Assessment of Patients With Cervical Cancer. Front Immunol (2018) 9:2729. doi: 10.3389/fimmu.2018.02729
19. Lang Kuhs KA, Lin SW, Hua X, Schiffman M, Burk RD, Rodriguez AC, et al. T Cell Receptor Repertoire Among Women Who Cleared and Failed to Clear Cervical Human Papillomavirus Infection: An Exploratory Proof-of-Principle Study. PloS One (2018) 13:e0178167. doi: 10.1371/journal.pone.0178167
20. Morrow MP, Kraynyak KA, Sylvester AJ, Shen X, Amante D, Sakata L, et al. Augmentation of Cellular and Humoral Immune Responses to HPV16 and HPV18 E6 and E7 Antigens by VGX-3100. Mol Ther Oncolytics (2016) 3:16025. doi: 10.1038/mto.2016.25
21. Laydon DJ, Bangham CR, Asquith B. Estimating T-Cell Repertoire Diversity: Limitations of Classical Estimators and a New Approach. Philos Trans R Soc Lond B Biol Sci (2015) 370:1–11. doi: 10.1098/rstb.2014.0291
22. Fink K. Can We Improve Vaccine Efficacy by Targeting T and B Cell Repertoire Convergence? Front Immunol (2019) 10:110. doi: 10.3389/fimmu.2019.00110
23. Hopkins AC, Yarchoan M, Durham JN, Yusko EC, Rytlewski JA, Robins HS, et al. T Cell Receptor Repertoire Features Associated With Survival in Immunotherapy-Treated Pancreatic Ductal Adenocarcinoma. JCI Insight (2018) 3. doi: 10.1172/jci.insight.122092
24. Inoue H, Park JH, Kiyotani K, Zewde M, Miyashita A, Jinnin M, et al. Intratumoral Expression Levels of PD-L1, GZMA, and HLA-A Along With Oligoclonal T Cell Expansion Associate With Response to Nivolumab in Metastatic Melanoma. Oncoimmunology (2016) 5:e1204507. doi: 10.1080/2162402X.2016.1204507
25. Tumeh PC, Harview CL, Yearley JH, Shintaku IP, Taylor EJ, Robert L, et al. PD-1 Blockade Induces Responses by Inhibiting Adaptive Immune Resistance. Nature (2014) 515:568–71. doi: 10.1038/nature13954
26. Rytlewski J, Deng S, Xie T, Davis C, Robins H, Yusko E, et al. Model to Improve Specificity for Identification of Clinically-Relevant Expanded T Cells in Peripheral Blood. PloS One (2019) 14:e0213684. doi: 10.1371/journal.pone.0213684
27. Durst M, Gissmann L, Ikenberg H, zur Hausen H. A Papillomavirus DNA From a Cervical Carcinoma and Its Prevalence in Cancer Biopsy Samples From Different Geographic Regions. Proc Natl Acad Sci USA (1983) 80:3812–5. doi: 10.1073/pnas.80.12.3812
28. Schiffman M, Doorbar J, Wentzensen N, de Sanjose S, Fakhry C, Monk BJ, et al. Carcinogenic Human Papillomavirus Infection. Nat Rev Dis Primers (2016) 2:16086. doi: 10.1038/nrdp.2016.86
29. Van Damme P, Bouillette-Marussig M, Hens A, De Coster I, Depuydt C, Goubier A, et al. GTL001, A Therapeutic Vaccine for Women Infected With Human Papillomavirus 16 or 18 and Normal Cervical Cytology: Results of a Phase I Clinical Trial. Clin Cancer Res (2016) 22:3238–48. doi: 10.1158/1078-0432.CCR-16-0085
30. Massarelli E, William W, Johnson F, Kies M, Ferrarotto R, Guo M, et al. Combining Immune Checkpoint Blockade and Tumor-Specific Vaccine for Patients With Incurable Human Papillomavirus 16-Related Cancer: A Phase 2 Clinical Trial. JAMA Oncol (2019) 5:67–73. doi: 10.1001/jamaoncol.2018.4051
31. Godi A, Panwar K, Haque M, Cocuzza CE, Andrews N, Southern J, et al. Durability of the Neutralizing Antibody Response to Vaccine and Non-Vaccine HPV Types 7 Years Following Immunization With Either Cervarix(R) or Gardasil(R) Vaccine. Vaccine (2019) 37:2455–62. doi: 10.1016/j.vaccine.2019.03.052
32. Sankaranarayanan R, Prabhu PR, Pawlita M, Gheit T, Bhatla N, Muwonge R, et al. Immunogenicity and HPV Infection After One, Two, and Three Doses of Quadrivalent HPV Vaccine in Girls in India: A Multicentre Prospective Cohort Study. Lancet Oncol (2016) 17:67–77. doi: 10.1016/S1470-2045(15)00414-3
33. Kim KH, Greenfield WW, Cannon MJ, Coleman HN, Spencer HJ, Nakagawa M. CD4+ T-Cell Response Against Human Papillomavirus Type 16 E6 Protein Is Associated With a Favorable Clinical Trend. Cancer Immunol Immunother (2012) 61:63–70. doi: 10.1007/s00262-011-1092-5
34. Coleman HA, Wang X, Greenfield WW, Nakagawa M. A Human Papillomavirus Type 16 E6 52-62 CD4 T-Cell Epitope Restricted by the HLA-DR11 Molecule Described in an Epitope Hotspot. MOJ Immunol (2014) 1:00018. doi: 10.15406/moji.2014.01.00018
35. Lo Presti E, Dieli F, Meraviglia S. Tumor-Infiltrating Gammadelta T Lymphocytes: Pathogenic Role, Clinical Significance, and Differential Programing in the Tumor Microenvironment. Front Immunol (2014) 5:607. doi: 10.3389/fimmu.2014.00607
36. Lu YC, Zheng Z, Robbins PF, Tran E, Prickett TD, Gartner JJ, et al. An Efficient Single-Cell RNA-Seq Approach to Identify Neoantigen-Specific T Cell Receptors. Mol Ther (2018) 26:379–89. doi: 10.1016/j.ymthe.2017.10.018
37. Morgan RA, Dudley ME, Wunderlich JR, Hughes MS, Yang JC, Sherry RM, et al. Cancer Regression in Patients After Transfer of Genetically Engineered Lymphocytes. Science (2006) 314:126–9. doi: 10.1126/science.1129003
38. Draper LM, Kwong ML, Gros A, Stevanovic S, Tran E, Kerkar S, et al. Targeting of HPV-16+ Epithelial Cancer Cells by TCR Gene Engineered T Cells Directed Against E6. Clin Cancer Res (2015) 21:4431–9. doi: 10.1158/1078-0432.CCR-14-3341
39. Nakagawa M, Stites DP, Farhat S, Sisler JR, Moss B, Kong F, et al. Cytotoxic T Lymphocyte Responses to E6 and E7 Proteins of Human Papillomavirus Type 16: Relationship to Cervical Intraepithelial Neoplasia. J Infect Dis (1997) 175:927–31. doi: 10.1086/513992
40. Robins HS, Campregher PV, Srivastava SK, Wacher A, Turtle CJ, Kahsai O, et al. Comprehensive Assessment of T-Cell Receptor Beta-Chain Diversity in Alphabeta T Cells. Blood (2009) 114:4099–107. doi: 10.1182/blood-2009-04-217604
41. Lefranc MP, Giudicelli V, Duroux P, Jabado-Michaloud J, Folch G, Aouinti S, et al. IMGT(R), the International ImMunoGeneTics Information System(R) 25 Years on. Nucleic Acids Res (2015) 43:D413–22. doi: 10.1093/nar/gku1056
42. Yousfi Monod M, Giudicelli V, Chaume D, Lefranc MP. IMGT/JunctionAnalysis: The First Tool for the Analysis of the Immunoglobulin and T Cell Receptor Complex V-J and V-D-J JUNCTIONs. Bioinformatics (2004) 20(Suppl 1):i379–85. doi: 10.1093/bioinformatics/bth945
43. Wu D, Emerson RO, Shwerwood A, Loh ML, Angiolillo A, Howie B, et al. Detection of Minimal Residual Disease in B Lymphoblastic Leukemia by High-Throughput Sequencing of IGH. Clin Cancer Res (2014) 20:4540–8. doi: 10.1158/1078-0432.CCR-13-3231
44. immunoSEQ Analyzer https://www.immunoseq.com/analyzer.
45. Nakagawa M, Kim KH, Gillam TM, Moscicki AB. HLA Class I Binding Promiscuity of the CD8 T-Cell Epitopes of Human Papillomavirus Type 16 E6 Protein. J Virol (2007) 81:1412–23. doi: 10.1128/JVI.01768-06
46. Nakagawa M, Kim KH, Moscicki AB. Different Methods of Identifying New Antigenic Epitopes of Human Papillomavirus Type 16 E6 and E7 Proteins. Clin Diagn Lab Immunol (2004) 11:889–96. doi: 10.1128/CDLI.11.5.889-896.2004
47. Wang X, Greenfield WW, Coleman HN, James LE, Nakagawa M. Use of Interferon-Gamma Enzyme-Linked Immunospot Assay to Characterize Novel T-Cell Epitopes of Human Papillomavirus. J Vis Exp (2012) 61:1–8. doi: 10.3791/3657
48. Wang X, Santin AD, Bellone S, Gupta S, Nakagawa M. A Novel CD4 T-Cell Epitope Described From One of the Cervical Cancer Patients Vaccinated With HPV 16 or 18 E7-Pulsed Dendritic Cells. Cancer Immunol Immunother (2009) 58:301–8. doi: 10.1007/s00262-008-0525-2
Keywords: cervical intraepithelial neoplasia, human papillomavirus, T cell receptor, therapeutic vaccine, clonal expansion
Citation: Shibata T, Shah S, Evans T, Coleman H, Lieblong BJ, Spencer HJ, Quick CM, Sasagawa T, Stephens OW, Peterson E, Johann D Jr., Lu Y-C and Nakagawa M (2021) Expansion of Human Papillomavirus-Specific T Cells in Periphery and Cervix in a Therapeutic Vaccine Recipient Whose Cervical High-Grade Squamous Intraepithelial Lesion Regressed. Front. Immunol. 12:645299. doi: 10.3389/fimmu.2021.645299
Received: 22 December 2020; Accepted: 31 August 2021;
Published: 30 September 2021.
Edited by:
Min Gong, Tianjin Medical University, ChinaReviewed by:
Maria Issagouliantis, Riga Stradiņš University, LatviaCopyright © 2021 Shibata, Shah, Evans, Coleman, Lieblong, Spencer, Quick, Sasagawa, Stephens, Peterson, Johann, Lu and Nakagawa. This is an open-access article distributed under the terms of the Creative Commons Attribution License (CC BY). The use, distribution or reproduction in other forums is permitted, provided the original author(s) and the copyright owner(s) are credited and that the original publication in this journal is cited, in accordance with accepted academic practice. No use, distribution or reproduction is permitted which does not comply with these terms.
*Correspondence: Mayumi Nakagawa, bW5ha2FnYXdhQHVhbXMuZWR1
Disclaimer: All claims expressed in this article are solely those of the authors and do not necessarily represent those of their affiliated organizations, or those of the publisher, the editors and the reviewers. Any product that may be evaluated in this article or claim that may be made by its manufacturer is not guaranteed or endorsed by the publisher.
Research integrity at Frontiers
Learn more about the work of our research integrity team to safeguard the quality of each article we publish.