- Immunology Research Area, IRCCS Bambino Gesù Children's Hospital, Rome, Italy
Myeloid derived suppressor cells (MDSC) are heterogeneous populations that through the release of soluble factors and/or by cell-to-cell interactions suppress both innate and adaptive immune effector cells. In pathological conditions, characterized by the presence of inflammation, a partial block in the differentiation potential of myeloid precursors causes an accumulation of these immunosuppressive cell subsets both in peripheral blood and in tissues. On the contrary, NK cells represent a major player of innate immunity able to counteract tumor growth. The anti-tumor activity of NK cells is primarily related to their cytolytic potential and to the secretion of soluble factors or cytokines that may act on tumors either directly or indirectly upon the recruitment of other cell types. NK cells have been shown to play a fundamental role in haploidentical hemopoietic stem cell transplantation (HSCT), for the therapy of high-risk leukemias. A deeper analysis of MDSC functional effects demonstrated that these cells are capable, through several mechanisms, to reduce the potent GvL activity exerted by NK cells. It is conceivable that, in this transplantation setting, the MDSC-removal or -inactivation may represent a promising strategy to restore the anti-leukemia effect mediated by NK cells. Thus, a better knowledge of the cellular interactions occurring in the tumor microenvironment could promote the development of novel therapeutic strategies for the treatment of solid and hematological malignances.
Introduction
Tumor microenvironment (TME) consists of an assortment of tumor and non-tumor cells (including mesenchymal stromal cells, endothelial cells, regulatory T-cells, and myeloid-derived suppressor cells), as well as soluble components. Tumor associated (TA)-cells may favor neoplastic transformation, tumor growth, and metastasis thus contributing to tumor escape from host immunity. In addition to TA-cells, TME also contains immune cells including innate and adaptive lymphocytes. A growing body of evidences has revealed the existence of a close relationship between tumor and immune components. Many of the interactions between TME and tumor infiltrating (TI) immune cells are already well-known (1). Consistent positive prognostic correlations have been reported for T-cells, especially cytotoxic T-cells, in different tumor types (2). In particular, TI-lymphocyte density can influence prognosis within each tumor, lymph node, and metastasis (TNM) stage, complementing or even outperforming pathological criteria alone, as shown in colorectal and lung cancers (3–5). In order to develop strategies to overcome immunosuppression and tumor escape it is important to further unravel the cellular interactions occurring in TME. This contribution is focalized on the polymorphonuclear (PMN)-MDSC, an important, strongly immunosuppressive myeloid component, which may greatly impair the anti-tumor defenses in particular those mediated by NK cells.
MDSC in Physiological and Pathological Condition
One important cellular subset present in TME is represented by MDSC. These cells were first identified in 1970 as a heterogeneous group of immune cells with immature features derived from a common myeloid progenitor (CMP) (6). They possess high immunosuppressive and pro-tumorigenic capabilities and actively cooperate with other myeloid regulatory cells as tumor-associated neutrophils (TANs), tumor-associated macrophages (TAMs), and regulatory dendritic cells to favor cancer development (7). MDSC are usually classified in two or three classes in mice and humans, respectively. In mice, MDSC are classified into monocytic (M-MDSC) (CD11b+Ly6ChiLy6G−) and PMN-MDSC (CD11b+Ly6CloLy6G+ cells) subsets. In humans, the granulocytic and the monocytic subsets are classified as Lin−(CD3, CD19, CD56), CD11b+CD33+CD15+CD66b+CD14−HLA-DRlow/− and Lin−CD11b+CD33+CD15−CD14+HLA-DRlow/−cells, respectively. A new subset of immature and early-stage MDSC (e-MDSC) has been found in humans and classified as Lin− HLA-DRlow/− CD11b+ CD14− CD15− CD33+. Recently, the lectin-type oxidized LDL receptor-1 (LOX-1) molecule has been suggested as novel marker to discriminate MDSC from neutrophils (8–10).
In physiological condition, the CMP differentiate into neutrophils or monocytes. In pathological conditions such as cancer or infection the inflammatory milieu (e.g., GM-CSF, TNF-α, VEGF, and PGE2) induces the CMP differentiation into M-MDSC, and subsequently TAM, or PMN-MDSC that can differentiate into TAN. In addition, chronic inflammatory conditions induce release of soluble mediators that are responsible for MDSC accumulation due to their reduced susceptibility to Fas-mediated apoptosis (11). In this setting, MDSC can help to control excessive inflammation, by reducing both innate and adaptive immune responses (12, 13), while their reduction normally occurs following inflammation resolution. Conversely, if MDSC numbers do not decrease, they can be associated to disease progression. Indeed, a continuous inhibitory effect of immune response can interfere with tissue homeostasis, energy metabolism, and dead cell removal.
In cancer, MDSC activity is mainly regulated by three key events: myelopoiesis impairment, MDSC migration to tumor site and subsequent activation. Thus, deregulated myelopoiesis leads to accumulation of immature MDSC in bone marrow that are subsequently recruited to primary and metastatic tumor sites by tumor-released chemokines. Due to their potent immunosuppressive and pro-tumorigenic potential, high levels of MDSC, especially PMN-MDSC, have been observed in high grade cancers and are correlated with poor prognosis, treatment resistance and reduced overall survival in solid cancers (14–18). Breast, ovarian and gastric cancer cells secrete CCL2 that recruits MDSC and sustains tumor growth (19). In addition, CCR2+MDSC can support tumor growth in colon-rectal-cancer (CRC)-bearing mice (20). CXCL1 is another cytokine, highly expressed in CRC that exerts chemoattractant activity on CXCR2+MDSC. Moreover, MDSC also express CCR5 that has been suggested to be involved in MDSC migration to tumor site (21, 22). Regarding MDSC recruitment, it has been demonstrated that the hematopoietic 5-lipoxygenase (5LO), a metabolite of the arachidonic acid implied in colon carcinogenesis, is involved into MDSC migration (23). In addition, MDSC can regulate and induce their recruitment by a positive feedback. Indeed, Reactive Nitrogen Species produced by MDSC lead to chemokine nitration that in turn recruit MDSC (24).
Following recruitment, MDSC are activated through many mechanisms. In particular, PGE2 can induce STAT3 phosphorylation that mediates both MDSC activation (25) and inhibition of their physiological differentiation toward neutrophils or monocytes (26, 27). Histamine can modulate the expression of Arginase-1 (ARG-1) and Nitric oxide synthase (iNOS) in M-MDSCs and PMN-MDSC, respectively (28, 29). Hypoxia can induce the Hypoxia-inducible Factor 1 alpha (HIF-1α) that in turn increases ARG-1 and iNOS activation in MDSC (30).
While many studies on the involvement of MDSC in hematological disorders have been performed, their actual role is still debated. High numbers of PMN-MDSC were reported in chronic myeloid leukemia (CML), possibly playing a role in CML cell immune escape (31–33). The increased numbers of PMN-MDSC, evaluated at diagnosis of CML, return to normal levels after treatment with Imatinib. In acute leukemia, the role of MDSC is not fully defined. Patients with acute myeloid leukemia (AML) display increased numbers of MDSC in PB and BM as compared to patients with acute lymphoblastic leukemia (ALL) and a significant correlation exists with conventional prognostic factors at diagnosis (34). Moreover, a significant decrease of MDSC was observed only in those patients in complete remission after treatment. In pediatric patients, the frequency and the strength of the immunosuppressive function correlated with classical prognostic markers such as MRD and CD20+ blast cell counts and with response to therapy. In addition, patients in remission have been reported to lose MDSC suppressive function further corroborating the effect of these cells in favoring immune evasion mechanisms (35, 36). In diffuse large B-cell lymphoma (37), indolent lymphoma (38), chronic lymphocytic leukemia (39), and Hodgkin lymphoma (40), the frequency of circulating MDSC has been correlated with poor prognosis. Recent studies, in the S100A9 knockout transgenic mice, revealed that MDSC are also involved in the pathogenesis and progression of Multiple myeloma (MM) (41). MDSC isolated from the PB of patients with MM display an inhibitory effect on T-cells which could be abrogated by drugs inhibiting arginase-1 and iNOS activity (42). Available data would suggest that MDSC represent a sizable subset present in MM patients that may play a role in the pathophysiology of the disease by favoring survival and proliferation of malignant plasma cells as a consequence of their suppressive activity on anti-tumor immune response. Finally, recent evidence suggests that MDSC have also a predominant role in the pathophysiology of Immune Thrombocytopenia and Chronic Idiopathic Neutropenia (43–45).
Immunosuppressive Mechanisms Exerted by MDSC
Several studies described different mechanisms adopted by MDSC to exert their immunomodulatory function either by mechanisms that require cell-to-cell contact or by the release of soluble factors. They can directly inhibit the innate or adaptive immune system or indirectly contribute to tumor progression through regulation of angiogenesis or cell motility. In particular, MDSC-derived Nitric oxide (NO) can suppress proliferation of T-cells by inhibiting the Jak/STAT5 pathway and inducing T-cell apoptosis (46). MDSC-derived NO can also impair T-cell migration by reducing E-selectin expression on endothelial cells (47). In addition, oxygen reactive species (ROS) produced by MDSC can induce apoptosis in T-cells by decreasing expression of the T-cell receptor (TCR) ζ-chain (48, 49). Immunosuppression of T-cell response by MDSC may be accomplished through cleavage of L-selectin on CD4+ and CD8+ T-cells by ADAM metallopeptidase domain 17 (ADAM17) and disintegrin thus impairing T-cell trafficking to tumor sites (50). Moreover, via the ARG-1 or IDO enzymes, MDSC can deprive TME of the amminoacids required by T-cells for proliferation (51–53). Secretion of IL-10 and TGF-β by MDSC represents another mechanism to induce immunosuppression trough Treg induction (54). In lung cancer, IL-10 secreted by M-MDSC has been reported to be in part responsible for Treg induction in vitro (55).
Notably, TGF-β and IL-10 can also mediate immunosuppression indirectly, by inducing CD39 and CD73 expression on MDSC that are receptors involved in ATP/ADP hydrolysis and AMP cleavage, respectively, therefore MDSC affect T and NK cell response also by interfering with the adenosine metabolism (56, 57).
Huang B and colleagues showed that IFN-γ secreted by T-cells leads MDSC to release IL-10 and TGF-β that in turn induce Treg (54). In addition to IL-10 and TGF-β, it has been demonstrated that cell-to-cell contact and the CD40 expression on MDSC surface are also required for Treg expansion (58). Contact-dependent mechanism has been demonstrated also in hepatocellular carcinoma where MDSC induce Treg expansion (59). Different factors present in TME (transmembrane TNF-α, TGF-β, lipopolysaccharide, Semaphorin 4D, NKG2D ligands and extracellular vesicles) and hypoxia, can upregulate the secretion of IL-10 by MDSC (60–63). In addition, other factor such as HIF-1α increases the immunosuppressive activity of MDSC by inducing Programmed Cell Death 1 (PD-1) expression and by upregulating the V-domain of Ig suppressor of T-cell activation (VISTA) (64, 65).
Notably, angiogenesis represents another immunosuppressive mechanisms used by MDSC and it is mediated by VEGF upregulation. It has been demonstrated that MDSC, previously activated with VEGF, have a more potent inhibitory activity (66). MDSC can also secrete proangiogenic factors as Metalloproteases (MMP2, MMP8, MMP9, MMP13, and MMP14) that can disrupt the extracellular matrix thus facilitating the extravasation (67).
Another mechanism able to induce immunosuppression is represented by the release of protumorigenic mediators such as S100A8/A9 by MDSC and tumor cells. These factors are capable to induce M2-macrophage polarization and MDSC chemotaxis in TME that results in immunosuppression of effector cells (68, 69).
NK Cells in Tumors
Natural killer (NK) cells belong to the innate lymphoid cell (ILC) family. ILCs have recently been classified into five different subsets: NK cells that represent killer ILC, and ILC1, ILC2 ILC3, and Lymphoid tissue-inducer cells (LTi) that belong to helper-ILC. Unlike NK cells, the other ILC subpopulations were discovered only recently because they are relatively infrequent and are prevalently located in mucosal tissues and secondary lymphoid organs (70).
NK cells are present primarily in the PB, spleen and bone marrow, but they can infiltrate tissues and are also found in the liver, lungs, gut, lymph nodes and uterus (71–73). Two major subsets of PB-NK cells were identified on the basis of the surface density of CD56 antigen (CD56bright and CD56dim). CD56dim NK cells are predominant in PB, display a potent cytolytic activity and release cytokines shortly after receptor-mediated signaling. CD56bright predominate in tissues and secondary lymphoid organs, are poorly cytolytic, while they produce cytokines (74, 75).
The anti-tumor activity of NK cells is primarily related to their cytolytic potential and to the secretion of soluble factors or cytokines that may act on tumors either directly or indirectly upon recruitment of other cell types. NK cell cytotoxicity is induced by surface receptors capable of recognizing ligands that are primarily expressed by tumor cells, but not by most normal resting cells (76). These receptors may induce NK cell activation resulting in tumor cell lysis and secretion of cytokines. The major activating NK receptors include Natural cytotoxic receptor (NCR) (i.e., NKp46, NKp44, and NKp30), DNAM-1 and NKG2D. In addition, NK cells, in most instances, do not kill normal cells thanks to a fail-safe mechanism involving inhibitory receptors specific for HLA-class I molecules. These include killer Ig-like receptors (KIRs) that recognize allotypic determinants of HLA-cl I molecules shared by different groups of alleles and CD94/NKG2A that recognizes HLA-E (77).
During cancer progression, the transformed cells display a decrease or even a loss of the surface expression of MHC-I (78) while strongly upregulate or acquire the expression of ligands for activating NK receptors: two events necessary for NK activation and induction of anti-tumor immune cell responses (79). However, the frequent downregulation of activating receptor expression in NK cells may result in decreased activity leading to increases in tumor expansion and metastases. Indeed, it is well-known that tumor cells may create an immunosuppressive environment through the modulation of inhibitory checkpoints expression on NK cells in order to evade their cytolytic activity and to induce tumor immune escape (79–82).
Some tumors are poorly permeable to NK cells, as the TME may affect their ability to infiltrate the tumor mass. In particular, colorectal carcinoma and melanoma lesions display poor NK cell infiltration (83, 84). On the other hand, NK cell infiltration has been described in other types of tumor and a high number of NK cells in neoplastic tissues has been associated with better survival. For example in breast cancers, tumor-infiltrating (TI) NK cells are used as biomarkers to predict the response to anti-HER2 mAbs therapy (85–87). In the Head and Neck cancers the presence of TI-NK cells correlated with a longer survival (88). Similarly, NK cell infiltration of renal tumors is associated with a good prognosis (85). On the contrary, NK cell infiltration has no impact on clinical outcome in non-small-cell lung cancer (NSCLC) (89, 90).
NK-MDSC Interactions
NK cells may interact with tumor cells and other cells present in TME through three fundamental pathways, i.e., cell-to cell contact, secretion of soluble molecules in the extracellular milieu, and release of extracellular vesicles (EVs) (91). In cancer patients and tumor mice models, an inverse correlation between the presence of MDSC and NK cells exists (92).
In mice, the mechanism of NK cell inhibition exerted by MDSC is mainly related to cell-to-cell contact and it requires TGF-β (93). Membrane-bound TGF-β on MDSC has been shown to induce NK cell anergy thus impairing their cytotoxic capability and reducing NKG2D expression and IFN-γ production (94). Another study reported IL-33 as a novel player in MDSC-NK interaction. Following stress or damage, IL-33 is secreted by endothelial and epithelial cells and recruits both pro-tumorigenic or anti-tumorigenic immune cells (95, 96).
Furthermore, it has been reported that, in the presence of IL-1β, a novel subset of Ly6Cneg MDSC with higher inhibitory properties to NK cells expands in mice (97).
In humans, the inhibition of IFN-γ production by NK cells is related to a NKp30-dependent mechanism (98). MDSC can impair NK cell activity also by interfering with NK FcR-mediated cytotoxicity, as shown in cancer patients NK cells displaying reduced antibody-dependent cytotoxicity and production of cytokines (99).
The IFN-γ and other molecules present in the inflammatory microenvironment are able to promote the expansion of MDSC that, in turn, release high amount of IL-10. IL-10 is considered an anti-inflammatory cytokine capable of inhibiting the release of inflammatory cytokines playing an important role in anti-tumor immunoresponse. In particular, IL-10 may induce a pro-tumorigenic microenvironment affecting both NK cell and CD8+cytotoxic T lymphocyte activation and promoting a switch toward type 2 immunoresponse. Targeting either MDSC or IL-10 may favor type1 response and improve the anti-tumor activity of immune cells (100, 101).
Checkpoint blockade immunotherapy targeting the PD-1/PD-L1 inhibitory axis produced remarkable results in the treatment of several types of cancer (102–106). PD-1 is mostly expressed by T-cells, but NK cells with an activated and more responsive phenotype can also express PD-1 (107–110). In TME, tumor cells and their soluble mediators can increase PD-L1 expression on tumor-infiltrating MDSC (111, 112). Thus, PD-L1 expressed by MDSC can suppress NK cell activity while PD-L1 blockade may restore NK and T-cell responses. In different tumor types, increased PD-L1+MDSC has been observed and, in some instances, a correlation between the percentage of PD-L1+MDSC and disease stages or clinical outcome has been reported (113). In addition, NO produced by MDSC has a potent inhibitory effect on NK cells by impairing the Fc receptor-mediated killing, the secretion of IFN-γ, TNF-α, and Granzyme B, as detected in MDSC-co-cultured NK cells (99). Furthermore, IDO produced by MDSC can impair development and activation of NK cells by decreasing expression of NKG2D, NCR, DNAM1, and IFN-γ secretion (99, 114). IDO production is regulated by STAT3- induced NF-κB activation. It has been demonstrated that blockage of STAT3 and TGF-β can revert the MDSC-mediated inhibition of NK cell function (115, 116).
On the other hand, STAT5 has an opposite effect to that of STAT3. Indeed, STAT5, induced by Jak3, is responsible for perforin, granzyme and IFN-γ production in IL-2 –activated NK cells (117). NK cells co-cultured with MDSC isolated from spleen of tumor-bearing mice, showed both Jak3-inhibition and reduced STAT5 activation (98).
Another mechanism occurring in NK-MDSC interaction involves the TIGIT-CD155 axis. Thus, analysis of patients with CMV+ myelodysplastic syndrome revealed the presence of adaptive NK cells with lower TIGIT expression (partially) resistant to MDSC-mediated immunosuppression (118). Recently, in NK cells the IL-1R8 has been suggested as a novel immune check-point that can potentially interact with MDSC cells (119).
MDSC and NK Cross Talk in HSCT
MDSC were originally described as cells able to inhibit T cell activation, proliferation, and function. Other studies provided evidences that MDSC could also interact and interfere with the function of other cells, including NK cells, B cells, NKT cells, and DCs. All these observations are in line with the suppressive effect of MDSC in the context of hematopoietic stem cell transplantation (HSCT). Notably, HSCT from HLA-matched donor, either related or unrelated, is extensively used to cure patients with Acute Leukemia. The HSCT from HLA-haploidentical relatives (haplo-HSCT) gave the opportunity of a prompt transplantation in patients with no HLA-matched donor. Graft-vs.-host disease (GvHD) and post-transplant lymphoproliferative disease (PTLD) are two life-threatening effects of un-manipulated HSCT, due to the presence of T cells and B cells in the graft. In haplo-HSCT, graft of “mega-doses” of highly purified CD34+ HSC has been applied for many years. However, the lack in the graft of different mature lymphoid subsets and of (CD34−) committed hematopoietic progenitors results in a prolonged lymphopenia and delayed immune reconstitution that causes an increased risk of non-relapse-related mortality (NRM), due primarily to opportunistic infections. Thus, selective depletion of αβ T lymphocytes, and of B cells was used more as a novel method of graft manipulation. This approach allows the infusion in the recipient not only of hematopoietic progenitors but also of high numbers of donor mature NK cells, γδ T-cells and myeloid cells. In particular, NK and γδ T-cells transferred with the graft may contribute to prevent leukemia relapses and severe viral infections and/or reactivation before the establishment of adaptive immune responses thanks to their activity against leukemia blasts remaining in the patient after the conditioning regimen (120). Notably, it has been shown that in αβ T- and B-cell depleted HSCT setting, the contribution of the NK cell alloreactivity to the 5 years' survival probability was partially obscured, possibly by the effect of γδ T-cells (121–123).
Regarding the strategy routinely applied to increase the number of circulating HSC to be infused, donors receive G-CSF for 5 days (Figure 1). G-CSF induces a proteolytic microenvironment and inhibits CXCL12 production, thus favoring HSC egress from BM. An adequate number of HSC can be achieved also in “poor mobilizer” donors, who, in addition to G-CSF, receive Plerixafor (PL), a CXCR4 antagonist, which inhibits HSC retention in the BM, favoring their collection in the peripheral blood (PB). The G-CSF mobilization regimen induces an accumulation in PB of PMN-MDSC (124).
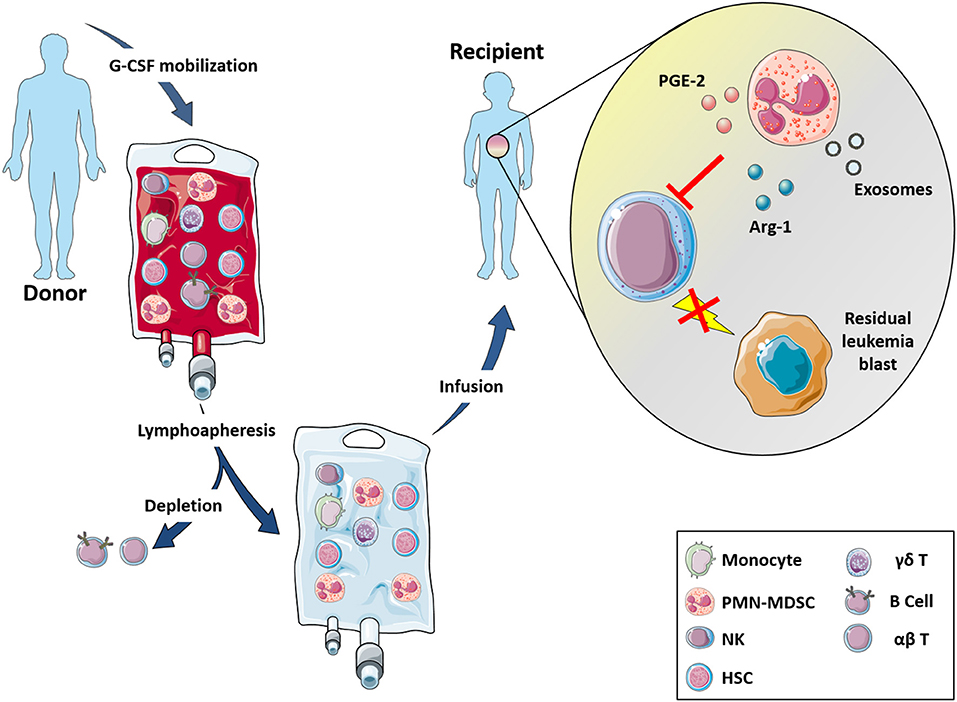
Figure 1. αβ T- and B-depleted hematopoietic stem cell transplantation protocol. Donors undergo lymphoapheresis after 5 days G-CSF stimulation for increasing the number of HSC cells and to induce PMN-MDSC mobilization. Thus, αβ T and B cells are depleted and infusion (enriched in NK cells, γδ T-cells and myeloid cells) is administered to recipient patients. In this transplant setting, the enrichment of PMN-MDSC helps to reduce NK cell cytotoxic effects against the graft by the release of IDO2, PGE2, and exosomes thus suggesting a possible involvement of these myeloid cells into the GvL activity mediated by NK cells.
We could demonstrate that PMN-MDSC derived from G-CSF mobilized donors did not interfere with the differentiation of donor HSC (124). On the contrary, they could affect the cytotoxic potential of donor-mature NK cells, which are infused into the patients during transplantation, compromising their GvL activity.
PMN-MDSC through the release of IDO metabolites and PGE2 down modulate the expression of intracellular polypeptides involved in the signal transduction and of the major activating NK receptors. In particular, signaling via activating receptor is mediated intracellularly by immunoreceptor tyrosine-based activation motifs (ITAM) and by downstream protein kinases. KARAP/DAP12 and CD3ζ are ITAM-bearing adaptor proteins known to associate with different activating NK receptors. These intracellular molecules involved in signal transduction were down-modulated in NK cells upon interaction with PMN-MDSC.
PMN-MDSC were also shown to affect NK cell degranulation, cytokine release and cytotoxicity. Indeed, in the presence of IDO- and PGE2-inhibitors the NK-cell activity can be recovered suggesting the involvement of IDO catabolites and PGE2 in the inhibition of the NK-mediated killing of leukemia blasts (124). It is known that MDSC may exploit additional immunomodulatory mechanisms including, for example, the release of exosomes. In this context, PMN-MDSC were able to release exosomes that are, in turn, internalized into NK cells and cause an impairment of their cytolytic activity (125) (Figure 1). Altogether, these data indicate that PMN-MDSC exert a potent inhibitory effect on anti-tumor NK cell function suggesting their possible involvement in the impairment of GvL activity mediated by NK cells.
Based on the in vitro data and on the role of these cells in hematologic malignancies, it is conceivable that MDSC may indeed represent a key immunosuppressive cell type induced in allogeneic HSCT. Further investigation regarding molecular and functional characteristics of MDSC may help to discover new strategies/drugs, to either dampen or enhance MDSC immunosuppressive activity, depending on the therapeutic need in different clinical contexts.
Concluding Remarks
A deeper comprehension of the mechanisms and relative molecular pathways adopted by MDSC present in TME to impair the anti-tumor function of immune effector cells may allow to identify novel therapeutic strategies capable to disrupt these potent inhibitory mechanisms. Thus, in HSCT the large proportion of PMN-MDSC can counteract the GvL activity mediated by donor-mature NK cells infused in the recipient, particularly in the early post-transplant period. Previous reports revealed that a reduction of the immunosuppressive activity of MDSC could be achieved by inducing their differentiation. It has been reported that the combined administration of ATRA (all-trans-retinoic acid) (126), paclitaxel (ultra-low non-cytotoxic doses) (127), vitamin D (128), and IL-2 (129) is able to induce MDSC differentiation by blocking their immunosuppressive activity and resulting in the recovery of immune response. In vitro data showed that chemotherapeutic agents (i.e., gemcitabine or 5-fluorouracil) could be used to selectively deplete MDSC with no toxic effects on other leukocyte populations (130, 131).
A better outstanding of interactions occurring between NK cells and PMN-MDSC, in particular in TME, may offer an interesting clue to further improve the efficacy of immunotherapy. In particular, in αβ T- and B cell-depleted haplo-HSCT setting, removing also PMN-MDSC, could preserve the NK-cell function with a further positive effect on the GvL activity and viral protection, obtaining a better patient's clinical outcome.
Author Contributions
All authors listed have made a substantial, direct and intellectual contribution to the work, and approved it for publication.
Funding
This work was supported by grants awarded by Associazione Italiana per la Ricerca sul Cancro (AIRC)-Special Program Metastatic disease: the key unmet need in oncology 5X1000 2018 Id. 21147 (LM), AIRC IG 2017 Id. 19920 (LM); RC-2020 OPBG (LM, PV); LQ has received funding from AIRC and from the European Union's Horizon 2020 research and innovation programme under the Marie Skłodowska-Curie grant agreement no 800924; NT and ALDP were supported by an AIRC fellowship for Italy.
Conflict of Interest
The authors declare that the research was conducted in the absence of any commercial or financial relationships that could be construed as a potential conflict of interest.
References
1. Dadi S, Chhangawala S, Whitlock BM, Franklin RA, Luo CT, Oh SA, et al. Cancer immunosurveillance by tissue-resident innate lymphoid cells and innate-like T cells. Cell. (2016) 164:365–77. doi: 10.1016/j.cell.2016.01.002
2. Vano YA, Petitprez F, Giraldo NA, Fridman WH, Sautes-Fridman C. Immune-based identification of cancer patients at high risk of progression. Curr Opin Immunol. (2018) 51:97–102. doi: 10.1016/j.coi.2018.03.005
3. Kwak Y, Koh J, Kim DW, Kang SB, Kim WH, Lee HS. Immunoscore encompassing CD3+ and CD8+ T cell densities in distant metastasis is a robust prognostic marker for advanced colorectal cancer. Oncotarget. (2016) 7:81778–90. doi: 10.18632/oncotarget.13207
4. Palucka AK, Coussens LM. The basis of oncoimmunology. Cell. (2016) 164:1233–47. doi: 10.1016/j.cell.2016.01.049
5. Fridman WH, Pages F, Sautes-Fridman C, Galon J. The immune contexture in human tumours: impact on clinical outcome. Nat Rev Cancer. (2012) 12:298–306. doi: 10.1038/nrc3245
6. Talmadge JE, Gabrilovich DI. History of myeloid-derived suppressor cells. Nat Rev Cancer. (2013) 13:739–52. doi: 10.1038/nrc3581
7. Gabrilovich DI, Nagaraj S. Myeloid-derived suppressor cells as regulators of the immune system. Nat Rev Immunol. (2009) 9:162–74. doi: 10.1038/nri2506
8. Condamine T, Dominguez GA, Youn JI, Kossenkov AV, Mony S, Alicea-Torres K, et al. Lectin-type oxidized LDL receptor-1 distinguishes population of human polymorphonuclear myeloid-derived suppressor cells in cancer patients. Sci Immunol. (2016) 1:aaf8943. doi: 10.1126/sciimmunol.aaf8943
9. Bronte V, Brandau S, Chen SH, Colombo MP, Frey AB, Greten TF, et al. Recommendations for myeloid-derived suppressor cell nomenclature and characterization standards. Nat Commun. (2016) 7:12150. doi: 10.1038/ncomms12150
10. Gabrilovich DI, Bronte V, Chen SH, Colombo MP, Ochoa A, Ostrand-Rosenberg S, et al. The terminology issue for myeloid-derived suppressor cells. Cancer Res. (2007) 67:425. doi: 10.1158/0008-5472.CAN-06-3037
11. Ostrand-Rosenberg S, Sinha P, Chornoguz O, Ecker C. Regulating the suppressors: apoptosis and inflammation govern the survival of tumor-induced myeloid-derived suppressor cells (MDSC). Cancer Immunol Immunother. (2012) 61:1319–25. doi: 10.1007/s00262-012-1269-6
12. Ray A, Chakraborty K, Ray P. Immunosuppressive MDSCs induced by TLR signaling during infection and role in resolution of inflammation. Front Cell Infect Microbiol. (2013) 3:52. doi: 10.3389/fcimb.2013.00052
13. Saiwai H, Kumamaru H, Ohkawa Y, Kubota K, Kobayakawa K, Yamada H, et al. Ly6C+ Ly6G- myeloid-derived suppressor cells play a critical role in the resolution of acute inflammation and the subsequent tissue repair process after spinal cord injury. J Neurochem. (2013) 125:74–88. doi: 10.1111/jnc.12135
14. Yamauchi Y, Safi S, Blattner C, Rathinasamy A, Umansky L, Juenger S, et al. Circulating and tumor myeloid-derived suppressor cells in resectable non-small cell lung cancer. Am J Respir Crit Care Med. (2018) 198:777–87. doi: 10.1164/rccm.201708-1707OC
15. Clavijo PE, Moore EC, Chen J, Davis RJ, Friedman J, Kim Y, et al. Resistance to CTLA-4 checkpoint inhibition reversed through selective elimination of granulocytic myeloid cells. Oncotarget. (2017) 8:55804–20. doi: 10.18632/oncotarget.18437
16. Chai E, Zhang L, Li C. LOX-1+ PMN-MDSC enhances immune suppression which promotes glioblastoma multiforme progression. Cancer Manag Res. (2019) 11:7307–15. doi: 10.2147/CMAR.S210545
17. Sheng IY, Diaz-Montero CM, Rayman P, Wei W, Finke JH, Kim JS, et al. Blood myeloid-derived suppressor cells correlate with neutrophil-to-lymphocyte ratio and overall survival in metastatic urothelial carcinoma. Target Oncol. (2020) 15:211–20. doi: 10.1007/s11523-020-00707-z
18. Zhong LM, Liu ZG, Zhou X, Song SH, Weng GY, Wen Y, et al. Expansion of PMN-myeloid derived suppressor cells and their clinical relevance in patients with oral squamous cell carcinoma. Oral oncology. (2019) 95:157–63. doi: 10.1016/j.oraloncology.2019.06.004
19. Huang B, Lei Z, Zhao J, Gong W, Liu J, Chen Z, et al. CCL2/CCR2 pathway mediates recruitment of myeloid suppressor cells to cancers. Cancer Lett. (2007) 252:86–92. doi: 10.1016/j.canlet.2006.12.012
20. Katoh H, Wang D, Daikoku T, Sun H, Dey SK, Dubois RN. CXCR2-expressing myeloid-derived suppressor cells are essential to promote colitis-associated tumorigenesis. Cancer Cell. (2013) 24:631–44. doi: 10.1016/j.ccr.2013.10.009
21. Blattner C, Fleming V, Weber R, Himmelhan B, Altevogt P, Gebhardt C, et al. CCR5(+) myeloid-derived suppressor cells are enriched and activated in melanoma lesions. Cancer Res. (2018) 78:157–67. doi: 10.1158/0008-5472.CAN-17-0348
22. Umansky V, Blattner C, Gebhardt C, Utikal J. CCR5 in recruitment and activation of myeloid-derived suppressor cells in melanoma. Cancer Immunol Immunother. (2017) 66:1015–23. doi: 10.1007/s00262-017-1988-9
23. Cheon EC, Khazaie K, Khan MW, Strouch MJ, Krantz SB, Phillips J, et al. Mast cell 5-lipoxygenase activity promotes intestinal polyposis in APCDelta468 mice. Cancer Res. (2011) 71:1627–36. doi: 10.1158/0008-5472.CAN-10-1923
24. Molon B, Ugel S, Del Pozzo F, Soldani C, Zilio S, Avella D, et al. Chemokine nitration prevents intratumoral infiltration of antigen-specific T cells. J Exp Med. (2011) 208:1949–62. doi: 10.1084/jem.20101956
25. Obermajer N, Muthuswamy R, Lesnock J, Edwards RP, Kalinski P. Positive feedback between PGE2 and COX2 redirects the differentiation of human dendritic cells toward stable myeloid-derived suppressor cells. Blood. (2011) 118:5498–505. doi: 10.1182/blood-2011-07-365825
26. Kortylewski M, Kujawski M, Wang T, Wei S, Zhang S, Pilon-Thomas S, et al. Inhibiting Stat3 signaling in the hematopoietic system elicits multicomponent antitumor immunity. Nature medicine. (2005) 11:1314–21. doi: 10.1038/nm1325
27. Nefedova Y, Cheng P, Gilkes D, Blaskovich M, Beg AA, Sebti SM, et al. Activation of dendritic cells via inhibition of Jak2/STAT3 signaling. J Immunol. (2005) 175:4338–46. doi: 10.4049/jimmunol.175.7.4338
28. Martin RK, Saleem SJ, Folgosa L, Zellner HB, Damle SR, Nguyen GK, et al. Mast cell histamine promotes the immunoregulatory activity of myeloid-derived suppressor cells. J Leukoc Biol. (2014) 96:151–9. doi: 10.1189/jlb.5A1213-644R
29. Saleem SJ, Martin RK, Morales JK, Sturgill JL, Gibb DR, Graham L, et al. Cutting edge: mast cells critically augment myeloid-derived suppressor cell activity. J Immunol. (2012) 189:511–5. doi: 10.4049/jimmunol.1200647
30. Corzo CA, Condamine T, Lu L, Cotter MJ, Youn JI, Cheng P, et al. HIF-1alpha regulates function and differentiation of myeloid-derived suppressor cells in the tumor microenvironment. J Exp Med. (2010) 207:2439–53. doi: 10.1084/jem.20100587
31. Christiansson L, Soderlund S, Svensson E, Mustjoki S, Bengtsson M, Simonsson B, et al. Increased level of myeloid-derived suppressor cells, programmed death receptor ligand 1/programmed death receptor 1, and soluble CD25 in Sokal high risk chronic myeloid leukemia. PloS ONE. (2013) 8:e55818. doi: 10.1371/journal.pone.0055818
32. Giallongo C, Parrinello N, Tibullo D, La Cava P, Romano A, Chiarenza A, et al. Myeloid derived suppressor cells (MDSCs) are increased and exert immunosuppressive activity together with polymorphonuclear leukocytes (PMNs) in chronic myeloid leukemia patients. PloS ONE. (2014) 9:e101848. doi: 10.1371/journal.pone.0101848
33. Giallongo C, Romano A, Parrinello NL, La Cava P, Brundo MV, Bramanti V, et al. Mesenchymal Stem Cells (MSC) regulate activation of Granulocyte-like Myeloid Derived Suppressor Cells (G-MDSC) in chronic myeloid leukemia patients. PloS ONE. (2016) 11:e0158392. doi: 10.1371/journal.pone.0158392
34. Sun H, Li Y, Zhang ZF, Ju Y, Li L, Zhang BC, et al. Increase in myeloid-derived suppressor cells (MDSCs) associated with minimal residual disease (MRD) detection in adult acute myeloid leukemia. Int J Hematol. (2015) 102:579–86. doi: 10.1007/s12185-015-1865-2
35. Liu YF, Chen YY, He YY, Wang JY, Yang JP, Zhong SL, et al. Expansion and activation of granulocytic, myeloid-derived suppressor cells in childhood precursor B cell acute lymphoblastic leukemia. J Leukoc Biol. (2017) 102:449–58. doi: 10.1189/jlb.5MA1116-453RR
36. Bansal AK, Sharawat SK, Gupta R, Vishnubhatla S, Dhawan D, Bakhshi S. Regulatory T cells in pediatric AML are associated with disease load and their serial assessment suggests role in leukemogenesis. Am J Blood Res. (2020) 10:90–6.
37. Marini O, Spina C, Mimiola E, Cassaro A, Malerba G, Todeschini G, et al. Identification of granulocytic myeloid-derived suppressor cells (G-MDSCs) in the peripheral blood of Hodgkin and non-Hodgkin lymphoma patients. Oncotarget. (2016) 7:27676–88. doi: 10.18632/oncotarget.8507
38. Lin Y, Gustafson MP, Bulur PA, Gastineau DA, Witzig TE, Dietz AB. Immunosuppressive CD14+HLA-DR(low)/- monocytes in B-cell non-Hodgkin lymphoma. Blood. (2011) 117:872–81. doi: 10.1182/blood-2010-05-283820
39. Gustafson MP, Abraham RS, Lin Y, Wu W, Gastineau DA, Zent CS, et al. Association of an increased frequency of CD14+ HLA-DR lo/neg monocytes with decreased time to progression in chronic lymphocytic leukaemia (CLL). Br J Haematol. (2012) 156:674–6. doi: 10.1111/j.1365-2141.2011.08902.x
40. Romano A, Parrinello NL, Vetro C, Forte S, Chiarenza A, Figuera A, et al. Circulating myeloid-derived suppressor cells correlate with clinical outcome in Hodgkin Lymphoma patients treated up-front with a risk-adapted strategy. Br J Haematol. (2015) 168:689–700. doi: 10.1111/bjh.13198
41. Malek E, de Lima M, Letterio JJ, Kim BG, Finke JH, Driscoll JJ, et al. Myeloid-derived suppressor cells: the green light for myeloma immune escape. Blood Rev. (2016) 30:341–8. doi: 10.1016/j.blre.2016.04.002
42. Serafini P, Meckel K, Kelso M, Noonan K, Califano J, Koch W, et al. Phosphodiesterase-5 inhibition augments endogenous antitumor immunity by reducing myeloid-derived suppressor cell function. J Exp Med. (2006) 203:2691–702. doi: 10.1084/jem.20061104
43. Hou Y, Feng Q, Xu M, Li GS, Liu XN, Sheng Z, et al. High-dose dexamethasone corrects impaired myeloid-derived suppressor cell function via Ets1 in immune thrombocytopenia. Blood. (2016) 127:1587–97. doi: 10.1182/blood-2015-10-674531
44. Shao X, Wu B, Cheng L, Li F, Zhan Y, Liu C, et al. Distinct alterations of CD68(+)CD163(+) M2-like macrophages and myeloid-derived suppressor cells in newly diagnosed primary immune thrombocytopenia with or without CR after high-dose dexamethasone treatment. J Transl Med. (2018) 16:48. doi: 10.1186/s12967-018-1424-8
45. Papadaki HA, Stamatopoulos K, Damianaki A, Gemetzi C, Anagnostopoulos A, Papadaki T, et al. Activated T-lymphocytes with myelosuppressive properties in patients with chronic idiopathic neutropenia. Br J Haematol. (2005) 128:863–76. doi: 10.1111/j.1365-2141.2005.05380.x
46. Wang Z, Jiang J, Li Z, Zhang J, Wang H, Qin Z. A myeloid cell population induced by Freund adjuvant suppresses T-cell-mediated antitumor immunity. J Immunother. (2010) 33:167–77. doi: 10.1097/CJI.0b013e3181bed2ba
47. Gehad AE, Lichtman MK, Schmults CD, Teague JE, Calarese AW, Jiang Y, et al. Nitric oxide-producing myeloid-derived suppressor cells inhibit vascular E-selectin expression in human squamous cell carcinomas. J Investig Dermatol. (2012) 132:2642–51. doi: 10.1038/jid.2012.190
48. Kusmartsev S, Nefedova Y, Yoder D, Gabrilovich DI. Antigen-specific inhibition of CD8+ T cell response by immature myeloid cells in cancer is mediated by reactive oxygen species. J Immunol. (2004) 172:989–99. doi: 10.4049/jimmunol.172.2.989
49. Tumino N, Turchi F, Meschi S, Lalle E, Bordoni V, Casetti R, et al. In HIV-positive patients, myeloid-derived suppressor cells induce T-cell anergy by suppressing CD3zeta expression through ELF-1 inhibition. AIDS. (2015) 29:2397–407. doi: 10.1097/QAD.0000000000000871
50. Hanson EM, Clements VK, Sinha P, Ilkovitch D, Ostrand-Rosenberg S. Myeloid-derived suppressor cells down-regulate L-selectin expression on CD4+ and CD8+ T cells. J Immunol. (2009) 183:937–44. doi: 10.4049/jimmunol.0804253
51. Rodriguez PC, Quiceno DG, Zabaleta J, Ortiz B, Zea AH, Piazuelo MB, et al. Arginase I production in the tumor microenvironment by mature myeloid cells inhibits T-cell receptor expression and antigen-specific T-cell responses. Cancer Res. (2004) 64:5839–49. doi: 10.1158/0008-5472.CAN-04-0465
52. Srivastava MK, Sinha P, Clements VK, Rodriguez P, Ostrand-Rosenberg S. Myeloid-derived suppressor cells inhibit T-cell activation by depleting cystine and cysteine. Cancer Res. (2010) 70:68–77. doi: 10.1158/0008-5472.CAN-09-2587
53. Rodriguez PC, Quiceno DG, Ochoa AC. L-arginine availability regulates T-lymphocyte cell-cycle progression. Blood. (2007) 109:1568–73. doi: 10.1182/blood-2006-06-031856
54. Huang B, Pan PY, Li Q, Sato AI, Levy DE, Bromberg J, et al. Gr-1+CD115+ immature myeloid suppressor cells mediate the development of tumor-induced T regulatory cells and T-cell anergy in tumor-bearing host. Cancer Res. (2006) 66:1123–31. doi: 10.1158/0008-5472.CAN-05-1299
55. Zhang G, Huang H, Zhu Y, Yu G, Gao X, Xu Y, et al. A novel subset of B7-H3(+)CD14(+)HLA-DR(-/low) myeloid-derived suppressor cells are associated with progression of human NSCLC. Oncoimmunology. (2015) 4:e977164. doi: 10.4161/2162402X.2014.977164
56. Li L, Wang L, Li J, Fan Z, Yang L, Zhang Z, et al. Metformin-induced reduction of CD39 and CD73 blocks myeloid-derived suppressor cell activity in patients with ovarian cancer. Cancer Res. (2018) 78:1779–91. doi: 10.1158/0008-5472.CAN-17-2460
57. Li J, Wang L, Chen X, Li L, Li Y, Ping Y, et al. CD39/CD73 upregulation on myeloid-derived suppressor cells via TGF-beta-mTOR-HIF-1 signaling in patients with non-small cell lung cancer. Oncoimmunology. (2017) 6:e1320011. doi: 10.1080/2162402X.2017.1320011
58. Pan PY, Ma G, Weber KJ, Ozao-Choy J, Wang G, Yin B, et al. Immune stimulatory receptor CD40 is required for T-cell suppression and T regulatory cell activation mediated by myeloid-derived suppressor cells in cancer. Cancer Res. (2010) 70:99–108. doi: 10.1158/0008-5472.CAN-09-1882
59. Hoechst B, Ormandy LA, Ballmaier M, Lehner F, Kruger C, Manns MP, et al. A new population of myeloid-derived suppressor cells in hepatocellular carcinoma patients induces CD4(+)CD25(+)Foxp3(+) T cells. Gastroenterology. (2008) 135:234–43. doi: 10.1053/j.gastro.2008.03.020
60. Younis RH, Han KL, Webb TJ. Human head and neck squamous cell carcinoma-associated semaphorin 4D induces expansion of myeloid-derived suppressor cells. J Immunol. (2016) 196:1419–29. doi: 10.4049/jimmunol.1501293
61. Domenis R, Cesselli D, Toffoletto B, Bourkoula E, Caponnetto F, Manini I, et al. Systemic T cells immunosuppression of glioma stem cell-derived exosomes is mediated by monocytic myeloid-derived suppressor cells. PloS ONE. (2017) 12:e0169932. doi: 10.1371/journal.pone.0169932
62. Qian L, Liu Y, Wang S, Gong W, Jia X, Liu L, et al. NKG2D ligand RAE1epsilon induces generation and enhances the inhibitor function of myeloid-derived suppressor cells in mice. J Cell Mol Med. (2017) 21:2046–54. doi: 10.1111/jcmm.13124
63. Hoechst B, Gamrekelashvili J, Manns MP, Greten TF, Korangy F. Plasticity of human Th17 cells and iTregs is orchestrated by different subsets of myeloid cells. Blood. (2011) 117:6532–41. doi: 10.1182/blood-2010-11-317321
64. Noman MZ, Desantis G, Janji B, Hasmim M, Karray S, Dessen P, et al. PD-L1 is a novel direct target of HIF-1alpha, and its blockade under hypoxia enhanced MDSC-mediated T cell activation. J Exp Med. (2014) 211:781–90. doi: 10.1084/jem.20131916
65. Spranger S, Koblish HK, Horton B, Scherle PA, Newton R, Gajewski TF. Mechanism of tumor rejection with doublets of CTLA-4, PD-1/PD-L1, or IDO blockade involves restored IL-2 production and proliferation of CD8(+) T cells directly within the tumor microenvironment. J Immunother Cancer. (2014) 2:3. doi: 10.1186/2051-1426-2-3
66. Wang Y, Ding Y, Guo N, Wang S. MDSCs: key criminals of tumor pre-metastatic niche formation. Front Immunol. (2019) 10:172. doi: 10.3389/fimmu.2019.00172
67. Rivera LB, Bergers G. Intertwined regulation of angiogenesis and immunity by myeloid cells. Trends Immunol. (2015) 36:240–9. doi: 10.1016/j.it.2015.02.005
68. Ostrand-Rosenberg S. Myeloid-derived suppressor cells: more mechanisms for inhibiting antitumor immunity. Cancer Immunol Immunother. (2010) 59:1593–600. doi: 10.1007/s00262-010-0855-8
69. Burke M, Choksawangkarn W, Edwards N, Ostrand-Rosenberg S, Fenselau C. Exosomes from myeloid-derived suppressor cells carry biologically active proteins. J Proteome Res. (2014) 13:836–43. doi: 10.1021/pr400879c
70. Artis D, Spits H. The biology of innate lymphoid cells. Nature. (2015) 517:293–301. doi: 10.1038/nature14189
71. Shi FD, Ljunggren HG, La Cava A, Van Kaer L. Organ-specific features of natural killer cells. Nat Rev Immunol. (2011) 11:658–71. doi: 10.1038/nri3065
72. Tumino N, Martini S, Munari E, Scordamaglia F, Besi F, Mariotti FR, et al. Presence of innate lymphoid cells in pleural effusions of primary and metastatic tumors: functional analysis and expression of PD-1 receptor. Int J Cancer. (2019) 145:1660–8. doi: 10.1002/ijc.32262
73. Sivori S, Pende D, Quatrini L, Pietra G, Della Chiesa M, Vacca P, et al. NK cells and ILCs in tumor immunotherapy. Mol Aspects Med. (2020) 100870. doi: 10.1016/j.mam.2020.100870
74. Moretta L. Dissecting CD56dim human NK cells. Blood. (2010) 116:3689–91. doi: 10.1182/blood-2010-09-303057
75. Cooper MA, Fehniger TA, Turner SC, Chen KS, Ghaheri BA, Ghayur T, et al. Human natural killer cells: a unique innate immunoregulatory role for the CD56(bright) subset. Blood. (2001) 97:3146–51. doi: 10.1182/blood.V97.10.3146
76. Moretta A, Bottino C, Vitale M, Pende D, Cantoni C, Mingari MC, et al. Activating receptors and coreceptors involved in human natural killer cell-mediated cytolysis. Ann Rev Immunol. (2001) 19:197–223. doi: 10.1146/annurev.immunol.19.1.197
77. Moretta A, Bottino C, Vitale M, Pende D, Biassoni R, Mingari MC, et al. Receptors for HLA class-I molecules in human natural killer cells. Ann Rev Immunol. (1996) 14:619–48. doi: 10.1146/annurev.immunol.14.1.619
78. DuPage M, Mazumdar C, Schmidt LM, Cheung AF, Jacks T. Expression of tumour-specific antigens underlies cancer immunoediting. Nature. (2012) 482:405–9. doi: 10.1038/nature10803
79. Fregni G, Perier A, Avril MF, Caignard A. NK cells sense tumors, course of disease and treatments: consequences for NK-based therapies. Oncoimmunology. (2012) 1:38–47. doi: 10.4161/onci.1.1.18312
80. Chinai JM, Janakiram M, Chen F, Chen W, Kaplan M, Zang X. New immunotherapies targeting the PD-1 pathway. Trends Pharmacol Sci. (2015) 36:587–95. doi: 10.1016/j.tips.2015.06.005
81. Zitvogel L, Galluzzi L, Smyth MJ, Kroemer G. Mechanism of action of conventional and targeted anticancer therapies: reinstating immunosurveillance. Immunity. (2013) 39:74–88. doi: 10.1016/j.immuni.2013.06.014
82. Habif G, Crinier A, Andre P, Vivier E, Narni-Mancinelli E. Targeting natural killer cells in solid tumors. Cell Mol Immunol. (2019) 16:415–22. doi: 10.1038/s41423-019-0224-2
83. Tartter PI, Steinberg B, Barron DM, Martinelli G. The prognostic significance of natural killer cytotoxicity in patients with colorectal cancer. Arch Surg. (1987) 122:1264–8. doi: 10.1001/archsurg.1987.01400230050009
84. Halama N, Braun M, Kahlert C, Spille A, Quack C, Rahbari N, et al. Natural killer cells are scarce in colorectal carcinoma tissue despite high levels of chemokines and cytokines. Clin Cancer Res. (2011) 17:678–89. doi: 10.1158/1078-0432.CCR-10-2173
85. Eckl J, Buchner A, Prinz PU, Riesenberg R, Siegert SI, Kammerer R, et al. Transcript signature predicts tissue NK cell content and defines renal cell carcinoma subgroups independent of TNM staging. J Mol Med. (2012) 90:55–66. doi: 10.1007/s00109-011-0806-7
86. Salgado R, Denkert C, Campbell C, Savas P, Nuciforo P, Aura C, et al. Tumor-infiltrating lymphocytes and associations with pathological complete response and event-free survival in HER2-positive early-stage breast cancer treated with lapatinib and trastuzumab: a secondary analysis of the NeoALTTO trial. JAMA Oncol. (2015) 1:448–54. doi: 10.1001/jamaoncol.2015.0830
87. Arnould L, Gelly M, Penault-Llorca F, Benoit L, Bonnetain F, Migeon C, et al. Trastuzumab-based treatment of HER2-positive breast cancer: an antibody-dependent cellular cytotoxicity mechanism? Br J Cancer. (2006) 94:259–67. doi: 10.1038/sj.bjc.6602930
88. Schantz SP, Ordonez NG. Quantitation of natural killer cell function and risk of metastatic poorly differentiated head and neck cancer. Nat Immunity Cell Growth Regul. (1991) 10:278–88.
89. Lavin Y, Kobayashi S, Leader A, Amir ED, Elefant N, Bigenwald C, et al. Innate immune landscape in early lung adenocarcinoma by paired single-cell analyses. Cell. (2017) 169:750–65 e17. doi: 10.1016/j.cell.2017.04.014
90. Carrega P, Morandi B, Costa R, Frumento G, Forte G, Altavilla G, et al. Natural killer cells infiltrating human nonsmall-cell lung cancer are enriched in CD56 bright CD16(-) cells and display an impaired capability to kill tumor cells. Cancer. (2008) 112:863–75. doi: 10.1002/cncr.23239
91. Di Pace AL, Tumino N, Besi F, Alicata C, Conti LA, Munari E, et al. Characterization of human NK cell-derived exosomes: role of DNAM1 receptor in exosome-mediated cytotoxicity against tumor. Cancers. (2020) 12:661. doi: 10.3390/cancers12030661
92. Wu AA, Drake V, Huang HS, Chiu S, Zheng L. Reprogramming the tumor microenvironment: tumor-induced immunosuppressive factors paralyze T cells. Oncoimmunology. (2015) 4:e1016700. doi: 10.1080/2162402X.2015.1016700
93. Li Z, Pang Y, Gara SK, Achyut BR, Heger C, Goldsmith PK, et al. Gr-1+CD11b+ cells are responsible for tumor promoting effect of TGF-beta in breast cancer progression. Int J Cancer. (2012) 131:2584–95. doi: 10.1002/ijc.27572
94. Li H, Han Y, Guo Q, Zhang M, Cao X. Cancer-expanded myeloid-derived suppressor cells induce anergy of NK cells through membrane-bound TGF-beta 1. J Immunol. (2009) 182:240–9. doi: 10.4049/jimmunol.182.1.240
95. Fournie JJ, Poupot M. The pro-tumorigenic IL-33 involved in antitumor immunity: a yin and yang cytokine. Front Immunol. (2018) 9:2506. doi: 10.3389/fimmu.2018.02506
96. Shen JX, Liu J, Zhang GJ. Interleukin-33 in malignancies: friends or foes? Front Immunol. (2018) 9:3051. doi: 10.3389/fimmu.2018.03051
97. Elkabets M, Ribeiro VS, Dinarello CA, Ostrand-Rosenberg S, Di Santo JP, Apte RN, et al. IL-1beta regulates a novel myeloid-derived suppressor cell subset that impairs NK cell development and function. Eur J Immunol. (2010) 40:3347–57. doi: 10.1002/eji.201041037
98. Hoechst B, Voigtlaender T, Ormandy L, Gamrekelashvili J, Zhao F, Wedemeyer H, et al. Myeloid derived suppressor cells inhibit natural killer cells in patients with hepatocellular carcinoma via the NKp30 receptor. Hepatology. (2009) 50:799–807. doi: 10.1002/hep.23054
99. Stiff A, Trikha P, Mundy-Bosse B, McMichael E, Mace TA, Benner B, et al. Nitric oxide production by myeloid-derived suppressor cells plays a role in impairing Fc receptor-mediated natural killer cell function. Clin Cancer Res. (2018) 24:1891–904. doi: 10.1158/1078-0432.CCR-17-0691
100. Ibrahim ML, Klement JD, Lu C, Redd PS, Xiao W, Yang D, et al. Myeloid-derived suppressor cells produce IL-10 to elicit DNMT3b-dependent IRF8 silencing to promote colitis-associated colon tumorigenesis. Cell Rep. (2018) 25:3036–46 e6. doi: 10.1016/j.celrep.2018.11.050
101. Yaseen MM, Abuharfeil NM, Darmani H, Daoud A. Mechanisms of immune suppression by myeloid-derived suppressor cells: the role of interleukin-10 as a key immunoregulatory cytokine. Open Biol. (2020) 10:200111. doi: 10.1098/rsob.200111
102. Baumeister SH, Freeman GJ, Dranoff G, Sharpe AH. Coinhibitory pathways in immunotherapy for cancer. Ann Immunol. (2016) 34:539–73. doi: 10.1146/annurev-immunol-032414-112049
103. Okazaki T, Chikuma S, Iwai Y, Fagarasan S, Honjo T. A rheostat for immune responses: the unique properties of PD-1 and their advantages for clinical application. Nat Immunol. (2013) 14:1212–8. doi: 10.1038/ni.2762
104. Okazaki T, Honjo T. PD-1 and PD-1 ligands: from discovery to clinical application. Int Immunol. (2007) 19:813–24. doi: 10.1093/intimm/dxm057
105. Schumacher TN, Schreiber RD. Neoantigens in cancer immunotherapy. Science. (2015) 348:69–74. doi: 10.1126/science.aaa4971
106. Sharma P, Allison JP. Immune checkpoint targeting in cancer therapy: toward combination strategies with curative potential. Cell. (2015) 161:205–14. doi: 10.1016/j.cell.2015.03.030
107. Barry KC, Hsu J, Broz ML, Cueto FJ, Binnewies M, Combes AJ, et al. A natural killer-dendritic cell axis defines checkpoint therapy-responsive tumor microenvironments. Nat Med. (2018) 24:1178–91. doi: 10.1038/s41591-018-0085-8
108. Hsu J, Hodgins JJ, Marathe M, Nicolai CJ, Bourgeois-Daigneault MC, Trevino TN, et al. Contribution of NK cells to immunotherapy mediated by PD-1/PD-L1 blockade. J Clin Investig. (2018) 128:4654–68. doi: 10.1172/JCI99317
109. Quatrini L, Mariotti FR, Munari E, Tumino N, Vacca P, Moretta L. The immune checkpoint PD-1 in natural killer cells: expression, function and targeting in tumour immunotherapy. Cancers. (2020) 12:3285. doi: 10.3390/cancers12113285
110. Mariotti FR, Petrini S, Ingegnere T, Tumino N, Besi F, Scordamaglia F, et al. PD-1 in human NK cells: evidence of cytoplasmic mRNA and protein expression. Oncoimmunology. (2019) 8:1557030. doi: 10.1080/2162402X.2018.1557030
111. Azzaoui I, Uhel F, Rossille D, Pangault C, Dulong J, Le Priol J, et al. T-cell defect in diffuse large B-cell lymphomas involves expansion of myeloid-derived suppressor cells. Blood. (2016) 128:1081–92. doi: 10.1182/blood-2015-08-662783
112. Lu C, Redd PS, Lee JR, Savage N, Liu K. The expression profiles and regulation of PD-L1 in tumor-induced myeloid-derived suppressor cells. Oncoimmunology. (2016) 5:e1247135. doi: 10.1080/2162402X.2016.1247135
113. Iwata T, Kondo Y, Kimura O, Morosawa T, Fujisaka Y, Umetsu T, et al. PD-L1(+)MDSCs are increased in HCC patients and induced by soluble factor in the tumor microenvironment. Sci Rep. (2016) 6:39296. doi: 10.1038/srep39296
114. Zhang J, Han X, Hu X, Jin F, Gao Z, Yin L, et al. IDO1 impairs NK cell cytotoxicity by decreasing NKG2D/NKG2DLs via promoting miR-18a. Mol Immunol. (2018) 103:144–55. doi: 10.1016/j.molimm.2018.09.011
115. Sun X, Sui Q, Zhang C, Tian Z, Zhang J. Targeting blockage of STAT3 in hepatocellular carcinoma cells augments NK cell functions via reverse hepatocellular carcinoma-induced immune suppression. Mol Cancer Ther. (2013) 12:2885–96. doi: 10.1158/1535-7163.MCT-12-1087
116. Sui Q, Zhang J, Sun X, Zhang C, Han Q, Tian Z. NK cells are the crucial antitumor mediators when STAT3-mediated immunosuppression is blocked in hepatocellular carcinoma. J Immunol. (2014) 193:2016–23. doi: 10.4049/jimmunol.1302389
117. Gotthardt D, Sexl V. STATs in NK-cells: the good, the bad, and the ugly. Front Immunol. (2016) 7:694. doi: 10.3389/fimmu.2016.00694
118. Sarhan D, Cichocki F, Zhang B, Yingst A, Spellman SR, Cooley S, et al. Adaptive NK cells with low TIGIT expression are inherently resistant to myeloid-derived suppressor cells. Cancer Res. (2016) 76:5696–706. doi: 10.1158/0008-5472.CAN-16-0839
119. Molgora M, Bonavita E, Ponzetta A, Riva F, Barbagallo M, Jaillon S, et al. IL-1R8 is a checkpoint in NK cells regulating anti-tumour and anti-viral activity. Nature. (2017) 551:110–4. doi: 10.1038/nature24293
120. Pistoia V, Tumino N, Vacca P, Veneziani I, Moretta A, Locatelli F, et al. Human gammadelta T-Cells: from surface receptors to the therapy of high-risk leukemias. Front Immunol. (2018) 9:984. doi: 10.3389/fimmu.2018.00984
121. Locatelli F, Merli P, Pagliara D, Li Pira G, Falco M, Pende D, et al. Outcome of children with acute leukemia given HLA-haploidentical HSCT after alphabeta T-cell and B-cell depletion. Blood. (2017) 130:677–85. doi: 10.1182/blood-2017-04-779769
122. Vacca P, Montaldo E, Croxatto D, Moretta F, Bertaina A, Vitale C, et al. NK cells and other innate lymphoid cells in hematopoietic stem cell transplantation. Front Immunol. (2016) 7:188. doi: 10.3389/fimmu.2016.00188
123. Pietra G, Vitale C, Pende D, Bertaina A, Moretta F, Falco M, et al. Human natural killer cells: news in the therapy of solid tumors and high-risk leukemias. Cancer Immunol Immunother. (2016) 65:465–76. doi: 10.1007/s00262-015-1744-y
124. Tumino N, Besi F, Di Pace AL, Mariotti FR, Merli P, Li Pira G, et al. PMN-MDSC are a new target to rescue graft-versus-leukemia activity of NK cells in haplo-HSC transplantation. Leukemia. (2020) 34:932–7. doi: 10.1038/s41375-019-0585-7
125. Zoller M, Zhao K, Kutlu N, Bauer N, Provaznik J, Hackert T, et al. Immunoregulatory effects of myeloid-derived suppressor cell exosomes in mouse model of autoimmune alopecia areata. Front Immunol. (2018) 9:1279. doi: 10.3389/fimmu.2018.01279
126. Ugel S, Delpozzo F, Desantis G, Papalini F, Simonato F, Sonda N, et al. Therapeutic targeting of myeloid-derived suppressor cells. Curr Opin Pharmacol. (2009) 9:470–81. doi: 10.1016/j.coph.2009.06.014
127. Sevko A, Michels T, Vrohlings M, Umansky L, Beckhove P, Kato M, et al. Antitumor effect of paclitaxel is mediated by inhibition of myeloid-derived suppressor cells and chronic inflammation in the spontaneous melanoma model. J Immunol. (2013) 190:2464–71. doi: 10.4049/jimmunol.1202781
128. Friedman AD. Transcriptional control of granulocyte and monocyte development. Oncogene. (2007) 26:6816–28. doi: 10.1038/sj.onc.1210764
129. Mirza N, Fishman M, Fricke I, Dunn M, Neuger AM, Frost TJ, et al. All-trans-retinoic acid improves differentiation of myeloid cells and immune response in cancer patients. Cancer Res. (2006) 66:9299–307. doi: 10.1158/0008-5472.CAN-06-1690
130. Suzuki E, Kapoor V, Jassar AS, Kaiser LR, Albelda SM. Gemcitabine selectively eliminates splenic Gr-1+/CD11b+ myeloid suppressor cells in tumor-bearing animals and enhances antitumor immune activity. Clin Cancer Res. (2005) 11:6713–21. doi: 10.1158/1078-0432.CCR-05-0883
Keywords: natural killer cells, myeloid-derived suppressor cells, hematopoietic stem cell transplantation, tumor microenvironment, hematological malignancies
Citation: Tumino N, Di Pace AL, Besi F, Quatrini L, Vacca P and Moretta L (2021) Interaction Between MDSC and NK Cells in Solid and Hematological Malignancies: Impact on HSCT. Front. Immunol. 12:638841. doi: 10.3389/fimmu.2021.638841
Received: 07 December 2020; Accepted: 25 January 2021;
Published: 12 February 2021.
Edited by:
Erik Wennerberg, Institute of Cancer Research (ICR), United KingdomReviewed by:
Dhifaf Sarhan, Karolinska Institutet (KI), SwedenSubramaniam Malarkannan, Medical College of Wisconsin, United States
Copyright © 2021 Tumino, Di Pace, Besi, Quatrini, Vacca and Moretta. This is an open-access article distributed under the terms of the Creative Commons Attribution License (CC BY). The use, distribution or reproduction in other forums is permitted, provided the original author(s) and the copyright owner(s) are credited and that the original publication in this journal is cited, in accordance with accepted academic practice. No use, distribution or reproduction is permitted which does not comply with these terms.
*Correspondence: Lorenzo Moretta, bG9yZW56by5tb3JldHRhQG9wYmcubmV0
†These authors share senior authorship