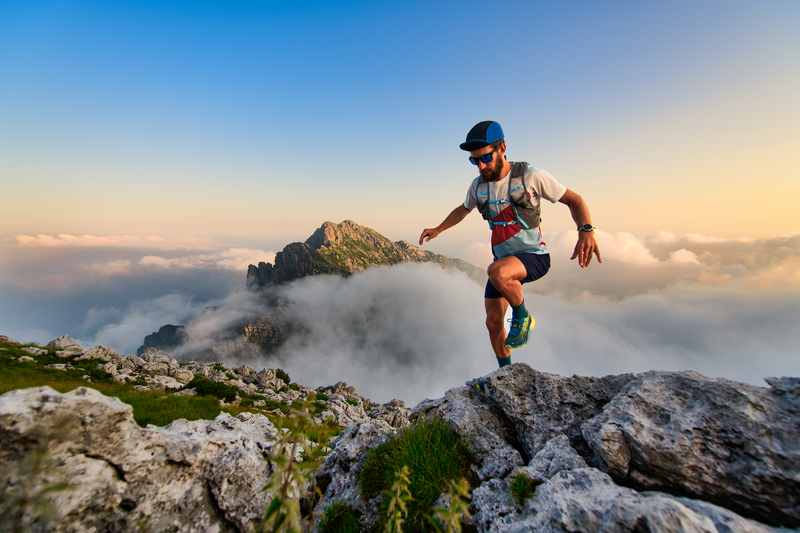
94% of researchers rate our articles as excellent or good
Learn more about the work of our research integrity team to safeguard the quality of each article we publish.
Find out more
ORIGINAL RESEARCH article
Front. Immunol. , 24 June 2021
Sec. Vaccines and Molecular Therapeutics
Volume 12 - 2021 | https://doi.org/10.3389/fimmu.2021.634738
A correction has been applied to this article in:
Expression of concern on: Immunoreactivity of sera from low to moderate malaria-endemic areas against plasmodium vivax rPvs48/45 proteins produced in Escherichia coli and Chinese hamster ovary systems
P48/45 is a conserved gametocyte antigen involved in Plasmodium parasite fertilization. A recombinant Plasmodium vivax P48/45 (Pvs48/45) protein expressed in Escherichia coli (E. coli) was highly antigenic and immunogenic in experimental animals and elicited specific transmission-blocking (TB) antibodies in a previous pilot study. Here, a similar Pvs48/45 gene was expressed in Chinese Hamster Ovary (CHO) cells and we compared its immunoreactivity with the E. coli product. Specific antibody titers were determined using plasma from Colombian individuals (n=227) living in endemic areas where both P. vivax and P. falciparum are prevalent and from Guatemala (n=54) where P. vivax is highly prevalent. In Colombia, plasma seroprevalence to CHO-rPvs48/45 protein was 46.3%, while for E. coli-rPvs48/45 protein was 36.1% (p<0.001). In Guatemala, the sero prevalence was 24.1% and 14.8% (p<0.001), respectively. Reactivity index (RI) against both proteins showed an age-dependent increase. IgG2 was the predominant subclass and the antibody avidity index evaluated by ELISA ranged between 4-6 mol/L. Ex vivo P. vivax mosquito direct membrane feeding assays (DMFA) performed in presence of study plasmas, displayed significant parasite transmission-blocking (TB), however, there was no direct correlation between antibody titers and oocysts transmission reduction activity (%TRA). Nevertheless, DMFA with CHO rPvs48/45 affinity purified IgG showed a dose response; 90.2% TRA at 100 μg/mL and 71.8% inhibition at 10 μg/mL. In conclusion, the CHO-rPvs48/45 protein was more immunoreactive in most of the malaria endemic places studied, and CHO-rPvs48/45 specific IgG showed functional activity, supporting further testing of the protein vaccine potential.
Malaria is transmitted in ~106 countries in tropical and subtropical regions where over 219 million clinical cases and 405,000 deaths were reported in 2018 (1). Whereas P. falciparum is highly prevalent in sub-Saharan Africa, both P. falciparum and P. vivax coexist in vast regions of Latin America, Asia, and Oceania, with many countries displaying greater P. vivax prevalence (2, 3); together these two species are responsible for >90% of the global malaria incidence. In Latin America, 21 countries are malaria-endemic, and in 2018 incidence reached ~632,000 cases; Brazil, Colombia, Peru, and Venezuela accounted for 93% of them (3, 4). Central America experienced a notable malaria reduction (>90%) in the last two decades (2). For example, Guatemala reported 3,521 cases in 2018; most of them (99%) were caused by P. vivax (3) and, together with other countries of the region, reached conditions to initiate malaria elimination programs (5). Elimination efforts would greatly benefit from novel and more cost-effective strategies such as vaccines (6), notably transmission-blocking (TB) vaccines (7). It has been observed that individuals continuously exposed to malaria infection in endemic regions develop immune responses that reduce or completely block Plasmodium transmission from humans to mosquitoes, which would decrease parasite spreading in endemic populations. This TB immunity is essential to develop vaccines that could accelerate malaria elimination (8, 9).
Several antigens are expressed on the surface of Plasmodium gametes (Zygotes/ookinetes) during the fertilization phase in the mosquito midguts (Pfs/Pvs25, Pfs/Pvs28) (10, 11). Others are expressed in gametocytes when present in the human body (Pfs/Pvs48/45, Pfs/Pvs230, Pfs/Pvs27). These antigens have shown the capacity to elicit specific antibodies capable of blocking parasite transmission to mosquitoes and therefore have been identified as potential TB vaccine candidates (11–13). Among them, Pfs25, Pfs230, and Pfs48/45 are three well-established vaccine candidates tested in preclinical (9, 14, 15) and in phase I clinical trials (16–19).
Pvs48/45 is a cysteine-rich conserved protein expressed on the gametocyte surface of several Plasmodium species, and is involved in parasite fertilization (20). A full-length version of this protein was formerly expressed in E. coli using a codon harmonization approach, and a pilot immunoreactivity study demonstrated that this antigen is recognized by ~60% of the sera from malaria-infected patients, apparently in an age-dependent manner (9). Additionally, immunization studies in BALB/c mice and Aotus monkeys indicated high Pvs48/45 immunogenicity and elicited antibodies that recognized the parasite protein in immunofluorescence assays and western blotting (WB) analyses. These antibodies also reduce parasite transmission to mosquitoes in ex-vivo direct DMFA (14). Despite considerable advantages of the E. coli system for recombinant protein production, the accumulation of proteins as inclusion bodies results in the generation of insoluble aggregates of the protein and endotoxin accumulation, among other factors, representing a technical hurdle for expansion of protein production. The CHO cell-line system is commonly used to express large quantities of recombinant proteins (21, 22), and was used here to express the full length Pvs48/45 protein. Immunoreactivity of both E. coli- and CHO-rPvs48/45 proteins was assessed using samples from different malaria-endemic areas of Colombia (COL) and Guatemala (GUA). Additionally, TB activity of anti-CHO-rPvs48/45 specific IgG was determined by ex-vivo DMFA.
This study was conducted using plasma samples from six sites selected from COL and GUA, previously characterized epidemiologically. Malaria epidemiology of Buenaventura, Tierralta, and Tumaco regions in COL, where both P. vivax and P. falciparum are endemic (8, 9), and Escuintla, Alta Verapaz, and Zacapa in GUA with almost exclusive (99%) P. vivax malaria transmission (23) were studied in a previous NIAID-ICEMR program (8).
This study was approved by the Institutional Review Board (IRB) at the Centro Internacional de Vacunas (CECIV, Cali-Colombia) in June 2017 (code CECIV 1506-2017) as well as by the Ethics Committee of the GUA Ministry of Health (CNES-dq-005-2015). Additional approval was obtained in GUA from local community leaders before data collection. Written informed consent (IC) was obtained from each volunteer at enrollment. Minors with ages between 7-18 years signed an informed assent (IA) form, and parents or legal guardians gave the corresponding consent. Donors of P. vivax infected blood for DMFA were also requested to provide IC.
In COL, 227 patients, men (56.8%) and women between 6-84 years of age, were recruited by passive surveillance and included in the study. Subjects were randomly selected among a larger group of patients harboring P. vivax or P. falciparum infections as determined microscopically and confirmed by qPCR. Whole blood samples (5-15mL) were collected in heparin vacutainer tubes by arm venipuncture, and plasma immediately separated by centrifugation. In GUA, 54 patients with ages between 1-70 years of age (38.9% men) with P. vivax infections diagnosed by thick blood smears and confirmed by qPCR, were recruited by active case surveillance, and blood samples were collected as described above. Plasma samples were kept and stored at -80°C until used for serological characterization and for specific CHO-rPvs48/45 antibody affinity purification.
The full-length rPvs48/45 gene was expressed in E. coli, as described before (24, 25). Briefly, the full codon Pvs48/45 gene was harmonized using the EuGene software v0.92 (25) and produced by Integrated DNA Technologies (Skokie, IL) into the IDT Blue vector. The synthetic gene was cloned into the pET32a vector for protein expression in Origami 2 (DE3) E. coli using a heat shock method (24). The protein of ~60kDa in SDS-PAGE, which corresponds to the predicted weight, was purified by affinity chromatography, using immobilized metal ion affinity chromatography with a histidine cobalt resin (Pierce Inc., USA). Endotoxins were removed using high-capacity removal resin (Pierce, USA). A mass spectrometry (LC-MS/MS) analysis of the protein was performed by using an ion triple quadrupole trap LC-MS/MS with 3200 Q-TRAP (Applied Biosystems, Foster City, CA) (24).
The CHO-rPvs48/45 protein was produced by transient gene expression (Excellgene SA, Monthey, Switzerland) with sufficient viable cell culture biomass of suspension adapted CHO-Express™ cells (21). Briefly, the Pvs48/45 codon harmonized gene was produced in CHO cell lines using the pXLG6 as expression vector, containing a signal peptide at the N-terminus, allowing secretion and a His-6 tag at its C-terminus to allow IMAC purification (or Western detection with an anti-6-His antibody). All production cultures (post-transfection) were performed in serum-free, animal protein-free medium (low protein content). A non-malaria related protein, expressed in parallel during the production of the CHO-rPvs48/45 protein, was used as a control production to ensure cell culture and methods accuracy. Each production vessel was verified for critical parameters such as cell culture dynamics and recombinant protein production on samples from day 7 and/or day 14, to confirm the appropriate protein cultures. A total of 150 mg of this antigen was produced after a single purification step on IMAC-FPLC. Two buffer exchanges were performed: one to optimize binding of the protein construct to the IMAC column, and the other one, after elution, to remove excess imidazole. SDS-PAGE analysis of both E. coli- and CHO-rPvs48/45 proteins under both reducing (0.05 mol/L dithiothreitol) and non-reducing conditions together with immunoblot and mass spectrometry (LC-MS/MS) confirmed the protein identity. Immunoblot analysis was carried out using sera (1:200 dilution) of P. vivax semi-immune subjects, plasma from Aotus monkeys previously immunized with the E. coli-rPvs48/45 protein (1:200 dilution), and a E. coli-rPvs48/45 monoclonal antibody; normal human serum was used as control and LC/MS/MS analysis was performed using peptides generated by trypsin digestion. Peptides were separated and analyzed using a Qexactive-HFX coupled to U3000 RSLC (Thermofisher). Mass spectra were processed with MaxQuant (v1.6.10.43) using a database consisting of Uniprot entries of Cricetulus griseus (UP000001075, 2020_06), 250 classical contaminants and the sequence of the construction.
ELISA was used to determine the two rPvs48/45 proteins’ immunoreactivity, as described before (24, 26). Briefly, 96-well plates (Nunc-Immuno Plate, Maxisorp, Roskilde, Denmark) were coated with 1 µg/mL of the two rPvs48/45 protein versions in 1x phosphate buffer saline (PBS), pH 7.4 at 4°C overnight. After plates were blocked with milk solution 5%, plasma samples diluted at 1:200 were added and incubated for 1 hour followed by incubation with alkaline phosphatase (1:1000) conjugated goat anti-human IgG antibody (Sigma Chemical Co., St Louis, MO) for 1 hour. Reactions were revealed with para-nitrophenyl phosphate substrate (p-NPP) (Sigma Aldrich) and read at 405 nm wavelength (Dynex Technologies, Inc., MRX Chantilly, VA). Cut-off points for ELISA were calculated as three SD above the mean absorbance value at 405 nm of sera from healthy adult volunteers who had never been exposed to malaria (n=60). The results were also expressed as reactivity index (RI) defined as OD values of tested samples divided by the cut-off value. P-value < 0.05 was considered significant.
A pool of selected COL plasma samples known to contain anti-P. vivax CHO-rPvs48/45 antibodies with ELISA reactive index > 5.0, as described elsewhere (8) was used for purification of anti-CHO-rPvs48/45-specific IgG. Briefly, total IgG was purified using a two-step procedure: protein G columns (Pierce, Inc., Rockford, IL), followed by anti-CHO-rPvs48/45-specific antibodies purification using an NHS-activated Sepharose column (GE Healthcare Life Sciences, Buckinghamshire, UK) coupled with CHO-rPvs48/45 protein, as described previously. Affinity adsorption was performed using the purified total IgG, and elution fraction was neutralized by 1 mol/L Tris, pH 9.0. Specific IgG was then dialyzed against 1x PBS using an Amicon Ultra-4 Centrifugal Filter Unit (30 kDa membrane EMD Millipore). The final protein concentration of specific IgG was measured by DS-11FX+ (DeNovix Inc. Wilmington, DE, USA) and adjusted to 0.5 mg/mL (19). A pool of normal human plasma samples was made from 20 donors, then processed in the same way and used as negative control. Standardized Elisa test was used for quantification of anti-CHO-rPvs48/45-specific antibodies (Elisa Units - EU) as described before (27).
Affinity-purified human anti-CHO-rPvs48/45 IgG was tested by competition ELISA to determine antibody specificity. Briefly, ELISA microplates were coated with 1 μg/mL of the CHO-rPvs48/45 protein and then incubated with the purified antibody; 10 μg/mL of IgG was mixed with increasing concentrations (0 to 100 μg/mL) of the CHO-rPvs48/45 recombinant protein competitor. The reaction was incubated for 30 min at RT and the reactivity of the antibody preparation was determined by ELISA as previously described (28). Results were expressed as a percent of binding inhibition of wells without a competitor.
Affinity-purified anti-CHO-rPvs48/45 IgG antibodies and four plasma samples from P. vivax infected donors (MPV 139: Tierralta, HPV 3031 and P 137: Buenaventura, and P72: Quibdó) displaying ELISA reactivity indices (RI) > 5.0 and four plasma sera from the same regions with RI between 2.0-3.0 (P76, P89, P95, P135) were selected to determine antibody avidity. Polystyrene microplates were coated with 1 μg/mL of the CHO-rPvs48/45 protein in duplicates. After overnight incubation plates were washed and blocked with 100 μL of PBS with 0.5% Tween (PBS-T) per well, 5% skim milk (Gibco™, USA) for 2 h at room temperature (RT). Specific IgG at 10 μg/mL or plasma samples diluted at 1:200 in PBS-T 2.5% skim milk was added to the plates and incubated for 2h at RT. After washing, plates were incubated with different urea concentrations (from 0 to 7 mol/L) in duplicates for 15 min to determine antibody avidity. The reaction was revealed using an anti-human IgG alkaline phosphatase conjugate as described above. The urea concentration resulting in 50% of the original ELISA units (IC50) was calculated using linear regression (19).
The rPvs48/45-specific IgG subclasses were determined using both CHO and E. coli proteins by ELISA as described elsewhere (26). Briefly, 1.0 μg/mL of the corresponding protein was used as antigen to coat microplates, and after blocking with PBS-T plus fat-free milk, plasma sample dilutions were then incubated for 1 hr. Twenty plasma samples were randomly selected from malaria-endemic areas of Colombia, and the purified CHO-rPvs48/45-specific human IgG was tested. Plates were washed with PBS-T and then incubated with the following mouse anti-human IgG subclass-specific antibodies: anti-IgG1, IgG3, and IgG4 (Skibio, Bedfordshire, England) and anti-IgG2 (Sigma, St. Louis, MO). Then a peroxidase-conjugated goat anti-mouse IgG antibody (Kierkegaard and Perry Laboratories, Gaithersburg, MD) was added, and the reaction read at 450 nm.
The TB activity of 143 plasma samples (1:2 dilution), and affinity-purified CHO-rPvs48/45 specific IgG antibodies at 10, 40, and 100 μg/mL was measured by a DMFA using P. vivax gametocyte-infected blood obtained from malaria patients, as previously described (9). Briefly, P. vivax infected blood was centrifuged, and cell-pellets containing infected RBC (iRBC) were subsequently reconstituted with a pool of heat-inactivated male AB+ sera obtained from healthy donors purchased from Sigma (H5667 Sigma-Aldrich Inc) or with test samples. A total of ~100 Anopheles albimanus mosquitoes previously subjected to overnight fasting were fed for 15-20 minutes/cage with this mixture. Mosquito midguts were stained with 2% mercurochrome on day 7 post-feeding, and the number of oocysts per mosquito midgut counted.
All statistical tests were performed in Prism 6 (GraphPad Software), and p-values <0.05 were considered significant. Antibody responses (seroprevalence and percentage of RI>2) for both CHO-rPvs48/45 and E. coli- were compared by Fisher’s exact test. Differences of RI between age groups were analyzed by Kruskal-Wallis, followed by Dunn’s multiple comparison test. Correlation between %TRA of human sera and RI by Spearman test. Avidity (IC50) of P. vivax plasma samples and affinity-purified CHO-rPvs48/45 specific IgG were compared by Mann-Whitney tests (20).
The percentage reduction in mean oocyst count/mosquitoes (TRA) was calculated using the following formula: [(Xc − Xa)/Xc)] × 100, where X is the arithmetic mean oocysts in control (c) and test (a) plasma or IgG. The 95% confidence interval and p-value for TRA was calculated using a zero-inflated negative binomial model as previously described (29).
Amplification of the E. coli-rPvs48/45 gene without the signal peptide and GPI anchor was sub-cloned in the E. coli system as described elsewhere (24). Both the affinity-purified CHO- and the E. coli-rPvs48/45 protein products were analyzed by SDS-PAGE under non-reducing conditions, indicating the presence of a ~50kDa and ~60kDa bands, respectively, corresponding to the expected product (Figure 1A) as previously described for P48/45 proteins (24, 30). The different MW of the two proteins is due to: 1) an additional thioredoxin protein fragment added to the E. coli protein (108 aa) inserted to avoid inclusion bodies formation (24, 31) whereas the CHO-rPvs48/45 has a shorter thioredoxin fragment (42aa), and a theoretical mass of 51,766.22 (see Supplementary Material). Both proteins have a ~89% similar sequence and were both recognized by sera from P. vivax malaria semi-immune subjects as well as from plasma of Aotus monkeys immunized with the E. coli-rPvs48/45 protein (Figure 1B: lane 1 and 2), and confirm the similarity of both proteins and previous results (24). In addition, a lower molecular band at the 25kDa appeared in the E. coli product in both reduced and non-reducing conditions and the western blot analysis under non-reducing conditions of this protein showed the recognition of this lower band by both a mouse monoclonal and a primate polyclonal anti-rPvs48/45 antibody (Figure 1B: lanes 3 and 4).
Figure 1 (A) 12% SDS-PAGE gel of CHO- and E. coli-rPvs48/45 proteins stained with Coomassie blue. Lane 1, molecular weight markers. Lane 2 and 3, CHO-rPvs48/45 protein (~ 50 kDa) under non-reducing and reducing conditions (0.05 M dithiothreitol) respectively. Line 4 and 5, E. coli-rPvs48/45 proteins (~ 60 kDa) under non-reducing and reducing conditions, respectively. (B) Western blot analysis under non-reducing conditions of the CHO-rPvs48/45 protein against PBS solution. Lane 1, Normal human sera, lane 2 sera from P. vivax malaria immune subject, lane 3, Normal monkey plasma, lane 4, Blank, lane 5, monkey plasma immunized with E coli-rPvs48/45 from a previous study (25). Lane 6, 7 and 8 western blot analysis under non-reducing conditions of the E. coli-rPvs48/45 protein using a mouse monoclonal, a primate polyclonal anti-rPvs48/45 antibody and Normal monkey plasma respectively. Lane 9, molecular weight markers.
From the total plasma samples studied (n= 281), ELISA assays indicated the presence of specific antibodies to the full-length CHO-rPvs48/45 in 42% (n=118) of samples and in 32% (n=90) to the E. coli-rPvs48/45 product (p<0.001) (Table 1). Immunoreactivity against both proteins was higher in the COL than in the GUA samples (CHO, p=0.0030, and E. coli, p=0.0026). In COL plasmas (n=227) the prevalence of specific antibodies was 46.3% and 36.1% to the CHO and E. coli products, respectively. In contrast, in GUA plasmas (n=54) it was 24.1% and 14.8%, respectively, indicating significant regional differences for both proteins (CHO and E. coli p<0.001). Also, samples from Tierralta, (COL), where P. vivax is highly prevalent (2,630 cases/100,000 habitants; 65% P. vivax), presented an immunoreactivity of 58.8% and 34.3% against the CHO and E. coli proteins, respectively. In contrast, samples from Tumaco, where P. falciparum is more prevalent (1,338 cases/100,000 habitants; 96.2%) than P. vivax (INS, 2018), ELISA showed 27.3% and 41.7%, respectively. Significant differences in prevalence among both proteins in samples from COL (Tierralta: p<0.001, Buenaventura: p<0.002) and GUA samples (p<0.001) was observed. In contrast, in Tumaco (COL) E. coli was better recognized than CHO (p<0.001) (Table 1). In Colombia, the seroprevalence of samples with RI > 2.0 was found in 9.7% (n=22) of the samples tested with the CHO protein, and 4.0% (n=9) in those tested with the E. coli protein (p<0.001). In GUA, people with > 2.0 RI were 8% (n=1) and 0% (n=0) to CHO and E. coli protein, respectively. Fisher tests indicated that plasma samples with RI > 2 were significantly more frequent in Colombia than in GUA (p=0.0042) to CHO protein. In contrast, there was no difference between both countries to E. coli protein. To determine the impact of age on the RI, in both COL and GUA, samples were stratified into three groups: 0-14 years/old n=41 (14.6%); 15-30 year/old n=118 (42%); and more than >30 years/old, n=122 (43.4%). The mean RI presented an age-dependent increasing trend (Figures 2A, B), which was more significant when plasma samples were tested using the CHO-rPvs48/45 protein (p<0.05). Individuals >30 years/old presented a RI to CHO mean of 1.45 whereas to E. coli, the mean was 1.13. Significant differences (p<0.05) were observed in the RI values between children (<15 years/old) and adults (>30) when the E. coli - rPvs48/45 protein was used (CHO = 0.86; E. coli = 0.81). Regarding TRA activity, 93 of the 143 (65.03%) plasma samples examined by DMFA displayed >80% TRA; 51samples (54.83%) were reactive to the CHO- and 29 (31.18%) reactive to E. coli-rPvs48/45 protein (Figure 2E). As shown in Figures 2C, D no correlation was found between % TRA activity and RI values in both proteins (Spearman test; CHO protein p>0.28 and E. coli p>0.63).
Table 1 Prevalence of antibodies reactive to CHO-rPvs48/45 and E. coli in plasma samples of subjects from Colombia and Guatemala.
Figure 2 Age-dependent reactivity index (A, B), and correlation between percentage inhibition in oocyst density (%TRA) of human sera and reactivity index of COL and GUA (C, D) to rPvs48/45 expressed in both CHO (A, C) and E. coli (B, D). (A, B) the bars and error bars correspond to mean values and 2SD. Statistical significance was observed between each age group in both proteins (p < 0.0001) by Kruskal Wallis analysis followed by Dunn’s multiple comparison tests between two groups. Bars indicated significant difference between groups of 0-14 and >30 years of age (***p < 0.0001; **p < 0.05). (C, D) show no correlation between %TRA and RI (CHO protein p>0.28 and E. coli p > 0.63) by Spearman tests. (E) A dot blotting chart showing the reactivity index of each sera for both proteins.
To investigate characteristics and functionality of the protein, specific antibodies were affinity purified from a pool of plasmas with high RI (>5.0) using the CHO rPvs48/45 protein. A total of 40 mg/mL of total IgG was obtained after protein G column purification and 500ug/mL of anti-CHO-rPvs48/45-specific antibodies after Sepharose column purification with specific antibody titers of 62.163 EU (27). Specificity of anti-CHO-rPvs48/45 specific antibodies was confirmed by ELISA competitive assay as shown in Figure 3A. At 50 μg/mL, the CHO rPvs48/45 competitor protein induced a remarkable inhibition when the purified specific-IgG was used at 10 μg/mL. i.e., ~80% of inhibition. In the IgG subclass analysis, IgG2 was the most abundant, both in affinity-purified anti-CHO-rPvs48/45 antibody preparation, as well in the plasma of donors with positive antibody titers to both CHO- (n=21) (Figure 3C) and E. coli-rPvs48/45 proteins (n=22) (Figure 3D).
Figure 3 (A) ELISA competition assay for the affinity-purified CHO-rPvs48/45-specific human IgG (▪) and normal IgG (•) against CHO-rPvs48/45 protein. Plates were coated with CHO-rPvs48/45 recombinant protein (1 μg/mL) and then a test IgG (10 μg/mL) was incubated together with the recombinant protein at different concentrations (0 to 100 μg/mL). (B) The IC50 mean of purified IgG and sera samples were determined by ELISA with increasing urea concentration (from 0 to 7 mol/L). The serum samples were selected for presenting the highest reactivity index (RI>5) of the sampling location: MPV 139 (Tierra Alta), HPV 3031 and P 137 (Buenaventura), and P72 (Quibdó) and reactivity index between 2-4: P76, P89, P95, P135 from the same regions. Anti-IgG1, IgG2 IgG3, and IgG4 subclasses are reported as OD mean of COL plasma samples (black symbols) randomly chosen and purified CHO-rPvs48/45-specific human IgG (grey symbols) using plates coated with either CHO- (C) or E. coli-rPvs48/45 (D) protein.
To determine the protein avidity, anti-CHO- and E. coli-rPvs48/45 specific IgG and sera samples from malaria-endemic regions were incubated with different concentrations of urea. All data sets fitted into the linear regression model (R2>0.94), and the anti-rPvs48/45 specific IgG presented a similar IC50 (4.1 and 4.5 mol/L respectively) with plasma samples with RI between 2-4 (P-76: 4.1 mol/L, P-89: 4.3 mol/L, P-95: 4.4 mol/L) and a slightly higher avidity with the HPV-3031 (5.5 mol/L), P-137 (5.6 mol/L), and P-72 (4.9 mol/L) of plasma samples with RI>5.0, except with the sample MPV-139 (3.9 mol/L) (Figure 3B).
Two independent DMFA assays were performed to determine oocyst reduction by specific IgG. CHO-rPvs48/45-specific IgG showed significant reduction in the mean oocyst numbers, compared to normal human IgG; and the inhibition was dose-dependent (Table 2). TRA increased from 71.8% to 90.2% when the IgG concentration was increased from 10 to 100 μg/mL.
Table 2 DMFA with human anti-CHO-rPvs48/45-specific IgGa.
This study confirms that plasma of a significant proportion of individuals living in malaria endemic areas of COL and GUA recognize the Pvs48/45, independently of the recombinant product used as antigen in the ELISA and about half of these plasma samples had the capacity to ex vivo block plasmodium transmission to Anopheles mosquitoes.
The results here where both P. vivax and P. falciparum coexist in most endemic regions are consistent with those obtained with the P. falciparum orthologous protein (Pfs48/45) in studies conducted in several countries in Africa where this species is almost exclusively transmitted and malaria transmission intensity is significantly higher than in Latin America (29, 32–34). In this study, we included GUA, where malaria is virtually produced exclusively by P. vivax, and COL where the study sites have transmission of both P. vivax and P. falciparum, in different proportions (35, 36). Likewise, it confirms previous results on the immunoreactivity of the rPvs48/45 expressed in E. coli and the ex vivo TB activity studied of plasma samples from endemic areas of COL (24) and from Africa (37).
The frequent presence of antibodies to rPvs48/45 in the study sites, known to be regions of low to intermediate malaria transmission intensity, is encouraging as it indirectly reflects the protein’s immunoreactivity in natural conditions, even when parasite exposure is low or moderate. Nevertheless, we found that the protein recognition is associated with the malaria transmission intensity in the study areas. i.e., it was higher in COL (106 cases/100,000 habitants) than in GUA (22 cases/100,000 habitants) in the year 2017 (PAHO, 2020). In this regard, in COL, immunoreactivity was higher and more prevalent in areas with the relatively higher transmission, e.g., in Tierralta (2,630 cases/100,000 habitants), Department of Cordoba (38). However, it is intriguing that in GUA that has reduced the malaria incidence by ~95% since 2000 (5, 23), individuals from endemic regions still present significant immunoreactivity with rPvs48/45. Colombia presented an incidence reduction of ~50% between 2000 and 2015 but still reports >60,000 clinical cases/year with >60% of the cases reported in the study areas, the Pacific coastal region and Cordoba department (39). This is coherent with the age-dependent trend of specific antibodies; subjects >30 years of age showed the highest RI against the rPvs48/45 protein.
Although both proteins were highly immunoreactive, CHO was significantly better recognized by all age groups, except in Tumaco (COL) where E. coli was significantly better recognized. The different immunoreactivity of the two proteins might be explained by 1) the conformational differences between the 3D structures of the two proteins and, thus, recognition of different epitopes, 2) by the additional thioredoxin fragment added to the E. coli protein to improve its expression, 3) by potential glycosylation in the CHO protein.
None of the constructs were recognized by sera from naïve individuals indicating that neither full-length Pvs48/45and nor the additional fragment are recognized by control sera.
Another intriguing observation is that in Tumaco (COL) the prevalence of P. falciparum is significantly higher that P. vivax, yet, the response to rPvs48/45 was similar than in Tierralta (COL) and GUA suggesting a parasite cross-reactivity between the orthologous proteins in natural conditions. To better understand the sero-epidemiology of the rPvs48/45, we are currently studying malaria-endemic regions with different P. vivax/P. falciparum proportions.
Although TB activity displayed by whole plasma can be the result of antibodies to multiple parasite antigens (Figures 2C, D), here, strong inhibition was induced by affinity purified anti-CHO-rPvs48/45 antibodies which confirmed the important role of this parasite antigen in TB immunity under natural conditions (8, 9). It is also remarkable that the DMFA performed with the affinity-purified antibodies displayed TRA dose responses (Table 2). This is an indirect indication, first, of the correct conformation of the protein and second, that anti-rPvs48/45 antibodies induced by natural infection retained the functional activity after purification.
The two proteins displayed different profile at the SDS-PAGE gel by the presence of two bands of high (~50kDa) and a low (~25kDa) molecular weight in the E. coli product. Previous studies using both recombinant and natural proteins have observed the same pattern (40, 41). Here it was confirmed that the smaller fragment corresponds to a proteolytic product of the protein by the blot reactivity with both a monoclonal and a polyclonal anti-Pvs48/45 antibody. Therefore, as this protein develops as vaccine candidate purification process must be optimized.
Although several IgG subclasses may contribute to the overall TB activity, the high prevalence of IgG2 suggests that anti-rPvs48/45 activity is independent of the classical complement; this issue requires further analyses.
The results described here, together with the progress achieved with the Pfs48/45 vaccine candidate (42, 43) and previous results of sera from mice and monkeys previously immunized with the rPvs48/45 E. coli version encourage further studies on the vaccine potential of this P. vivax protein. It is of great importance to conduct studies using plasma from areas where P. falciparum is exclusively transmitted, i.e., Africa, which may contribute to elucidate the potential cross-reactivity of the protein in the field. If the cross reactivity in nature were accompanied by cross functional activity, i.e. TB activity, it would be of great importance for vaccine development.
The raw data supporting the conclusions of this article will be made available by the authors, without undue reservation.
The studies involving human participants were reviewed and approved by Institutional Review Board (IRB) at the Centro Internacional de Vacunas (CECIV, Cali-Colombia) (code CECIV 1506-2017) Ethics Committee of the GUA Ministry of Health (CNES-dq-005-2015). Written informed consent to participate in this study was provided by the participants’ legal guardian/next of kin.
Conceived and designed the experiments: MA-H and SH. Performed the experiments: NC, CE, KM, ES, AC, JR, and AM. Analyzed the data: MA-H, SH, GC, and CL. LC/MS/MS CHO-rPvs48/45 analysis: AK and GC. Contributed reagents/materials/analysis tools: MA-H, NC, and SH. Wrote the paper: MA-H, NC, GC, CL, and AK. All authors contributed to the article and approved the submitted version.
NIH/NIAID 1R01AI121237-01 sponsored this study and in part by the Intramural Research Program of NIAID, NIH.
The Supplementary Material for this article can be found online at: https://www.frontiersin.org/articles/10.3389/fimmu.2021.634738/full#supplementary-material
The authors declare that the research was conducted in the absence of any commercial or financial relationships that could be construed as a potential conflict of interest.
The authors are grateful for the participation of the community from malaria-endemic regions of Colombia and other staff members of the Center (A. Amado, AF. Chamorro and F. Meneses). We thank S. Urbach and D. Xirodimas for assistance with mass spectrometry measurements. Mass spectrometry experiments were carried out using the facilities of the Montpellier Proteomics Platform (PPM, BioCampus Montpellier).
2. Ferreira MU, Castro MC. Malaria Situation in Latin America and the Caribbean: Residual and Resurgent Transmission and Challenges for Control and Elimination. Methods Mol Biol (2019) 2013:57–70. doi: 10.1007/978-1-4939-9550-9_4
3. PAHO. Situation of Malaria in the Region of the Americas 2017-2019 (2017). Available at: https://wwwpahoorg.
4. Recht J, Siqueira AM, Monteiro WM, Herrera SM, Herrera S, Lacerda MVG. Malaria in Brazil, Colombia, Peru and Venezuela: Current Challenges in Malaria Control and Elimination. Malar J (2017) 16(1):273–90. doi: 10.1186/s12936-017-1925-6
5. Herrera S, Ochoa-Orozco SA, Gonzalez IJ, Peinado L, Quinones ML, Arevalo-Herrera M. Prospects for Malaria Elimination in Mesoamerica and Hispaniola. PloS Negl Trop Dis (2015) 9(5):e0003700. doi: 10.1371/journal.pntd.0003700
6. Duffy PE, Patrick Gorres J. Malaria Vaccines Since 2000: Progress, Priorities, Products. NPJ Vaccines (2020) 5:1–9. doi: 10.1038/s41541-020-0196-3
7. Ishino T, Tsuboi T. Progress Toward a Transmission-Blocking Vaccine Against Malaria. Lancet Infect Dis (2018) 18(9):927–8. doi: 10.1016/S1473-3099(18)30358-X
8. Arevalo-Herrera M, Solarte Y, Rocha L, Alvarez D, Beier JC, Herrera S. Characterization of Plasmodium Vivax Transmission-Blocking Activity in Low to Moderate Malaria Transmission Settings of the Colombian Pacific Coast. Am J Trop Med Hyg (2011) 84(2 Suppl):71–7. doi: 10.4269/ajtmh.2011.10-0085
9. Arevalo-Herrera M, Solarte Y, Zamora F, Mendez F, Yasnot MF, Rocha L, et al. Plasmodium Vivax: Transmission-Blocking Immunity in a Malaria-Endemic Area of Colombia. Am J Trop Med Hyg (2005) 73(5 Suppl):38–43. doi: 10.4269/ajtmh.2005.73.5_suppl.0730038
10. Kapulu MC, Da DF, Miura K, Li Y, Blagborough AM, Churcher TS, et al. Comparative Assessment of Transmission-Blocking Vaccine Candidates Against Plasmodium Falciparum. Sci Rep (2015) 5:11193–207. doi: 10.1038/srep11193
11. Menon V, Kapulu MC, Taylor I, Jewell K, Li Y, Hill F, Long CA, et al. Assessment of Antibodies Induced by Multivalent Transmission-Blocking Malaria Vaccines. Front Immunol (2017) 8:1998–2009. doi: 10.3389/fimmu.2017.01998
12. Acquah FK, Adjah J, Williamson KC, Amoah LE, et al. Transmission-Blocking Vaccines: Old Friends and New Prospects. Infect Immun (2019) 87(6):1–19. doi: 10.1128/IAI.00775-18
13. Coelho CH, Rappuoli R, Hotez PJ, Duffy PE. Transmission-Blocking Vaccines for Malaria: Time to Talk About Vaccine Introduction. Trends Parasitol (2019) 35(7):483–6. doi: 10.1016/j.pt.2019.04.008
14. Arevalo-Herrera M, Solarte Y, Yasnot MF, Castellanos A, Rincon A, Saul A, et al. Induction of Transmission-Blocking Immunity in Aotus Monkeys by Vaccination With a Plasmodium Vivax Clinical Grade PVS25 Recombinant Protein. Am J Trop Med Hyg (2005) 73(5 Suppl):32–7. doi: 10.4269/ajtmh.2005.73.32
15. Herrera S, Bonelo A, Perlaza BL, Fernandez OL, Victoria S, Lenis AM, et al. Safety and Elicitation of Humoral and Cellular Responses in Colombian Malaria-Naive Volunteers by a Plasmodium Vivax Circumsporozoite Protein-Derived Synthetic Vaccine. Am J Trop Med Hyg (2005) 73(5 Suppl):3–9. doi: 10.4269/ajtmh.2005.73.3
16. Chichester JA, Green BJ, Jones RM, Shoji Y, Miura K, Long CA, et al. Safety and Immunogenicity of a Plant-Produced Pfs25 Virus-Like Particle as a Transmission Blocking Vaccine Against Malaria: A Phase 1 Dose-Escalation Study in Healthy Adults. Vaccine (2018) 36(39):5865–71. doi: 10.1016/j.vaccine.2018.08.033
17. Sagara I, Healy SA, Assadou MH, Gabriel EE, Kone M, Sissoko K, et al. Safety and Immunogenicity of Pfs25H-EPA/Alhydrogel, a Transmission-Blocking Vaccine Against Plasmodium Falciparum: A Randomised, Double-Blind, Comparator-Controlled, Dose-Escalation Study in Healthy Malian Adults. Lancet Infect Dis (2018) 18(9):969–82. doi: 10.1016/S1473-3099(18)30344-X
18. Talaat KR, Ellis RD, Hurd J, Hentrich A, Gabriel E, Hynes NA, et al. Safety and Immunogenicity of Pfs25-EPA/Alhydrogel(R), a Transmission Blocking Vaccine Against Plasmodium Falciparum: An Open Label Study in Malaria Naive Adults. PloS One (2016) 11(10):e0163144. doi: 10.1371/journal.pone.0163144
19. Wu Y, Ellis RD, Shaffer D, Fontes E, Malkin Y, Mahanty S, et al. Phase 1 Trial of Malaria Transmission Blocking Vaccine Candidates Pfs25 and Pvs25 Formulated With Montanide ISA 51. PloS One (2008) 3(7):e2636. doi: 10.1371/journal.pone.0002636
20. Vallejo AF, Garcia J, Amado-Garavito AB, Arevalo-Herrera M, Herrera S. Plasmodium Vivax Gametocyte Infectivity in Sub-Microscopic Infections. Malar J (2016) 15:48–56. doi: 10.1186/s12936-016-1104-1
21. Ritacco FV, Wu Y, Khetan A. Cell Culture Media for Recombinant Protein Expression in Chinese Hamster Ovary (CHO) Cells: History, Key Components, and Optimization Strategies. Biotechnol Prog (2018) 34(6):1407–26. doi: 10.1002/btpr.2706
22. Thoring L, Dondapati SK, Stech M, Wustenhagen DA, Kubick S. High-Yield Production of “Difficult-to-Express” Proteins in a Continuous Exchange Cell-Free System Based on CHO Cell Lysates. Sci Rep (2017) 7(1):11710–24. doi: 10.1038/s41598-017-12188-8
23. Lennon SE, Miranda A, Henao J, Vallejo AF, Perez J, Alvarez A, et al. Malaria Elimination Challenges in Mesoamerica: Evidence of Submicroscopic Malaria Reservoirs in Guatemala. Malar J (2016) 15(1):441–9. doi: 10.1186/s12936-016-1500-6
24. Arevalo-Herrera M, Chowdhury DR, Angov E, Kariuki T, Kumar N. Recombinant Pvs48/45 Antigen Expressed in E. Coli Generates Antibodies That Block Malaria Transmission in Anopheles Albimanus Mosquitoes. PloS One (2015) 10(3):e0119335. doi: 10.1371/journal.pone.0119335
25. Chowdhury DR, Angov E, Kariuki T, Kumar N. A Potent Malaria Transmission Blocking Vaccine Based on Codon Harmonized Full Length Pfs48/45 Expressed in Escherichia Coli. PloS One (2009) 4(7):e6352. doi: 10.1371/journal.pone.0006352
26. Herrera S, Fernández OL, Vera O, Cárdenas W, Ramírez O, Palacios R, et al. Phase I Safety and Immunogenicity Trial of Plasmodium Vivax CS Derived Long Synthetic Peptides Adjuvanted With Montanide ISA 720 or Montanide ISA 51. Am J Trop Med Hyg (2011) 84(2 Suppl):12–20. doi: 10.4269/ajtmh.2011.09-0516
27. Miura K, Orcutt AC, Muratova OV, Miller LH, Saul A, Long CA. Development and Characterization of a Standardized ELISA Including a Reference Serum on Each Plate to Detect Antibodies Induced by Experimental Malaria Vaccines. Vaccine (2008) 26(2):193–200. doi: 10.1016/j.vaccine.2007.10.064
28. Arevalo-Herrera M, Roggero MA, Gonzalez JM, Vergara J, Corradin M, Lopez JA, et al. Mapping and Comparison of the B-cell Epitopes Recognized on the Plasmodium Vivax Circumsporozoite Protein by Immune Colombians and Immunized Aotus Monkeys. Ann Trop Med Parasitol (1998) 92(5):539–51. doi: 10.1080/00034989859230
29. Miura K, Swihart BJ, Deng B, Zhou L, Pham TP, Diouf A, et al. Transmission-Blocking Activity is Determined by Transmission-Reducing Activity and Number of Control Oocysts in Plasmodium Falciparum Standard Membrane-Feeding Assay. Vaccine (2016) 34(35):4145–51. doi: 10.1016/j.vaccine.2016.06.066
30. Rener J, Graves PM, Carter R, Williams JL, Burkot TR. Target Antigens of Transmission-Blocking Immunity on Gametes of Plasmodium Falciparum. J Exp Med (1983) 158(3):976–81. doi: 10.1084/jem.158.3.976
31. LaVallie ER, Lu Z, Diblasio-Smith EA, Collins-Racie LA, Mccoy JM. Thioredoxin as a Fusion Partner for Production of Soluble Recombinant Proteins in Escherichia Coli. Methods Enzymol (2000) 326:322–40. doi: 10.1016/S0076-6879(00)26063-1
32. Bousema T, Roeffen W, Meijerink H, Mwerinde H, Mwakalinga S, Van Gemert GJ. The Dynamics of Naturally Acquired Immune Responses to Plasmodium Falciparum Sexual Stage Antigens Pfs230 & Pfs48/45 in a Low Endemic Area in Tanzania. PloS One (2010) 5(11):e14114. doi: 10.1371/journal.pone.0014114
33. Jones S, Grignard L, Nebie I, Chilongola J, Dodoo D, Sauerwein R, et al. Naturally Acquired Antibody Responses to Recombinant Pfs230 and Pfs48/45 Transmission Blocking Vaccine Candidates. J Infect (2015) 71(1):117–27. doi: 10.1016/j.jinf.2015.03.007
34. Ouedraogo AL, Roeffen W, Luty AJ, De Vlas SJ, Nebie I, Ilboudo-Sanogo E, et al. Naturally Acquired Immune Responses to Plasmodium Falciparum Sexual Stage Antigens Pfs48/45 and Pfs230 in an Area of Seasonal Transmission. Infect Immun (2011) 79(12):4957–64. doi: 10.1128/IAI.05288-11
35. Chaparro P, Padilla J, Vallejo AF, Herrera S, et al. Characterization of a Malaria Outbreak in Colombia in 2010. Malar J (2013) 12:330–40. doi: 10.1186/1475-2875-12-330
36. Feged-Rivadeneira A, Angel A, Gonzalez-Casabianca F, Rivera C. Malaria Intensity in Colombia by Regions and Populations. PloS One (2018) 13(9):e0203673. doi: 10.1371/journal.pone.0203673
37. Bansal GP, Vengesai A, Cao Y, Mduluza T, Kumar N. Antibodies Elicited During Natural Infection in a Predominantly Plasmodium Falciparum Transmission Area Cross-React With Sexual Stage-Specific Antigen in P. Vivax. Acta Trop (2017) 170:105–11. doi: 10.1016/j.actatropica.2017.02.032
38. Instituto Nacional de Salud. Boletín Epidemiologico Semanal No. 52 Colombia. Ministerio de Salud (2017). https://www.ins.gov.co
39. Instituto Nacional de Salud. Boletín Epidemiologico Semanal No. 52 Colombia. Ministerio de Salud (2018). https://www.ins.gov.co
40. Cao Y, Bansal GP, Merino K, Kumar N, et al. Immunological Cross-Reactivity Between Malaria Vaccine Target Antigen P48/45 in Plasmodium Vivax and P. Falciparum and Cross-Boosting of Immune Responses. PloS One (2016) 11(7):e0158212. doi: 10.1371/journal.pone.0158212
41. Outchkourov N, Vermunt A, Jansen J, Kaan A, Roeffen W, Teelen K, et al. Epitope Analysis of the Malaria Surface Antigen pfs48/45 Identifies a Subdomain That Elicits Transmission Blocking Antibodies. J Biol Chem (2007) 282(23):17148–56. doi: 10.1074/jbc.M700948200
42. Chaturvedi N, Bharti PK, Tiwari A, Singh N, et al. Strategies & Recent Development of Transmission-Blocking Vaccines Against Plasmodium Falciparum. Indian J Med Res (2016) 143(6):696–711. doi: 10.4103/0971-5916.191927
Keywords: malaria, Plasmodium vivax, gametocytes, transmission blocking, Pvs48/45, vaccines
Citation: Arévalo-Herrera M, Miura K, Cespedes N, Echeverry C, Solano E, Castellanos A, Ramirez JS, Miranda A, Kajava AV, Long C, Corradin G and Herrera S (2021) Immunoreactivity of Sera From Low to Moderate Malaria-Endemic Areas Against Plasmodium vivax rPvs48/45 Proteins Produced in Escherichia coli and Chinese Hamster Ovary Systems. Front. Immunol. 12:634738. doi: 10.3389/fimmu.2021.634738
Received: 28 November 2020; Accepted: 25 May 2021;
Published: 24 June 2021.
Edited by:
Pedro A. Reche, Complutense University of Madrid, SpainReviewed by:
Gabriel M. Gutierrez, Leidos, United StatesCopyright © 2021 Arévalo-Herrera, Miura, Cespedes, Echeverry, Solano, Castellanos, Ramirez, Miranda, Kajava, Long, Corradin and Herrera. This is an open-access article distributed under the terms of the Creative Commons Attribution License (CC BY). The use, distribution or reproduction in other forums is permitted, provided the original author(s) and the copyright owner(s) are credited and that the original publication in this journal is cited, in accordance with accepted academic practice. No use, distribution or reproduction is permitted which does not comply with these terms.
*Correspondence: Myriam Arévalo-Herrera, marevalo@inmuno.org
Disclaimer: All claims expressed in this article are solely those of the authors and do not necessarily represent those of their affiliated organizations, or those of the publisher, the editors and the reviewers. Any product that may be evaluated in this article or claim that may be made by its manufacturer is not guaranteed or endorsed by the publisher.
Research integrity at Frontiers
Learn more about the work of our research integrity team to safeguard the quality of each article we publish.