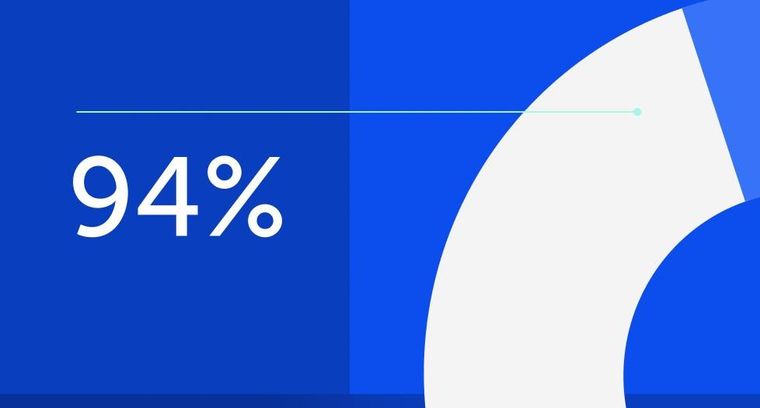
94% of researchers rate our articles as excellent or good
Learn more about the work of our research integrity team to safeguard the quality of each article we publish.
Find out more
REVIEW article
Front. Immunol., 10 March 2021
Sec. Immunological Tolerance and Regulation
Volume 12 - 2021 | https://doi.org/10.3389/fimmu.2021.633436
This article is part of the Research TopicMolecular Mechanisms of Dendritic Cell-Mediated Immune Tolerance and AutoimmunityView all 17 articles
Dendritic cells (DC) are antigen-presenting cells that can communicate with T cells both directly and indirectly, regulating our adaptive immune responses against environmental and self-antigens. Under some microenvironmental conditions DC develop into anti-inflammatory cells which can induce immunologic tolerance. A substantial body of literature has confirmed that in such settings regulatory DC (DCreg) induce T cell tolerance by suppression of effector T cells as well as by induction of regulatory T cells (Treg). Many in vitro studies have been undertaken with human DCreg which, as a surrogate marker of antigen-specific tolerogenic potential, only poorly activate allogeneic T cell responses. Fewer studies have addressed the abilities of, or mechanisms by which these human DCreg suppress autologous effector T cell responses and induce infectious tolerance-promoting Treg responses. Moreover, the agents and properties that render DC as tolerogenic are many and varied, as are the cells’ relative regulatory activities and mechanisms of action. Herein we review the most current human and, where gaps exist, murine DCreg literature that addresses the cellular and molecular biology of these cells. We also address the clinical relevance of human DCreg, highlighting the outcomes of pre-clinical mouse and non-human primate studies and early phase clinical trials that have been undertaken, as well as the impact of innate immune receptors and symbiotic microbial signaling on the immunobiology of DCreg.
In the early 1970s, dendritic cells (DC) were discovered and identified as proficient antigen-presenting cells that were capable of potently activating T cells (1–4). By the early 1990s, DC researchers had begun to uncover the regulatory roles of naturally-occurring DC (5), as well as new ways to convert immature DC into tolerance-promoting antigen-presenting cells (6, 7). However, these experiments were limited due to the small numbers of differentiated DC that can be collected from human or mouse tissues (8, 9), such that it was not until methods were developed to differentiate DC in vitro that DC research really became a mainstream sub-discipline in immunology.
As noted above, DC develop from bone marrow progenitor cells that complete their differentiation in the periphery. Classically, they were thought to differentiate into either “migratory” or “tissue resident” cells, although later studies exploring their relative gene expression patterns delineated a more specific classification system based primarily on lineage (10–13). There are now considered to be five categories of DC: plasmacytoid DC (pDC); conventional DC (cDC), including cDC1 and cDC2 (the latter are also called myeloid DC [mDC]); monocyte-derived DC (mo-DC); and Langerhans cells (14–17). Each of these has been identified in multiple mammalian species, including mice, humans, non-human primates (NHP), and pigs (10, 12, 18). The anatomic localization, immunologic functions, and expression of surface markers, secreted mediators and Toll-like receptors [TLR(s)] by these different groups of murine and human DC has recently been reviewed (19).
In general, cDC are well suited for extra- and intracellular pathogen recognition and antigen presentation to naïve CD4+ and CD8+ T cells, while pDC are more often associated with protective antiviral and systemic autoimmune responses, a consequence of being activated primarily by TLRs that recognize intracellular viral or self-DNA/RNA species (20–24). Langerhans cells are present in the epidermis and have a role in both tolerance and immune priming in that compartment. Mo-DCs differentiate from monocytes recruited during ongoing tissue inflammatory responses [e.g. (25)] and in turn direct the differentiation of CD4+ T cells to Th1, Th2, or Th17 cells (26, 27). Recent research has revealed that these DC categories are much more fluid than once thought, inasmuch as convergence of differentiation pathways and transitioning between the different types of DC is evident (28–30), making categorization of distinct DC sub-populations an increasingly nebulous concept.
As suggested, in the later 1990s the development of in vitro conditions for generating DC from bone marrow and/or peripheral blood progenitors (31, 32) allowed for a surge in research progress. As sentinel antigen-presenting cells DC are well situated immediately adjacent to or integrated into our epithelial cell interfaces with our external environment (e.g., lungs and gut). As steady state cells they are avidly phagocytic, such that they regularly sample their external environment, ingesting and processing all manner of exogenous and endogenous agents, including apoptotic cells, and load the processed antigen peptides onto MHCII molecules for presentation to T cells. DC that are exposed to inflammatory signals during antigen acquisition upregulate their expression of peptide-loaded MHCII, co-stimulatory (e.g., CD40, CD86) and lymph node-homing chemokine receptors, and their expression of inflammatory mediators such as IL-12, while down-regulating their phagocytic activities. They do so while migrating to draining lymph nodes, where they present their processed antigen peptides to T cells in an immunostimulatory context, activating effector T cell (e.g., Th1, Th17) responses (33). If, however, tissue DC acquire otherwise innocuous antigens (e.g., apoptotic cells) in the absence of inflammatory signals, they do not upregulate their antigen-presenting machinery or inflammatory cytokine secretion. As such they provide only low levels of MHCII-bound antigen peptides, co-stimulatory markers and secreted IL-12 signaling to T cells, and thereby induce T cell anergy (34). On the other hand, the DC from mice exposed to innocuous respiratory antigens produce low levels of IL-10 (and less IL-12), while presenting the processed antigens to T cells, and thereby induce Treg responses (35). In the gut, steady state epithelial cells secrete retinoic acid and TGFβ, such that the endogenous gut DC express CD103, TGFβ, and retinoic acid, and thereby induce the differentiation of TGFβ-secreting CD25+LAP+Foxp3− Th3-type Treg (36–38). As DC research became more accessible it was clear that such regulatory DC (DCreg) could be induced under many different conditions, many of which gave rise to DCreg of a unique phenotype, with differing capacities to and mechanisms by which they regulate immune responses (39). Because of this, harnessing the tolerogenic potential of DCreg for the treatment of disease calls for careful consideration of the optimal type of DCreg for each application.
Naturally-occurring and induced DCreg are able to generate robust antigen-specific tolerance by suppressing other immune cells, including Teff, as well as by inducing the differentiation of CD4+ regulatory T cell (Treg) populations. Tolerogenic DC can also directly or indirectly (40) induce regulatory responses among B cells, natural killer cells and CD8+ T cells (41–49), but we will focus herein on DCreg-CD4+ T cell interactions. Specifically, we will review more recent DCreg research, focusing on the studies that have been conducted since our last review of this topic (50). We will address recent progress with human DCreg, but we will also discuss non-human studies where they may shed light on gaps in our knowledge. Furthermore, we will examine the literature that characterizes such DCreg at the cellular and molecular levels and will address the tolerogenic potential and clinical applicability of these cells.
DCreg-T cell conversations are facilitated by cell surface molecules and secreted mediators that can directly suppress effector T cell (Teff) responses. We have reported that steady-state CD8α+ splenic DC from mice can suppress Th2 Teff cell responses through their expression of the tryptophan-catabolizing enzyme indoleamine-2,3-dioxygenase (IDO), but also through expression of IL-10 and TGFβ (51). IDO depletes the local cell environment of tryptophan, an essential amino acid for T cells, and thereby activates the Generalized Controller Non-derepressible-2 Kinase (GCN2) (16, 32, 33), a sensor of cellular amino acid levels, leading to T cell apoptosis (52). In addition, kynurenine break-down products of tryptophan, including 3-hydroxyanthranilic and quinolinic acids, can induce a caspase-8-dependent, but Fas-independent apoptosis of T cells (53). Nevertheless, Fas/FasL signaling can also play a role in DCreg suppression of Teff cells. For example, CD8α+ splenic DC (54) and splenic stroma-educated DCreg (55) induce T cell apoptosis in a Fas/FasL-dependent fashion. In the latter case, FasL signaling activates caspases-3 and -8 in T cells to directly activate apoptosis. However, FasL signaling by DCreg also augments IFNγ secretion by T cells, which in turn induces NO production by the DC, and that further augments CD4+ T effector cell apoptosis (55).
Many reports have addressed the induction of T cell anergy by IL-10-induced human DCreg which, as semi-mature (56) or immature (57) DC (so-called DC10 or DC-10, respectively), express an array of inhibitory receptors (e.g., PD-L1, PD-L2, ILT3, ILT4, HLA-G) (56, 57). It has long been known that HLA-G-expressing antigen-presenting cells can induce T cell anergy (58), but it was more recently recognized that the levels at which HLA-G is expressed by DC-10, at least, correlates with the cell’s regulatory activities (59). However, not all inhibitory factors expressed by a DCreg necessarily contribute to the cell’s regulatory activities. Thus, vitamin D/IFNγ-induced human DCreg express IL-10, HLA-G, PD-L1 and low levels of FasL, but among these it was only their expression of PD-L1 that was reported as integral to the cell’s regulatory activities (60). It is also apparent that inhibitory receptors can play more than one role in immune tolerance. For example, the PD-L1 expressed by TGFβ-induced DCreg is important both to the induction of T cell apoptosis and the differentiation of Treg (61), both of which are essential to successful immunotherapeutic outcomes. Indeed, it has been suggested that the PD-L1:CD86 expression ratio within vitamin D/IL-10-induced DCreg will be a useful predictive marker of the cell’s immunotherapeutic efficacy in the clinic (62). Another, less-reported DC inhibitory receptor is CD31, expressed at high levels on GM-CSF-induced mouse bone marrow DC and on human CD34+ stem cell-differentiated DC that have been exposed to VitD. Engagement of CD31+ DC with T cells strongly inhibits their activation (63), at least in part by inducing rapid T cell disengagement from the DC, effectively raising their activation threshold [reviewed in ref (64)].
Regulatory DC are also well known for their soluble mediators that contribute importantly to their tolerogenic activities. For example, IL-10 is probably the most reported of the inhibitory signals emanating from DCreg (50, 65). It inhibits the antigen-presenting functions of DC (66), but also leads to its own upregulation in these cells. We know that IL-10 expression is essential to the tolerogenicity of IL-10-induced murine DC10 - silencing or deletion of its expression in these cells eliminates their regulatory activities (67, 68). Human and murine DC10 have been reported to suppress Th2 responses in vitro and in vivo (56, 68–70), perhaps related to IL-10-induced granzyme B expression in these T cells and thereby apoptosis (71). TGFβ secretion also contributes to immune tolerance. Its expression by tumor cells can foster the expression of IDO by endogenous tumor DC and thereby suppress anti-tumor immunity (72), but numerous populations of DCreg have been shown to also express TGFβ and to similarly suppress Teff cells [reviewed in (50)]. Vitamin D/dexamethasone-induced DCreg secretion of TGFβ suppresses both IFNγ production and proliferation of CD4+ T cells from rheumatoid arthritis patients (73), just as TGFβ produced by steady state CD8α+ DC or all-trans retinoic acid-induced DCreg (DC-RA) contributes to suppression of allergic donor Teff cell responses in mouse models (51, 74). TGFβ signaling dampens TCR-induced Ca++ influx in T cells, preventing their activation, but it also silences expression of the transcription factors T-bet and STAT4, which are critical to Th1 cell differentiation (72). IL-27 is another cytokine that is intimately linked to the induction of tolerance—it can reduce IL-2 expression during Th1 cell differentiation but also, when coupled with IL-6 signaling, can induce Th1, Th2, and Th17 cells to secrete IL-10 (75). We reported that the DC-RA noted above also secrete high levels of IL-27, but that neutralizing IL-27 does not affect the activation of Th2 cells from peanut allergic mice seen in co-cultures of DC-RA and allergen-presenting immunostimulatory DC. Rather, wild-type (w.t.) DC-RA fully protect against peanut-induced anaphylaxis in vivo by driving the differentiation of LAG3+CD49b+Maf+Foxp3− Treg—unlike w.t. DC-RA, IL-27-/- DC-RA are of no therapeutic benefit (74). IL-27 has also been reported to induce the differentiation of IL-10-secreting Tr1 cells through induction of c-Maf, IL-21 and ICOS in T cells [reviewed in (75)].
There are of course multiple populations of CD4+ Treg. CD25+Foxp3+ Treg, which are probably the most commonly reported Treg, include both naturally-occurring thymic emigrants [natural Treg (nTreg)] as well as cells induced to differentiate in the periphery (induced Treg) from either naïve (76) or effector (77) CD4+ T cells. Interestingly, when the activities of nTreg and DC10-induced Treg of identical TCR specificity were compared in a preclinical model of asthma, the latter cells carried markedly (i.e., ≈5-10-fold) greater regulatory activity than the nTreg (78). There are also inducible IL-10-dependent type-1 Treg (Tr1 cells) that are CD4+CD25−CD49b+LAG3+Foxp3- (79), IL-10-independent CD25+CD49b-LAG3+Foxp3− Treg (74), and oral tolerance-associated, TGFβ-dependent CD4+ Th3 cells (80).
While there is a large body of literature from preclinical models that confirms that DCreg can induce antigen-specific T cell tolerance in vivo (50, 65, 81–83), the collective literature addressing the function of human DCreg is less robust. Most studies with human DCreg have been restricted to showing that these DC only poorly activate allogeneic T cell responses in vitro (50). Fewer studies have assessed whether human DCreg suppress autologous Teff responses and induce Treg differentiation [e.g., (56)], or critically examined the phenotype and tolerogenic mechanisms of the induced Treg. This is an important issue, for expression of Treg markers in itself does not necessarily mean that the putative Treg are functional. A case in point is a recent report relating to the use of immature human DC-10 to induce tolerance among circulating T cells of peanut-allergic donors. Peanut allergen-presenting DC-10 did indeed induce the differentiation of T cells that expressed the expected Tr1 markers, but these cells lacked Tr1 cell activity, as determined in functional assays (84). Whether this could be related to the recently reported Th2 adjuvant activities of peanut proteins (85) has not been assessed as yet. However, we have shown that allergen-loaded semi-mature DC10 from grass- or cat-allergic donors both suppress Th2 responses and induce the differentiation of fully functional CD25+LAG3+CTLA4+Foxp3+ Treg (56).
As in many areas of health research, we have gained important insights into the disease processes and therapeutic approaches from lessons learned in animal models. For example, just as IL-10-induced human monocyte-derived DC [whether immature or semi-mature (56, 57)] are tolerogenic in vitro, murine bone marrow-derived DC10 are potently tolerogenic in vitro and in mouse models of, for example, asthma (68, 69, 77). The regulatory activities of murine DC10 are critically dependent on their expression of IL-10 (67, 68) but also to a lesser extent their expression of CD80 and CD86 (67), IDO (70) and CD40 (Dawicki et al., under review). Interestingly, they induce cognate Th2 cells with which they are cultured to proliferate, but in doing so these Teff cells transdifferentiate into highly effective CD25+Foxp3+ Treg (77, 78). In contrast, mature retinoic acid-induced DC (DC-RA) induce no proliferative responses among Th2 cells, although the T cells differentiate into potent LAG3+CD49b-Foxp3- Treg (74). Thus, overall it is clear that numerous signals that DCreg can bring to interactions with naïve or effector T cells can induce these cells to take on a regulatory phenotype.
Regulatory T cells are activated in a T cell receptor-dependent fashion but, once activated, they are able to suppress the responses of by-stander T cells through a number of non-specific signals (e.g., secretion of IL-10) (86–91). However, bystander DC can similarly adopt a regulatory phenotype following interactions with Treg, and thereby further foster tolerance through a process known as infectious tolerance (92). Treg have a number of mechanisms by which they can induce DCreg, including CTLA-4 induction of IDO (88), LAG3 activation of inhibitory signals (93), or neuropilin-1 signaling (88). Expression of galectin-1 by Foxp3+ Treg (86) induces DC to secrete IL-10 and IL-27 (94), through which they can foster Tr1 cell differentiation (95). And Treg can themselves recruit additional populations of Treg without a need for a DC intermediary. For example, TGFβ released from Th3 cells triggers development of CD25+Foxp3+ Treg (96), while LAP-TGFβ on activated Foxp3+ Treg can induce naïve T cells to adopt a similar Foxp3+ Treg phenotype (97).
Currently, the most commonly used approach for generation of human DCreg includes differentiation of purified CD14+ peripheral blood monocytes into immature DC by culture with GM-CSF and IL-4 (98–100). Murine DCreg, thought to belong to the cDC2 category, are similarly differentiated with GM-CSF and IL-4, but most often from bone marrow progenitors (101). In principle, induced pluripotent stem cells could be used to generate very large numbers of DCreg (102–105), but use of such approaches has been tempered by concerns about the potential for pre-existing epigenetic programming in the stem cells and, more on moral grounds, the phenotypic similarities of stem cells to human foetal cells (106–109). All things considered, the use of mo-DCs is presently the industry standard for in vitro generation of human DCreg for clinical and experimental applications. Tolerogenic agents [reviewed in (50)] are added to the cultures of differentiating cells at varying times, depending on the type of DCreg in question. Because of the high likelihood that DCreg being used for immunotherapeutic purposes will be exposed to marked inflammatory signals after delivery to the recipient, it is critical that the treatment DCreg not convert into immunostimulatory cells that might exacerbate, rather than ameliorate pathology (110). As such, it is routine that DCreg are assessed for their abilities to withstand such phenotypic conversion following challenge with inflammatory mediators (e.g., IL-1β, IL-6, TNF) or TLR agonists [reviewed in (50)]. Maturational markers for DC include increased expression of HLA-DR, co-stimulatory molecules and inflammatory cytokines (e.g., IL-12) (33, 111–113). On the other hand, inflammation-resistant DCreg retain reduced expression levels of these maturational markers while maintaining their expression of inhibitory receptors and anti-inflammatory cytokine secretion, as noted above (44, 45, 73, 74, 94, 95, 114–117).
There are many well-established protocols to generate DCreg, but it is important to keep in mind that the populations of regulatory cells that we generate include both DCreg and, almost inevitably, additional sub-populations of monocyte-derived progeny that may or may not have their own activities. While some DCreg reports do include, for example, a final CD11c+ DC selection step (e.g., MACS- or FACS-sorting) prior to use of the DCreg generated, the majority of reports do not, suggesting that any effects observed may not be exclusively attributable to the DCreg. For example, a recent report of murine DCreg induction with varying doses of vitamin D (DC-VitD) revealed that there was a dose-dependent output of CD11c+ cells. The control immature DC in these experiments were ≈82% CD11c+, while the DC-VitD comprised up to 92% CD11c+ cells. Thus, while the “DC-VitD” pool of cells strongly suppressed CD4+ T cell activation, potential contributions to that activity of the ≥8% non-DC-VitD were not assessed (63). Depending on the precise culture conditions, culture of CD14+ human monocytes in GM-CSF and IL-4 can lead to differentiation of a mixed population of CD83+ DC, CD14+ macrophage-like cells, and/or myeloid-derived stem cells [reviewed in (118)]. Moreover, while CD83+ DCreg remain the predominant cell in these cultures, a proportion of the CD14− cells present subsequently regain their expression of CD14 and their macrophage-like properties when exposed to IL-10 and maturational signals. The authors reported that the presence of even small numbers of these contaminating CD14+ macrophage-like cells skewed the apparent regulatory phenotype of the “DCreg population” in a dose-dependent fashion (118). It has also been reported that human DC10 generated from plastic-adherent monocytes comprise two major sub-populations, including CD83hiHLA-DRhiCCR7+ cells that strongly express CD25, and CD83loHLA-DRloCCR7lo cells. Both populations can suppress Teff cell proliferation and induce Treg differentiation, but the CD83hiHLA-DRhi population is significantly better in both tasks, at least in part because the regulatory activities in these DC10 cultures were CD25-dependent (119). Taken together, these outcomes do not diminish the importance of DCreg, but they do raise the question of whether contaminating cells in, for example, monocyte-derived cultures might also contribute significantly, but in a negative manner, to the regulatory outcomes observed. This would be relevant for functional studies, but it would be critical to have such insights when undertaking, for example, global transcriptomic studies of DCreg.
In order for DCreg to be clinically relevant, the protocols used for their induction must be optimized, keeping in mind the intended target disease. This is in part because the types of infectious tolerance processes launched by the chosen DCreg must fit the clinical indication. For example, as suggested above, both DC-VitD/dex and DC10 secrete IL-10 and thereby induce Th2 cells to differentiate into CD25+Foxp3+ Treg which can fully reverse the asthma phenotype (68, 69, 77, 120). On the other hand, as noted above DC-RA production of IL-10, TGFβ, and IL-27 suppresses peanut anaphylaxis-inducing Th2 cells through induction of LAG3+CD49b−Foxp3− Treg (74). The relevance of this distinction lies in the observation that intestinal inflammation can suppress Foxp3 expression in a mouse model of colitis, such that intestinal Foxp3+ Treg therein convert into pathogenic Th17 cells (121). This begs the question of whether use of DCreg strategies that induce Foxp3− Treg, which could be more inflammation-resistant than the Foxp3+ Treg, would be better suited in this context. Similar inflammation-associated adverse outcomes have been reported in other DCreg immunotherapy models (122, 123). Having said that, it has also been reported that CD40/CD80/CD86-knock down DCreg also induce the differentiation of Foxp3+ Treg in a murine colitis model, but that this treatment is successful in preventing leukocyte infiltration and disease development (124). This indicates that, while it is critical that they be taken into consideration, inflammatory conditions that may be seen by treatment DCreg in vivo do not necessarily lead to adverse outcomes, and that bodes well for DCreg immunotherapeutics.
DCreg routinely recruit Treg into infectious tolerance processes, but induction of Treg is not an essential facet of DCreg-induced tolerance. For example, it has been reported that human vitamin D-induced DCreg foster allogeneic T cell differentiation into classical Treg (125–128), but also that at least some forms of vitamin D-induced DCreg can instead foster a Treg-independent type of tolerance. Thus, murine CD11c+ DC that had been differentiated in GM-CSF and vitamin D, or human DCreg differentiated from CD34+ stem cell precursors with vitamin D do not induce Treg responses, but instead impair CD4+ T cell priming in a CD31-dependent fashion (63). Silencing of CD31 increases T cell activation in DCreg: T cell co-cultures, while its overexpression leads to substantially reduced DC:T cell contact times, with reductions in IL-2 production by the T cells and a consequent loss of T cell priming (63). Collectively this evidence suggests that we must be cognizant that even seemingly closely-related populations of DCreg may utilize quite disparate regulatory mechanisms, and that should be taken into consideration when planning immunotherapeutic approaches. Although such variance may be difficult to predict, there are emerging trends in the published literature.
Tables 1 and 2 outline our more recent advances in the biology of non-human (murine, NHP) and human DCreg, respectively. Because we comprehensively reviewed this area previously (50), these tables provide only an update on observations regarding novel agents to induce the DCreg, or the use of previously reported DCreg in new models, rather than a comprehensive listing of all types of DCreg investigated to date. Thus, the interested reader can find additional information on human DCreg found across our organ systems and those induced in vitro with IL-10 (6, 56, 57, 68–70, 153–161) or other cytokines (160–165), corticosteroids (156, 166–168), vitamin D3 (127, 167, 169–172), rapamycin (156, 167, 173, 174), and neuropeptides (175, 176) in the references cited. Table 1 provides in vivo data from animal models of human diseases, and includes the agents used to induce and mature murine and NHP DCreg, the disease model addressed, the phenotype of the DCreg and the mechanisms by which it induces tolerance, its clinical effects in that model and whether it activates secondary regulatory processes (e.g., Treg).
Table 2 provides data from human DCreg generated from CD14+ monocytes in vitro, and includes the agents used to induce and mature the cells, the phenotype of the DCreg, the mechanisms by which they induce their immunologic effects and whether they activate Treg. While the regulatory activities for most of these cells were assessed exclusively in vitro, the IL-10-lentivirus-tranfected human DC10 were also assessed for their abilities to protect humanized otherwise immunocompromised mice from graft-versus-host disease (149).
There are a number of questions that should be addressed before DCreg immunotherapy can become mainstream as a clinical approach. As suggested above, a particularly important one is the nature of inflammatory conditions the DCreg may face after delivery to the host and their impact on the cell’s regulatory activities. We have previously reviewed the array of innate immune receptors expressed by DC, which include protease-activated receptors (PARs), TLR, C-type lectin receptors, retinoic acid-inducible gene-1 (RIG-1), and the melanoma differentiation-associated gene-5 (MDA-5) (50), but they also express receptors for an array of other inflammatory mediators (177, 178). As noted previously, there are multiple reports that the tolerogenic potential of DCreg can be arrested, or even reversed, under inflammatory conditions, such that the cells instead activate effector T cells and thereby exacerbate pathology (122, 123, 179). Despite the fact that many microbial stimuli have adverse effects on DCreg, there is an accumulating body of evidence that other microbial signals contribute importantly to the regulatory activities of these cells. For example, delivery of the TLR5 agonist flagellin to asthmatic mice ameliorates their disease phenotype through induction of DCreg and, subsequently, Treg responses (180). Moreover, exposure of monocyte-derived DC from house dust mite (HDM)-asthmatic individuals to flagellin also empowers the DC to take on a regulatory phenotype. These flagellin-induced DCreg express high levels of IL-10, TGFβ and HLA-G, such that they strongly foster CD25+Foxp3+ Treg responses (180). In a similar manner, delivery of the dectin-1/TLR2 agonist curdlan (181), a bacterial cell wall exopolysaccharide, to asthmatic mice induces differentiation of IL-10-expressing, Maf+Foxp3− Treg (i.e., Tr1 cells), and thereby reverses the animal’s asthma phenotype (182). On the other hand, human IL-10-lentivirus-transfected DCreg display very complex responses to microbial agonists (149), including polyinosinic:polycytidylic acid (Poly I:C), LPS, flagellin and CpG (agonists specific for TLR3, TLR4, TLR5, and TLR9, respectively), as well as Listeria monocytogenes (149). The authors of this report found that a number of classical DCreg markers were differentially regulated on exposure to these agonists. For example, poly I:C challenge increased HLA-G and decreased ILT4 expression, while LPS exposure down-regulated HLA-G and up-regulated ILT4. In contrast to IL-10-lentivirus-transfected murine DCreg, which induce differentiation of CD25+Foxp3+ Treg (159), the IL-10-lentivirus-transfected human DCreg instead induced the development of Tr1 responses (149). Interestingly, however, while the numbers of Tr1 marker-positive cells were differentially affected by the microbial agonists added to the human DCreg-T cell cultures, marked differences in the suppressive activities or cytokine profiles of the Tr1 cells so induced were not observed (149).
The recognition that our microbiome contributes importantly to our overall health has brought its impact on immune regulation by DC into focus. We know, for example, that the diversity of the microbiome in neonates of ≤100 days of age can predict their likelihood of developing asthma as children (183). Reduced levels of Lachnospira, Veillonella, Faecalibacterium, and Rothia genera bacteria in the neonatal gut are associated with an increased risk of asthma. The neonates so affected display reduced levels of fecal acetate, but see increased urinary excretion of a number of secondary bile acids with potential links to this intestinal dysbiosis (183). We know that microbial catabolites (e.g., short-chain fatty acids) such as butyrate and propionate can induce a tolerogenic phenotype in DC, dampening LPS-induced expression of costimulatory molecules (e.g., CD83, CD40) and augmenting downstream Tr1 responses (184). And culture with Lactobacillus paracasei L9 induces murine bone marrow-derived DC to strongly upregulate IL-10 secretion and to take on a regulatory phenotype, such that they induce CD4+ effector T cells from β-lactoglobulin-allergic mice to differentiate into Foxp3+ Treg (185). Similarly, in experimental germ-free mice β-glucan/galactan polysaccharides and polysaccharide A secreted by intestinal Bifidobacterium bifidum strain PRI1 are associated with increased expression of IL-10, TGFβ, IDO, and PD-L2 within the lamina propria CD103+ DC population (186). Importantly, these DC in turn induce the development of Treg with TCR specificity not only for B. bifidum, but also for otherwise unrelated intestinal antigens (186). And Bifidobacterium infantis colonization has been reported to induce shrimp tropomyosin tolerance in a mouse model of shrimp allergy, increasing the gut population of CD103+ DCreg, expression of IL-10 and TGFβ, and the numbers of CD4+CD25+CD127- Treg (187).
Unlike pathogenic enteric bacteria, which actively secrete pathogenic mediators within the gut, tolerance-promoting gut symbionts reportedly interact with their hosts via capsular polysaccharides that decorate outer membrane vesicles (OMV) shed by the bacteria. Thus, Bacteroides fragilis OMV signal to gut DC through TLR2, which induces the development of DCreg and Treg (188), but there can be heterogeneity in the way that gut DC respond to such signaling. Thus, while Bacteroides thetaiotaomicron OMVs induce IL-10 expression and a regulatory phenotype in colonic DC of healthy subjects, such immune regulation does not occur with DC from individuals with ulcerative colitis (189). Organisms with beneficial properties can also be found in the external environment. Indeed, curdlan, the tolerogenic β-glucan noted above (182), is derived from the environmental bacterium Alcaligenes faecalis. In a similar manner, exopolysaccharides from Cyanobacterium aponinum, an organism represented at high levels in the waters of the Blue Lagoon of Iceland, induce human DC to express IL-10 and CD141, leading to Treg induction (190, 191). Bathing in the Blue Lagoon is renowned for its abilities to alleviate psoriatic plaques (191).
There have been scores of preclinical studies in mouse models documenting that DCreg can induce immunologic tolerance and reverse the disease phenotype in models of allergy and asthma, autoimmune diseases and transplant rejection responses [reviewed in (50)]. As part of moving DCreg closer to clinical application there have also been a number of DCreg trials undertaken in NHP models (Table 1). An early study with maturation-resistant VitD3/IL-10-induced rhesus macaque DCreg showed that cells given i.v. led to an initial increase in recipient blood levels of anti-donor and -third party T cell reactivity, but this subsequently waned to below pre-treatment levels in animals also treated with the clinical immunosuppresant CTLA4Ig. There was no induction of anti-donor IgG or IgM alloantibodies detected and, in animals given both DCreg and CTLA4Ig, alloreactive IL-10-producing T cells were detected at ≥28 days post-infusion (143). Culture of such VitD/IL-10-induced NHP DCreg with purified allogeneic peripheral blood CD4+CD127lo cells, which were enriched in Foxp3-expressing cells (i.e., Treg), for up to 2 weeks led to an expansion of the CD4+CD127lo Treg, and these in turn induced a dose-dependent suppression of CD4+ Teff cell responses (144). A subsequent study with kidney-engrafted rhesus macaque monkeys treated with donor-derived VitD3/IL-10 DCreg showed that the DCreg significantly prolonged graft survival (from ≈39 to 113 dy) and reduced donor-reactive memory T cell:regulatory T cell ratios, with no evidence of circulating donor-specific alloantibodies (146). In another study, VitD/IL-10-induced kidney transplant recipient DCreg were pulsed with donor-derived PBMC membrane vesicles and used to treat transplanted monkeys that were also administered CTLA4Ig and rapamycin. Overall, the authors observed ≈2-fold-prolonged graft rejection times, attenuated systemic IL-17 levels, and modulated CD4+ and CD8+ T cell responses to donor antigens, although these were not statistically significant effects (142). The most recent NHP study reported addressed the impact of VitD/IL-10 DCreg treatment on CD4+CTLA4hi (i.e., Treg) in CTLA4Ig-treated renal allo-transplant recipients. They found that in the absence of DCreg therapy, CTLA4Ig treatment led to reductions in the numbers of circulating CD4+CTLA4hi Treg, while these reductions were not observed in DCreg-treated recipients (145). Overall, these studies highlighted the potential utility of DCreg immunotherapy in preventing rejection of organ transplants [reviewed in (192)].
Consistent with the outcomes of these NHP DCreg studies, there have been a number of successful Phase I or I/II human DCreg clinical trials completed by pioneers in this area in recent years (Table 3). There are reportedly additional trials that are still in recruitment stages or that have not yet published their results (200), as well as several long-term trials ongoing in organ transplant recipients [reviewed in ref (192)]. As these have been largely Phase 1 or I/II trials, their aims were solely to determine whether the DCreg immunotherapy approach in question is safe and well-tolerated, and it is clear that that is the case—the vast majority of subjects have not suffered significant adverse events (193, 195–199). Although, as might be expected for such trials, abrogation of disease was not seen under any of these protocols, reductions in symptoms scores were reported in a small subset of treatment group subjects in the rheumatoid arthritis (195, 197) and Crohn’s disease (196) trials. The observed changes in immunologic parameters in the treatment groups across these studies were encouraging, given that these were Phase I studies, though quite modest (Table 3).
Human monocyte-derived DC are, overall, HLA-DR+CD11c+BDCA3− and express an array of other prototypical DC markers (28) but there is substantial heterogeneity in the markers expressed by, and activities associated with any one DC population. Even seemingly very small differences in the DC culture conditions, from how the starting CD14+ monocyte population is isolated and its purity, to the concentrations of GM-CSF or IL-4 employed, the type of serum or serum-free supplement used in the cultures, times in culture, etc., can have very significant effects on the DC generated. This raises the academic question of whether differentiated tissue DC (e.g., blood, tonsillar) might be better candidate cells for DCreg immunotherapeutics—arguably their use could call for fewer investigator manipulations of the cells. Nevertheless, global transcript profiling of monocyte-derived DC, a number of DC cell lines and purified tissue DC reveals that there is substantial phenotypic heterogeneity here too. Monocyte-derived cells cluster in principle component analyses closely with tonsillar DC, but not other populations, while the blood DC populations tend to loosely cluster by themselves (201). However, developing a DCreg therapy using primary populations of DC would bring its own challenges, not the least of which is the relative scarcity of the cells. Fully differentiated blood DC constitute only 0.1%–1.0% of peripheral blood mononuclear cells (9, 202), such that it would not likely be feasible to purify sufficient numbers of primary cells from any one donor for an effective treatment. Thus, at this point in time, use of well-characterized and standardized monocyte-derived populations of DCreg would appear to be our best clinical option.
One of the more important factors in optimizing DCreg immunotherapy is identification of DCreg of a phenotype that best fits the intended application. There have been a number of reports that have run head-to-head comparisons of human DCreg differentiated with an array of mediators, including comparisons of vitamin D3-, dexamethasone- and rapamycin-induced cells (167), or of vitamin D3-, IL-10-, dexamethasone-, TGFβ− and rapamycin-induced DCreg (156), and assessments of DCreg differentiated with protein kinase C inhibitor versus dexamethasone, vitamin D3, rapamycin, IL-10, TGFβ or a combination of peroxisome proliferator-activated receptor gamma (PPAR-γ) agonist and retinoic acid (203). This has provided important insights into DC yields & viabilities, expression of co-stimulatory or inhibitory markers, IL-10 production, resistance to inflammatory stimuli, and abilities to activate allogeneic T cells or induce Treg (156, 167). Nevertheless, multiple questions remain even given our knowledge and advances in DCreg immunotherapy. While it is easy to appreciate the simplicity of using allogeneic cells for functional readouts with human DCreg, we question whether assessing their impact on autologous, disease-related T cells would more directly address the question of the cell’s suitability for immunotherapeutic applications (i.e., other than in the context of allogeneic transplantation). That is, given that the raison d’etre for DCreg therapy is more often than not suppression of specific antigen-driven pathology, would assessing the cell’s abilities to suppress autologous cognate T cell responses be more relevant (197)? Is assessing expression of Treg-associated markers (e.g., Foxp3) adequate to conclude that the putative Treg induced by these DCreg treatments are functional as regulatory cells? As noted above, at least some DCreg can induce differentiation of T cells that express Treg markers as determined by FACS, despite the fact that they are not functional regulatory cells (84). And, given that different DCreg use different strategies to induce tolerance (204) and thereby induce distinct types of Treg (50), should the nature of the condition being treated (e.g., low versus high levels of target tissue inflammation) impact the choice of the DCreg to be employed? As alluded to above, are there indications that we should target selective induction of Foxp3+ versus Foxp3− Treg or, for that matter, CD4+ versus CD8+ or other regulatory cells? Another question is whether in vitro assays of DCreg function provide sufficiently robust data on the activities of these cells to validate them as candidates for clinical trials. Could in vivo modeling in, for example, humanized mice (205) or NHP (142–146) provide more meaningful insights?
While we often assess a standard set of markers associated with DCreg, it is clear that functional DCreg are more than cells that express low levels of CD40 or CD86, or high levels of PD-1 and IL-10. Indeed, numerous transcriptomic analyses have identified many hundreds of markers that are differentially expressed by DCreg (170, 206–210) but only three immunologically-relevant genes [annexin A1, glucocorticoid-induced leucine zipper (GILZ) and IDO] have been reported to display differential increases in expression within the DCreg across five or more of these reports, while several more (cathepsins B, C, D & L, ILT3, stabilin 1, and TGFβ) saw increases across three of these reports (211). Nevertheless, it is interesting that IL-10 expression, as an example, has not been so identified, particularly given its intimate association with DCreg across a spectrum of reports [reviewed in (50, 65)]. Are there other leads we are missing in this search? Examination of the blood DC from allergic individuals who have undergone allergen-specific immunotherapy have identified a number of markers associated with successful tolerance induction (e.g., C1q, stabilin-1) (210). Indeed, administration of C1q in mouse models of asthma was subsequently shown to also effectively reduce the asthma phenotype in these animals (212). Intuitively, it seems that each population of DCreg likely enjoys contributions from a large number of regulation-associated inputs (e.g., expression of inhibitory mediators and receptors, low levels of HLA-DR and co-stimulation, etc.) that cumulatively define the cell’s regulatory activities, with different populations of DCreg employing distinct levels of large numbers of these as unique inputs.
Currently, the standard of care for allergies and autoimmune disorders comprises the use of immunosuppressive drugs, agents which often have substantial deleterious side-effects and must be administered for the life of the patient. Regulatory dendritic cell research is promising in that DCreg-induced immune regulation appears to be robust and enduring (70), at least as far as we have been able to ascertain in animal models. Murine DCreg treatments can reverse or eliminate even severe disease in models of allergies and autoimmune diseases and extend the life of organ transplants, sometimes indefinitely. They do so by activating infectious tolerance processes that incorporate secondary induction of different types of Treg which, in turn, can recruit additional generations of regulatory cells. Though animal model outcomes cannot be directly translated to human conditions, the burgeoning in vitro research and early-stage clinical trial outcomes with human DCreg bode well for their use in the clinic. Certainly, our DCreg clinical trials to date indicate that DCreg immunotherapy is safe and well-tolerated. Our increasing advances in the cellular and molecular biology of these cells is likely to have a significant impact on the efficacy of DCreg in the clinic in the foreseeable future. Unlike our current pharmacological management of these diseases, immunotherapy with DCreg would seem to allow for the possibility of long-term restoration of the physiologic equilibrium associated with immunologic tolerance.
SN wrote the first drafts of the manuscript. SL contributed to the literature searches. JG contributed to the writing and edited the manuscript. All authors contributed to the article and approved the submitted version.
Funding was provided by grants from the Canadian Institutes of Health Research (MOP53167) and the AllerGen Networks of Centers of Excellence (16B&B10).
The authors declare that the research was conducted in the absence of any commercial or financial relationships that could be construed as a potential conflict of interest.
1. Steinman RM, Cohn ZA. Identification of a novel cell type in peripheral lymphoid organs of mice. I. Morphology, quantitation, tissue distribution. J Exp Med (1973) 137(5):1142–62. doi: 10.1084/jem.137.5.1142
2. Steinman RM, Cohn ZA. Identification of a novel cell type in peripheral lymphoid organs of mice. II. Functional properties in vitro. J Exp Med (1974) 139(2):380–97. doi: 10.1084/jem.139.2.380
3. Steinman RM, Kaplan G, Witmer MD, Cohn ZA. Identification of a novel cell type in peripheral lymphoid organs of mice. V. Purification of spleen dendritic cells, new surface markers, and maintenance in vitro. J Exp Med (1979) 149(1):1–16. doi: 10.1084/jem.149.1.1
4. Steinman RM, Witmer MD. Lymphoid dendritic cells are potent stimulators of the primary mixed leukocyte reaction in mice. Proc Natl Acad Sci U S A (1978) 75(10):5132–6. doi: 10.1073/pnas.75.10.5132
5. Steinman RM. The dendritic cell system and its role in immunogenicity. Annu Rev Immunol (1991) 9:271–96. doi: 10.1146/annurev.iy.09.040191.001415
6. Enk AH, Angeloni VL, Udey MC, Katz SI. Inhibition of Langerhans cell antigen-presenting function by IL-10. A role for IL-10 in induction of tolerance. J Immunol (1993) 151(5):2390–8.
7. Simon JC, Hara H, Denfeld RW, Martin S. UVB-irradiated dendritic cells induce nonproliferating, regulatory type T cells. Skin Pharmacol Appl Skin Physiol (2002) 15(5):330–4. doi: 10.1159/000064537
8. Crowley M, Inaba K, Witmer-Pack M, Steinman RM. The cell surface of mouse dendritic cells: FACS analyses of dendritic cells from different tissues including thymus. Cell Immunol (1989) 118(1):108–25. doi: 10.1016/0008-8749(89)90361-4
9. Freudenthal PS, Steinman RM. The distinct surface of human blood dendritic cells, as observed after an improved isolation method. Proc Natl Acad Sci U S A (1990) 87(19):7698–702. doi: 10.1073/pnas.87.19.7698
10. Heidkamp GF, Sander J, Lehmann CHK, Heger L, Eissing N, Baranska A, et al. Human lymphoid organ dendritic cell identity is predominantly dictated by ontogeny, not tissue microenvironment. Sci Immunol (2016) 1(6):eaaai7677. doi: 10.1126/sciimmunol.aai7677
11. Granot T, Senda T, Carpenter DJ, Matsuoka N, Weiner J, Gordon CL, et al. Dendritic Cells Display Subset and Tissue-Specific Maturation Dynamics over Human Life. Immunity (2017) 46(3):504–15. doi: 10.1016/j.immuni.2017.02.019
12. Guilliams M, Dutertre CA, Scott CL, McGovern N, Sichien D, Chakarov S, et al. Unsupervised High-Dimensional Analysis Aligns Dendritic Cells across Tissues and Species. Immunity (2016) 45(3):669–84. doi: 10.1016/j.immuni.2016.08.015
13. Guilliams M, Ginhoux F, Jakubzick C, Naik SH, Onai N, Schraml BU, et al. Dendritic cells, monocytes and macrophages: a unified nomenclature based on ontogeny. Nat Rev Immunol (2014) 14(8):571–8. doi: 10.1038/nri3712
14. Dzionek A, Fuchs A, Schmidt P, Cremer S, Zysk M, Miltenyi S, et al. BDCA-2, BDCA-3, and BDCA-4: three markers for distinct subsets of dendritic cells in human peripheral blood. J Immunol (2000) 165(11):6037–46. doi: 10.4049/jimmunol.165.11.6037
15. Liu YJ. IPC: professional type 1 interferon-producing cells and plasmacytoid dendritic cell precursors. Annu Rev Immunol (2005) 23:275–306. doi: 10.1146/annurev.immunol.23.021704.115633
16. MacDonald KP, Munster DJ, Clark GJ, Dzionek A, Schmitz J, Hart DN. Characterization of human blood dendritic cell subsets. Blood (2002) 100(13):4512–20. doi: 10.1182/blood-2001-11-0097
17. Ziegler-Heitbrock L, Ancuta P, Crowe S, Dalod M, Grau V, Hart DN, et al. Nomenclature of monocytes and dendritic cells in blood. Blood (2010) 116(16):e74–80. doi: 10.1182/blood-2010-02-258558
18. Auray G, Keller I, Python S, Gerber M, Bruggmann R, Ruggli N, et al. Characterization and Transcriptomic Analysis of Porcine Blood Conventional and Plasmacytoid Dendritic Cells Reveals Striking Species-Specific Differences. J Immunol (2016) 197(12):4791–806. doi: 10.4049/jimmunol.1600672
19. Nutt SL, Chopin M. Transcriptional Networks Driving Dendritic Cell Differentiation and Function. Immunity (2020) 52(6):942–56. doi: 10.1016/j.immuni.2020.05.005
20. Ah Kioon MD, Tripodo C, Fernandez D, Kirou KA, Spiera RF, Crow MK, et al. Plasmacytoid dendritic cells promote systemic sclerosis with a key role for TLR8. Sci Transl Med (2018) 10(423):eaam8458. doi: 10.1126/scitranslmed.aam8458
21. Rowland SL, Riggs JM, Gilfillan S, Bugatti M, Vermi W, Kolbeck R, et al. Early, transient depletion of plasmacytoid dendritic cells ameliorates autoimmunity in a lupus model. J Exp Med (2014) 211(10):1977–91. doi: 10.1084/jem.20132620
22. Sisirak V, Ganguly D, Lewis KL, Couillault C, Tanaka L, Bolland S, et al. Genetic evidence for the role of plasmacytoid dendritic cells in systemic lupus erythematosus. J Exp Med (2014) 211(10):1969–76. doi: 10.1084/jem.20132522
23. Swiecki M, Colonna M. The multifaceted biology of plasmacytoid dendritic cells. Nat Rev Immunol (2015) 15(8):471–85. doi: 10.1038/nri3865
24. Swiecki M, Gilfillan S, Vermi W, Wang Y, Colonna M. Plasmacytoid dendritic cell ablation impacts early interferon responses and antiviral NK and CD8(+) T cell accrual. Immunity (2010) 33(6):955–66. doi: 10.1016/j.immuni.2010.11.020
25. Gordon JR. TGFbeta1 and TNFalpha secreted by mast cells stimulated via the FcepsilonRI activate fibroblasts for high-level production of monocyte chemoattractant protein-1 (MCP-1). Cell Immunol (2000) 201(1):42–9. doi: 10.1006/cimm.2000.1631
26. Liu K, Waskow C, Liu X, Yao K, Hoh J, Nussenzweig M. Origin of dendritic cells in peripheral lymphoid organs of mice. Nat Immunol (2007) 8(6):578–83. doi: 10.1038/ni1462
27. Fucikova J, Palova-Jelinkova L, Bartunkova J, Spisek R. Induction of Tolerance and Immunity by Dendritic Cells: Mechanisms and Clinical Applications. Front Immunol (2019) 10:2393. doi: 10.3389/fimmu.2019.02393
28. Collin M, Bigley V. Human dendritic cell subsets: an update. Immunology (2018) 154(1):3–20. doi: 10.1111/imm.12888
29. Musumeci A, Lutz K, Winheim E, Krug AB. What Makes a pDC: Recent Advances in Understanding Plasmacytoid DC Development and Heterogeneity. Front Immunol (2019) 10:1222. doi: 10.3389/fimmu.2019.01222
30. Rhodes JW, Tong O, Harman AN, Turville SG. Human Dendritic Cell Subsets, Ontogeny, and Impact on HIV Infection. Front Immunol (2019) 10:1088. doi: 10.3389/fimmu.2019.01088
31. Ito T, Wang YH, Duramad O, Hanabuchi S, Perng OA, Gilliet M, et al. OX40 ligand shuts down IL-10-producing regulatory T cells. Proc Natl Acad Sci U S A (2006) 103(35):13138–43. doi: 10.1073/pnas.0603107103
32. Inaba K, Inaba M, Romani N, Aya H, Deguchi M, Ikehara S, et al. Generation of large numbers of dendritic cells from mouse bone marrow cultures supplemented with granulocyte/macrophage colony-stimulating factor. J Exp Med (1992) 176(6):1693–702. doi: 10.1084/jem.176.6.1693
33. Banchereau J, Briere F, Caux C, Davoust J, Lebecque S, Liu YJ, et al. Immunobiology of dendritic cells. Annu Rev Immunol (2000) 18:767–811. doi: 10.1146/annurev.immunol.18.1.767
34. Steinbrink K, Mahnke K, Grabbe S, Enk AH, Jonuleit H. Myeloid dendritic cell: From sentinel of immunity to key player of peripheral tolerance? Hum Immunol (2009) 70(5):289–93. doi: 10.1016/j.humimm.2009.02.003
35. Akbari O, DeKruyff RH, Umetsu DT. Pulmonary dendritic cells producing IL-10 mediate tolerance induced by respiratory exposure to antigen. Nat Immunol (2001) 2(8):725–31. doi: 10.1038/90667
36. Faria AM, Weiner HL. Oral tolerance. Immunol Rev (2005) 206:232–59. doi: 10.1111/j.0105-2896.2005.00280.x
37. Jaensson E, Uronen-Hansson H, Pabst O, Eksteen B, Tian J, Coombes JL, et al. Small intestinal CD103+ dendritic cells display unique functional properties that are conserved between mice and humans. J Exp Med (2008) 205(9):2139–49. doi: 10.1084/jem.20080414
38. Yokota A, Takeuchi H, Maeda N, Ohoka Y, Kato C, Song SY, et al. GM-CSF and IL-4 synergistically trigger dendritic cells to acquire retinoic acid-producing capacity. Int Immunol (2009) 21(4):361–77. doi: 10.1093/intimm/dxp003
39. Steptoe RJ, Thomson AW. Dendritic cells and tolerance induction. Clin Exp Immunol (1996) 105(3):397–402. doi: 10.1046/j.1365-2249.1996.d01-779.x
40. Ma Y, Dawicki W, Zhang X, Gordon JR. Contributions of direct versus indirect mechanisms for regulatory dendritic cell suppression of asthmatic allergen-specific IgG1 antibody responses. PloS One (2018) 13(1):e0190414. doi: 10.1371/journal.pone.0190414
41. Boldison J, Da Rosa LC, Davies J, Wen L, Wong FS. Dendritic cells license regulatory B cells to produce IL-10 and mediate suppression of antigen-specific CD8 T cells. Cell Mol Immunol (2020) 17(8):843–55. doi: 10.1038/s41423-019-0324-z
42. Garcia-Gonzalez P, Ubilla-Olguin G, Catalan D, Schinnerling K, Aguillon JC. Tolerogenic dendritic cells for reprogramming of lymphocyte responses in autoimmune diseases. Autoimmun Rev (2016) 15(11):1071–80. doi: 10.1016/j.autrev.2016.07.032
43. Audiger C, Rahman MJ, Yun TJ, Tarbell KV, Lesage S. The Importance of Dendritic Cells in Maintaining Immune Tolerance. J Immunol (2017) 198(6):2223–31. doi: 10.4049/jimmunol.1601629
44. Bourque J, Hawiger D. Immunomodulatory Bonds of the Partnership between Dendritic Cells and T Cells. Crit Rev Immunol (2018) 38(5):379–401. doi: 10.1615/CritRevImmunol.2018026790
45. Esebanmen GE, Langridge WHR. The role of TGF-beta signaling in dendritic cell tolerance. Immunol Res (2017) 65(5):987–94. doi: 10.1007/s12026-017-8944-9
46. Iberg CA, Hawiger D. Natural and Induced Tolerogenic Dendritic Cells. J Immunol (2020) 204(4):733–44. doi: 10.4049/jimmunol.1901121
47. Iberg CA, Jones A, Hawiger D. Dendritic Cells As Inducers of Peripheral Tolerance. Trends Immunol (2017) 38(11):793–804. doi: 10.1016/j.it.2017.07.007
48. Zanoni I, Granucci F, Foti M, Ricciardi-Castagnoli P. Self-tolerance, dendritic cell (DC)-mediated activation and tissue distribution of natural killer (NK) cells. Immunol Lett (2007) 110(1):6–17. doi: 10.1016/j.imlet.2007.03.001
49. Elizondo DM, Andargie TE, Haddock NL, da Silva RLL, de Moura TR, Lipscomb MW. IL-10 producing CD8(+) CD122(+) PD-1(+) regulatory T cells are expanded by dendritic cells silenced for Allograft Inflammatory Factor-1. J Leukoc Biol (2019) 105(1):123–30. doi: 10.1002/JLB.1A0118-010RR
50. Gordon JR, Ma Y, Churchman L, Gordon SA, Dawicki W, et al. Regulatory dendritic cells for immunotherapy in immunologic diseases. Front Immunol (2014) 5:7. doi: 10.3389/fimmu.2014.00007
51. Gordon JR, Li F, Nayyar A, Xiang J, Zhang X, et al. CD8 alpha+, but not CD8 alpha-, dendritic cells tolerize Th2 responses via contact-dependent and -independent mechanisms, and reverse airway hyperresponsiveness, Th2, and eosinophil responses in a mouse model of asthma. J Immunol (2005) 175(3):1516–22. doi: 10.4049/jimmunol.175.3.1516
52. Huang YS, Ogbechi J, Clanchy FI, Williams RO, Stone TW, Disorders CNS, et al. IDO and Kynurenine Metabolites in Peripheral and CNS Disorders. Front Immunol (2020) 11:388. doi: 10.3389/fimmu.2020.00388
53. Fallarino F, Grohmann U, Vacca C, Bianchi R, Orabona C, Spreca A, et al. T cell apoptosis by tryptophan catabolism. Cell Death Differ (2002) 9(10):1069–77. doi: 10.1038/sj.cdd.4401073
54. Suss G, Shortman K. A subclass of dendritic cells kills CD4 T cells via Fas/Fas-ligand-induced apoptosis. J Exp Med (1996) 183(4):1789–96. doi: 10.1084/jem.183.4.1789
55. Xu X, Yi H, Guo Z, Qian C, Xia S, Yao Y, et al. Splenic stroma-educated regulatory dendritic cells induce apoptosis of activated CD4 T cells via Fas ligand-enhanced IFN-gamma and nitric oxide. J Immunol (2012) 188(3):1168–77. doi: 10.4049/jimmunol.1101696
56. Li XL, Yang AM, Huang H, Zhang XB, Town J, Davis B, et al. Induction of Type 2 T helper cell allergen tolerance by IL-10-differentiated regulatory dendritic cells. Am J Resp Cell Mol Biol (2010) 42(2):190–9. doi: 10.1165/rcmb.2009-0023OC
57. Gregori S, Tomasoni D, Pacciani V, Scirpoli M, Battaglia M, Magnani CF, et al. Differentiation of type 1 T regulatory cells (Tr1) by tolerogenic DC-10 requires the IL-10-dependent ILT4/HLA-G pathway. Blood (2010) 116(6):935–44. doi: 10.1182/blood-2009-07-234872
58. LeMaoult J, Krawice-Radanne I, Dausset J, Carosella ED. HLA-G1-expressing antigen-presenting cells induce immunosuppressive CD4+ T cells. Proc Natl Acad Sci U S A (2004) 101(18):7064–9. doi: 10.1073/pnas.0401922101
59. Amodio G, Comi M, Tomasoni D, Gianolini ME, Rizzo R, LeMaoult J, et al. HLA-G expression levels influence the tolerogenic activity of human DC-10. Haematologica (2015) 100(4):548–57. doi: 10.3324/haematol.2014.113803
60. Svajger U, Rozman PJ. Synergistic Effects of Interferon-gamma and Vitamin D3 Signaling in Induction of ILT-3(high)PDL-1(high) Tolerogenic Dendritic Cells. Front Immunol (2019) 10:2627. doi: 10.3389/fimmu.2019.02627
61. Song SS, Yuan PF, Wu HX, Chen JY, Fu JJ, Li PP, et al. Dendritic cells with an increased PD-L1 by TGF-beta induce T cell anergy for the cytotoxicity of hepatocellular carcinoma cells. Int Immunopharmacol (2014) 20(1):117–23. doi: 10.1016/j.intimp.2014.02.027
62. Zahorchak AF, Macedo C, Hamm DE, Butterfield LH, Metes DM, Thomson AW, et al. High PD-L1/CD86 MFI ratio and IL-10 secretion characterize human regulatory dendritic cells generated for clinical testing in organ transplantation. Cell Immunol (2018) 323:9–18. doi: 10.1016/j.cellimm.2017.08.008
63. Saul L, Mair I, Ivens A, Brown P, Samuel K, Campbell JDM, et al. 1,25-Dihydroxyvitamin D3 Restrains CD4(+) T Cell Priming Ability of CD11c(+) Dendritic Cells by Upregulating Expression of CD31. Front Immunol (2019) 10:600. doi: 10.3389/fimmu.2019.00600
64. Clement M, Fornasa G, Guedj K, Ben Mkaddem S, Gaston AT, Khallou-Laschet J, et al. CD31 is a key coinhibitory receptor in the development of immunogenic dendritic cells. Proc Natl Acad Sci U S A (2014) 111(12):E1101–10. doi: 10.1073/pnas.1314505111
65. Schulke S. Induction of Interleukin-10 Producing Dendritic Cells As a Tool to Suppress Allergen-Specific T Helper 2 Responses. Front Immunol (2018) 9:455. doi: 10.3389/fimmu.2018.00455
66. Ouyang W, O’Garra A. IL-10 Family Cytokines IL-10 and IL-22: from Basic Science to Clinical Translation. Immunity (2019) 50(4):871–91. doi: 10.1016/j.immuni.2019.03.020
67. Huang H, Dawicki W, Lu M, Nayyar A, Zhang X, Gordon JR. Regulatory dendritic cell expression of MHCII and IL-10 are jointly requisite for induction of tolerance in a murine model of OVA-asthma. Allergy (2013) 68(9):1126–35. doi: 10.1111/all.12203
68. Koya T, Matsuda H, Takeda K, Matsubara S, Miyahara N, Balhorn A, et al. IL-10-treated dendritic cells decrease airway hyperresponsiveness and airway inflammation in mice. J Allergy Clin Immunol (2007) 119(5):1241–50. doi: 10.1016/j.jaci.2007.01.039
69. Lu M, Dawicki W, Zhang X, Huang H, Nayyar A, Gordon JR. Therapeutic induction of tolerance by IL-10-differentiated dendritic cells in a mouse model of house dust mite-asthma. Allergy (2011) 66(5):612–20. doi: 10.1111/j.1398-9995.2010.02526.x
70. Nayyar A, Dawicki W, Huang H, Lu MP, Zhang XB, Gordon JR. Induction of prolonged asthma tolerance by IL-10-differentiated dendritic cells: differential impact on airway hyperresponsiveness and the Th2 immunoinflammatory response. J Immunol (2012) 189(1):72–9. doi: 10.4049/jimmunol.1103286
71. Coomes SM, Kannan Y, Pelly VS, Entwistle LJ, Guidi R, Perez-Lloret J, et al. CD4(+) Th2 cells are directly regulated by IL-10 during allergic airway inflammation. Mucosal Immunol (2017) 10(1):150–61. doi: 10.1038/mi.2016.47
72. Batlle E, Massague J. Transforming Growth Factor-beta Signaling in Immunity and Cancer. Immunity (2019) 50(4):924–40. doi: 10.1016/j.immuni.2019.03.024
73. Anderson AE, Swan DJ, Wong OY, Buck M, Eltherington O, Harry RA, et al. Tolerogenic dendritic cells generated with dexamethasone and vitamin D3 regulate rheumatoid arthritis CD4(+) T cells partly via transforming growth factor-beta1. Clin Exp Immunol (2017) 187(1):113–23. doi: 10.1111/cei.12870
74. Dawicki W, Li CY, Town J, Zhang XB, Gordon JR. Therapeutic reversal of food allergen sensitivity by mature retinoic acid-differentiated dendritic cell induction of LAG3(+)CD49b(-)Foxp3(-) regulatory T cells. J Allergy Clin Immunol (2017) 139(5):1608–20. doi: 10.1016/j.jaci.2016.07.042
75. Qi J, Zhang Z, Tang X, Li W, Chen W, Yao G. IL-27 Regulated CD4(+)IL-10(+) T Cells in Experimental Sjogren Syndrome. Front Immunol (2020) 11:1699. doi: 10.3389/fimmu.2020.01699
76. Feuerer M, Hill JA, Mathis D, Benoist C. Foxp3+ regulatory T cells: differentiation, specification, subphenotypes. Nat Immunol (2009) 10(7):689–95. doi: 10.1038/ni.1760
77. Huang H, Dawicki W, Zhang XB, Town J, Gordon JR. Tolerogenic dendritic cells induce CD4(+)CD25(hi)Foxp3(+) regulatory T cell differentiation from CD4(+)CD25(-/lo)Foxp3(-) effector T cells. J Immunol (2010) 185(9):5003–10. doi: 10.4049/jimmunol.0903446
78. Huang H, Ma Y, Dawicki W, Zhang X, Gordon JR. Comparison of induced versus natural regulatory T cells of the same TCR specificity for induction of tolerance to an environmental antigen. J Immunol (2013) 191(3):1136–43. doi: 10.4049/jimmunol.1201899
79. Roncarolo MG, Gregori S, Bacchetta R, Battaglia M, Gagliani N, et al. The Biology of T Regulatory Type 1 Cells and Their Therapeutic Application in Immune-Mediated Diseases. Immunity (2018) 49(6):1004–19. doi: 10.1016/j.immuni.2018.12.001
80. Carrier Y, Yuan J, Kuchroo VK, Weiner HL. Th3 cells in peripheral tolerance. I. Induction of Foxp3-positive regulatory T cells by Th3 cells derived from TGF-beta T cell-transgenic mice. J Immunol (2007) 178(1):179–85. doi: 10.4049/jimmunol.178.1.179
81. Du X, Chang S, Guo W, Zhang S, Chen ZK. Progress in Liver Transplant Tolerance and Tolerance-Inducing Cellular Therapies. Front Immunol (2020) 11:1326. doi: 10.3389/fimmu.2020.01326
82. Thomson AW, Zahorchak AF, Ezzelarab MB, Butterfield LH, Lakkis FG, Metes DM. Prospective Clinical Testing of Regulatory Dendritic Cells in Organ Transplantation. Front Immunol (2016) 7:15. doi: 10.3389/fimmu.2016.00015
83. Ritprajak P, Kaewraemruaen C, Hirankarn N. Current Paradigms of Tolerogenic Dendritic Cells and Clinical Implications for Systemic Lupus Erythematosus. Cells (2019) 8(10):1291. doi: 10.3390/cells8101291
84. Pellerin L, Jenks JA, Chinthrajah S, Dominguez T, Block W, Zhou X, et al. Peanut-specific type 1 regulatory T cells induced in vitro from allergic subjects are functionally impaired. J Allergy Clin Immunol (2017) 141:202–13. doi: 10.1016/j.jaci.2017.05.045
85. Ruiter B, Smith NP, Fleming E, Patil SU, Hurlburt BK, Maleki SJ, et al. Peanut protein acts as a Th2 adjuvant by inducing RALDH2 in human antigen-presenting cells. J Allergy Clin Immunol (2021). doi: 10.1016/j.jaci.2020.11.047
86. Garin MI, Chu CC, Golshayan D, Cernuda-Morollon E, Wait R, Lechler RI. Galectin-1: a key effector of regulation mediated by CD4+CD25+ T cells. Blood (2007) 109(5):2058–65. doi: 10.1182/blood-2006-04-016451
87. Okeke EB, Uzonna JE. The Pivotal Role of Regulatory T Cells in the Regulation of Innate Immune Cells. Front Immunol (2019) 10:680. doi: 10.3389/fimmu.2019.00680
88. Shevach EM. Mechanisms of foxp3+ T regulatory cell-mediated suppression. Immunity (2009) 30(5):636–45. doi: 10.1016/j.immuni.2009.04.010
89. Zou W. Regulatory T cells, tumour immunity and immunotherapy. Nat Rev Immunol (2006) 6(4):295–307. doi: 10.1038/nri1806
90. Kanamori M, Nakatsukasa H, Okada M, Lu Q, Yoshimura A. Induced Regulatory T Cells: Their Development, Stability, and Applications. Trends Immunol (2016) 37(11):803–11. doi: 10.1016/j.it.2016.08.012
91. Ohkura N, Kitagawa Y, Sakaguchi S. Development and maintenance of regulatory T cells. Immunity (2013) 38(3):414–23. doi: 10.1016/j.immuni.2013.03.002
92. Gravano DM, Vignali DA. The battle against immunopathology: infectious tolerance mediated by regulatory T cells. Cell Mol Life Sci (2012) 69(12):1997–2008. doi: 10.1007/s00018-011-0907-z
93. Liang B, Workman C, Lee J, Chew C, Dale BM, Colonna L, et al. Regulatory T cells inhibit dendritic cells by lymphocyte activation gene-3 engagement of MHC class II. J Immunol (2008) 180(9):5916–26. doi: 10.4049/jimmunol.180.9.5916
94. Awasthi A, Carrier Y, Peron JP, Bettelli E, Kamanaka M, Flavell RA, et al. A dominant function for interleukin 27 in generating interleukin 10-producing anti-inflammatory T cells. Nat Immunol (2007) 8(12):1380–9. doi: 10.1038/ni1541
95. Ilarregui JM, Croci DO, Bianco GA, Toscano MA, Salatino M, Vermeulen ME, et al. Tolerogenic signals delivered by dendritic cells to T cells through a galectin-1-driven immunoregulatory circuit involving interleukin 27 and interleukin 10. Nat Immunol (2009) 10(9):981–91. doi: 10.1038/ni.1772
96. Carrier Y, Yuan J, Kuchroo VK, Weiner HL. Th3 cells in peripheral tolerance. II. TGF-beta-transgenic Th3 cells rescue IL-2-deficient mice from autoimmunity. J Immunol (2007) 178(1):172–8. doi: 10.4049/jimmunol.178.1.172
97. Andersson J, Tran DQ, Pesu M, Davidson TS, Ramsey H, O'Shea JJ, et al. CD4+ FoxP3+ regulatory T cells confer infectious tolerance in a TGF-beta-dependent manner. J Exp Med (2008) 205(9):1975–81. doi: 10.1084/jem.20080308
98. Kim SH, Jung HH, Lee CK. Generation, Characteristics and Clinical Trials of Ex Vivo Generated Tolerogenic Dendritic Cells. Yonsei Med J (2018) 59(7):807–15. doi: 10.3349/ymj.2018.59.7.807
99. Nair S, Archer GE, Tedder TF. Isolation and generation of human dendritic cells. Curr Protoc Immunol (2012) Chapter 7:Unit7 32. doi: 10.1002/0471142735.im0732s99
100. Romani N, Gruner S, Brang D, Kampgen E, Lenz A, Trockenbacher B, et al. Proliferating dendritic cell progenitors in human blood. J Exp Med (1994) 180(1):83–93. doi: 10.1084/jem.180.1.83
101. Zhang J, Supakorndej T, Krambs JR, Rao M, Abou-Ezzi G, Ye RY, et al. Bone marrow dendritic cells regulate hematopoietic stem/progenitor cell trafficking. J Clin Invest (2019) 129(7):2920–31. doi: 10.1172/JCI124829
102. Sachamitr P, Leishman AJ, Davies TJ, Fairchild PJ. Directed Differentiation of Human Induced Pluripotent Stem Cells into Dendritic Cells Displaying Tolerogenic Properties and Resembling the CD141(+) Subset. Front Immunol (2017) 8:1935. doi: 10.3389/fimmu.2017.01935
103. Baghbaderani BA, Tian X, Neo BH, Burkall A, Dimezzo T, Sierra G, et al. cGMP-Manufactured Human Induced Pluripotent Stem Cells Are Available for Pre-clinical and Clinical Applications. Stem Cell Rep (2015) 5(4):647–59. doi: 10.1016/j.stemcr.2015.08.015
104. Choi KD, Vodyanik MA, Slukvin II. Generation of mature human myelomonocytic cells through expansion and differentiation of pluripotent stem cell-derived lin-CD34+CD43+CD45+ progenitors. J Clin Invest (2009) 119(9):2818–29. doi: 10.1172/JCI38591
105. Senju S, Haruta M, Matsumura K, Matsunaga Y, Fukushima S, Ikeda T, et al. Generation of dendritic cells and macrophages from human induced pluripotent stem cells aiming at cell therapy. Gene Ther (2011) 18(9):874–83. doi: 10.1038/gt.2011.22
106. Goriely S, Van Lint C, Dadkhah R, Libin M, De Wit D, Demonte D, et al. A defect in nucleosome remodeling prevents IL-12(p35) gene transcription in neonatal dendritic cells. J Exp Med (2004) 199(7):1011–6. doi: 10.1084/jem.20031272
107. Goriely S, Vincart B, Stordeur P, Vekemans J, Willems F, Goldman M, et al. Deficient IL-12(p35) gene expression by dendritic cells derived from neonatal monocytes. J Immunol (2001) 166(3):2141–6. doi: 10.4049/jimmunol.166.3.2141
108. Kim K, Doi A, Wen B, Ng K, Zhao R, Cahan P, et al. Epigenetic memory in induced pluripotent stem cells. Nature (2010) 467(7313):285–90. doi: 10.1038/nature09342
109. Polo JM, Liu S, Figueroa ME, Kulalert W, Eminli S, Tan KY, et al. Cell type of origin influences the molecular and functional properties of mouse induced pluripotent stem cells. Nat Biotechnol (2010) 28(8):848–55. doi: 10.1038/nbt.1667
110. Schmidt SV, Nino-Castro AC, Schultze JL. Regulatory dendritic cells: there is more than just immune activation. Front Immunol (2012) 3:274. doi: 10.3389/fimmu.2012.00274
111. Banchereau J, Steinman RM. Dendritic cells and the control of immunity. Nature (1998) 392(6673):245–52. doi: 10.1038/32588
112. Horton C, Shanmugarajah K, Fairchild PJ. Harnessing the properties of dendritic cells in the pursuit of immunological tolerance. BioMed J (2017) 40(2):80–93. doi: 10.1016/j.bj.2017.01.002
113. Mellman I, Steinman RM. Dendritic cells: specialized and regulated antigen processing machines. Cell (2001) 106(3):255–8. doi: 10.1016/S0092-8674(01)00449-4
114. Yamazaki S, Bonito AJ, Spisek R, Dhodapkar M, Inaba K, Steinman RM. Dendritic cells are specialized accessory cells along with TGF- for the differentiation of Foxp3+ CD4+ regulatory T cells from peripheral Foxp3 precursors. Blood (2007) 110(13):4293–302. doi: 10.1182/blood-2007-05-088831
115. Nguyen QT, Jang E, Le HT, Kim S, Kim D, Dvorina N, et al. IL-27 targets Foxp3+ Tregs to mediate antiinflammatory functions during experimental allergic airway inflammation. JCI Insight (2019) 4(2):e123216. doi: 10.1172/jci.insight.123216
116. Peng Q, Qiu X, Zhang Z, Zhang S, Zhang Y, Liang Y, et al. PD-L1 on dendritic cells attenuates T cell activation and regulates response to immune checkpoint blockade. Nat Commun (2020) 11(1):4835. doi: 10.1038/s41467-020-18570-x
117. Sun W, Wei JW, Li H, Wei FQ, Li J, Wen WP, et al. Adoptive cell therapy of tolerogenic dendritic cells as inducer of regulatory T cells in allergic rhinitis. Int Forum Allergy Rhinol (2018) 8(11):1291–9. doi: 10.1002/alr.22217
118. Krakow S, Crescimone ML, Bartels C, Wiegering V, Eyrich M, Schlegel PG, et al. Re-expression of CD14 in Response to a Combined IL-10/TLR Stimulus Defines Monocyte-Derived Cells With an Immunoregulatory Phenotype. Front Immunol (2019) 10:1484. doi: 10.3389/fimmu.2019.01484
119. Kryczanowsky F, Raker V, Graulich E, Domogalla MP, Steinbrink K. IL-10-Modulated Human Dendritic Cells for Clinical Use: Identification of a Stable and Migratory Subset with Improved Tolerogenic Activity. J Immunol (2016) 197(9):3607–17. doi: 10.4049/jimmunol.1501769
120. Van Overtvelt L, Lombardi V, Razafindratsita A, Saint-Lu N, Horiot S, Moussu H, et al. IL-10-inducing Adjuvants enhance sublingual immunotherapy efficacy in a murine asthma model. Int Arch Allergy Immunol (2008) 145(2):152–62. doi: 10.1159/000108140
121. Yurchenko E, Shio MT, Huang TC, Da Silva Martins M, Szyf M, Levings MK, et al. Inflammation-driven reprogramming of CD4+ Foxp3+ regulatory T cells into pathogenic Th1/Th17 T effectors is abrogated by mTOR inhibition in vivo. PloS One (2012) 7(4):e35572. doi: 10.1371/journal.pone.0035572
122. Lim DS, Kang MS, Jeong JA, Bae YS. Semi-mature DC are immunogenic and not tolerogenic when inoculated at a high dose in collagen-induced arthritis mice. Eur J Immunol (2009) 39(5):1334–43. doi: 10.1002/eji.200838987
123. Voigtlander C, Rossner S, Cierpka E, Theiner G, Wiethe C, Menges M, et al. Dendritic cells matured with TNF can be further activated in vitro and after subcutaneous injection in vivo which converts their tolerogenicity into immunogenicity. J Immunother (2006) 29(4):407–15. doi: 10.1097/01.cji.0000210081.60178.b4
124. Engman C, Garciafigueroa Y, Phillips BE, Trucco M, Giannoukakis N. Co-Stimulation-Impaired Bone Marrow-Derived Dendritic Cells Prevent Dextran Sodium Sulfate-Induced Colitis in Mice. Front Immunol (2018) 9:894. doi: 10.3389/fimmu.2018.00894
125. Vanherwegen AS, Cook DP, Ferreira GB, Gysemans C, Mathieu C, et al. Vitamin D-modulated dendritic cells delay lethal graft-versus-host disease through induction of regulatory T cells. J Steroid Biochem Mol Biol (2019) 188:103–10. doi: 10.1016/j.jsbmb.2018.12.013
126. Penna G, Roncari A, Amuchastegui S, Daniel KC, Berti E, Colonna M, et al. Expression of the inhibitory receptor ILT3 on dendritic cells is dispensable for induction of CD4+Foxp3+ regulatory T cells by 1,25-dihydroxyvitamin D3. Blood (2005) 106(10):3490–7. doi: 10.1182/blood-2005-05-2044
127. Unger WW, Laban S, Kleijwegt FS, van der Slik AR, Roep BO. Induction of Treg by monocyte-derived DC modulated by vitamin D3 or dexamethasone: differential role for PD-L1. Eur J Immunol (2009) 39(11):3147–59. doi: 10.1002/eji.200839103
128. van der Aar AM, Sibiryak DS, Bakdash G, van Capel TM, van der Kleij HP, Opstelten DJ, et al. Vitamin D3 targets epidermal and dermal dendritic cells for induction of distinct regulatory T cells. J Allergy Clin Immunol (2011) 127(6):1532–40.e7. doi: 10.1016/j.jaci.2011.01.068
129. Kraus LF, Scheurmann N, Frenzel DF, Tasdogan A, Weiss JM. 9-cis-Retinoic acid induces a distinct regulatory dendritic cell phenotype that modulates murine delayed-type allergy. Contact Dermatitis (2018) 78(1):41–54. doi: 10.1111/cod.12868
130. Elizondo DM, Andargie TE, Yang D, Kacsinta AD, Lipscomb MW. Inhibition of Allograft Inflammatory Factor-1 in Dendritic Cells Restrains CD4(+) T Cell Effector Responses and Induces CD25(+)Foxp3(+) T Regulatory Subsets. Front Immunol (2017) 8:1502. doi: 10.3389/fimmu.2017.01502
131. Jimenez RV, Wright TT, Jones NR, Wu J, Gibson AW, Szalai AJ. C-Reactive Protein Impairs Dendritic Cell Development, Maturation, and Function: Implications for Peripheral Tolerance. Front Immunol (2018) 9:372. doi: 10.3389/fimmu.2018.00372
132. Suzuki M, Yokota M, Kanemitsu Y, Min WP, Ozaki S, Nakamura Y. Intranasal administration of regulatory dendritic cells is useful for the induction of nasal mucosal tolerance in a mice model of allergic rhinitis. World Allergy Organ J (2020) 13(8):100447. doi: 10.1016/j.waojou.2020.100447
133. Santos ES, de Aragao-Franca LS, Meira CS, Cerqueira JV, Vasconcelos JF, Nonaka CKV, et al. Tolerogenic Dendritic Cells Reduce Cardiac Inflammation and Fibrosis in Chronic Chagas Disease. Front Immunol (2020) 11:488. doi: 10.3389/fimmu.2020.00488
134. de Aragao-Franca LS, Aragao-Franca LS, Rocha VCJ, Rocha VCJ, Cronemberger-Andrade A, da Costa FHB, et al. Tolerogenic Dendritic Cells Reduce Airway Inflammation in a Model of Dust Mite Triggered Allergic Inflammation. Allergy Asthma Immunol Res (2018) 10(4):406–19. doi: 10.4168/aair.2018.10.4.406
135. Lynch K, Treacy O, Gerlach JQ, Annuk H, Lohan P, Cabral J, et al. Regulating Immunogenicity and Tolerogenicity of Bone Marrow-Derived Dendritic Cells through Modulation of Cell Surface Glycosylation by Dexamethasone Treatment. Front Immunol (2017) 8:1427. doi: 10.3389/fimmu.2017.01427
136. Kim N, Park CS, Im SA, Kim JW, Lee JH, Park YJ, et al. Minocycline promotes the generation of dendritic cells with regulatory properties. Oncotarget (2016) 7(33):52818–31. doi: 10.18632/oncotarget.10810
137. Lee JH, Park CS, Jang S, Kim JW, Kim SH, Song S, et al. Tolerogenic dendritic cells are efficiently generated using minocycline and dexamethasone. Sci Rep (2017) 7(1):15087. doi: 10.1038/s41598-017-15569-1
138. Wan J, Huang F, Hao S, Hu W, Liu C, Zhang W, et al. Interleukin-10 Gene-Modified Dendritic Cell-Induced Type 1 Regulatory T Cells Induce Transplant-Tolerance and Impede Graft Versus Host Disease After Allogeneic Stem Cell Transplantation. Cell Physiol Biochem (2017) 43(1):353–66. doi: 10.1159/000480415
139. Wu H, Lo Y, Chan A, Law KS, Mok MY. Rel B-modified dendritic cells possess tolerogenic phenotype and functions on lupus splenic lymphocytes in vitro. Immunology (2016) 149(1):48–61. doi: 10.1111/imm.12628
140. Wu H, Xu Z, Wang Z, Ren Z, Li L, Ruan Y. Dendritic cells with METTL3 gene knockdown exhibit immature properties and prolong allograft survival. Genes Immun (2020) 21(3):193–202. doi: 10.1038/s41435-020-0099-3
141. Jansen MAA, Spiering R, Ludwig IS, van Eden W, Hilkens CMU, Broere F. Matured Tolerogenic Dendritic Cells Effectively Inhibit Autoantigen Specific CD4(+) T Cells in a Murine Arthritis Model. Front Immunol (2019) 10:2068. doi: 10.3389/fimmu.2019.02068
142. Ezzelarab MB, Raich-Regue D, Lu L, Zahorchak AF, Perez-Gutierrez A, Humar A, et al. Renal Allograft Survival in Nonhuman Primates Infused With Donor Antigen-Pulsed Autologous Regulatory Dendritic Cells. Am J Transplant (2017) 17(6):1476–89. doi: 10.1111/ajt.14182
143. Zahorchak AF, Kean LS, Tokita D, Turnquist HR, Abe M, Finke J, et al. Infusion of stably immature monocyte-derived dendritic cells plus CTLA4Ig modulates alloimmune reactivity in rhesus macaques. Transplantation (2007) 84(2):196–206. doi: 10.1097/01.tp.0000268582.21168.f6
144. Zahorchak AF, Raimondi G, Thomson AW. Rhesus monkey immature monocyte-derived dendritic cells generate alloantigen-specific regulatory T cells from circulating CD4+CD127-/lo T cells. Transplantation (2009) 88(9):1057–64. doi: 10.1097/TP.0b013e3181ba6b1f
145. Ezzelarab MB, Lu L, Shufesky WF, Morelli AE, Thomson AW. Donor-Derived Regulatory Dendritic Cell Infusion Maintains Donor-Reactive CD4(+)CTLA4(hi) T Cells in Non-Human Primate Renal Allograft Recipients Treated with CD28 Co-Stimulation Blockade. Front Immunol (2018) 9:250. doi: 10.3389/fimmu.2018.00250
146. Ezzelarab MB, Zahorchak AF, Lu L, Morelli AE, Chalasani G, Demetris AJ, et al. Regulatory dendritic cell infusion prolongs kidney allograft survival in nonhuman primates. Am J Transplant (2013) 13(8):1989–2005. doi: 10.1111/ajt.12310
147. Wang G, Zhang J, Fang Y, Cao W, Xu B, Chen X. Stimulation of tolerogenic dendritic cells using dexamethasone and 1,25-dihydroxyvitamin D3 represses autologous T cell activation and chondrocyte inflammation. Exp Ther Med (2019) 17(1):679–88. doi: 10.3892/etm.2018.7036
148. Djedovic N, Mansilla MJ, Jevtic B, Navarro-Barriuso J, Saksida T, Martinez-Caceres EM, et al. Ethyl Pyruvate Induces Tolerogenic Dendritic Cells. Front Immunol (2019) 10:157. doi: 10.3389/fimmu.2019.00157
149. Comi M, Amodio G, Passeri L, Fortunato M, Santoni de Sio FR, Andolfi G, et al. Generation of Powerful Human Tolerogenic Dendritic Cells by Lentiviral-Mediated IL-10 Gene Transfer. Front Immunol (2020) 11:1260. doi: 10.3389/fimmu.2020.01260
150. Agrawal S, Ganguly S, Tran A, Sundaram P, Agrawal A. Retinoic acid treated human dendritic cells induce T regulatory cells via the expression of CD141 and GARP which is impaired with age. Aging (Albany NY) (2016) 8(6):1223–35. doi: 10.18632/aging.100973
151. Richardson JR, Armbruster NS, Gunter M, Henes J, Autenrieth SE, et al. Staphylococcus aureus PSM Peptides Modulate Human Monocyte-Derived Dendritic Cells to Prime Regulatory T Cells. Front Immunol (2018) 9:2603. doi: 10.3389/fimmu.2018.02603
152. Sato K, Yamashita N, Matsuyama T. Human peripheral blood monocyte-derived interleukin-10-induced semi-mature dendritic cells induce anergic CD4(+) and CD8(+) T cells via presentation of the internalized soluble antigen and cross-presentation of the phagocytosed necrotic cellular fragments. Cell Immunol (2002) 215(2):186–94. doi: 10.1016/S0008-8749(02)00021-7
153. Enk AH, Jonuleit H, Saloga J, Knop J. Dendritic cells as mediators of tumor-induced tolerance in metastatic melanoma. Int J Cancer (1997) 73(3):309–16. doi: 10.1002/(SICI)1097-0215(19971104)73:3<309::AID-IJC1>3.0.CO;2-3
154. Steinbrink K, Wolfl M, Jonuleit H, Knop J, Enk AH. Induction of tolerance by IL-10-treated dendritic cells. J Immunol (1997) 159(10):4772–80.
155. Bellinghausen I, Brand U, Steinbrink K, Enk AH, Knop J, Saloga J, et al. Inhibition of human allergic T-cell responses by IL-10-treated dendritic cells: differences from hydrocortisone-treated dendritic cells. J Allergy Clin Immunol (2001) 108(2):242–9. doi: 10.1067/mai.2001.117177
156. Boks MA, Kager-Groenland JR, Haasjes MS, Zwaginga JJ, van Ham SM, ten Brinke A. IL-10-generated tolerogenic dendritic cells are optimal for functional regulatory T cell induction–a comparative study of human clinical-applicable DC. Clin Immunol (2012) 142(3):332–42. doi: 10.1016/j.clim.2011.11.011
157. Chen L, Qiu M, He W, Huang A, Liu J. Functional study of immature dendritic cells co-transfected with IL-10 and TGF-beta 1 genes in vitro. Mol Biol Rep (2012) 39(6):6633–9. doi: 10.1007/s11033-012-1468-4
158. Figueroa-Vega N, Galindo-Rodriguez G, Bajana S, Portales-Perez D, Abud-Mendoza C, Sanchez-Torres C, et al. Phenotypic analysis of IL-10-treated, monocyte-derived dendritic cells in patients with systemic lupus erythematosus. Scand J Immunol (2006) 64(6):668–76. doi: 10.1111/j.1365-3083.2006.01849.x
159. Henry E, Desmet CJ, Garze V, Fievez L, Bedoret D, Heirman C, et al. Dendritic cells genetically engineered to express IL-10 induce long-lasting antigen-specific tolerance in experimental asthma. J Immunol (2008) 181(10):7230–42. doi: 10.4049/jimmunol.181.10.7230
160. Menges M, Rossner S, Voigtlander C, Schindler H, Kukutsch NA, Bogdan C, et al. Repetitive injections of dendritic cells matured with tumor necrosis factor alpha induce antigen-specific protection of mice from autoimmunity. J Exp Med (2002) 195(1):15–21. doi: 10.1084/jem.20011341
161. Sato K, Yamashita N, Baba M, Matsuyama T. Modified myeloid dendritic cells act as regulatory dendritic cells to induce anergic and regulatory T cells. Blood (2003) 101(9):3581–9. doi: 10.1182/blood-2002-09-2712
162. Rutella S, Bonanno G, Procoli A, Mariotti A, de Ritis DG, Curti A, et al. Hepatocyte growth factor favors monocyte differentiation into regulatory interleukin (IL)-10++IL-12low/neg accessory cells with dendritic-cell features. Blood (2006) 108(1):218–27. doi: 10.1182/blood-2005-08-3141
163. Rutella S, Danese S, Leone G. Tolerogenic dendritic cells: cytokine modulation comes of age. Blood (2006) 108(5):1435–40. doi: 10.1182/blood-2006-03-006403
164. Torres-Aguilar H, Aguilar-Ruiz SR, Gonzalez-Perez G, Munguia R, Bajana S, Meraz-Rios MA, et al. Tolerogenic dendritic cells generated with different immunosuppressive cytokines induce antigen-specific anergy and regulatory properties in memory CD4+ T cells. J Immunol (2010) 184(4):1765–75. doi: 10.4049/jimmunol.0902133
165. Zhang-Hoover J, Finn P, Stein-Streilein J. Modulation of ovalbumin-induced airway inflammation and hyperreactivity by tolerogenic APC. J Immunol (2005) 175(11):7117–24. doi: 10.4049/jimmunol.175.11.7117
166. Piemonti L, Monti P, Allavena P, Sironi M, Soldini L, Leone BE, et al. Glucocorticoids affect human dendritic cell differentiation and maturation. J Immunol (1999) 162(11):6473–81.
167. Naranjo-Gomez M, Raich-Regue D, Onate C, Grau-Lopez L, Ramo-Tello C, Pujol-Borrell R, et al. Comparative study of clinical grade human tolerogenic dendritic cells. J Transl Med (2011) 9:89. doi: 10.1186/1479-5876-9-89
168. Xia CQ, Peng R, Beato F, Clare-Salzler MJ. Dexamethasone induces IL-10-producing monocyte-derived dendritic cells with durable immaturity. Scand J Immunol (2005) 62(1):45–54. doi: 10.1111/j.1365-3083.2005.01640.x
169. Penna G, Adorini L. 1 Alpha,25-dihydroxyvitamin D3 inhibits differentiation, maturation, activation, and survival of dendritic cells leading to impaired alloreactive T cell activation. J Immunol (2000) 164(5):2405–11. doi: 10.4049/jimmunol.164.5.2405
170. Chamorro S, Garcia-Vallejo JJ, Unger WW, Fernandes RJ, Bruijns SC, Laban S, et al. TLR triggering on tolerogenic dendritic cells results in TLR2 up-regulation and a reduced proinflammatory immune program. J Immunol (2009) 183(5):2984–94. doi: 10.4049/jimmunol.0801155
171. Ferreira GB, Kleijwegt FS, Waelkens E, Lage K, Nikolic T, Hansen DA, et al. Differential protein pathways in 1,25-dihydroxyvitamin d(3) and dexamethasone modulated tolerogenic human dendritic cells. J Proteome Res (2012) 11(2):941–71. doi: 10.1021/pr200724e
172. Ferreira GB, van Etten E, Verstuyf A, Waer M, Overbergh L, Gysemans C, et al. 1,25-Dihydroxyvitamin D3 alters murine dendritic cell behaviour in vitro and in vivo. Diabetes Metab Res Rev (2011) 27(8):933–41. doi: 10.1002/dmrr.1275
173. Macedo C, Turquist H, Metes D, Thomson AW. Immunoregulatory properties of rapamycin-conditioned monocyte-derived dendritic cells and their role in transplantation. Transplant Res (2012) 1(1):16. doi: 10.1186/2047-1440-1-16
174. Li X, Li JJ, Yang JY, Wang DS, Zhao W, Song WJ, et al. Tolerance induction by exosomes from immature dendritic cells and rapamycin in a mouse cardiac allograft model. PloS One (2012) 7(8):e44045. doi: 10.1371/journal.pone.0044045
175. Gonzalez-Rey E, Chorny A, Fernandez-Martin A, Ganea D, Delgado M. Vasoactive intestinal peptide generates human tolerogenic dendritic cells that induce CD4 and CD8 regulatory T cells. Blood (2006) 107(9):3632–8. doi: 10.1182/blood-2005-11-4497
176. Toscano MG, Delgado M, Kong W, Martin F, Skarica M, Ganea D, et al. Dendritic cells transduced with lentiviral vectors expressing VIP differentiate into VIP-secreting tolerogenic-like DCs. Mol Ther (2010) 18(5):1035–45. doi: 10.1038/mt.2009.293
177. Joffre O, Nolte MA, Sporri R, Reis e Sousa C, et al. Inflammatory signals in dendritic cell activation and the induction of adaptive immunity. Immunol Rev (2009) 227(1):234–47. doi: 10.1111/j.1600-065X.2008.00718.x
178. Qian C, Cao X. Dendritic cells in the regulation of immunity and inflammation. Semin Immunol (2018) 35:3–11. doi: 10.1016/j.smim.2017.12.002
179. Yurchenko E, Levings MK, Piccirillo CA. CD4+ Foxp3+ regulatory T cells suppress gammadelta T-cell effector functions in a model of T-cell-induced mucosal inflammation. Eur J Immunol (2011) 41(12):3455–66. doi: 10.1002/eji.201141814
180. Shim JU, Lee SE, Hwang W, Lee C, Park JW, Sohn JH, et al. Flagellin suppresses experimental asthma by generating regulatory dendritic cells and T cells. J Allergy Clin Immunol (2016) 137(2):426–35. doi: 10.1016/j.jaci.2015.07.010
181. Kim HS, Park KH, Lee HK, Kim JS, Kim YG, Lee JH, et al. Curdlan activates dendritic cells through dectin-1 and toll-like receptor 4 signaling. Int Immunopharmacol (2016) 39:71–8. doi: 10.1016/j.intimp.2016.07.013
182. Kawashima S, Hirose K, Iwata A, Takahashi K, Ohkubo A, Tamachi T, et al. beta-glucan curdlan induces IL-10-producing CD4+ T cells and inhibits allergic airway inflammation. J Immunol (2012) 189(12):5713–21. doi: 10.4049/jimmunol.1201521
183. Arrieta MC, Stiemsma LT, Dimitriu PA, Thorson L, Russell S, Yurist-Doutsch S, et al. Early infancy microbial and metabolic alterations affect risk of childhood asthma. Sci Transl Med (2015) 7(307):307ra152. doi: 10.1126/scitranslmed.aab2271
184. Pujari R, Banerjee G. Impact of prebiotics on immune response: from the bench to the clinic. Immunol Cell Biol (2020). doi: 10.1111/imcb.12409
185. Yang J, Ren F, Zhang H, Jiang L, Hao Y, Luo X. Induction of Regulatory Dendritic Cells by Lactobacillus paracasei L9 Prevents Allergic Sensitization to Bovine beta-Lactoglobulin in Mice. J Microbiol Biotechnol (2015) 25(10):1687–96. doi: 10.4014/jmb.1503.03022
186. Verma R, Lee C, Jeun EJ, Yi J, Kim KS, Ghosh A, et al. Cell surface polysaccharides of Bifidobacterium bifidum induce the generation of Foxp3(+) regulatory T cells. Sci Immunol (2018) 3(28):eaat6975. doi: 10.1126/sciimmunol.aat6975
187. Fu L, Song J, Wang C, Fu S, Wang Y. Bifidobacterium infantis potentially alleviates shrimp tropomyosin-induced allergy by tolerogenic dendritic cell-dependent induction of regulatory T cells and alterations in gut microbiota. Front Immunol (2017) 8:1536. doi: 10.3389/fimmu.2017.01536
188. Shen Y, Giardino Torchia ML, Lawson GW, Karp CL, Ashwell JD, Mazmanian SK. Outer membrane vesicles of a human commensal mediate immune regulation and disease protection. Cell Host Microbe (2012) 12(4):509–20. doi: 10.1016/j.chom.2012.08.004
189. Durant L, Stentz R, Noble A, Brooks J, Gicheva N, Reddi D, et al. Bacteroides thetaiotaomicron-derived outer membrane vesicles promote regulatory dendritic cell responses in health but not in inflammatory bowel disease. Microbiome (2020) 8(1):88. doi: 10.1186/s40168-020-00868-z
190. Gudmundsdottir AB, Brynjolfsdottir A, Olafsdottir ES, Hardardottir I, Freysdottir J. Exopolysaccharides from Cyanobacterium aponinum induce a regulatory dendritic cell phenotype and inhibit SYK and CLEC7A expression in dendritic cells, T cells and keratinocytes. Int Immunopharmacol (2019) 69:328–36. doi: 10.1016/j.intimp.2019.01.044
191. Gudmundsdottir AB, Omarsdottir S, Brynjolfsdottir A, Paulsen BS, Olafsdottir ES, Freysdottir J, et al. Exopolysaccharides from Cyanobacterium aponinum from the Blue Lagoon in Iceland increase IL-10 secretion by human dendritic cells and their ability to reduce the IL-17+RORgammat+/IL-10+FoxP3+ ratio in CD4+ T cells. Immunol Lett (2015) 163(2):157–62. doi: 10.1016/j.imlet.2014.11.008
192. Thomson AW, Ezzelarab MB. Generation and functional assessment of nonhuman primate regulatory dendritic cells and their therapeutic efficacy in renal transplantation. Cell Immunol (2020) 351:104087. doi: 10.1016/j.cellimm.2020.104087
193. Giannoukakis N, Phillips B, Finegold D, Harnaha J, Trucco M, Phase I. Phase I (safety) study of autologous tolerogenic dendritic cells in type 1 diabetic patients. Diabetes Care (2011) 34(9):2026–32. doi: 10.2337/dc11-0472
194. Joo YB, Park JE, Choi CB, Choi J, Jang JA, Heo M, et al. Phase 1 Study of Immunotherapy Using Autoantigen-Loaded Dendritic Cells in Patients with Anti-Citrullinated Peptide Antigen Positive Rheumatoid Arthritis. Arthritis Rheumatol (2014) 66:S420–1.
195. Benham H, Nel HJ, Law SC, Mehdi AM, Street S, Ramnoruth N, et al. Citrullinated peptide dendritic cell immunotherapy in HLA risk genotype-positive rheumatoid arthritis patients. Sci Trans Med (2015) 7(290):290ra87. doi: 10.1126/scitranslmed.aaa9301
196. Jauregui-Amezaga A, Cabezon R, Ramirez-Morros A, Espana C, Rimola J, Bru C, et al. Intraperitoneal Administration of Autologous Tolerogenic Dendritic Cells for Refractory Crohn’s Disease: A Phase I Study. J Crohns Colitis (2015) 9(12):1071–8. doi: 10.1093/ecco-jcc/jjv144
197. Bell GM, Anderson AE, Diboll J, Reece R, Eltherington O, Harry RA, et al. Autologous tolerogenic dendritic cells for rheumatoid and inflammatory arthritis. Ann Rheum Dis (2017) 76(1):227–34. doi: 10.1136/annrheumdis-2015-208456
198. Zubizarreta I, Florez-Grau G, Vila G, Cabezon R, Espana C, Andorra M, et al. Immune tolerance in multiple sclerosis and neuromyelitis optica with peptide-loaded tolerogenic dendritic cells in a phase 1b trial. Proc Natl Acad Sci U S A (2019) 116(17):8463–70. doi: 10.1073/pnas.1820039116
199. Macedo C, Tran LM, Zahorchak AF, Dai H, Gu X, Ravichandran R, et al. Donor-derived regulatory dendritic cell infusion results in host cell cross-dressing and T cell subset changes in prospective living donor liver transplant recipients. Am J Transplant (2020). doi: 10.1111/ajt.16393
200. Ten Brinke A, Martinez-Llordella M, Cools N, Hilkens CMU, van Ham SM, Sawitzki B, et al. Ways Forward for Tolerance-Inducing Cellular Therapies- an AFACTT Perspective. Front Immunol (2019) 10:181. doi: 10.3389/fimmu.2019.00181
201. Lundberg K, Albrekt AS, Nelissen I, Santegoets S, de Gruijl TD, Gibbs S, et al. Transcriptional profiling of human dendritic cell populations and models–unique profiles of in vitro dendritic cells and implications on functionality and applicability. PloS One (2013) 8(1):e52875. doi: 10.1371/journal.pone.0052875
202. Bol KF, Schreibelt G, Rabold K, Wculek SK, Schwarze JK, Dzionek A, et al. The clinical application of cancer immunotherapy based on naturally circulating dendritic cells. J Immunother Cancer (2019) 7(1):109. doi: 10.1186/s40425-019-0580-6
203. Adnan E, Matsumoto T, Ishizaki J, Onishi S, Suemori K, Yasukawa M, et al. Human tolerogenic dendritic cells generated with protein kinase C inhibitor are optimal for functional regulatory T cell induction - A comparative study. Clin Immunol (2016) 173:96–108. doi: 10.1016/j.clim.2016.09.007
204. Hasegawa H, Matsumoto T. Mechanisms of Tolerance Induction by Dendritic Cells In Vivo. Front Immunol (2018) 9:350. doi: 10.3389/fimmu.2018.00350
205. Funda DP, Palova-Jelinkova L, Golias J, Kroulikova Z, Fajstova A, Hudcovic T, et al. Optimal Tolerogenic Dendritic Cells in Type 1 Diabetes (T1D) Therapy: What Can We Learn From Non-obese Diabetic (NOD) Mouse Models? Front Immunol (2019) 10:967. doi: 10.3389/fimmu.2019.00967
206. Gueguen C, Bouley J, Moussu H, Luce S, Duchateau M, Chamot-Rooke J, et al. Changes in markers associated with dendritic cells driving the differentiation of either TH2 cells or regulatory T cells correlate with clinical benefit during allergen immunotherapy. J Allergy Clin Immunol (2016) 137(2):545–58. doi: 10.1016/j.jaci.2015.09.015
207. Navarro-Barriuso J, Mansilla MJ, Naranjo-Gomez M, Sanchez-Pla A, Quirant-Sanchez B, Teniente-Serra A, et al. Comparative transcriptomic profile of tolerogenic dendritic cells differentiated with vitamin D3, dexamethasone and rapamycin. Sci Rep (2018) 8(1):14985. doi: 10.1038/s41598-018-33248-7
208. Nikolic T, Woittiez NJC, van der Slik A, Laban S, Joosten A, Gysemans C, et al. Differential transcriptome of tolerogenic versus inflammatory dendritic cells points to modulated T1D genetic risk and enriched immune regulation. Genes Immun (2017) 18(3):176–83. doi: 10.1038/gene.2017.18
209. Garcia-Gonzalez PA, Schinnerling K, Sepulveda-Gutierrez A, Maggi J, Mehdi AM, Nel HJ, et al. Dexamethasone and Monophosphoryl Lipid A Induce a Distinctive Profile on Monocyte-Derived Dendritic Cells through Transcriptional Modulation of Genes Associated With Essential Processes of the Immune Response. Front Immunol (2017) 8:1350. doi: 10.3389/fimmu.2017.01350
210. Zimmer A, Bouley J, Le Mignon M, Pliquet E, Horiot S, Turfkruyer M, et al. A regulatory dendritic cell signature correlates with the clinical efficacy of allergen-specific sublingual immunotherapy. J Allergy Clin Immunol (2012) 129(4):1020–30. doi: 10.1016/j.jaci.2012.02.014
211. Navarro-Barriuso J, Mansilla MJ, Martinez-Caceres EM. Searching for the Transcriptomic Signature of Immune Tolerance Induction-Biomarkers of Safety and Functionality for Tolerogenic Dendritic Cells and Regulatory Macrophages. Front Immunol (2018) 9:2062. doi: 10.3389/fimmu.2018.02062
Keywords: tolerance, dendritic cell, regulatory T cell, immunologic disease, mechanism
Citation: Ness S, Lin S and Gordon JR (2021) Regulatory Dendritic Cells, T Cell Tolerance, and Dendritic Cell Therapy for Immunologic Disease. Front. Immunol. 12:633436. doi: 10.3389/fimmu.2021.633436
Received: 25 November 2020; Accepted: 18 January 2021;
Published: 10 March 2021.
Edited by:
Fang Zhou, CAS Lamvac Biotech Co., Ltd., ChinaReviewed by:
Silvia Gregori, San Raffaele Telethon Institute for Gene Therapy (SR-Tiget), ItalyCopyright © 2021 Ness, Lin and Gordon. This is an open-access article distributed under the terms of the Creative Commons Attribution License (CC BY). The use, distribution or reproduction in other forums is permitted, provided the original author(s) and the copyright owner(s) are credited and that the original publication in this journal is cited, in accordance with accepted academic practice. No use, distribution or reproduction is permitted which does not comply with these terms.
*Correspondence: John R. Gordon, am9obi5nb3Jkb25AdXNhc2suY2E=
Disclaimer: All claims expressed in this article are solely those of the authors and do not necessarily represent those of their affiliated organizations, or those of the publisher, the editors and the reviewers. Any product that may be evaluated in this article or claim that may be made by its manufacturer is not guaranteed or endorsed by the publisher.
Research integrity at Frontiers
Learn more about the work of our research integrity team to safeguard the quality of each article we publish.