- 1Center for Microbial Pathogenesis, Abigail Wexner Research Institute at Nationwide Children's Hospital, Columbus, OH, United States
- 2Department of Pediatrics, The Ohio State University College of Medicine, Columbus, OH, United States
Recurrent S. aureus infections are common, suggesting that natural immune responses are not protective. All candidate vaccines tested thus far have failed to protect against S. aureus infections, highlighting an urgent need to better understand the mechanisms by which the bacterium interacts with the host immune system to evade or prevent protective immunity. Although there is evidence in murine models that both cellular and humoral immune responses are important for protection against S. aureus, human studies suggest that T cells are critical in determining susceptibility to infection. This review will use an “anatomic” approach to systematically outline the steps necessary in generating a T cell-mediated immune response against S. aureus. Through the processes of bacterial uptake by antigen presenting cells, processing and presentation of antigens to T cells, and differentiation and proliferation of memory and effector T cell subsets, the ability of S. aureus to evade or inhibit each step of the T-cell mediated response will be reviewed. We hypothesize that these interactions result in the redirection of immune responses away from protective antigens, thereby precluding the establishment of “natural” memory and potentially inhibiting the efficacy of vaccination. It is anticipated that this approach will reveal important implications for future design of vaccines to prevent these infections.
Introduction
Staphylococcus aureus is an aerobic gram-positive organism that can cause local and systemic infections in humans, ranging in severity from skin and soft tissue infection (SSTI) to more invasive infections such as osteomyelitis, septic arthritis, pneumonia, bacteremia, and septic shock (1). 20–80% of humans are colonized with S. aureus in the nasopharynx, skin, and/or gastrointestinal tract, providing a reservoir for subsequent infection and transmission (2, 3). A major issue in the field is that “natural” immune responses against S. aureus infection do not seem to be protective and recurrent infection is common—roughly 50% of adults and children with SSTI have a recurrence within a year (4, 5). Developing an effective vaccine has been challenging; all candidate vaccines tested thus far have failed to protect against S. aureus (6–8). These failures must be considered in the context of nearly ubiquitous exposure to S. aureus; it is accepted that most individuals are exposed to S. aureus shortly after birth and throughout childhood (9). This is reflected in the fact that most people, regardless of age or history of symptomatic infection, have detectable levels of anti-staphylococcal antibodies (9). However, whether these antibodies are protective remains elusive. Although there is evidence in murine models that both cellular and humoral immune responses are important for protection against S. aureus, human studies suggest that T cells are most important in determining susceptibility to infection (10, 11).
An “Anatomic” Approach to Understanding S. aureus Evasion of Adaptive Immunity
Herein, we take a systematic approach toward identifying knowledge gaps in our understanding of protective adaptive immunity against S. aureus by reviewing the “anatomy” of the immune response. We focus on current knowledge of how anti-staphylococcal immune responses are generated at each step of the process, and how S. aureus can evade or interfere with these processes. During infection, antigen presenting cells (APCs) phagocytose bacteria and “process” them into smaller peptides by proteolysis (Figure 1) (12). These peptides, called epitopes, may then bind to Major Histocompatibility Complex (MHC) proteins depending on the specific binding affinity of each peptide for the MHC proteins (13). Epitope-bound MHC proteins are then trafficked to the surface of the APCs, where they are presented to cognate T cell receptors (TCR) on naïve T cells within secondary lymphoid organs (MHC Class I for CD8+ T cells, MHC Class II for CD4+ T cells) (14). Binding of the epitope-MHC complex to its cognate T cell receptor on naïve T cells results in differentiation into one of a number of T cell subsets, depending on the local inflammatory milieu and cytokines expressed by innate immune cells (15). These T cell subsets include both effector and memory T cell populations, the latter of which is responsible for the establishment of immunological memory (15). Based on accumulated evidence regarding the importance of T cell responses in defense against S. aueus infection, this review will focus primarily on CD4+ T cell responses in the context of protective adaptive immunity. It is anticipated that this approach will reveal important implications for future design of vaccines to prevent these important infections.
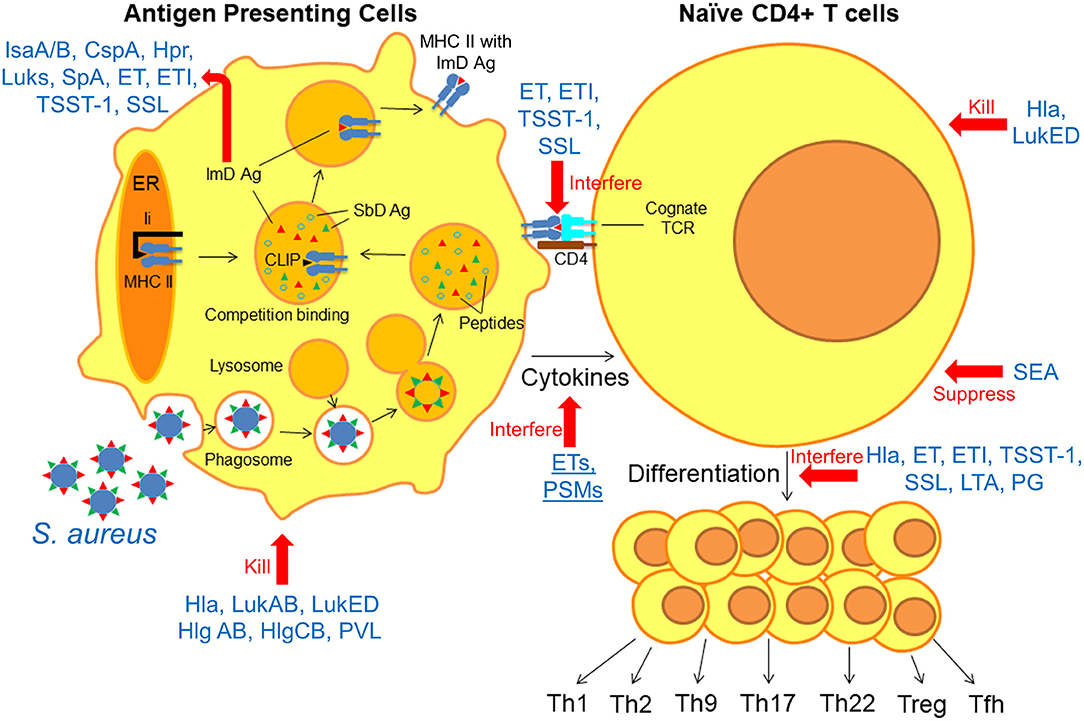
Figure 1. Anatomy of the interfering S. aureus virulence factors with antigen presentation and T cell differentiation. Following phagocytosis of S. aureus by APCs, bacterial antigens are “processed” into epitopes by proteolysis. Immunodominant (ImD) epitopes bind to MHC II based on binding affinity and are trafficked to the cell surface, where they “find” cognate TCRs on naïve T cells. Based on these model, there is a competition between different staphylococcal antigens for binding to MHC II proteins, and ImD antigens such as IsaA/B, CspA, Hpr, Luks, SpA, ET, ETI, TSST-1, and SSL are more successful in this competition. However, non-protective ImD antigens can “outcompete” protective subdominant (SbD) antigens. S. aureus can interfere with the antigen presentation and T cell differentiation in multiple steps; Hla, LukAB, LukED, Hlg AB, HlgCB, and PVL directly kill APCs and T cells. ET, ETI, TSST-1, and SSL as superantigens interfere with peptide-MHC II and TCR interaction. ETs and PSMs interfere with APC cytokines production. SEA, Hla, ET, ETI, TSST-1, SSL, LTA, and PG suppress T cell activation and interfere with T cell differentiation.
S. aureus and Antigen Presenting Cells
Overview
APCs activate T cells to shape immunological memory. Professional APCs include dendritic cells, macrophages, and B cells and are located in a variety of tissues. Dendritic cells are present in the skin (Langerhans cells) and the lining of the nose, lungs, stomach, and intestines (16). Macrophages, primarily differentiated from peripheral blood monocytes, are found in many tissues (17). B cells are produced in the bone marrow and migrate to the spleen and other secondary lymphoid tissues for maturation (18). APCs promote adaptive immune response by secreting cytokines and by presenting specific epitopes bound to MHC proteins. APCs provide three signals to stimulate CD4+ T cells; peptide-MHC II complex, co-stimulatory molecules such as B7.1 and B7.2, and stimulatory cytokines such as IL-12 (19). During infection, S. aureus manipulates these signals to evade host immune responses (20).
Manipulation of APC Cytokine Secretion
Generally, activation of human and mouse DCs results in secretion of IL-12, which in turn promotes Th1 immune responses. Th1 cells secrete IFNγ, a cytokine that activates macrophages at the site of infection to clear pathogens (21). Moreover, stimulation of epidermal DCs (Langerhans cells) results in secretion of the proinflammatory cytokine IL-6 and IL-12 and inhibition of TRAC, a cytokine that promotes Th2 responses (22). Several S. aureus virulence factors impact APC cytokine secretion (23). For example, S. aureus enterotoxin B induces production of high levels of TNF-α and low levels of IL-12 in DCs (24). In mice, depletion of DCs prior to S. aureus infection resulted in higher lethality accompanied by higher bacterial burdens in the kidneys and lungs (25). This was concluded to be secondary to inhibition of IL-12 production because protection was restored by injection of recombinant IL-12. Similarly, phenol-soluble-modulins (PSMs) produced by CA-MRSA strains upregulate CCR7 on the surface of DC subsets and stimulate IL-10 secretion, while inhibiting TNF production (26). Together, these findings suggest that S. aureus can have tolerigenic effects of DCs. In contrast, S. aureus induces production of high levels of IL-12 and IL-23 by monocytes, monocyte-derived macrophages, and DCs, resulting in robust Th1 (IFNγ) and Th17 (IL-17) responses (27). These opposing data suggest that S. aureus can elicit protective or inhibitory responses in APCs, depending on expression of specific virulence factors and the local milieu.
Toxin-Mediated Killing of APCs
A major mechanism by which S. aureus may interfere with APC function is by toxin-mediated APC killing. S. aureus produces a number of bi-component pore-forming leukotoxins that directly kill APCs by creating channels in the plasma membrane (28). Bicomponent toxins are comprised of two subunits, called S (slow) and F (fast), that oligomerize on the surface of target cells to form membrane-spanning pores (29). S. aureus strains isolated from humans produce at least four leukotoxins; the Panton-Valentine Leukocidin (PVL), gamma (γ)-hemolysin (HlgACB), Leukotoxin ED (LukED), and Leukotoxin AB/GH (LukAB/GH) (30). Each leukotoxin has distinct cellular targets that are defined by receptor-specific interactions; monocytes, macrophages, and DCs are targeted by LukAB (CD11b), LukED (CCR5, CXCR1, CXCR2), Hlg AB (CCR2, CXCR1, CXCR2), HlgCB (C5aR1, C5aR2), and PVL (C5aR1, C5aR2)(29). In the context of this review, the direct toxicity of leukotoxins is particularly noteworthy because APCs are an essential link between innate and adaptive immunity (18).
α- toxin (Hla) is a small β-barrel toxin that oligerimizes to form pores in host cell membranes, resulting in cell lysis and death by osmotic swelling and rupture (31). Hla binds to its cellular receptor, ADAM10 (32), resulting in toxicity toward a wide range of mammalian immune cells, including T cells, monocytes, dendritic cells, macrophages, and neutrophils (33). Therefore, similar to the bicomponent leukotoxins, Hla expression can disrupt antigen presentation to T cells by directly killing APCs and by inhibiting differentiation of T cells to effector and memory cells (34). Primary infection in C57BL/6 mice with Hla-producing S. aureus impaired protection against recurrent infection (35). This was attributed, at least in part, to direct toxicity of Hla to dendritic cells, whose numbers were decreased following skin infection with wild type S. aureus, but not an Hla mutant (35). Consistent with this notion, anti-Hla IgG protects against necrosis in the skin and lungs (36, 37), but the effects of antibody on immune cell toxicity are not yet clear and may depend on the site of infection (37). Along these lines, passive transfer of anti-Hla antibody into mice protected against dermonecrosis by neutralizing toxin, rather than by enhancing opsonophagocytosis (38). In this model, Hla-specific antibody also protected against toxicity toward dermal monocytes/macrophages. Therefore, while it is tempting to speculate that Hla-specific IgG protects in part by inhibiting toxicity toward APCs, further elucidation of the mechanisms of protection is necessary. Taken together, these findings demonstrate that S. aureus toxins can directly kill APCs, but the importance of these processes in disturbing adaptive immune responses has yet to be conclusively demonstrated in the clinical setting.
Presentation of S. aureus-Specific Epitopes by APCs
Overview
Once APCs internalize organisms, proteins are cleaved to small peptides, which are bound to MHC and trafficked to the cell surface for presentation to cognate TCRs on naïve T cells (14). Presentation of specific epitopes is highly dependent on binding to MHC, which is dependent on the affinity of peptide-MHC binding. Peptides that are bound strongly to MHC are more “available” for presentation. These are called immunodominant (ImD) peptides, and this step is critical for determining the epitopes against which the immune response is focused. In contrast, peptides with a low affinity for MHC are less efficiently presented, and are termed subdominant (SbD) epitopes (39). ImD epitopes may also be determined by the affinity of peptide-MHC presented epitopes to bind to their cognate T cell receptors (TCRs). The selection of epitopes that drive ImD/SbD antibody responses is somewhat different. For example, un-processed antigens should be accessible to B cell receptors (BCR). After processing by B cells and presentation of epitopes to helper T cells, B cells will be activated against specific epitopes, subsequently followed by antibody affinity maturation and isotype switching. Therefore, antibodies develop against the antigens that are both “available” to BCRs and bind BCRs with strong affinity (40). One of the challenges in establishing immunological memory against S. aureus is that the staphylococcal ImD peptides may not elicit protective memory T cell and antibody responses. In this scenario, one can envision that a strong response against non-protective ImD epitopes may be generated at the expense of a response against more protective, but SbD, epitopes. Therefore, identification of ImD epitopes is critical to better understand how natural immune responses develop.
Immunodominant S. aureus Antigens that Drive Antibody Responses
In addition to establishing immunological memory against S. aureus, T cells work in concert with B cells resulting in high affinity antibody production. A durable and effective antibody response requires T helper cells to assist B cells for antibody affinity maturation and isotype switching. Clearly, quantification of antibody levels is simpler and more reproducible than T cell responses. Therefore, the majority of studies on S. aureus-specific immunity to date have focused on antibody levels. While a detailed review of antibody levels in children and adults with S. aureus infection is beyond the scope of this review, a few key observations have emerged. First, children develop antibody responses during the first year of life and the antibody levels increase throughout childhood (41, 42). Second, high levels of antibodies against selected S. aureus antigens are stable for years in healthy individuals and appear to be functional (41). However, despite high antibody levels during childhood, there may be diminished ability of antibodies to neutralize critical S. aureus toxins (42). Third, children with S. aureus infections generally have higher antibody levels, compared with healthy children (41, 43).
Because antibody levels are more readily quantifiable, compared with T cell responses, one approach is to extrapolate immunodominant antigens from the many antibody studies reported. For example, Lorenz et al. identified four immunodominant proteins during S. aureus infection; IsaA, IsaB, CspA and Hpr (44). Although healthy S. aureus carriers had significantly higher levels of IgG against IsaA comparing to non-carriers, active immunization against IsaA is not protective in mice and anti-IsaA levels are not correlated with protection against S. aureus infection (45). Antibody levels against LukS, LukE, HlgA, HlgC, LukF, LukD, HlgB, Hla, and Hla were high in children with S. aureus infection, compared with healthy controls (46). Importantly, antibody levels correlate with antigen-specific circulating memory B cells (47). Radke et al. identified ImD antigens using a proteomic approach to quantify antibody levels against over 2600 S. aureus antigens. They identified 104 proteins against which all patients had high-level reactivity. All of the above-mentioned ImD proteins are reported within top fifty highly reactive proteins, suggesting some level of conservation of ImD antigens within the population (48).
Immunodominant T Cell Antigens in S. aureus
Unfortunately, epitopes that are ImD in driving B cell/antibody responses are not necessarily the same epitope that drive ImD T cell responses. A variety of ImD T cell epitopes have been identified in animal models, including epitopes within a phosphodiesterase (Plc) (49), LukE and LukS-PV (50), nuclease (51), and IsdB (52), and clumping factor A and protein A appear to elicit T cell responses in both mice and humans (53, 54). However, unlike antibody responses, a relative hierarachy for T cell antigens/epitopes has not been established. To address this, Kolata et al. treated PBMCs from healthy adults with conserved extracellular proteins of S. aureus that elicit an antibody response in most individuals, including the lipase Geh, the phosphodiesterase GlpQ, the phospholipase Plc, and Hla. They observed that the strongest responses were specific for Hla and they found high frequencies of Hla-specific proliferating T cells, compared with the other proteins tested (55). Since Hla also elicits ImD antibody responses, this may suggest that the same antigens drive ImD antibody and T cell responses. However, it is likely that different epitopes within each antigen separately drive antibody and T cell responses. In the future, functional studies should be complemented by in silico approaches that use structure-based algorithms to predict ImD T cell epitopes (49, 56).
Protective vs. ImD Responses
Since individuals are exposed to S. aureus quickly after birth, it is conceivable that immunological memory develops primarily against non-protective ImD antigens. In this scenario, pre-existing memory against non-protective ImD antigens may inhibit vaccination later in life. This is reminiscent of Francis's theory of Original Antigenic Sin (OAS) (57, 58). In OAS, sequential exposure to antigen variants induces a preferential antibody response to an antigen encountered in the past. Consequently, the immune response to the current antigen is weaker (59). However, there remains no compelling evidence that OAS mechanisms are operant in S. aureus-specific immunity. Another model of immune imprinting that describes how ongoing exposure to pathogens may reinforce immune responses against ImD antigens is called “antigenic seniority.” In contrast to OAS, in which patterned immune responses are assumed to be disadvantageous, antigenic seniority describes a process in which early life exposures “build the framework for a hierarchy of immune responses” (40). In this context, ImD responses that are elicited early in life are thought to have a “senior” or privileged position, but subsequent exposures, while boosting these responses, may also produce responses against other SbD antigens. There is some evidence for these mechanisms in the development of S. aureus-specific immunity. For example, Pelzek et al. demonstrated that adults with S. aureus SSTI infection have a diverse set of antigen-specific memory B cells, and these memory B cells correlate well with antigen-specific antibody levels (47). However, much of the antibody response was directed toward cross-reactive antibodies that recognized multiple leukotoxins. Importantly, despite the presence of memory B cells, they did not observe significant increases in antigen-reactive antibody-secreting plasmablasts and plasma cells during infection. Similarly, they found increased memory B cell frequencies only for certain antigens.
Together, these findings suggest a clonal response focused on a limited number of cross-reactive epitopes and provide evidence for a patterned B cell response that limits the diversity of the immune response. A potentially similar mechanism was elucidated by Pauli et al. They found that staphylococcal protein A (SpA) polarized plasmablast responses away from other antigens, suggesting that SpA acts as an ImD antigen in limiting responses against other, potentially protective, antigens (60). Pre-existing natural antibodies may also “mask” protective but SbD epitopes (40), thereby precluding the ability of exposure to these antigens to elicit protective responses. This may be of particular relevance given the broad range of antigen-specific antibodies observed in individuals with S. aureus infection (48). While we do not yet know whether similar mechanisms might inhibit the diversity of T cell responses during S. aureus infection, it is of interest that similar mechanisms have been a challenge for influenza vaccination, in which past exposure may shape the immune system such that vaccination may reinforce responses to epitopes from past exposures, rather than those targeted by the current vaccine (61). We hypothesize that natural immune responses directed against non-protective staphylococcal ImD antigens result in a phenomenon similar to these models. If this is the case, natural exposure to S. aureus may pattern a non-protective memory response over a lifetime, which is not able to prevent re-infection and may even interfere with subsequent vaccine attempts later in life. However, much work needs to be done to test this hypothesis. For example, identification of antibody and T cell responses that predict protection against S. aureus infection must be prioritized in order to move forward (4). In the context of vaccines, mechanistic studies that use “pre-exposed” rather than naïve mice would prove informative and may better simulate vaccination of a human population.
Role of MHC Haplotypes
There is considerable heterogeneity of HLA (Human Leukocyte Antigen) /MHC haplotypes in the human population, and certain haplotypes have been associated with susceptibility to a number of infections. For example, associations between specific HLA Class II polymorphisms and susceptibility to HIV infection, hepatitis, leprosy, tuberculosis, malaria, leishmaniasis, and schistosomiasis have been reported (62). Consistent with this notion, there is an association between HLA Class II gene polymorphisms and susceptibility to S. aureus infection in white and African-American populations (63, 64). Mouse models have uncovered one possible mechanistic explanation for these observations. BALB/c mice are protected against secondary SSTI, but C57BL/6 mice are not (50). These divergent phenotypes were explained by the different MHC class II haplotypes in the mouse strains: BALB/c mice express MHC H-2d and C57BL/6 express H-2b (50). In this model, antibody responses against Hla and Th17 responses against LukE and LukS-PV were observed only in mice that express MHC H-2d. Moreover, concomitant infection inhibited vaccine efficacy in C57BL/6 mice, but not BALB/c mice. The mechanism of this inhibition was due to strong binding of protective epitopes to MHC H-2d, but not H-2b (50). Based on these findings, a model emerges of competition between different staphylococcal antigens for binding to MHC proteins, and ImD antigens are more successful in this competition. However, non-protective ImD antigens can “outcompete” protective antigens, depending on the host genetic background (50). Fortunately, vaccination of naïve mice expressing either H-2d or H-2b was effective. However, these findings have not been translated to human infection.
Nonspecific T Cell Activation: S. aureus Superantigens
Another mechanism by which S. aureus can inhibit protective T cell responses is by expression of superantigens. More than 20 superantigens have been identified in different strains of S. aureus. Approximately 80% of S. aureus isolates from infected patients harbor at least one superantigen, although most isolates express more than one (65). Staphylococcal superantigens are classified as Enterotoxins (ETs), Enterotoxin like proteins (ETls), Toxic Shock Syndrome Toxin−1 (TSST-1) and staphylococcal superantigen-like proteins (SSL) (66). Conventional T cell responses are mediated by the interaction of antigens with hypervariable regions of the αβ T cell receptor (TCR). As such, conventional antigens can stimulate ~0.01% of naïve T cells due to the diversity of CDR3 (Complementarity-determining region 3) in T cell receptors. In contrast, superantigens do not bind to CDR3, but instead bind T cells via a TCR β-chain variable domain (Vβ)-dependent mechanism (65) Because there are limited numbers of functional Vβ regions (around 50) in humans, superantigens can activate many T cells with different TCR (65, 67). Furthermore, superantigens can activate T cells much more strongly than conventional antigens and they are able to activate up to 30% of the T cell pool in picogram concentrations (67).
The consequences of superantigen expression on development of adaptive immunity remain to be fully elucidated. Staphylococcal superantigens are unique in that they activate T cell responses to evade host immunity (68). One mechanism by which superantigens impair cellular memory is by interfering with signaling through the TCR and induction of clonal tolerance (anergy) (69). For example, following stimulation of PBMCs by staphylococcal Enterotoxin C1, CD25+ FoxP3+ regulatory T cells proliferated and secreted the immunosuppressive cytokine IL-10 (70). Because responses against staphylococcal superantigens are highly immunodominant, there has been considerable interest in pursuing a superantigen vaccine (71–73). Unfortunately, none has yet proven effective in clinical studies.
Expansion of NAÏVE to Memory and Effector T Cells in S. aureus Infection
Overview
T cells circulate in blood, lymph vessels, and secondary and peripheral lymphoid tissues. Once mature naive T cells migrate from the thymus to secondary lymphoid organs (lymph nodes, spleen or MALT (Mucosa-associated lymphoid tissues), the interaction between peptide-loaded MHC on the surface of APCs and a cognate TCR results in activation and subsequent differentiation to effecter or memory cells (74, 75). There are various subsets of T cells with different functions in host immunity whose differentiation from naïve T cells depends upon distinct cues (e.g., different cytokines, MHC Class II-peptide complex, and costimulatory signals). Naïve CD4+ T cells can differentiate into several subsets, including Th1, Th2, Th9, Th17, Th22, Treg and Tfh. The classical distinction among these subsets is simplified and many of these cells may have characteristics of one or more subsets and they also may retain plasticity (76). For example, following presentation of their cognate epitope, naïve CD4+ T cells differentiate to Th1 cells following stimulation with IL-12 and IFNγ, to Th2 cells following stimulation by IL-4 and IL-2, or to Th17 cells in the presence of TGF-β and IL-6 (77). Depending on the tissue and the specific stimulus, activated T cells may return to the bloodstream and migrate to the sites of infection or inflammation in peripheral tissues (78).
Importance of T Lymphocytes in Defense Against S. aureus
There is accumulated evidence that T cells response are critical for defense against S. aureus infection in humans and in experimental models. The importance of T cell subsets in defense against S. aureus has been the subject of several outstanding reviews, and will only be briefly discussed here (79, 80). For example, it is well-established that Th17 cells are important in defense against extracellular bacteria (such as S. aureus) via the production of a number of cytokines, resulting in neutrophil activation and recruitment to the site of infection (81). Individuals with hyper immunoglobulin E syndrome, classically caused by mutations in the DNA-binding domain of STAT3, have defects in pathways that result in Th17 cell differentiation and are highly susceptible to recurrent mucocutaneous S. aureus infections (82, 83). Individuals with poorly controlled HIV with low CD4+ counts are also susceptible to S. aureus infection (84). These studies are complemented by a number of animals studies demonstrating the importance of Th17/IL-17A mediated immunity (85–87). The role of Th1-mediated immunity is less clear, as several studies have demonstrated a protective role for this subset in mouse models, but several groups have also reported that Th1-mediated responses may also inhibit protective immunity (53, 88, 89). A role for Th2 responses has been established in allergic diseases mediated by S. aureus (90). While Th22 responses may complement Th17-mediated protection at the mucocutaneous interface (91), the role of this subset is less well-defined. γδ T cells, which display neither CD4 nor CD8 markers on their surface, are a major source of IL-17 production in mouse models (92), but may be more polarized toward IFNγ secretion in humans (93).
S. aureus Toxins Kill T Cells
As alluded to earlier, a number of staphylococcal toxins are able to directly kill T cells. For example, Alonzo et al. showed that LukE binds to CCR5 on the surface of CD4+ T cells, resulting in oligomerization of LukE and LukD (94). This subsequently results in killing of CCR5+ T cells. In support of the importance of this process, CCR5-deficient mice are strongly protected from lethal S. aureus infection. Incubation of peripheral lymphocytes with LukED resulted in CCR5+ T cell depletion, most of which were effector memory T cells. Of note, CCR5 is expressed on both Th1 and Th17 subsets, suggesting a potential evasion strategy by which S. aureus directly kills IL-17 and IFN-γ-producing T cells. Similarly, Hla induces programmed cell death of human T cells during USA300 infection (95). In a mouse model, expression of Hla during primary infection results in abrogated memory T cell responses, at least in part due to direct toxicity on T cells (35). In comparison with wild-type S. aureus, infection with a Hla deletion mutant resulted in greater expansion of antigen- specific memory T cells. Interestingly, maternal immunization with Hla resulted in enhanced development of memory T cells in pups following post-natal infection, supporting the idea that early exposure to Hla interferes with the development of immunological memory (35). Bonifacius et al. have recently reported that Hla induced direct death of Th1-polarized cells, while Th17 cells were relatively resistant. They demonstrated that toxicity is independent of the Hla-ADAM10 interaction and is not due to differential activation of caspases. Instead, they suggested an increased susceptibility of Th1 cells toward Ca2+-mediated activation-induced cell death (96).
Other Mechanisms by Which S. aureus Suppresses T Cells
Leech et al. demonstrated that Hla limits the expansion of tolerigenic Tregs (97). They showed that the number of Tregs in neonatal mice colonized with S. aureus is relatively low upon cutaneous re-exposure as adults and that colonization with an Hla mutant resulted in recovery of pathogen-specific Tregs. Interestingly, topical application of recombinant Hla during S. epidermidis colonization resulted in a lower percentage of S. epidermidis-specific Tregs, but whether this is due to direct toxicity toward Tregs remains to be determined. Other staphylococcal virulence factors also suppress T cell responses. For example, staphylococcal cell wall components such as lipoteichoic acid or O-acetylation of peptidoglycan suppressed T cell proliferation and polarization of Th cells to Th1 and Th17 (98, 99). Staphylococcal enterotoxin A (SEA) upregulated anergy-related genes in CD4+ T cells isolated from Atopic Dermitidis patients (100). S. aureus may also suppress T cell responses by eliciting the expansion of other suppressive immune cells. For example, S. aureus infection in mice resulted in expansion of granulocytic and monocytic Myeloid-Derived Suppressor Cells (MDSCs) (101). This expansion was accompanied by suppression of T cell responses. Taken together, these findings demonstrate that S. aureus is able to suppress T cells via multiple mechanisms.
Challenges in Creating a Protective Vaccine
Overview
The enormous burden of S. aureus infections and emerging antimicrobial resistance makes a vaccine to prevent these infections a worthy goal (102). Despite a lack of understanding of naturally-acquired immunity against S. aureus, several large vaccine tials have targeted adults populations with a high incidence of S. aureus infection (6). Unfortunately, despite promising protection in pre-clinical models, none that advanced to clinical trial has proven effective against human infection (103). Examples include the capsular proteins CP5/CP8 (StaphVAX, Nabi) in patients undergoing hemodialysis, the iron scavenger protein IsdB (V710, Merck) in patients underoing cardiac surgery, and a combination of capsular proteins, clumping factor A (ClfA), and a manganese transporter (MntC) in patients undergoing orthopedic surgery (SA4Ag, Pfizer) (104–106). In each case, vaccination failed to prevent infection despite high levels of elicited antibody in vaccine recipients. There are several possibilities to explain these failures, including high levels of pre-existing immunity among vaccine recipients, the antigens and preclinical models selected for evaluation, the exclusion of vaccine adjuvants, a lack of identified correlates of immunity, and the chosen target populations for vaccination.
Antigen Selection and Preclinical Models
We believe that antigenic seniority may be an obstacle toward developing a successful vaccine against S. aureus infection. Because early life exposure by S. aureus so strongly influences the developing immune system, it is probable that this exposure not only prevents protective immunity, but may also inhibit subsequent vaccine efforts in older individuals (20, 61). If, as in influenza, antigenic seniority is a phenomenon that primarily impacts antibody responses, one approach to enhance vaccine efficacy may be to target T cell responses, rather than antibody responses. This would have the additional benefit of targeting responses that are likely to be more important in human infection. For example, candidate antigens that induce protective Th17 immunity may both enhance efficacy and overcome patterned antibody responses (107). In this context, toxins would be attractive candidate antigens, because they interfere with nearly every step of the host adaptive immune responses. However, the high “natural” levels of toxin-specific antibodies, many of which are cross-reactive, suggests that it will be necessary to identify protective SbD epitopes that can be used to elicit protective responses. An approach to overcome epitope masking by naturally elicited antibodies would be the design of epitope-focused vaccines that target protective but SbD epitopes (108). In order to increase the likelihood of the success of these approaches, pre-clinical studies should focus on attempting to vaccinate animals that have already been exposed to S. aureus, rather than reliance on naïve animals. The genetic background of experimental animals should also be considered here, since different mouse strains respond differently to S. aureus infection. Finally, because of the documented differences between S. aureus infection in mice and humans (109) and the wide-range of virulence factors that drive different infectious syndromes (110), candidate vaccines should be tested against multiple types of S. aureus infection, and alternative models such as rabbits and non-human primates should be considered to complement mouse studies.
Adjuvants
Novel adjuvants that stimulate certain T cell responses may also help to overwrite patterned immunity. For example, Bagnoli et al. used a novel TLR7-dependent adjuvant to induce strong and broad protection against S. aureus with a multivalent vaccine including Hla, EsxA, EsxB, and the surface proteins ferric hydroxamate uptake D2 (FhuD2) and conserved staphylococcal antigen 1A (Csa1A). Importantly, they demonstrated superior protection with the TLR7 adjuvant, compared with alum (111). Monaci et al. also used MF59, an oil-in-water emulsion licensed in human vaccines, with 4C-Staph (FhuD2, Csa1A, α-Hemolysin, EsxA, and EsxB) induced stronger antigen-specific IgG titers and CD4+ T-cell responses compairing with alum (112). The use of novel adjuvants, perhaps in combination with epitope-focused approaches, may also improve our ability to generate antibody and T cell responses against SbD protective antigens. Given the emergence of novel adjuvants and vaccine formulations, more work is needed in this area.
Correlates of Protection
As mentioned above, a major challenge in the development of staphylococcal vaccines is that there is a dearth of identified correlates of protection. One such example is antibody levels against Hla, which correlate with protection against recurrent infection in children (4). However, despite high anti-Hla antibody levels in children, there is some evidence that children have lower levels of neutralizing antibody. Future work should focus on identifying both serologic and cellular correlates of protection. This would enable secondary targets of vaccine efficacy, which might be particularly important in vaccinating against a relatively rare infection. Perhaps more importantly, identification of correlates of immunity will provide important mechanistic insight that can provide the foundation for future vaccine efforts. One possibility would be to determine whether S. aureus-specific Th17 or memory T cells can be a suitable biomarker to predict human protection against infection (113).
Target Population
Finally, there has been much debate about the ideal target population (and infectious syndrome) for a S. aureus vaccine. As discussed, previous approaches have focused on populations with a high incidence of infection. However, given the high burden of S. aureus infection in children (114), we believe that a successful vaccine should be implemented on the population level and administered during childhood. This will have several benefits. First, this approach would leverage the childhood vaccine infrastructure and prevent infections in vulnerable populations that would not otherwise be protected. Second, if patterned immune responses prevent vaccine efficacy, vaccination prior to the onset of these responses would be anticipated to be more effective. However, this approach would require very large studies of vaccine efficacy. A corollary to this approach would be maternal vaccination. The exciting findings that vaccination of pregnant mice resulted in the protection of offspring provide pre-clinical rationale for this approach (35). However, it is appreciated that S. aureus is a commensal, and therefore bacterial eradication may not be possible. Future work should focus on how vaccines may prevent common infectious syndromes (e.g., skin infections) and their impact on asymptomatic colonization. It is anticipated that pragmatic application of detailed mechanistic insight will be necessary to drive the field forward.
Conclusion
Understanding the mechanisms by which S. aureus evades immunological memory is critical to design a protective vaccine. As reviewed here, we believe that future studies should focus on developing strategies to circumvent the multiple mechanisms used by S. aureus to prevent protective T cell responses. It is anticipated that a better understanding of these host-pathogen interactions can be leveraged toward future vaccine efforts.
Author Contributions
OT performed the literature search and drafted the manuscript. CM revised the manuscript. All authors contributed to the article and approved the submitted version.
Funding
This study was funded by the National Institute for Allergy and Infectious Diseases (AI125489 to CM).
Conflict of Interest
The authors declare that the research was conducted in the absence of any commercial or financial relationships that could be construed as a potential conflict of interest.
References
1. David MZ, Daum RS. Community-associated methicillin-resistant Staphylococcus aureus: epidemiology and clinical consequences of an emerging epidemic. Clin Microbiol Rev. (2010) 23:616–87. doi: 10.1128/CMR.00081-09
2. Sakr A, Bregeon F, Mege JL, Rolain JM, Blin O. Staphylococcus aureus nasal colonization: an update on mechanisms, epidemiology, risk factors, and subsequent infections. Front Microbiol. (2018) 9:2419. doi: 10.3389/fmicb.2018.02419
3. Kumar N, David MZ, Boyle-Vavra S, Sieth J, Daum RS. High Staphylococcus aureus colonization prevalence among patients with skin and soft tissue infections and controls in an urban emergency department. J Clin Microbiol. (2015) 53:810–5. doi: 10.1128/JCM.03221-14
4. Fritz SA, Tiemann KM, Hogan PG, Epplin EK, Rodriguez M, Al-Zubeidi DN, et al. A serologic correlate of protective immunity against community-onset Staphylococcus aureus infection. Clin Infect Dis. (2013) 56:1554–61. doi: 10.1093/cid/cit123
5. Miller LG, Eells SJ, David MZ, Ortiz N, Taylor AR, Kumar N, et al. Staphylococcus aureus skin infection recurrences among household members: an examination of host, behavioral, and pathogen-level predictors. Clin Infect Dis. (2015) 60:753–63. doi: 10.1093/cid/ciu943
6. Miller LS, Fowler VG, Shukla SK, Rose WE, Proctor RA. Development of a vaccine against Staphylococcus aureus invasive infections: evidence based on human immunity, genetics and bacterial evasion mechanisms. FEMS Microbiol Rev. (2020) 44:123–53. doi: 10.1093/femsre/fuz030
7. Fowler VG, Allen KB, Moreira ED, Moustafa M, Isgro F, Boucher HW, et al. Effect of an investigational vaccine for preventing Staphylococcus aureus infections after cardiothoracic surgery: a randomized trial. JAMA. (2013) 309:1368–78. doi: 10.1001/jama.2013.3010
8. Gurtman A, Begier E, Mohamed N, Baber J, Sabharwal C, Haupt RM, et al. The development of a staphylococcus aureus four antigen vaccine for use prior to elective orthopedic surgery. Hum Vaccin Immunother. (2019) 15:358–70. doi: 10.1080/21645515.2018.1523093
9. Verkaik NJ, de Vogel CP, Boelens HA, Grumann D, Hoogenboezem T, Vink C, et al. Anti-staphylococcal humoral immune response in persistent nasal carriers and noncarriers of Staphylococcus aureus. J Infect Dis. (2009) 199:625–32. doi: 10.1086/596743
10. Holland SM. Chronic granulomatous disease. Clin Rev Allergy Immunol. (2010) 38:3–10. doi: 10.1007/s12016-009-8136-z
11. Milner JD, Brenchley JM, Laurence A, Freeman AF, Hill BJ, Elias KM, et al. Impaired T(H)17 cell differentiation in subjects with autosomal dominant hyper-IgE syndrome. Nature. (2008) 452:773–6. doi: 10.1038/nature06764
12. Blum JS, Wearsch PA, Cresswell P. Pathways of antigen processing. Annu Rev Immunol. (2013) 31:443–73. doi: 10.1146/annurev-immunol-032712-095910
13. Wieczorek M, Abualrous ET, Sticht J, Alvaro-Benito M, Stolzenberg S, Noe F, et al. Major Histocompatibility Complex. (MHC) Class I and MHC Class II Proteins: Conformational plasticity in antigen presentation. Front Immunol. (2017) 8:292. doi: 10.3389/fimmu.2017.00292
14. Hirosue S, Dubrot J. Modes of antigen presentation by lymph node stromal cells and their immunological implications. Front Immunol. (2015) 6:446. doi: 10.3389/fimmu.2015.00446
15. Pennock ND, White JT, Cross EW, Cheney EE, Tamburini BA, Kedl RM. T cell responses: naive to memory and everything in between. Adv Physiol Educ. (2013) 37:273–83. doi: 10.1152/advan.00066.2013
16. Romani N, Clausen BE, Stoitzner P. Langerhans cells and more: langerin-expressing dendritic cell subsets in the skin. Immunol Rev. (2010) 234:120–41. doi: 10.1111/j.0105-2896.2009.00886.x
17. Honold L, Nahrendorf M. Resident and monocyte-derived macrophages in cardiovascular disease. Circ Res. (2018) 122:113–27. doi: 10.1161/CIRCRESAHA.117.311071
18. Gaudino SJ, Kumar P. Cross-talk between antigen presenting cells and t cells impacts intestinal homeostasis, bacterial infections, and tumorigenesis. Front Immunol. (2019) 10:360. doi: 10.3389/fimmu.2019.00360
19. Sallusto F, Lanzavecchia A. The instructive role of dendritic cells on T-cell responses. Arthritis Res. (2002) 4(Suppl 3):S127–32. doi: 10.1186/ar567
20. Thammavongsa V, Kim HK, Missiakas D, Schneewind O. Staphylococcal manipulation of host immune responses. Nat Rev Microbiol. (2015) 13:529–43. doi: 10.1038/nrmicro3521
21. Frucht DM, Fukao T, Bogdan C, Schindler H, O'Shea JJ, Koyasu S. IFN-gamma production by antigen-presenting cells: mechanisms emerge. Trends Immunol. (2001) 22:556–60. doi: 10.1016/S1471-4906(01)02005-1
22. Mutyambizi K, Berger CL, Edelson RL. The balance between immunity and tolerance: the role of Langerhans cells. Cell Mol Life Sci. (2009) 66:831–40. doi: 10.1007/s00018-008-8470-y
23. Wu X, Xu F. Dendritic cells during Staphylococcus aureus infection: subsets and roles. J Transl Med. (2014) 12:358. doi: 10.1186/s12967-014-0358-z
24. Coutant KD, de Fraissinette AB, Cordier A, Ulrich P. Modulation of the activity of human monocyte-derived dendritic cells by chemical haptens, a metal allergen, and a staphylococcal superantigen. Toxicol Sci. (1999) 52:189–98. doi: 10.1093/toxsci/52.2.189
25. Schindler D, Gutierrez MG, Beineke A, Rauter Y, Rohde M, Foster S, et al. Dendritic cells are central coordinators of the host immune response to Staphylococcus aureus bloodstream infection. Am J Pathol. (2012) 181:1327–37. doi: 10.1016/j.ajpath.2012.06.039
26. Richardson JR, Armbruster NS, Gunter M, Biljecki M, Klenk J, Heumos S, et al. PSM Peptides from community-associated methicillin-resistant Staphylococcus aureus impair the adaptive immune response via modulation of dendritic cell subsets in vivo. Front Immunol. (2019) 10:995. doi: 10.3389/fimmu.2019.00995
27. Frodermann V, Chau TA, Sayedyahossein S, Toth JM, Heinrichs DE, Madrenas J. A modulatory interleukin-10 response to staphylococcal peptidoglycan prevents Th1/Th17 adaptive immunity to Staphylococcus aureus. J Infect Dis. (2011) 204:253–62. doi: 10.1093/infdis/jir276
28. Alonzo F III, Torres VJ. The bicomponent pore-forming leucocidins of Staphylococcus aureus. Microbiol Mol Biol Rev. (2014) 78:199–230. doi: 10.1128/MMBR.00055-13
29. Spaan AN, van Strijp JAG, Torres VJ. Leukocidins: staphylococcal bi-component pore-forming toxins find their receptors. Nat Rev Microbiol. (2017) 15:435–47. doi: 10.1038/nrmicro.2017.27
30. Yoong P, Torres VJ. The effects of Staphylococcus aureus leukotoxins on the host: cell lysis and beyond. Curr Opin Microbiol. (2013) 16:63–9. doi: 10.1016/j.mib.2013.01.012
31. Seilie ES, Bubeck Wardenburg J. Staphylococcus aureus pore-forming toxins: the interface of pathogen and host complexity. Semin Cell Dev Biol. (2017) 72:101–16. doi: 10.1016/j.semcdb.2017.04.003
32. Wilke GA, Bubeck Wardenburg J. Role of a disintegrin and metalloprotease 10 in Staphylococcus aureus alpha-hemolysin-mediated cellular injury. Proc Natl Acad Sci USA. (2010) 107:13473–8. doi: 10.1073/pnas.1001815107
33. Becker RE, Berube BJ, Sampedro GR, DeDent AC, Bubeck Wardenburg J. Tissue-specific patterning of host innate immune responses by Staphylococcus aureus alpha-toxin. J Innate Immun. (2014) 6:619–31. doi: 10.1159/000360006
34. Berube BJ, Bubeck Wardenburg J. Staphylococcus aureus alpha-toxin: nearly a century of intrigue. Toxins. (2013) 5:1140–66. doi: 10.3390/toxins5061140
35. Lee B, Olaniyi R, Kwiecinski JM, Wardenburg JB. Staphylococcus aureus toxin suppresses antigen-specific T cell responses. J Clin Invest. (2020) 130:1122–7. doi: 10.1172/JCI130728
36. Ragle BE, Bubeck Wardenburg J. Anti-alpha-hemolysin monoclonal antibodies mediate protection against Staphylococcus aureus pneumonia. Infect Immun. (2009) 77:2712–8. doi: 10.1128/IAI.00115-09
37. Sampedro GR, DeDent AC, Becker RE, Berube BJ, Gebhardt MJ, Cao H, et al. Targeting Staphylococcus aureus alpha-toxin as a novel approach to reduce severity of recurrent skin and soft-tissue infections. J Infect Dis. (2014) 210:1012–8. doi: 10.1093/infdis/jiu223
38. Yang C, de Dios Ruiz-Rosado J, Robledo-Avila FH, Li Z, Jennings RN, Partida-Sanchez S, et al. Antibody-mediated protection against Staphylococcus aureus dermonecrosis: synergy of toxin neutralization and neutrophil recruitment. J Invest Dermatol. (2020) 16;S0022-202X(20)32064-9. doi: 10.1016/j.jid.2020.09.001
39. Akram A, Inman RD. Immunodominance: a pivotal principle in host response to viral infections. Clin Immunol. (2012) 143:99–115. doi: 10.1016/j.clim.2012.01.015
40. Knight M, Changrob S, Li L, Wilson PC. Imprinting, immunodominance, and other impediments to generating broad influenza immunity. Immunol Rev. (2020) 296:191–204. doi: 10.1111/imr.12900
41. Dryla A, Prustomersky S, Gelbmann D, Hanner M, Bettinger E, Kocsis B, et al. Comparison of antibody repertoires against Staphylococcus aureus in healthy individuals and in acutely infected patients. Clin Diagn Lab Immunol. (2005) 12:387–98. doi: 10.1128/CDLI.12.3.387-398.2005
42. Wu Y, Liu X, Akhgar A, Li JJ, Mok H, Sellman BR, et al. Prevalence of IgG and neutralizing antibodies against Staphylococcus aureus alpha-toxin in healthy human subjects and diverse patient populations. Infect Immun. (2018) 86:e00671–17. doi: 10.1128/IAI.00671-17
43. Hermos CR, Yoong P, Pier GB. High levels of antibody to panton-valentine leukocidin are not associated with resistance to Staphylococcus aureus-associated skin and soft-tissue infection. Clin Infect Dis. (2010) 51:1138–46. doi: 10.1086/656742
44. Lorenz U, Ohlsen K, Karch H, Hecker M, Thiede A, Hacker J. Human antibody response during sepsis against targets expressed by methicillin resistant Staphylococcus aureus. FEMS Immunol Med Microbiol. (2000) 29:145–53. doi: 10.1111/j.1574-695X.2000.tb01517.x
45. Koedijk D, Pastrana FR, Hoekstra H, Berg SVD, Back JW, Kerstholt C, et al. Differential epitope recognition in the immunodominant staphylococcal antigen A of Staphylococcus aureus by mouse versus human IgG antibodies. Sci Rep. (2017) 7:8141. doi: 10.1038/s41598-017-08182-9
46. Thomsen IP, Dumont AL, James DB, Yoong P, Saville BR, Soper N, et al. Children with invasive Staphylococcus aureus disease exhibit a potently neutralizing antibody response to the cytotoxin LukAB. Infect Immun. (2014) 82:1234–42. doi: 10.1128/IAI.01558-13
47. Pelzek AJ, Shopsin B, Radke EE, Tam K, Ueberheide BM, Fenyo D, et al. Human memory B cells targeting Staphylococcus aureus exotoxins are prevalent with skin and soft tissue infection. mBio. (2018) 9:e02125–17. doi: 10.1128/mBio.02125-17
48. Radke EE, Brown SM, Pelzek AJ, Fulmer Y, Hernandez DN, Torres VJ, et al. Hierarchy of human IgG recognition within the Staphylococcus aureus immunome. Sci Rep. (2018) 8:13296. doi: 10.1038/s41598-018-31424-3
49. Soltan MA, Magdy D, Solyman SM, Hanora A. Design of Staphylococcus aureus new vaccine candidates with B and T cell epitope mapping. reverse vaccinology, and immunoinformatics. OMICS. (2020) 24:195–204. doi: 10.1089/omi.2019.0183
50. Si Y, Zhao F, Beesetty P, Weiskopf D, Li Z, Tian Q, et al. Inhibition of protective immunity against Staphylococcus aureus infection by MHC-restricted immunodominance is overcome by vaccination. Sci Adv. (2020) 6:eaaw7713. doi: 10.1126/sciadv.aaw7713
51. Nikcevich KM, Kopielski D, Finnegan A. Interference with the binding of a naturally processed peptide to class II alters the immunodominance of T cell epitopes in vivo. J Immunol. (1994). 153:1015–26.
52. Yu S, Zhang H, Yao D, et al. Identification of CD4+ T-cell epitopes on iron-regulated surface determinant B of Staphylococcus aureus. Microb Pathog. (2015) 89:108–13. doi: 10.1016/j.micpath.2015.09.006
53. Brown AF, Murphy AG, Lalor SJ, Leech JM, O'Keeffe KM, Mac Aogain M, et al. Memory Th1 cells are protective in invasive Staphylococcus aureus infection. PLoS Pathog. (2015) 11:e1005226. doi: 10.1371/journal.ppat.1005226
54. Uebele J, Stein C, Nguyen MT, Schneider A, Kleinert F, Ticha O, et al. Antigen delivery to dendritic cells shapes human CD4+ and CD8+ T cell memory responses to Staphylococcus aureus. PLoS Pathog. (2017) 13:e1006387. doi: 10.1371/journal.ppat.1006387
55. Kolata JB, Kuhbandner I, Link C, Normann N, Vu CH, Steil L, et al. The FALL OF A Dogma? Unexpected high T-cell memory response to Staphylococcus aureus in humans. J Infect Dis. (2015) 212:830–8. doi: 10.1093/infdis/jiv128
56. Oprea M, Antohe F. Reverse-vaccinology strategy for designing T-cell epitope candidates for Staphylococcus aureus endocarditis vaccine. Biologicals. (2013) 41:148–53. doi: 10.1016/j.biologicals.2013.03.001
57. Monto AS, Malosh RE, Petrie JG, Martin ET. The doctrine of original antigenic sin: separating good from evil. J Infect Dis. (2017) 215:1782–8. doi: 10.1093/infdis/jix173
58. Francis T. On the doctrine of original antigenic sin. Proc. Am. Philosoph. Soc. (1960) 104:572–8.
59. Kim JH, Davis WG, Sambhara S, Jacob J. Strategies to alleviate original antigenic sin responses to influenza viruses. Proc Natl Acad Sci USA. (2012) 109:13751–6. doi: 10.1073/pnas.0912458109
60. Pauli NT, Kim HK, Falugi F, Huang M, Dulac J, Henry Dunand C, et al. Staphylococcus aureus infection induces protein A-mediated immune evasion in humans. J Exp Med. (2014) 211:2331–9. doi: 10.1084/jem.20141404
61. Henry C, Palm AE, Krammer F, Wilson PC. From original antigenic sin to the universal influenza virus vaccine. Trends Immunol. (2018) 39:70–9. doi: 10.1016/j.it.2017.08.003
62. Blackwell JM, Jamieson SE, Burgner D. HLA and infectious diseases. Clin Microbiol Rev. (2009) 22:370–85. doi: 10.1128/CMR.00048-08
63. DeLorenze GN, Nelson CL, Scott WK, Allen AS, Ray GT, Tsai AL, et al. Polymorphisms in HLA class II genes are associated with susceptibility to Staphylococcus aureus infection in a white population. J Infect Dis. (2016) 213:816–23. doi: 10.1093/infdis/jiv483
64. Cyr DD, Allen AS, Du GJ, Ruffin F, Adams C, Thaden JT, et al. Evaluating genetic susceptibility to Staphylococcus aureus bacteremia in African Americans using admixture mapping. Genes Immun. (2017) 18:95–9. doi: 10.1038/gene.2017.6
65. Xu SX, McCormick JK. Staphylococcal superantigens in colonization and disease. Front Cell Infect Microbiol. (2012) 2:52. doi: 10.3389/fcimb.2012.00052
66. Krakauer T. Update on staphylococcal superantigen-induced signaling pathways and therapeutic interventions. Toxins. (2013) 5:1629–54. doi: 10.3390/toxins5091629
67. Muller-Alouf H, Carnoy C, Simonet M, Alouf JE. Superantigen bacterial toxins: state of the art. Toxicon. (2001) 39:1691–701. doi: 10.1016/S0041-0101(01)00156-8
68. Nizet V. Understanding how leading bacterial pathogens subvert innate immunity to reveal novel therapeutic targets. J Allergy Clin Immunol. (2007) 120:13–22. doi: 10.1016/j.jaci.2007.06.005
69. Watson AR, Janik DK, Lee WT. Superantigen-induced CD4 memory T cell anergy. I. Staphylococcal enterotoxin B induces Fyn-mediated negative signaling. Cell Immunol. (2012) 276:16–25. doi: 10.1016/j.cellimm.2012.02.003
70. Lee J, Park N, Park JY, Kaplan BLF, Pruett SB, Park JW, et al. Induction of immunosuppressive CD8(+)CD25(+)FOXP3(+) regulatory T cells by suboptimal stimulation with staphylococcal enterotoxin C1. J Immunol. (2018) 200:669–80. doi: 10.4049/jimmunol.1602109
71. Venkatasubramaniam A, Adhikari RP, Kort T, Liao GC, Conley S, Abaandou L, et al. TBA225, a fusion toxoid vaccine for protection and broad neutralization of staphylococcal superantigens. Sci Rep. (2019) 9:3279. doi: 10.1038/s41598-019-39890-z
72. Gampfer J, Thon V, Gulle H, Wolf HM, Eibl MM. Double mutant and formaldehyde inactivated TSST-1 as vaccine candidates for TSST-1-induced toxic shock syndrome. Vaccine. (2002) 20:1354–64. doi: 10.1016/S0264-410X(01)00470-4
73. Hu DL, Omoe K, Sasaki S, Sashinami H, Sakuraba H, Yokomizo Y, et al. Vaccination with nontoxic mutant toxic shock syndrome toxin 1 protects against Staphylococcus aureus infection. J Infect Dis. (2003) 188:743–52. doi: 10.1086/377308
74. Weinreich MA, Jameson SC, Hogquist KA. Postselection thymocyte maturation and emigration are independent of IL-7 and ERK5. J Immunol. (2011) 186:1343–7. doi: 10.4049/jimmunol.1002238
75. Ng YH, Chalasani G. Role of secondary lymphoid tissues in primary and memory T-cell responses to a transplanted organ. Transplant Rev. (2010) 24:32–41. doi: 10.1016/j.trre.2009.09.003
76. Bonelli M, Shih HY, Hirahara K, Singelton K, Laurence A, Poholek A, et al. Helper T cell plasticity: impact of extrinsic and intrinsic signals on transcriptomes and epigenomes. Curr Top Microbiol Immunol. (2014) 381:279–326. doi: 10.1007/82_2014_371
77. Zhu J, Yamane H, Paul WE. Differentiation of effector CD4 T cell populations. (*). Annu Rev Immunol. (2010) 28:445–89. doi: 10.1146/annurev-immunol-030409-101212
78. Hampton HR, Chtanova T. Lymphatic migration of immune cells. Front Immunol. (2019) 10:1168. doi: 10.3389/fimmu.2019.01168
79. Broker BM, Mrochen D, Peton V. The T cell response to Staphylococcus aureus. Pathogens. (2016) 5:31. doi: 10.3390/pathogens5010031
80. Miller LS, Cho JS. Immunity against Staphylococcus aureus cutaneous infections. Nat Rev Immunol. (2011) 11:505–18. doi: 10.1038/nri3010
81. Ge Y, Huang M, Yao YM. Biology of interleukin-17 and its pathophysiological significance in sepsis. Front Immunol. (2020) 11:1558. doi: 10.3389/fimmu.2020.01558
82. Renner R, Hipler C, Treudler R, Harth W, Suss A, Simon JC. Identification of a 27 kDa protein in patients with anaphylactic reactions to mango. J Investig Allergol Clin Immunol. (2008) 18:476–81.
83. Dellinger RP, Levy MM, Carlet JM, Bion J, Parker MM, Jaeschke R, et al. Surviving sepsis campaign: international guidelines for management of severe sepsis and septic shock: 2008. Crit Care Med. (2008) 36:296–327. doi: 10.1097/01.CCM.0000298158.12101.41
84. Hidron AI, Kempker R, Moanna A, Rimland D. Methicillin-resistant Staphylococcus aureus in HIV-infected patients. Infect Drug Resist. (2010) 3:73–86. doi: 10.2147/IDR.S7641
85. Reiss-Mandel A, Rubin C, Zayoud M, Rahav G, Regev-Yochay G. Staphylococcus aureus colonization induces strain-specific suppression of interleukin-17. Infect Immun. (2018) 86:e00834–17. doi: 10.1128/IAI.00834-17
86. Cho JS, Pietras EM, Garcia NC, Ramos RI, Farzam DM, Monroe HR, et al. IL-17 is essential for host defense against cutaneous Staphylococcus aureus infection in mice. J Clin Invest. (2010) 120:1762–73. doi: 10.1172/JCI40891
87. Ishigame H, Kakuta S, Nagai T, Kadoki M, Nambu A, Komiyama Y, et al. Differential roles of interleukin-17A and−17F in host defense against mucoepithelial bacterial infection and allergic responses. Immunity. (2009) 30:108–19. doi: 10.1016/j.immuni.2008.11.009
88. Montgomery CP, Daniels M, Zhao F, Alegre ML, Chong AS, Daum RS. Protective immunity against recurrent Staphylococcus aureus skin infection requires antibody and interleukin-17A. Infect Immun. (2014) 82:2125–34. doi: 10.1128/IAI.01491-14
89. Karauzum H, Haudenschild CC, Moore IN, Mahmoudieh M, Barber DL, Datta SK. Lethal CD4 T cell responses induced by vaccination against Staphylococcus aureus bacteremia. J Infect Dis. (2017) 215:1231–9. doi: 10.1093/infdis/jix096
90. Kim J, Kim BE, Ahn K, Leung DYM. Interactions between atopic dermatitis and staphylococcus aureus infection: clinical implications. Allergy Asthma Immunol Res. (2019) 11:593–603. doi: 10.4168/aair.2019.11.5.593
91. Aujla SJ, Chan YR, Zheng M, Fei M, Askew DJ, Pociask DA, et al. IL-22 mediates mucosal host defense against Gram-negative bacterial pneumonia. Nat Med. (2008) 14:275–81. doi: 10.1038/nm1710
92. Cheng P, Liu T, Zhou WY, Zhuang Y, Peng LS, Zhang JY, et al. Role of gamma-delta T cells in host response against Staphylococcus aureus-induced pneumonia. BMC Immunol. (2012) 13:38. doi: 10.1186/1471-2172-13-38
93. Cooper AJR, Lalor SJ, McLoughlin RM. Activation of human Vdelta2(+) gammadelta T Cells by Staphylococcus aureus promotes enhanced anti-staphylococcal adaptive immunity. J Immunol. (2020) 205:1039–49. doi: 10.4049/jimmunol.2000143
94. Alonzo F III, Kozhaya L, Rawlings SA, Reyes-Robles T, DuMont AL, Myszka DG, et al. CCR5 is a receptor for Staphylococcus aureus leukotoxin ED. Nature. (2013) 493:51–5. doi: 10.1038/nature11724
95. Nygaard TK, Pallister KB, DuMont AL, DeWald M, Watkins RL, Pallister EQ, et al. Alpha-toxin induces programmed cell death of human T cells, B cells, and monocytes during USA300 infection. PLoS ONE. (2012) 7:e36532. doi: 10.1371/journal.pone.0036532
96. Bonifacius A, Goldmann O, Floess S, Holtfreter S, Robert PA, Nordengrun M, et al. Staphylococcus aureus Alpha-toxin limits type 1 while fostering type 3 immune responses. Front Immunol. (2020) 11:1579. doi: 10.3389/fimmu.2020.01579
97. Leech JM, Dhariwala MO, Lowe MM, Chu K, Merana GR, Cornuot C, et al. Toxin-triggered interleukin-1 receptor signaling enables early-life discrimination of pathogenic versus commensal skin bacteria. Cell Host Microbe. (2019) 26:795–809.e5. doi: 10.1016/j.chom.2019.10.007
98. Kaesler S, Skabytska Y, Chen KM, Kempf WE, Volz T, Koberle M, et al. Staphylococcus aureus-derived lipoteichoic acid induces temporary T-cell paralysis independent of Toll-like receptor 2. J Allergy Clin Immunol. (2016) 138:780–90.e6. doi: 10.1016/j.jaci.2015.11.043
99. Sanchez M, Kolar SL, Muller S, Reyes CN, Wolf AJ, Ogawa C, et al. O-acetylation of peptidoglycan limits helper T cell priming and permits Staphylococcus aureus reinfection. Cell Host Microbe. (2017) 22:543–51 e4. doi: 10.1016/j.chom.2017.08.008
100. Orfali RL, Yoshikawa FSY, Oliveira L, Pereira NZ, de Lima JF, Ramos YAL, et al. Staphylococcal enterotoxins modulate the effector CD4(+) T cell response by reshaping the gene expression profile in adults with atopic dermatitis. Sci Rep. (2019) 9:13082. doi: 10.1038/s41598-019-49421-5
101. Tebartz C, Horst SA, Sparwasser T, Huehn J, Beineke A, Peters G, et al. A major role for myeloid-derived suppressor cells and a minor role for regulatory T cells in immunosuppression during Staphylococcus aureus infection. J Immunol. (2015) 194:1100–11. doi: 10.4049/jimmunol.1400196
102. Tong SY, Davis JS, Eichenberger E, Holland TL, Fowler VG Jr. Staphylococcus aureus infections: epidemiology, pathophysiology, clinical manifestations, and management. Clin Microbiol Rev. (2015) 28:603–61. doi: 10.1128/CMR.00134-14
103. Redi D, Raffaelli CS, Rossetti B, De Luca A, Montagnani F. Staphylococcus aureus vaccine preclinical and clinical development: current state of the art. New Microbiol. (2018) 41:208–13.
104. McNeely TB, Shah NA, Fridman A, Joshi A, Hartzel JS, Keshari RS, et al. Mortality among recipients of the Merck V710 Staphylococcus aureus vaccine after postoperative S. aureus infections: an analysis of possible contributing host factors. Hum Vaccin Immunother. (2014) 10:3513–6. doi: 10.4161/hv.34407
105. Fattom A, Fuller S, Propst M, Winston S, Muenz L, He D, et al. Safety and immunogenicity of a booster dose of Staphylococcus aureus types 5 and 8 capsular polysaccharide conjugate vaccine. (StaphVAX) in hemodialysis patients. Vaccine. (2004) 23:656–63. doi: 10.1016/j.vaccine.2004.06.043
106. Frenck RW Jr, Creech CB, Sheldon EA, Seiden DJ, Kankam MK, Baber J, et al. Safety, tolerability, and immunogenicity of a 4-antigen Staphylococcus aureus vaccine. (SA4Ag): results from a first-in-human randomised, placebo-controlled phase 1/2 study. Vaccine. (2017) 35:375–84. doi: 10.1016/j.vaccine.2016.11.010
107. Proctor RA. Challenges for a universal Staphylococcus aureus vaccine. Clin Infect Dis. (2012) 54:1179–86. doi: 10.1093/cid/cis033
108. Oscherwitz J, Cease KB. Identification and validation of a linear protective neutralizing epitope in the beta-pore domain of alpha toxin. PLoS ONE. (2015) 10:e0116882. doi: 10.1371/journal.pone.0116882
109. Salgado-Pabon W, Herrera A, Vu BG. Staphylococcus aureus beta-toxin production is common in strains with the beta-toxin gene inactivated by bacteriophage. J Infect Dis. (2014) 210:784–92. doi: 10.1093/infdis/jiu146
110. Kim HK, Missiakas D, Schneewind O. Mouse models for infectious diseases caused by Staphylococcus aureus. J Immunol Methods. (2014) 410:88–99. doi: 10.1016/j.jim.2014.04.007
111. Bagnoli F, Fontana MR, Soldaini E, Mishra RP, Fiaschi L, Cartocci E, et al. Vaccine composition formulated with a novel TLR7-dependent adjuvant induces high and broad protection against Staphylococcus aureus. Proc Natl Acad Sci USA. (2015) 112:3680–5. doi: 10.1073/pnas.1424924112
112. Monaci E, Mancini F, Lofano G, Bacconi M, Tavarini S, Sammicheli C, et al. MF59- and Al(OH)3-adjuvanted Staphylococcus aureus (4C-Staph) vaccines induce sustained protective humoral and cellular immune responses, with a critical role for effector CD4 T cells at low antibody titers. Front Immunol. (2015) 6:439. doi: 10.3389/fimmu.2015.00439
113. Proctor RA. Recent developments for Staphylococcus aureus vaccines: clinical and basic science challenges. Eur Cell Mater. (2015) 30:315–26. doi: 10.22203/eCM.v030a22
Keywords: S. aureus, vaccine, T cell, antigen presenting cell (APC), human leukocyte antigen (HLA)
Citation: Teymournejad O and Montgomery CP (2021) Evasion of Immunological Memory by S. aureus Infection: Implications for Vaccine Design. Front. Immunol. 12:633672. doi: 10.3389/fimmu.2021.633672
Received: 25 November 2020; Accepted: 03 February 2021;
Published: 22 February 2021.
Edited by:
Isabelle Bekeredjian-Ding, Paul-Ehrlich-Institut (PEI), GermanyReviewed by:
Martin Krönke, University Hospital of Cologne, GermanyGabriel M. Gutierrez, Leidos, United States
Copyright © 2021 Teymournejad and Montgomery. This is an open-access article distributed under the terms of the Creative Commons Attribution License (CC BY). The use, distribution or reproduction in other forums is permitted, provided the original author(s) and the copyright owner(s) are credited and that the original publication in this journal is cited, in accordance with accepted academic practice. No use, distribution or reproduction is permitted which does not comply with these terms.
*Correspondence: Omid Teymournejad, omid.teymournejad@nationwidechildrens.org