- 1IRMB, University of Montpellier, INSERM, CHU Montpellier, Montpellier, France
- 2Clinical Immunology and Osteoarticular Diseases Therapeutic Unit, Department of Rheumatology, Lapeyronie University Hospital, Montpellier, France
Mesenchymal stromal cells (MSCs) are the most commonly tested adult progenitor cells in regenerative medicine. They stimulate tissue repair primarily through the secretion of immune-regulatory and pro-regenerative factors. There is increasing evidence that most of these factors are carried on extracellular vesicles (EVs) that are released by MSCs, either spontaneously or after activation. Exosomes and microvesicles are the most investigated types of EVs that act through uptake by target cells and cargo release inside the cytoplasm or through interactions with receptors expressed on target cells to stimulate downstream intracellular pathways. They convey different types of molecules, including proteins, lipids and acid nucleics among which, miRNAs are the most widely studied. The cargo of EVs can be impacted by the culture or environmental conditions that MSCs encounter and by changes in the energy metabolism that regulate the functional properties of MSCs. On the other hand, MSC-derived EVs are also reported to impact the metabolism of target cells. In the present review, we discuss the role of MSC-EVs in the regulation of the energy metabolism and oxidative stress of target cells and tissues with a focus on the role of miRNAs.
Introduction
Mitochondria are complex organelles that play a central role in energy metabolism, biosynthetic processes and control of stress responses. Mitochondrial function or ability to generate energy through OXPHOS (oxidative phosphorylation) is vital for cell homeostasis. Mitochondrial dysfunctions are a hallmark of many diseases including metabolic disorders, cardiomyopathies, neurodegenerative diseases and cancer, tightly associated with programmed cell death (apoptosis). The regulation of mitochondrial metabolism is therefore essential to maintain tissue homeostasis (1).
Mesenchymal stromal cells (MSCs) have been shown to impact mitochondrial function [for review, see (2)]. MSCs are multipotent adult progenitor cells first identified in the bone marrow (BM) (3). In addition to BM, MSCs have been described and isolated from several tissues, including adipose tissue, umbilical cord, placenta, or dental pulp (4). The International Society for Cellular Therapy (ISCT) proposes three criteria to define MSCs: i) their adherence to plastic, ii) their immunophenotype CD105+, CD73+, CD90+, and CD45-, CD34-, CD14-, or CD11b-, CD79a- or CD19-, HLA-DR-, and iii) their capacity to differentiate into osteoblasts, adipocytes and chondrocytes (5). MSCs express varying levels of tissue factor (TF/CD142) depending on the tissue source, which may trigger instant blood-mediated inflammatory reaction (IBMIR) and should be checked before clinical application when intravascular delivery is intended (6). MSCs are also characterized by their paracrine function: i) they support survival and differentiation of hematopoietic stromal cells, ii) induce cell proliferation and iii) have anti-fibrotic, anti-apoptotic, pro-angiogenic, anti-bacterial and anti-inflammatory functions (7). Although MSCs from different tissue sources share similar properties, they may display differences in their differentiation potential or trophic capacity (8, 9). They are the most commonly used cells in tissue engineering and regenerative medicine by promoting tissue repair through different mechanisms and function by both cell contact-dependent and independent mechanisms. It is generally assumed that a large share of their effector function is primarily mediated through both cell surface presentation or extracellular secretion of both cell-bound and soluble trophic and immunomodulatory factors (10, 11). These factors can be secreted as single factors or contained within extracellular vesicles (EVs), which are loaded with a complex cargo and mediate their effector functions after entrapment by target cells (12).
EVs are a heterogeneous family of vesicles characterized by a lipid bilayer. On the basis of their biogenesis and size, they are classified into three major subtypes: exosomes (<150 nm) released from the endosomal compartment, microvesicles or microparticles (>150 nm) produced by budding from the plasma membrane and apoptotic bodies originating from disassembling apoptotic cells (13). EVs are major actors in extracellular communication via the delivery of their cargo either by fusion with the plasma membrane of target cells or by endocytosis and release into the cytosol (14). EVs contains proteins, lipids and nucleic acids, including mRNA, lncRNA, miRNA, whose identity and quantity vary according to the parental cell and the physiologic environment [for review, see (15)]. MiRNAs are small noncoding RNAs acting as post-transcriptional regulators of gene expression. The role of miRNAs in the therapeutic function of MSC-derived EVs has been widely investigated and demonstrated in a number of diseases [for review, see (16)]. miRNA can target genes involved in mitochondrial function. In this review, we propose to discuss the impact of MSC-EVs on mitochondrial metabolism and the role played by miRNAs in this regulation.
Mitochondrial Metabolism
The Mitochondrial Organelle: Structure and Function
Mitochondria are found in most eukaryotic cells and are the cell energy-producing organelles. There are up to 2,000 per cell (according to cell type), and are preferentially located in adenosine triphosphate (ATP)-consuming cellular areas. They are small organelles (0.5 to 1 µM) surrounded by a double membrane; each membrane being composed of a phospholipid bilayer (17). The two membranes, the outer (OMM) and the inner mitochondrial membrane (INM), delimit three media: the extra-mitochondrial medium (cytoplasm of the cell), the mitochondrial intermembrane space (IMS), and the mitochondrial matrix (Figure 1).
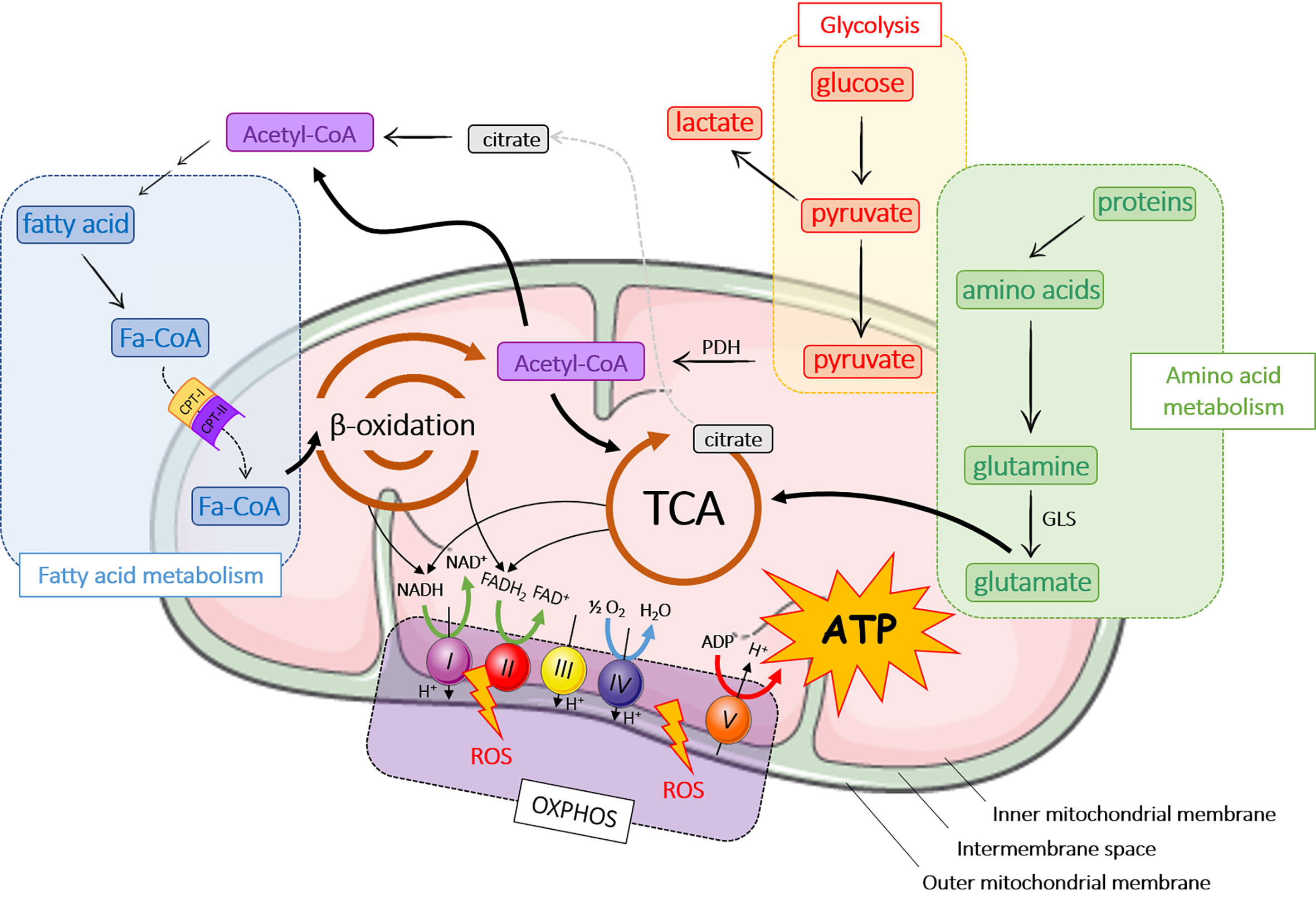
Figure 1 Major metabolic pathways in mitochondria. ADP, adenosine diphosphate; ATP, adenosine triphosphate; CPT, carnitine palmitoyltransferase; FADH2, flavin adenine dinucleotide; GLS, glutaminase; OXPHOS, oxidative phosphorylation; NADH, nicotinamide adenine dinucleotide hydrogenase; PDH, pyruvate dehydrogenase; ROS, reactive oxygen species; TCA, tricarboxylic acid cycle.
Mitochondria contribute to the cellular respiration processes through ATP production. ATP is the ubiquitous energy molecule used in a very large number of cell metabolic reactions [for review, see (18, 19)]. Apart from their involvement in ATP production, mitochondria play also a key role in cell signalling, differentiation, cell death, and control of cell growth (20). Mitochondria have their own genome, transcription and translation systems, but they also require proteins encoded by the nucleus to be functionally active (21). Mitochondria are therefore in the core of several biological processes, but are also involved in mitochondrial dysregulation-based diseases.
Mitochondrial Metabolic Pathways
The main role of mitochondria is to produce energy through ATP release following a series of chemical reactions, better known as the TriCarboxylic Acid cycle (TCA) or Krebs cycle (Figure 1). ATP is also generated from fatty acid metabolism and β-oxidation, OXPHOS and amino acid metabolism (Figure 1). In aerobic organisms, cellular energy mostly derived from glycolysis that converts glucose into pyruvate in the cytoplasm. Pyruvate enters mitochondria where it is converted into acetyl-CoA by pyruvate dehydrogenase (PDH) and then into citrate during the first step of TCA. TCA cycle is composed of ten steps catalyzed by eight different enzymes that produce nicotinamide adenine dinucleotide hydrogenase (NADH) and flavin adenine dinucleotide (FADH2) that are oxidized by the respiratory chain or OXPHOS to produce ATP. OXPHOS consists of the electron transport chain (ETC), which includes a series of protein complexes in the IMS: NADH-dehydrogenase (complex I), succinate dehydrogenase (complex II), ubiquinone, bc1 complex (complex III), cytochrome c and cytochrome c oxidase (CYTC, CCO; complex IV) and ATP synthase (complex V). ETC generates a proton gradient, which drives the synthesis of ATP. During OXPHOS process, mitochondria generate reactive oxygen species (ROS), which participate to cell homeostasis, primarily by the genesis of hypoxia-inducible factors (HIF) (22–24). However, ROS accumulation induces mitochondrial damage and dysfunction leading to cellular modifications.
A part from glycolysis, acetyl-CoA can be produced by the breakdown of lipids (β-oxidation) or proteins (amino acid metabolism) (25). Fatty acid oxidation occurs in mitochondria after fatty acids have crossed mitochondrial membranes under the form of fatty acyl-CoA (Fa-CoA) that is converted into acyl-carnitine by carnitine palmitoyltransferase 1 (CPT-1) in the OMM (26). In the mitochondria, carnitine palmitoyl transferase 2 (CPT-2) removes carnitine from the acyl-carnitine and regenerates acetyl-CoA. During β-oxidation, Fa-CoAs are subsequently cleaved into two carbon segments to synthesize acetyl-CoA. As the final product of β-oxidation, acetyl-CoA takes part in other reactions, primarily TCA cycle and de novo lipid synthesis.
Several pathways of the amino acid metabolism are located in mitochondria. Amino acids are either ketogenic (lysine and leucine), glucogenic (glycine, serine, glutamine…), or both (tyrosine, tryptophan…). Depending on their carbon skeleton, ketogenic amino acids are converted into acetyl-CoA or acetoacetyl-CoA, while glucogenic amino acids are converted into glucose, pyruvate or a TCA cycle intermediate. In this pathway, glutaminase converts glutamine to glutamate, which is further catabolized in TCA cycle (21).
Mitochondria-Related Pathologies
The role of mitochondria in multiple other functions, notably Ca2+ homeostasis, ROS generation and apoptosis, has been shown (27). Mitochondria are therefore in the centre of cell homeostasis and their dysfunctions are associated to pathological conditions (28, 29). Mitochondrial disorders are characterized by defective oxidative phosphorylation and mainly observed in energy-dependent tissues such as skeletal muscle, heart, peripheral, and central nervous system, eyes, kidney or endocrine glands. Dysfunctions include depolarization, ETC inhibition and network fragmentation that impact secretion of metabolites, ROS production and affect cell signalling pathways. Main causes are related to mitochondrial or genomic DNA mutations, which alter the synthesis of ETC enzymatic complexes (30), endoplasmic reticulum stress (31), supercomplex destabilization (32) or mitochondrial protein aggregates (33). Growing evidence has highlighted that oxidative stress, characterized by overproduction of ROS, is also largely involved in mitochondrial disorders. Overproduction of ROS induces mitochondrial DNA mutation, ETC damage, membrane permeability and Ca2+ homeostasis alteration (34). Current treatment strategies investigate gene therapy of mitochondrial DNA (mtDNA) (35) or stem cell transplantation (36). In this context, MSCs have been reported to regulate oxidative stress and redox imbalance and may be of therapeutic interest to counteract mitochondrial disorders (28, 37). In addition, the energetic metabolism of MSCs regulate their immunomodulatory function and consequently their therapeutic properties (38).
Mesenchymal Stromal Cells and Mitochondrial Metabolism of Target Cells
The exact role of MSCs on the mitochondrial metabolism of target cell is still unclear, but co-culture experiments clearly demonstrated the antioxidant properties of MSCs (28). MSCs act directly by reducing the oxidative stress (39) related to injury or inflammation, or indirectly by upregulating the antioxidant defences of target cells and altering cellular bioenergetics (37).
Mesenchymal Stromal Cells Improve the Mitochondrial Function
The MSC potential to attenuate oxidative injury is based on the dampening of ROS production and enhancement of mitochondrial function (37). Reduction of ROS results from the secretion of anti-oxidant components [superoxyde dismutase (SOD), catalase, Glutathione S-transferases (GST), …], which re-equilibrate the redox balance in the host cell (40).
Mesenchymal Stromal Cells Equilibrate Mitochondrial Dynamics
Both fission and fusion processes are linked to mitochondrial metabolism (28). Excessive mitochondrial fission is associated with reduction in OXPHOS and the balance between fusion and fission events is required to maintain cell homeostasis (41–44). Mitochondrial fusion is not essential for cell survival, but for normal development. MSCs can rescue aberrant morphology from a fission- to a fusion-like state and restore OXPHOS, by 962 increasing metabolic capacities and ROS production (28). They can induce expression of mitochondrial fusion genes [mitofusin (mfn1, mfn2) and optic atrophy 1 (Opa1)] protecting cells against environmental damages by improving respiration parameters (45).
Mesenchymal Stromal Cells Minimize Mitochondrial Injury
MSCs can protect injured cells from mitochondria-related apoptosis and resulting oxidative damage by reducing release of CYTC into the cytoplasm. They also secrete a series of cytokines and growth factors, which upregulate anti-apoptotic proteins (BCL-XL, BCL-2), downregulate pro-apoptotic proteins (BAX, BAK, BAD) and CYTC and finally minimize mitochondria injury (2).
Mesenchymal Stromal Cells Accelerate Mitochondria Recovery
Mitochondrial recovery is a strategy for restricting mitochondrial dysfunction and Sirtuin 3 (SIRT3) plays an important role in this process. Through SIRT3 activation and upregulation of peroxisome proliferator-activated receptor gamma coactivator 1-alpha (PGC-1α), mitochondrial biogenesis increases and ROS production decreases (46, 47). MSCs can enhance PGC-1α expression and normalise mitochondrial shape, density and mass (46), thereby re-equilibrating the cell energetic metabolism.
Mesenchymal Stromal Cells Transfer Mitochondrial Cargo
Mitochondrial transfer mechanisms between MSCs and recipient cells are based on cell fusion, EV secretion, gap junctions or tunnelling nanotubes (48). In the acute phase of lung injury, internalized mitochondria were shown to increase ATP concentration in recipient cells, leading to bioenergetics restoration and cell protection (49). MSC mitochondrial transfer plays multiple roles, including tissue repair during injuries, apoptosis prevention in endothelial cells during ischemic stress or metabolic reprogramming (50, 51). Horizontal transfer of mitochondria or mitochondrial DNA between cells address therapeutic applications in MSCs regenerative medicine (48).
Role of miRNAs in Mitochondrial Metabolism
miRNAs: Synthesis, Mechanism, and Function
MicroRNAs (miRNAs) are non-coding endogenous ˜22nt (18 – 25nt) RNAs, which form a large family of post-transcriptional regulators. Within the nucleus, miRNA genes are transcribed by RNA polymerases as long precursor pri-miRNAs (> 500 bases), which harbour stem-loop structures (52). Maturation of miRNAs starts by the nuclear cleavage of the pri-miRNA by the Drosha RNA III endonuclease, which liberates a smaller pre-miRNA molecule. Pre-miRNAs are then exported to the cytoplasm by a GTP-dependent exportin (53). Once in the cytoplasm, pre-miRNAs are cleaved by an enzyme of the DICER family to release a small double-stranded RNA called “miRNA/miRNA duplex”. The passenger strand is generally degraded. The miRNA major strand binds to its mRNA target, which contains a perfectly complementary seed sequence on its 3’ UTR end (52, 54). MiRNAs act by post-transcriptional gene silencing or mRNA degradation and are involved in major biological processes, including cell metabolism (54–57). The process of miRNA and pre-miRNA sorting in EVs remains elusive (58). MiRNAs can diffuse through intracellular space to the plasma membrane and be loaded by the Annexin A2‐dependent pathway (59, 60). MiRNAs can also be transported by RNA-binding proteins (RBPs) toward multivesicular bodies (MVBs) and be packed by budding-in process (61) [for review see (62)]. Several studies indicated that miRNA packaging in EVs could serve as a major mechanism of miRNA transfer between cells. Of interest, there is increasing evidence for the antioxidant role of miRNAs contained within MSC-EVs for different therapeutic applications (12, 63) (Figure 2).
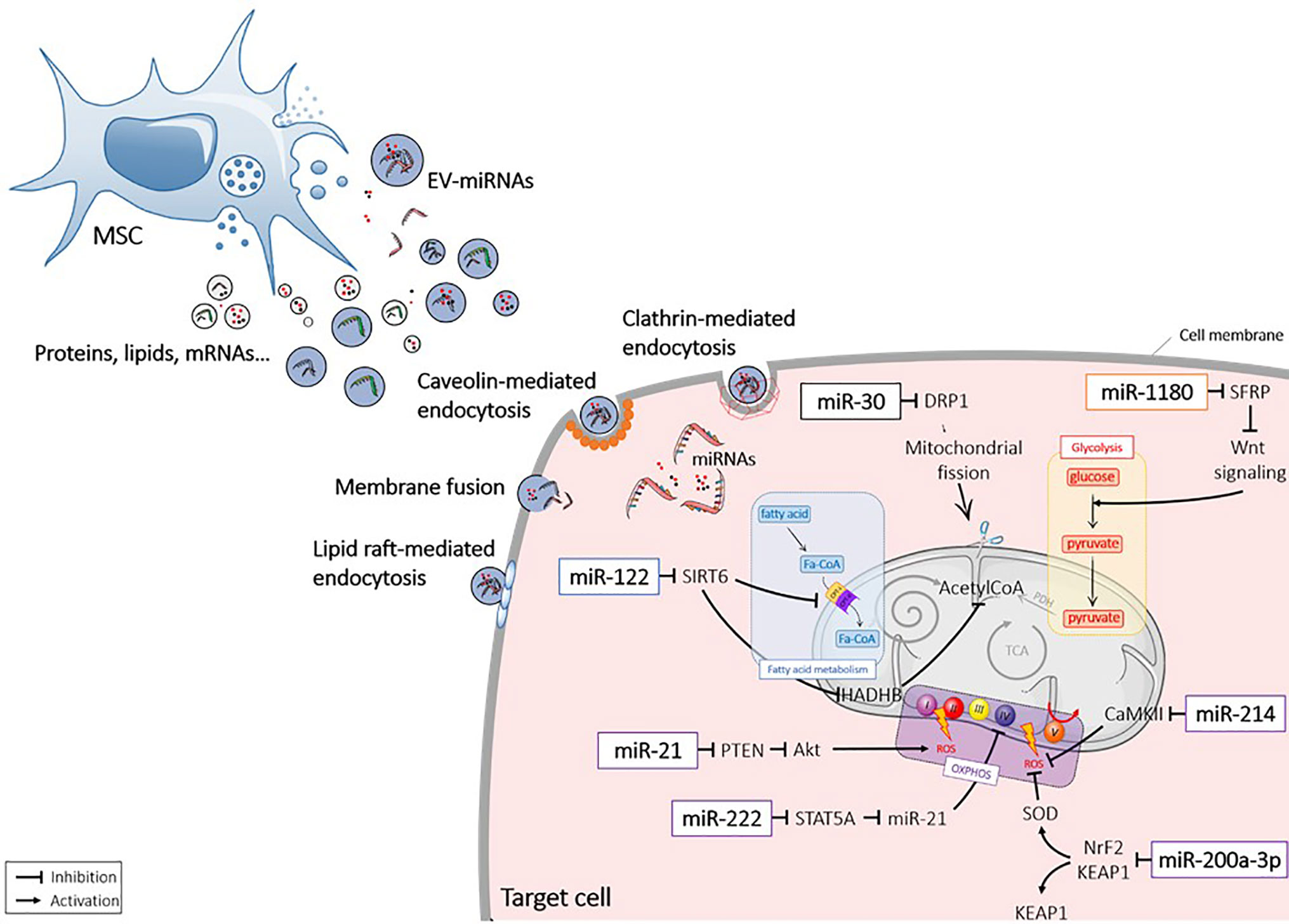
Figure 2 Extracellular vesicle-mediated delivery of miRNAs into recipient cells. Mesenchymal stromal cell (MSC) released extracellular vesicles (EVs) that contain miRNAs (EV-miRNAs). MiRNAs are taken up by the recipient cells via different mechanisms and act in the cytosol by interacting with targets. Akt1, protein kinase B; CamKII, calmodulin-dependent protein kinase II; DRP1, Dynamin-related protein 1; Fa-CoA. Fatty acid coenzyme A; HADHB, Hydroxyacyl-CoA Dehydrogenase Trifunctional Multienzyme Complex Subunit Beta; KEAP1, Kelch-like ECH-associated protein 1; Nrf2, NF-E2 p45-related factor 2; OXPHOS, oxidative phosphorylation; PDH, Pyruvate dehydrogenase; PTEN, Phosphatase and tensin homolog; ROS, reactive oxygen species; SFRP, secreted frizzled-related protein; SIRT, sirtuin; SOD, superoxydes dismutase; STAT, signal transducer and activator of transcription; TCA, tricarboxylic acid cycle; Wnt, wingless.
miRNAs Target Mitochondrial Metabolic Pathways
Recent studies have shown that miRNAs are able to translocate into the mitochondrial compartment and modulate mitochondrial activities (30). A particular miRNA subset, called mitomiR for mitochondrial microRNAs, is localized within mitochondria and transcribed either from nuclear or mitochondrial genome (64). Imported mature mitomiRs are translocated after pre-miRNAs are processed by DICER (65). Among mitomiRs, some have been described as specific regulators of mitochondrial metabolism (66). As an example, mir-149 targets Poly(ADP-Ribose) Polymerase-2 (PARP-2) that activates SIRT1 and subsequently, increases mitochondrial function and biogenesis via PGC-1 activation (66). MiRNAs target directly or indirectly a number of key enzymes involved in glycolysis regulation (67). One such example is miR-326 that is overexpressed in metabolically active cancer cells and targets Pyruvate Kinase M2 (PKM2) (68). Some miRNAs are involved in the repair of damages induced by ROS (69). MiR-128a targets Polycomb complex protein (BMI-1) involved in the mitochondrial and redox homeostasis and cellular senescence in a medulloblastoma model (70). In absence of BMI-1, ETC flow is interrupted decreasing ROS generation. Other miRNAs, such as miR-25, target genes that play crucial roles in Ca2+ uptake and consequently, ROS production by targeting Mitochondrial Calcium Uniporter (MCU) (71).
miRNAs and Oxidative Phosphorylation
MiRNAs can target OXPHOS either directly, by targeting mRNAs of essential ETC factors and/or indirectly, by targeting the biosynthesis pathways of essential cofactors (17). MiR-338 and miR-181c have been shown to target complex IV of respiratory chain (COXIV) (21, 72). Overexpression of miR-338 decreased COXIV levels and subsequently, oxidative phosphorylation while miR-181c remodels COXIV by targeting cytochrome c oxidase subunit 1 (COX1) mRNA (72, 73). ATP synthase is a direct target of miR-101 and miR-127 (74). MiR-210 affects the cytoplasmic Iron Sulphur Cluster homologue (ISCU) and blocks ETC (21). SIRT4, which modulates mitochondrial oxidative capacity by targeting glutamate dehydrogenase (GDH), is regulated by miR-15b (75). Inhibition of miR-15b promotes mitochondrial ROS and decreases mitochondrial membrane potential in a SIRT4-dependent manner (76).
miRNAs and TriCarboxylic Acid Cycle
MiRNAs can target central biochemical reactions, shared by many pathways. For example, the citrate synthase from the TCA cycle is targeted by a set of miRNAs: miR-152, miR-148a, miR-148b, miR-299-5p, miR-19b, miR-122a, miR-421, miR-494, and miR-19a (77). As a result, 78 pathways including the purine metabolism, pentose phosphate pathway, fatty acid biosynthesis, as well as carbon, nucleotide and amino acid metabolisms are affected (78). Another enzyme of TCA cycle, Aconitate hydratase (ACO2), which metabolizes α-ketoglutarate, a product of glutamine oxidation is targeted by miR-450 (79). MiR-450 decreases mitochondrial membrane potential and increases glucose uptake (80).
miRNAs and Amino Acid Metabolism
Many miRNAs, including miR-23a/b (81), miR-137 (82), miR-153 (83) and miR192/204 (84), target glutaminase and modulate ROS production. Another enzyme, Serine Hydroxyl-Methyl Transferase (SHMT), which converts serine to glycine, is targeted by several miRNAs, such as miR-193 and miR-642a-5p (85, 86). The Phosphoserine aminotransferase (PSAT1) which catalyzes the reversible conversion of 3-phosphohydroxypyruvate (3-PHP) to phosphoserine (3-PS) is also targeted by miR-340 (87) and miR-29a-3p (Bony et al., submitted).
miRNAs and Fatty Acid Metabolism
Several miRNAs have been described to regulate lipid metabolism. MiR-370, miR-148a, and miR-33 target CPT1A, which is responsible for the translocation of fatty acids from the cytosol to mitochondrial matrix, thereby reducing fatty acid oxidation (88–90). MiR-696 regulates fatty acid oxidation by targeting PGC-1α (91).
The regulation of mitochondrial proteins by miRNAs can therefore modulate mitochondrial function suggesting that targeting miRNAs might provide a new therapeutic approach for the treatment of mitochondria-related diseases.
Role of Extracellular Vesicles-miRNAs on Mitochondrial Metabolism Regulation in Diseases
Role of Mesenchymal Stromal Cell-Derived Extracellular Vesicles in the Regulation of Mitochondrial Metabolism
Various studies have shown that EVs from different cell types can release miRNAs in the cytoplasm of recipient cells where they target mitochondrial metabolism pathways (92). Exposure to EVs significantly increases the mitochondrial respiration, especially through the increase of ROS production and ATP production (93, 94). Notably, MSC-derived EVs (MSC-EVs) play a central role in promoting mitochondrial function. Proteomic and RNAseq analysis demonstrated that MSC-EVs contain several proteins, genes, mRNA, and miRNA involved in glycolysis such as GAPDH, Glucose-6-phosphate isomerase (GPI), in the TCA cycle [2-oxoglutarate dehydrogenase (OGDH)], and ETC (ATPase). In the rat model of pulmonary arterial hypertension (PAH), MSC-EVs upregulated both PDH and Glutamate Dehydrogenase 1 (GLUD1) gene expression in an hypoxic environment and increased glucose oxidation by TCA cycle (92). In a model of ischemia-reperfusion injury, umbilical cord MSC-EVs were shown to contain manganese-dependent superoxide dismutase (MnSOD), which decreased ROS levels and prevented oxidative stress in hepatic tissue (95).
MiRNAs Loaded by Mesenchymal Stromal Cell-Extracellular Vesicles Improve Mitochondrial Dysfunction in Pathological Conditions
Renal Diseases
Acute kidney injury (AKI) results from severe renal ischemia reperfusion injury (IRI), a common clinical situation during transplantation. Renal reperfusion induces oxidative stress, characterized by increased ROS and/or reactive nitrogen species (RNS) (96). MSC-EVs have been shown to alleviate AKI through miRNA transfer to resident renal cells (97).
miR-30
In a rat model of AKI, IRI caused a lower expression of miR-30-b, miR-30-c, and miR-30-d in renal cells. MSC-EVs were shown to protect kidney from IRI by inhibiting mitochondrial fission through miR-30 transfer (98). Transfer of miR-30 mediated the regenerative effect of MSCs by decreasing Dynamin-related protein 1 (DRP1), which induces mitochondrial fragmentation, and subsequently protecting kidney from ischemia (63).
miR-200a-3p
MSC-EVs have been reported to stimulate ATP production and the antioxidant defense of tubular epithelia cells (TECs) through activation of KEAP1-NRF2 signaling pathway (99). In vivo, MSC-EVs were shown to significantly increase the expression of the antioxidant NRF2 and SOD2, both at the protein and mRNA level, while the pro-oxidant KEAP1 was decreased. By investigating the miRNA profile of injured kidney cells, miR-200a-3p was significantly increased after MSC-EV injection. Importantly, EVs isolated from antagomiR-200a-3p-treated MSCs failed to preserve in vitro the structural integrity of mitochondria in TECs under oxidative damage. The authors concluded that miR-200a-3p was shuttled by MSC-EVs to target the KEAP1-NRF2 signaling pathway.
miR-222
In diabetes, hyperglycemia (HG) leads to the down-regulation of COXIV in the ETC complex, leading to mitochondrial disorders and renal tissue alterations. Transfer of miR-222 from MSC-EVs to mesangial cells reduced STAT5A expression, leading to miR-21 down-regulation (100). A previous study showed that miR-21 silencing enhanced mitochondrial function, by reducing ROS production (101). Therefore, MSC-EVs rescued COXIV expression in mesangial cells by miR-222 transfer and miR-21 down-regulation.
Cardiac Diseases
Cardiac stem cells (CSCs) have been reported to be largely involved in cardiac regeneration and repair after injury [for review, see (90)]. CSCs have emerged as a promising therapeutic tool but their poor survival and engraftment prevent their use. However, endogenous CSC function can be improved by miRNAs released by MSCs-EVs after heart damage (102–104).
miR-214
Previous studies indicated that miR-214 was up-regulated by hypoxic stress to protect cardiac myocytes from damage (105). In a hypoxic context, MSC-EVs were reported to decrease the apoptotic rate of CSCs and the production of ROS mediated by CaMKII activation, which is a direct target of miR-214 (106). Transfer of miR-214 by MSC-EVs is likely one of the main effector that protects CSCs from oxidative damage in myocardial infarction.
miR-21
Mir-21 is another miRNA whose cardioprotective role has been demonstrated (107). To mimic the pathophysiology of cardiovascular environment, MSC-EVs were collected from H2O2-treated MSCs and showed to contain higher levels of miR-21 than EVs from non-treated MSCs. Actually, miR-21 up-regulation in H2o2-treated MSC-EVs was shown to regulate the apoptosis of CSCs through PTEN down-regulation and PI3K/AKT activation.
Ovarian Cancer
In the 1920s, Otto Warburg described that cancer cells use higher levels of glucose than normal cells, thereby leading to increased lactate production (108). Since, this phenomenon of aerobic glycolysis, called Warburg’s effect, has been observed in different tumours, including, breast, lung, colorectal cancer and glioblastoma (109) and the role of MSC-EVs in regulating tumour metabolism has been investigated.
miR-1180
MiR-1180 was the most abundant miRNA detected in the conditioned medium of MSCs that were able to stimulate both glycolysis of ovarian cancer cells and chemoresistance to cisplatin treatment (110, 111). Overexpression of miR-1180 in MSCs resulted in the activation of Wnt signaling pathway and expression of its downstream components (Wnt5a, β-catenin, c-Myc…) responsible for glycolysis-induced chemoresistance. Conversely, the supernatant of MSCs treated with miR-1180 inhibitors suppressed cell proliferation and ATP production in cancer cells and restored their chemosensitivity. The effect of MSC-EVs was associated with the down-regulation of Secreted Frizzled-Related Protein 1 (SFRP1), a negative regulator of the Wnt signaling pathway, by miR-1180. Thus, MSC-derived miR-1180 stimulated cancer cell proliferation by stimulating glycolysis-induced chemoresistance.
Liver Diseases
Previous studies have demonstrated that MSCs can reduce inflammatory responses, hepatocyte apoptosis, liver fibrosis and, enhance liver regeneration and functionality [for review, see (101)]. This therapeutic effect of MSCs was attributed to their capacities to secrete trophic factors (111, 112).
miR-122
MiR-122 has a critical role in liver function as being involved in cholesterol biosynthesis, fatty acid synthesis and β-oxidation (113). In normal conditions, miR-122 is one of the most abundant miRNAs, but its expression is reduced in advanced liver diseases. MSC-EVs from patients cannot prevent liver impairment but miR-122-engineered MSCs can release EVs containing high amounts of miR-122. Hepatic cells incubated with miR-122-containing EVs expressed lower levels of miR-122 target genes, including Prolyl 4-hydroxylase subunit alpha-1 (P4HA1) involved in collagen synthesis and insulin-like growth factor 1 receptor (IGF1R) (114). Mir-122 also repressed SIRT6 leading to lower expression levels of acetyl-CoA acyltransferase (HADHB) and carnitine palmitoyltransferase I (CPT1), involved in fatty acid synthesis and β-oxidation (115). MiR-122 is therefore proposed as a key regulator of liver function playing a central role in lipid metabolism and the regulation of metabolic diseases.
Conclusion
In the current study, we described various miRNAs that are expressed by MSCs and packaged in EVs and that directly or indirectly regulate critical functions of mitochondria. MSC-derived miRNAs are promising actors in regenerative medicine and in therapeutics as they can modulate several pathways that are altered in mitochondrial dysfunction-related diseases. Data are still sparse but encouraging results have been reported. Manipulating miRNA expression using miRNA mimics and inhibitors may serve as potential therapeutic approach for diverse diseases. A better understanding of miRNA biogenesis, import and function is needed but should provide new insights on the feasibility of this novel strategy for mitochondria-associated disorders.
Author Contributions
All authors contributed to the article and approved the submitted version.
Funding
We gratefully acknowledge the Agence Nationale pour la Recherche for support of the national infrastructure: “ECELLFRANCE: Development of a national adult mesenchymal stem cell based therapy platform” (ANR-11-INSB-005). The study was also supported by the European Union Horizon 2020 Programme (project RESPINE, grant agreement #: 732163). The materials presented and views expressed here are the responsibility of the authors only. The EU Commission takes no responsibility for any use made of the information set out. Funding for staff exchange was received by Programme Ulysses 2018 (project #: P41059WK).
Conflict of Interest
The authors declare that the research was conducted in the absence of any commercial or financial relationships that could be construed as a potential conflict of interest.
Acknowledgments
We acknowledge support from the Inserm Institute, the University of Montpellier, and University Hospital of Montpellier. We also acknowledge the Société Française de Rhumatologie.
References
1. Duarte FV, Amorim JA, Palmeira CM, Rolo AP. Regulation of Mitochondrial Function and its Impact in Metabolic Stress. Curr Med Chem (2015) 22:2468–79. doi: 10.2174/0929867322666150514095910
2. Zhao L, Hu C, Zhang P, Jiang H, Chen J. Mesenchymal stem cell therapy targeting mitochondrial dysfunction in acute kidney injury. J Transl Med (2019) 17:142. doi: 10.1186/s12967-019-1893-4
3. Friedenstein AJ, Gorskaja JF, Kulagina NN. Fibroblast precursors in normal and irradiated mouse hematopoietic organs. Exp Hematol (1976) 4:267–74.
4. da Silva Meirelles L, Chagastelles PC, Nardi NB. Mesenchymal stem cells reside in virtually all post-natal organs and tissues. J Cell Sci (2006) 119:2204–13. doi: 10.1242/jcs.02932
5. Viswanathan S, Shi Y, Galipeau J, Krampera M, Leblanc K, Martin I, et al. Mesenchymal stem versus stromal cells: International Society for Cell & Gene Therapy (ISCT®) Mesenchymal Stromal Cell committee position statement on nomenclature. Cytotherapy (2019) 21:1019–24. doi: 10.1016/j.jcyt.2019.08.002
6. Moll G, Ankrum JA, Kamhieh-Milz J, Bieback K, Ringdén O, Volk H-D, et al. Intravascular Mesenchymal Stromal/Stem Cell Therapy Product Diversification: Time for New Clinical Guidelines. Trends Mol Med (2019) 25:149–63. doi: 10.1016/j.molmed.2018.12.006
7. Maumus M, Jorgensen C, Noël D. Mesenchymal stem cells in regenerative medicine applied to rheumatic diseases: Role of secretome and exosomes. Biochimie (2013) 95:2229–34. doi: 10.1016/j.biochi.2013.04.017
8. Keyser KA, Beagles KE, Kiem H-P. Comparison of mesenchymal stem cells from different tissues to suppress T-cell activation. Cell Transplant (2007) 16:555–62. doi: 10.3727/000000007783464939
9. Kern S, Eichler H, Stoeve J, Klüter H, Bieback K. Comparative Analysis of Mesenchymal Stem Cells from Bone Marrow, Umbilical Cord Blood, or Adipose Tissue. Stem Cells (2006) 24:1294–301. doi: 10.1634/stemcells.2005-0342
10. Luz-Crawford P, Djouad F, Toupet K, Bony C, Franquesa M, Hoogduijn MJ, et al. Mesenchymal Stem Cell-Derived Interleukin 1 Receptor Antagonist Promotes Macrophage Polarization and Inhibits B Cell Differentiation. Stem Cells (2016) 34:483–92. doi: 10.1002/stem.2254
11. Doorn J, Moll G, Le Blanc K, van Blitterswijk C, de Boer J. Therapeutic applications of mesenchymal stromal cells: paracrine effects and potential improvements. Tissue Eng Part B Rev (2012) 18:101–15. doi: 10.1089/ten.TEB.2011.0488
12. Park K-S, Bandeira E, Shelke GV, Lässer C, Lötvall J. Enhancement of therapeutic potential of mesenchymal stem cell-derived extracellular vesicles. Stem Cell Res Ther (2019) 10:288. doi: 10.1186/s13287-019-1398-3
13. Akers JC, Gonda D, Kim R, Carter BS, Chen CC. Biogenesis of extracellular vesicles (EV): exosomes, microvesicles, retrovirus-like vesicles, and apoptotic bodies. J Neurooncol (2013) 113:1–11. doi: 10.1007/s11060-013-1084-8
14. Abels ER, Breakefield XO. Introduction to Extracellular Vesicles: Biogenesis, RNA Cargo Selection, Content, Release, and Uptake. Cell Mol Neurobiol (2016) 36:301–12. doi: 10.1007/s10571-016-0366-z
15. Colombo M, Raposo G, Théry C. Biogenesis, secretion, and intercellular interactions of exosomes and other extracellular vesicles. Annu Rev Cell Dev Biol (2014) 30:255–89. doi: 10.1146/annurev-cellbio-101512-122326
16. Asgarpour K, Shojaei Z, Amiri F, Ai J, Mahjoubin-Tehran M, Ghasemi F, et al. Exosomal microRNAs derived from mesenchymal stem cells: cell-to-cell messages. Cell Commun Signal (2020) 18:149. doi: 10.1186/s12964-020-00650-6
17. Geiger J, Dalgaard LT. Interplay of mitochondrial metabolism and microRNAs. Cell Mol Life Sci (2017) 74:631–46. doi: 10.1007/s00018-016-2342-7
18. Osellame LD, Blacker TS, Duchen MR. Cellular and molecular mechanisms of mitochondrial function. Best Pract Res Clin Endocrinol Metab (2012) 26:711–23. doi: 10.1016/j.beem.2012.05.003
19. Prasai K. Regulation of mitochondrial structure and function by protein import: A current review. Pathophysiology (2017) 24:107–22. doi: 10.1016/j.pathophys.2017.03.001
20. McBride HM, Neuspiel M, Wasiak S. Mitochondria: More Than Just a Powerhouse. Curr Biol (2006) 16:R551–60. doi: 10.1016/j.cub.2006.06.054
21. Li P, Jiao J, Gao G, Prabhakar BS. Control of mitochondrial activity by miRNAs. J Cell Biochem (2012) 113:1104–10. doi: 10.1002/jcb.24004
22. Orrenius S. Reactive Oxygen Species in Mitochondria-Mediated Cell Death. Drug Metab Rev (2007) 39:443–55. doi: 10.1080/03602530701468516
23. Dröge W. Free radicals in the physiological control of cell function. Physiol Rev (2002) 82:47–95. doi: 10.1152/physrev.00018.2001
24. Qutub AA, Popel AS. Reactive oxygen species regulate hypoxia-inducible factor 1alpha differentially in cancer and ischemia. Mol Cell Biol (2008) 28:5106–19. doi: 10.1128/MCB.00060-08
25. Vidali S, Aminzadeh S, Lambert B, Rutherford T, Sperl W, Kofler B, et al. Mitochondria: The ketogenic diet–A metabolism-based therapy. Int J Biochem Cell Biol (2015) 63:55–9. doi: 10.1016/j.biocel.2015.01.022
26. Munday MR. Regulation of mammalian acetyl-CoA carboxylase. Biochem Soc Trans (2002) 30:1059–64. doi: 10.1042/bst0301059
27. van der Bliek AM, Sedensky MM, Morgan PG. Cell Biology of the Mitochondrion. Genetics (2017) 207:843–71. doi: 10.1534/genetics.117.300262
28. Newell C, Sabouny R, Hittel Dustin S, Shutt TE, Khan A, Klein MS, et al. Mesenchymal Stem Cells Shift Mitochondrial Dynamics and Enhance Oxidative Phosphorylation in Recipient Cells. Front Physiol (2018) 9:1–6. doi: 10.3389/fphys.2018.01572
29. Pieczenik SR, Neustadt J. Mitochondrial dysfunction and molecular pathways of disease. Exp Mol Pathol (2007) 83:84–92. doi: 10.1016/j.yexmp.2006.09.008
30. Tuppen HAL, Blakely EL, Turnbull DM, Taylor RW. Mitochondrial DNA mutations and human disease. Biochim Biophys Acta (2010) 1797:113–28. doi: 10.1016/j.bbabio.2009.09.005
31. Vannuvel K, Renard P, Raes M, Arnould T. Functional and morphological impact of ER stress on mitochondria. J Cell Physiol (2013) 228:1802–18. doi: 10.1002/jcp.24360
32. Dudkina NV, Kouril R, Peters K, Braun H-P, Boekema EJ. Structure and function of mitochondrial supercomplexes. Biochim Biophys Acta (2010) 1797:664–70. doi: 10.1016/j.bbabio.2009.12.013
33. Pellegrino MW, Nargund AM, Haynes CM. Signaling the mitochondrial unfolded protein response. Biochim Biophys Acta (2013) 1833:410–6. doi: 10.1016/j.bbamcr.2012.02.019
34. Stepien KM, Roncaroli F, Turton N, Hendriksz CJ, Roberts M, Heaton RA, et al. Mechanisms of Mitochondrial Dysfunction in Lysosomal Storage Disorders: A Review. J Clin Med (2020) 9:1–22. doi: 10.3390/jcm9082596
35. Craven L, Alston CL, Taylor RW, Turnbull DM. Recent Advances in Mitochondrial Disease. Annu Rev Genomics Hum Genet (2017) 18:257–75. doi: 10.1146/annurev-genom-091416-035426
36. Wallace DC, Fan W, Procaccio V. Mitochondrial energetics and therapeutics. Annu Rev Pathol (2010) 5:297–348. doi: 10.1146/annurev.pathol.4.110807.092314
37. Stavely R, Nurgali K. The emerging antioxidant paradigm of mesenchymal stem cell therapy. Stem Cells Transl Med (2020) 9:985–1006. doi: 10.1002/sctm.19-0446
38. Yuan X, Logan TM, Ma T. Metabolism in Human Mesenchymal Stromal Cells: A Missing Link Between hMSC Biomanufacturing and Therapy? Front Immunol (2019) 10:1–11. doi: 10.3389/fimmu.2019.00977
39. Denu RA, Hematti P. Effects of Oxidative Stress on Mesenchymal Stem Cell Biology. Oxid Med Cell Longev (2016) 2016:2989076. doi: 10.1155/2016/2989076
40. Redondo J, Sarkar P, Kemp K, Heesom KJ, Wilkins A, Scolding NJ, et al. Dysregulation of Mesenchymal Stromal Cell Antioxidant Responses in Progressive Multiple Sclerosis. Stem Cells Transl Med (2018) 7:748–58. doi: 10.1002/sctm.18-0045
41. Sabouny R, Shutt TE. Reciprocal Regulation of Mitochondrial Fission and Fusion. Trends Biochem Sci (2020) 45:564–77. doi: 10.1016/j.tibs.2020.03.009
42. Sebastián D, Palacín M, Zorzano A. Mitochondrial Dynamics: Coupling Mitochondrial Fitness with Healthy Aging. Trends Mol Med (2017) 23:201–15. doi: 10.1016/j.molmed.2017.01.003
43. Wai T, Langer T. Mitochondrial Dynamics and Metabolic Regulation. Trends Endocrinol Metab (2016) 27:105–17. doi: 10.1016/j.tem.2015.12.001
44. Samudio I, Fiegl M, McQueen T, Clise-Dwyer K, Andreeff M. The Warburg effect in leukemia-stroma cocultures is mediated by mitochondrial uncoupling associated with uncoupling protein 2 activation. Cancer Res (2008) 68:5198–205. doi: 10.1158/0008-5472.CAN-08-0555
45. Maremanda KP, Sundar IK, Rahman I. Protective role of mesenchymal stem cells and mesenchymal stem cell-derived exosomes in cigarette smoke-induced mitochondrial dysfunction in mice. Toxicol Appl Pharmacol (2019) 385:114788. doi: 10.1016/j.taap.2019.114788
46. Perico L, Morigi M, Rota C, Breno M, Mele C, Noris M, et al. Human mesenchymal stromal cells transplanted into mice stimulate renal tubular cells and enhance mitochondrial function. Nat Commun (2017) 8:983. doi: 10.1038/s41467-017-00937-2
47. Bell EL, Guarente L. The SirT3 divining rod points to oxidative stress. Mol Cell (2011) 42:561–8. doi: 10.1016/j.molcel.2011.05.008
48. Mohammadalipour A, Dumbali SP, Wenzel PL. Mitochondrial Transfer and Regulators of Mesenchymal Stromal Cell Function and Therapeutic Efficacy. Front Cell Dev Biol (2020) 8:603292. doi: 10.3389/fcell.2020.603292
49. Liang X, Ding Y, Zhang Y, Tse H-F, Lian Q. Paracrine mechanisms of mesenchymal stem cell-based therapy: current status and perspectives. Cell Transplant (2014) 23:1045–59. doi: 10.3727/096368913X667709
50. Spees JL, Lee RH, Gregory CA. Mechanisms of mesenchymal stem/stromal cell function. Stem Cell Res Ther (2016) 7:125. doi: 10.1186/s13287-016-0363-7
51. Acquistapace A, Bru T, Lesault P-F, Figeac F, Coudert AE, le Coz O, et al. Human mesenchymal stem cells reprogram adult cardiomyocytes toward a progenitor-like state through partial cell fusion and mitochondria transfer. Stem Cells (2011) 29:812–24. doi: 10.1002/stem.632
52. Lee Y, Kim M, Han J, Yeom KH, Lee S, Baek SH, et al. MicroRNA genes are transcribed by RNA polymerase II. EMBO J (2004) 23:4051–60. doi: 10.1038/sj.emboj.7600385
53. Bohnsack MT, Czaplinski K, Gorlich D. Exportin 5 is a RanGTP-dependent dsRNA-binding protein that mediates nuclear export of pre-miRNAs. Rna (2004) 10:185–91. doi: 10.1261/rna.5167604
54. Bartel DP. MicroRNAs: genomics, biogenesis, mechanism, and function. Cell (2004) 116:281–97. doi: 10.1016/S0092-8674(04)00045-5
55. O’Brien J, Hayder H, Zayed Y, Peng C. Overview of MicroRNA Biogenesis, Mechanisms of Actions, and Circulation. Front Endocrinol (2018) 9:1–12. doi: 10.3389/fendo.2018.00402
56. Rottiers V, Näär AM. MicroRNAs in metabolism and metabolic disorders. Nat Rev Mol Cell Biol (2012) 13:239–50. doi: 10.1038/nrm3313
57. Wahid F, Shehzad A, Khan T, Kim YY. MicroRNAs: Synthesis, mechanism, function, and recent clinical trials. Biochim Biophys Acta (BBA) Mol Cell Res (2010) 1803:1231–43. doi: 10.1016/j.bbamcr.2010.06.013
58. Munir J, Yoon JK, Ryu S. Therapeutic miRNA-Enriched Extracellular Vesicles: Current Approaches and Future Prospects. Cells (2020) 9:1–18. doi: 10.3390/cells9102271
59. Mills J, Capece M, Cocucci E, Tessari A, Palmieri D. Cancer-Derived Extracellular Vesicle-Associated MicroRNAs in Intercellular Communication: One Cell’s Trash Is Another Cell’s Treasure. Int J Mol Sci (2019) 20:1–27. doi: 10.3390/ijms20246109
60. Hagiwara K, Katsuda T, Gailhouste L, Kosaka N, Ochiya T. Commitment of Annexin A2 in recruitment of microRNAs into extracellular vesicles. FEBS Lett (2015) 589:4071–8. doi: 10.1016/j.febslet.2015.11.036
61. Chen J, Hu C, Pan P. Extracellular Vesicle MicroRNA Transfer in Lung Diseases. Front Physiol (2017) 8:1–9. doi: 10.3389/fphys.2017.01028
62. Janas T, Janas MM, Sapoń K, Janas T. Mechanisms of RNA loading into exosomes. FEBS Lett (2015) 589:1391–8. doi: 10.1016/j.febslet.2015.04.036
63. Qiu G, Zheng G, Ge M, Wang J, Huang R, Shu Q, et al. Mesenchymal stem cell-derived extracellular vesicles affect disease outcomes via transfer of microRNAs. Stem Cell Res Ther (2018) 9:1–9. doi: 10.1186/s13287-018-1069-9
64. Bandiera S, Matégot R, Girard M, Demongeot J, Henrion-Caude A. MitomiRs delineating the intracellular localization of microRNAs at mitochondria. Free Radic Biol Med (2013) 64:12–9. doi: 10.1016/j.freeradbiomed.2013.06.013
65. Song R, Hu X-Q, Zhang L. Mitochondrial MiRNA in Cardiovascular Function and Disease. Cells (2019) 8:1–13. doi: 10.3390/cells8121475
66. Duarte FV, Palmeira CM, Rolo AP. The Role of microRNAs in Mitochondria: Small Players Acting Wide. Genes (Basel) (2014) 5:865–86. doi: 10.3390/genes5040865
67. Singh PK, Mehla K, Hollingsworth MA, Johnson KR. Regulation of Aerobic Glycolysis by microRNAs in Cancer. Mol Cell Pharmacol (2011) 3:125–34.
68. Kefas B, Comeau L, Erdle N, Montgomery E, Amos S, Purow B. Pyruvate kinase M2 is a target of the tumor-suppressive microRNA-326 and regulates the survival of glioma cells. Neuro-oncology (2010) 12:1102–12. doi: 10.1093/neuonc/noq080
69. Torma F, Gombos Z, Jokai M, Berkes I, Takeda M, Mimura T, et al. The roles of microRNA in redox metabolism and exercise-mediated adaptation. J Sport Health Sci (2020) 9:405–14. doi: 10.1016/j.jshs.2020.03.004
70. Babu K R, Tay Y. The Yin-Yang Regulation of Reactive Oxygen Species and MicroRNAs in Cancer. Int J Mol Sci (2019) 20:1–21. doi: 10.3390/ijms20215335
71. Jaquenod De Giusti C, Roman B, Das S. The Influence of MicroRNAs on Mitochondrial Calcium. Front Physiol (2018) 9:1291. doi: 10.3389/fphys.2018.01291
72. Das S, Ferlito M, Kent OA, Fox-Talbot K, Wang R, Liu D, et al. Nuclear miRNA regulates the mitochondrial genome in the heart. Circ Res (2012) 110:1596–603. doi: 10.1161/CIRCRESAHA.112.267732
73. Aschrafi A, Kar AN, Natera-Naranjo O, MacGibeny MA, Gioio AE, Kaplan BB. MicroRNA-338 regulates the axonal expression of multiple nuclear-encoded mitochondrial mRNAs encoding subunits of the oxidative phosphorylation machinery. Cell Mol Life Sci (2012) 69:4017–27. doi: 10.1007/s00018-012-1064-8
74. Baradan R, Hollander JM, Das S. Mitochondrial miRNAs in diabetes: just the tip of the iceberg. Can J Physiol Pharmacol (2017) 95:1156–62. doi: 10.1139/cjpp-2016-0580
75. Lang A, Grether-Beck S, Singh M, Kuck F, Jakob S, Kefalas A, et al. MicroRNA-15b regulates mitochondrial ROS production and the senescence-associated secretory phenotype through sirtuin 4/SIRT4. Aging (Albany NY) (2016) 8:484–505. doi: 10.18632/aging.100905
76. Haigis MC, Mostoslavsky R, Haigis KM, Fahie K, Christodoulou DC, Murphy AJ, et al. SIRT4 inhibits glutamate dehydrogenase and opposes the effects of calorie restriction in pancreatic beta cells. Cell (2006) 126:941–54. doi: 10.1016/j.cell.2006.06.057
77. Tomasetti M, Amati M, Santarelli L, Neuzil J. MicroRNA in Metabolic Re-Programming and Their Role in Tumorigenesis. Int J Mol Sci (2016) 17:1–19. doi: 10.3390/ijms17050754
78. Tibiche C, Wang E. MicroRNA Regulatory Patterns on the Human Metabolic Network. Open Syst Biol J (2008) 1:1–8. doi: 10.2174/1876392800801010001
79. miR-450a Acts as a Tumor Suppressor in Ovarian Cancer by Regulating Energy Metabolism | Cancer Research. Available at: https://cancerres-aacrjournals-org.proxy.insermbiblio.inist.fr/content/79/13/3294.long (Accessed April 30, 2020).
80. Muys BR, Sousa JF, Plaça JR, de Araújo LF, Sarshad AA, Anastasakis DG, et al. miR-450a Acts as a Tumor Suppressor in Ovarian Cancer by Regulating Energy Metabolism. Cancer Res (2019) 79:3294–305. doi: 10.1158/0008-5472.CAN-19-0490
81. Gao P, Tchernyshyov I, Chang T-C, Lee Y-S, Kita K, Ochi T, et al. c-Myc suppression of miR-23a/b enhances mitochondrial glutaminase expression and glutamine metabolism. Nature (2009) 458:762–5. doi: 10.1038/nature07823
82. Luan W, Zhou Z, Zhu Y, Xia Y, Wang J, Xu B. miR-137 inhibits glutamine catabolism and growth of malignant melanoma by targeting glutaminase. Biochem Biophys Res Commun (2018) 495:46–52. doi: 10.1016/j.bbrc.2017.10.152
83. Liu Z, Wang J, Li Y, Fan J, Chen L, Xu R. MicroRNA-153 regulates glutamine metabolism in glioblastoma through targeting glutaminase. Tumour Biol (2017) 39:1010428317691429. doi: 10.1177/1010428317691429
84. Ge Y, Yan X, Jin Y, Yang X, Yu X, Zhou L, et al. MiRNA-192 [corrected] and miRNA-204 Directly Suppress lncRNA HOTTIP and Interrupt GLS1-Mediated Glutaminolysis in Hepatocellular Carcinoma. PloS Genet (2015) 11:e1005726. doi: 10.1371/journal.pgen.1005726
85. Leivonen S-K, Rokka A, Östling P, Kohonen P, Corthals GL, Kallioniemi O, et al. Identification of miR-193b Targets in Breast Cancer Cells and Systems Biological Analysis of Their Functional Impact. Mol Cell Proteomics (2011) 10:1–9. doi: 10.1074/mcp.M110.005322
86. Lin C, Zhang Y, Chen Y, Bai Y, Zhang Y. Long noncoding RNA LINC01234 promotes serine hydroxymethyltransferase 2 expression and proliferation by competitively binding miR-642a-5p in colon cancer. Cell Death Dis (2019) 10:1–16. doi: 10.1038/s41419-019-1352-4
87. Yan S, Jiang H, Fang S, Yin F, Wang Z, Jia Y, et al. MicroRNA-340 Inhibits Esophageal Cancer Cell Growth and Invasion by Targeting Phosphoserine Aminotransferase 1. Cell Physiol Biochem (2015) 37:375–86. doi: 10.1159/000430361
88. Fernández-Hernando C, Suárez Y, Rayner KJ, Moore KJ. MicroRNAs in lipid metabolism. Curr Opin Lipidol (2011) 22:86–92. doi: 10.1097/MOL.0b013e3283428d9d
89. Karunakaran D, Thrush AB, Nguyen M-A, Richards L, Geoffrion M, Singaravelu R, et al. Macrophage Mitochondrial Energy Status Regulates Cholesterol Efflux and Is Enhanced by Anti-miR33 in Atherosclerosis. Circ Res (2015) 117:266–78. doi: 10.1161/CIRCRESAHA.117.305624
90. Wagschal A, Najafi-Shoushtari SH, Wang L, Goedeke L, Sinha S, deLemos AS, et al. Genome-wide identification of microRNAs regulating cholesterol and triglyceride homeostasis. Nat Med (2015) 21:1290–7. doi: 10.1038/nm.3980
91. Aoi W, Naito Y, Mizushima K, Takanami Y, Kawai Y, Ichikawa H, et al. The microRNA miR-696 regulates PGC-1{alpha} in mouse skeletal muscle in response to physical activity. Am J Physiol Endocrinol Metab (2010) 298:E799–806. doi: 10.1152/ajpendo.00448.2009
92. Hogan SE, Rodriguez Salazar MP, Cheadle J, Glenn R, Medrano C, Petersen TH, et al. Mesenchymal stromal cell-derived exosomes improve mitochondrial health in pulmonary arterial hypertension. Am J Physiol Lung Cell Mol Physiol (2019) 316:L723–37. doi: 10.1152/ajplung.00058.2018
93. Russell AE, Jun S, Sarkar S, Geldenhuys WJ, Lewis SE, Rellick SL, et al. Extracellular Vesicles Secreted in Response to Cytokine Exposure Increase Mitochondrial Oxygen Consumption in Recipient Cells. Front Cell Neurosci (2019) 13:1–12. doi: 10.3389/fncel.2019.00051
94. Bland CL, Byrne-Hoffman CN, Fernandez A, Rellick SL, Deng W, Klinke DJ. Exosomes derived from B16F0 melanoma cells alter the transcriptome of cytotoxic T cells that impacts mitochondrial respiration. FEBS J (2018) 285:1033–50. doi: 10.1111/febs.14396
95. Yao J, Zheng J, Cai J, Zeng K, Zhou C, Zhang J, et al. Extracellular vesicles derived from human umbilical cord mesenchymal stem cells alleviate rat hepatic ischemia-reperfusion injury by suppressing oxidative stress and neutrophil inflammatory response. FASEB J (2019) 33:1695–710. doi: 10.1096/fj.201800131RR
96. Li H, Xia Z, Chen Y, Qi D, Zheng H. Mechanism and Therapies of Oxidative Stress-Mediated Cell Death in Ischemia Reperfusion Injury. Oxid Med Cell Longev (2018) 2018:1–2. doi: 10.1155/2018/2910643
97. Wang S-Y, Hong Q, Zhang C-Y, Yang Y-J, Cai G-Y, Chen X-M. miRNAs in stem cell-derived extracellular vesicles for acute kidney injury treatment: comprehensive review of preclinical studies. Stem Cell Res Ther (2019) 10:281. doi: 10.1186/s13287-019-1371-1
98. Gu D, Zou X, Ju G, Zhang G, Bao E, Zhu Y. Mesenchymal Stromal Cells Derived Extracellular Vesicles Ameliorate Acute Renal Ischemia Reperfusion Injury by Inhibition of Mitochondrial Fission through miR-30. Stem Cells Int (2016) 2016:2093940. doi: 10.1155/2016/2093940
99. Cao H, Cheng Y, Gao H, Zhuang J, Zhang W, Bian Q, et al. In Vivo Tracking of Mesenchymal Stem Cell-Derived Extracellular Vesicles Improving Mitochondrial Function in Renal Ischemia-Reperfusion Injury. ACS Nano (2020) 14:4014–26. doi: 10.1021/acsnano.9b08207
100. Gallo S, Gili M, Lombardo G, Rossetti A, Rosso A, Dentelli P, et al. Stem Cell-Derived, microRNA-Carrying Extracellular Vesicles: A Novel Approach to Interfering with Mesangial Cell Collagen Production in a Hyperglycaemic Setting. PloS One (2016) 11:1–18. doi: 10.1371/journal.pone.0162417
101. Gomez IG, MacKenna DA, Johnson BG, Kaimal V, Roach AM, Ren S, et al. Anti-microRNA-21 oligonucleotides prevent Alport nephropathy progression by stimulating metabolic pathways. J Clin Invest (2015) 125:141–56. doi: 10.1172/JCI75852
102. Wang K, Jiang Z, Webster KA, Chen J, Hu H, Zhou Y, et al. Enhanced Cardioprotection by Human Endometrium Mesenchymal Stem Cells Driven by Exosomal MicroRNA-21. Stem Cells Trans Med (2017) 6:209–22. doi: 10.5966/sctm.2015-0386
103. Zhu J, Lu K, Zhang N, Zhao Y, Ma Q, Shen J, et al. Myocardial reparative functions of exosomes from mesenchymal stem cells are enhanced by hypoxia treatment of the cells via transferring microRNA-210 in an nSMase2-dependent way. Artif Cells Nanomed Biotechnol (2018) 46:1659–70. doi: 10.1080/21691401.2017.1388249
104. Hashimoto H, Olson EN, Bassel-Duby R. Therapeutic approaches for cardiac regeneration and repair. Nat Rev Cardiol (2018) 15:585–600. doi: 10.1038/s41569-018-0036-6
105. Lv G, Shao S, Dong H, Bian X, Yang X, Dong S. MicroRNA-214 protects cardiac myocytes against H2O2-induced injury. J Cell Biochem (2014) 115:93–101. doi: 10.1002/jcb.24636
106. Wang Y, Zhao R, Liu D, Deng W, Xu G, Liu W, et al. Exosomes Derived from miR-214-Enriched Bone Marrow-Derived Mesenchymal Stem Cells Regulate Oxidative Damage in Cardiac Stem Cells by Targeting CaMKII. Oxid Med Cell Longev (2018) 2018:4971261. doi: 10.1155/2018/4971261
107. Shi B, Wang Y, Zhao R, Long X, Deng W, Wang Z. Bone marrow mesenchymal stem cell-derived exosomal miR-21 protects C-kit+ cardiac stem cells from oxidative injury through the PTEN/PI3K/Akt axis. PloS One (2018) 13:e0191616. doi: 10.1371/journal.pone.0191616
108. Warburg O, Wind F, Negelein E. THE METABOLISM OF TUMORS IN THE BODY. J Gen Physiol (1927) 8:519–30. doi: 10.1085/jgp.8.6.519
109. Potter M, Newport E, Morten KJ. The Warburg effect: 80 years on. Biochem Soc Trans (2016) 44:1499–505. doi: 10.1042/BST20160094
110. Gu Z-W, He Y-F, Wang W-J, Tian Q, Di W. MiR-1180 from bone marrow-derived mesenchymal stem cells induces glycolysis and chemoresistance in ovarian cancer cells by upregulating the Wnt signaling pathway. J Zhejiang Univ Sci B (2019) 20:219–37. doi: 10.1631/jzus.B1800190
111. Eom YW, Shim KY, Baik SK. Mesenchymal stem cell therapy for liver fibrosis. Korean J Intern Med (2015) 30:580–9. doi: 10.3904/kjim.2015.30.5.580
112. Alfaifi M, Eom YW, Newsome PN, Baik SK. Mesenchymal stromal cell therapy for liver diseases. J Hepatol (2018) 68:1272–85. doi: 10.1016/j.jhep.2018.01.030
113. Esau C, Davis S, Murray SF, Yu XX, Pandey SK, Pear M, et al. miR-122 regulation of lipid metabolism revealed by in vivo antisense targeting. Cell Metab (2006) 3:87–98. doi: 10.1016/j.cmet.2006.01.005
114. Lou G, Yang Y, Liu F, Ye B, Chen Z, Zheng M, et al. MiR-122 modification enhances the therapeutic efficacy of adipose tissue-derived mesenchymal stem cells against liver fibrosis. J Cell Mol Med (2017) 21:2963–73. doi: 10.1111/jcmm.13208
Keywords: mesenchymal stem cell, miRNA, mitochondrial metabolism, extracellular vesicle, mitochondrial dysfunction
Citation: Loussouarn C, Pers Y-M, Bony C, Jorgensen C and Noël D (2021) Mesenchymal Stromal Cell-Derived Extracellular Vesicles Regulate the Mitochondrial Metabolism via Transfer of miRNAs. Front. Immunol. 12:623973. doi: 10.3389/fimmu.2021.623973
Received: 30 October 2020; Accepted: 14 January 2021;
Published: 16 March 2021.
Edited by:
Irun R. Cohen, Weizmann Institute of Science, IsraelReviewed by:
Jeffrey Louis Curtis, University of Michigan, United StatesGuido Moll, Charité – Universitätsmedizin Berlin, Germany
Copyright © 2021 Loussouarn, Pers, Bony, Jorgensen and Noël. This is an open-access article distributed under the terms of the Creative Commons Attribution License (CC BY). The use, distribution or reproduction in other forums is permitted, provided the original author(s) and the copyright owner(s) are credited and that the original publication in this journal is cited, in accordance with accepted academic practice. No use, distribution or reproduction is permitted which does not comply with these terms.
*Correspondence: Danièle Noël, ZGFuaWVsZS5ub2VsQGluc2VybS5mcg==