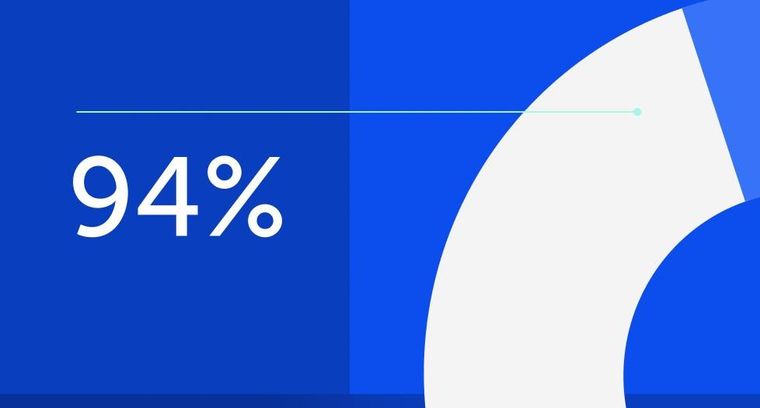
94% of researchers rate our articles as excellent or good
Learn more about the work of our research integrity team to safeguard the quality of each article we publish.
Find out more
REVIEW article
Front. Immunol., 22 February 2021
Sec. Inflammation
Volume 12 - 2021 | https://doi.org/10.3389/fimmu.2021.620333
This article is part of the Research TopicRecent Advances in Basic and Translational OsteoimmunologyView all 18 articles
Osteoimmunology highlights the two-way communication between bone and immune cells. T cell protein tyrosine phosphatase (TCPTP), also known as protein-tyrosine phosphatase non-receptor 2 (PTPN2), is an intracellular protein tyrosine phosphatase (PTP) essential in regulating immune responses and bone metabolism via dephosphorylating target proteins. Tcptp knockout in systemic or specific immune cells can seriously damage the immune function, resulting in bone metabolism disorders. This review provided fresh insights into the potential role of TCPTP in osteoimmunology. Overall, the regulation of osteoimmunology by TCPTP is extremely complicated. TCPTP negatively regulates macrophages activation and inflammatory factors secretion to inhibit bone resorption. TCPTP regulates T lymphocytes differentiation and T lymphocytes-related cytokines signaling to maintain bone homeostasis. TCPTP is also expected to regulate bone metabolism by targeting B lymphocytes under certain time and conditions. This review offers a comprehensive update on the roles of TCPTP in osteoimmunology, which can be a promising target for the prevention and treatment of inflammatory bone loss.
Inflammatory bone diseases characterized by severe bone loss, such as osteoarthritis, rheumatoid arthritis, and periodontitis, are a manifestation of imbalance between the skeletal and immune systems (1–3). Their interactions, known as osteoimmunology, were firstly proposed by Choi and Aaron in 2000, which highlights the two-way communication between bone and immune cells (4). With a comprehensive and profound acknowledgment of osteoimmunology, targeting regulatory proteins involved in osteoimmune responses can be a feasible means against inflammatory bone diseases. T-cell protein tyrosine phosphatase (TCPTP), one of the protein tyrosine phosphatases (PTPs) family, was identified by Cool et al. using T-cell-based cDNA library screening (5). There is growing evidence that TCPTP is a critical regulator in immune responses and bone metabolism. Nevertheless, the potential effect of TCPTP in the field of osteoimmunology is less explored. As a result, we intended to offer a comprehensive update on the known and potential roles of TCPTP in osteoimmunology in this review. Our study will lay a theoretical foundation for further basic researches of TCPTP in the field of osteoimmunology and provide references for treatment strategies of inflammatory bone diseases.
During osteoimmune responses, different immune cells and bone cells interact reciprocally to maintain the homeostasis between the immune and skeletal systems (Figure 1). The two-way communication may influence either immune or bone cells via cytokine activities in the immune-bone interface. The proposal of screening new targets interfering with osteoimmunology may be feasible for identifying critical targets suppressing immune hyperactivity in inflammatory bone loss diseases.
Figure 1 Diagram of osteoimmunology and potential regulatory sites of TCPTP. Regarding the regulation of bone-related cells by immune cells, some CD4+ T cell subsets can produce osteoclastogenic cytokines (e.g., TNF-α from Th1 cells and IL−17 from Th17 cells), while other subsets secrete anti-osteoclastogenic cytokines (e.g., IL−4 from Th2 cells, IL−10 and CTLA4 from Treg cells). IFN-γ released by Th1 cells may exert both pro- and anti-osteoclastogenic effects as reported by previous studies. B cells physiologically inhibit osteoclastogenesis but stimulate osteoclastogenesis through activating the RANK/RANKL axis in the pathological state. Different stages of dendritic cells exhibit distinct properties in immune responses: immature dendritic cells differentiate into osteoclasts in response to M-CSF, RANKL, TNF-α, IL-1, and IL-17, while mature ones drive the activation and expansion of Th17 cells. Macrophages are essential to bone loss with the involvement of TNF-α, IL-1, and IL-6 released by themselves. On the other hand, bone-related cells also provide feedback to immune cells. Osteoblasts secrete G-CSF, IL−1, IL−6, IL−7, and CXCL12, which are required for HSCs maintenance. In addition, osteoblasts secrete IL-7 to support B lymphopoiesis and regulate DLL4-Notch signaling pathway to support T lymphopoiesis. Osteocytes are supposed to mobilize HSCs and are involved in myelopoiesis. Osteocyte-derived RANKL participates in estrogen deficiency-induced bone loss by indirect regulation of B cell development. Osteoclasts are also involved in antigen presentation and T cell activation. Osteoclasts secrete tolerogenic cytokines (e.g., IL-10, TGF-β) and activate regulatory T cells in physiological conditions, while in pathological conditions, osteoclasts secrete inflammatory cytokines (e.g., TNFα, IL-1β) and activate TNF-α producing CD4+ T cells. Generally, TCPTP regulates bone metabolism mainly by changing the biofunction of macrophages, T cells, and B cells. TCPTP, T cell protein tyrosine phosphatase; DC cell, dendritic cell; IL-6, interleukin-6; IL-23, interleukin-23; IL-17, interleukin-17; TNF-α, tumor necrosis factor-α; RANK, receptor activator of nuclear factor−κB; RANKL, receptor activator of nuclear factor−κB ligand; IL-1, interleukin-1; IL-4, interleukin-4; IL-10, interleukin-10; CTLA4, cytotoxic T lymphocyte protein 4; OPG, osteoprotegerin; Th17, T helper 17 cells; Th1, T helper 1 cells; Th2, T helper 2 cells; Treg, regulatory T cells; G-CSF, granulocyte colony-stimulating factor; M-CSF, macrophage colony-stimulating factor; TGF-β, transforming growth factor β; DLL4, Delta-like protein 4; IL-1β, interleukin-1β; IL-7, interleukin-7; CXCL12, CXC-motif chemokine 12; HSCs, hematopoietic stem cells.
Immune cells, such as T lymphocytes (Th1, Th2, Treg, and Th17 cells), B lymphocytes, dendritic cells, and macrophages, actively regulate the homeostasis of bone metabolism. Th1 cells secrete interferon-γ (IFN-γ) that has been found to exert controversial effects in bone metabolism (6, 7). Sato et al. demonstrated that Th1 cells generated amounts of IFN-γ and mediated osteoclastogenesis inhibition in vitro (8). However, another study reported that IFN-γ could promote osteoclast maturation in the late period of osteoclastogenesis (9). Th1 cells are found to induce orthodontic tooth movement and bone resorption indirectly by upregulating the tumor necrosis factor-alpha (TNF-α) secretion and promoting osteoclastogenesis (10, 11). Th2 cells secrete interleukin-4 (IL-4), interleukin-5 (IL-5), and interleukin-13 (IL-13) leading to the osteoclastogenesis inhibition in a signal transducer and activator of transcription 6 (STAT6)-dependent pathway (12). Cytotoxic T-lymphocyte antigen 4 (CTLA4) secreted by Treg cells can promote apoptosis of osteoclasts via binding to CD80/CD86 on osteoclast precursors (13). Besides, Treg cells not only inhibit osteoclastogenesis directly via suppressing receptor activator of nuclear factor-κB ligand (RANKL) generation (14) but also suppress osteoclast differentiation and bone resorption by secreting interleukin-10 (IL-10) and transforming growth factor-β (TGF-β) (15). Th17 cells are one of the osteoclastogenic subsets of T cells that participate in various inflammatory diseases, such as rheumatoid arthritis, osteoporosis, inflammatory bowel disease, and periodontal disease (16–18). In the process of osteoclastogenesis and bone loss, higher amounts of osteoclastogenic cytokines, including interleukin-17 (IL-17), interleukin-6 (IL-6), interleukin-1 (IL-1), and TNF-α, are released from Th17 cells (19, 20). Among these cytokines, IL-17 stimulates the synthesis of cyclooxygenase-2 dependent prostaglandin E2 and the gene transcription of osteoclast differentiation factor (ODF) in osteoblasts to induce osteoclastogenesis (21). B cells inhibit osteoclastogenesis via secreting osteoprotegerin in the physiological state but stimulate osteoclastogenesis through activating the receptor activator of nuclear factor−κB (RANK)/RANKL axis in the pathological state (22, 23). Human immature dendritic cells differentiate into osteoclasts in response to macrophage colony-stimulating factor (M-CSF), RANKL, TNF-α, IL-1, and IL-17 (24–26), while mature dendritic cells can drive the activation of Th17 cells that produce IL-17, thereby enhancing osteoclastogenesis (27). Macrophages are reported to secrete different proinflammatory cytokines (e.g., TNF-α, IL-1, and IL-6) to enhance bone loss (28).
Bone-related cells (e.g., osteoblasts, osteocytes, osteoclasts) could also regulate the immune system. In this process, numerous cytokines (e.g., granulocyte colony-stimulating factor [G−CSF], IL−1, IL−6, IL−7, and CXC-motif chemokine 12 [CXCL12]) are secreted from osteoblasts for hematopoietic stem cell (HSC) maintenance, lymphoid progenitor cell maintenance, as well as the balance of T cell or B cell generation (29–31). Zhu et al. reported that osteoblasts support all stages of B lymphopoiesis via locally secreting interleukin-7 (IL-7) and stromal cell derived factor-1 (SDF-1) (32, 33). Osteoblasts-specific knockout of osteocalcin results in a marked reduction in mature T cells through disrupting the delta-like protein 4-notch signaling (34). Osteocytes are supposed to mobilize hematopoietic stem cells (HSCs) and might also be involved in myelopoiesis. In mice with targeted ablation of osteocytes, the mobilization of HSCs was suppressed in bone marrow (35). Besides, deficiency of the G−protein subunit GSα in osteocytes results in increased G−CSF production and dramatic expansion of myeloid lineage cells (36). RANKL generated by osteocytes participates in estrogen deficiency-induced bone loss by regulating B cell development indirectly (37). Several studies reported that osteoclasts could promote the mobilization of hematopoietic progenitor cells (38), while others revealed that they were dispensable for HSC maintenance and mobilization (39). Osteoclasts are also involved in antigen presentation and T cell activation. In the physiological conditions, osteoclasts secrete tolerogenic cytokines (such as IL-10 and TGF-β) and activate CD4+ and CD8+ regulatory T cells, while in the pathological conditions, osteoclasts activate TNF-α producing CD4+ T cells via unleashing myriads of inflammatory cytokines (e.g., TNF-α and IL-1) (26, 40, 41).
TCPTP is a tyrosine-specific phosphatase that is firstly identified by Cool et al. (5). There are two splice variants of TCPTP: TC45 (45 kDa) which is located in nuclear and TC48 (48 kDa) which is located in the endoplasmic reticulum (42). TC45 is a widely expressed form in various species, including humans and mice, and TC48 is human-specific. In most species, TC45 shuttles between the nucleus and cytoplasm in response to cytokine stimulation (43).
TCPTP regulates diverse signaling pathways related to glucose metabolism, inflammation control, cancer progress, and other biological processes via dephosphorylation of distinct substrates (44–50). Experiments in vitro and in vivo have confirmed that TCPTP could regulate several cytokine signaling pathways by inhibiting Janus activated kinase (JAK)/signal transducer and activator of transcription (STAT) predominantly (51–54). The direct substrates have been recognized as JAK1, JAK3, STAT1, STAT3, and STAT5 (55–59). Some members of the tyrosine kinase receptor (RTK) family, comprising insulin receptors (IRs) (60, 61), epidermal growth factor receptors (EGFRs) (62, 63), vascular endothelial growth factor receptors (VEGFRs) (64), platelet-derived growth factor receptors (PDGFRs) (65, 66) and colony-stimulating factor-1 receptors (CSF-1Rs) are also the specific dephosphorylating substrates of TCPTP (67).
Growing evidence has indicated that TCPTP is a key player in regulating innate and acquired immune responses. GWA studies found single nucleotide polymorphisms (SNPs) of TCPTP are associated with the onset of several inflammatory diseases and autoimmunology disorders, such as inflammatory bowel disease (68, 69), ocular Behcet’s disease (70), rheumatoid arthritis (53), and juvenile inflammatory arthritis (71). Tcptp knockout in the systemic or specific cells can seriously jeopardize immune reactions.
Mice null for Tcptp (Tcptp−/−) showed a smaller body size, decreased mobility, severe anemia, and diarrhea followed by death at three to 5 weeks of age (72). From the perspective of histology, Tcptp−/− mice showed mononuclear cell infiltration in the salivary gland and gastric mucosa at 1 week of age (72). Dramatic increases in TNF-α and inducible nitric oxide synthase (iNOS) were also detected at 3 weeks of age in Tcptp−/− mice (73).
Loss of PTPN2 in T cells (TCPTP-CD4Cre) not only led to increased intestinal inflammation risk but also resulted in T-lymphocyte infiltration in the liver, kidney, and skin (74, 75). This may be caused by the enhanced induction of Th1 cells, Th17 cells, and effector and memory CD8+ T cells, but the impaired induction of Tregs after T cell-specific knockout of Tcptp. Myeloid cell-specific loss of TCPTP (TCPTP-LysMCre) also enhanced susceptibility to colitis and serum IL-1β levels in mice (76). Spalinger et al. reported that TCPTP loss in macrophages compromises epithelial cell-macrophage interactions and reduces epithelial barrier integrity (77). Furthermore, TCPTP knockdown in THP-1 cells elevated the IFN-γ-induced secretion of the proinflammatory cytokines IL-6 (78). TCPTP silencing in rheumatoid arthritis synovial fibroblasts could also increase IL-6 production (53).
Although ubiquitously expressed, TCPTP is pronouncedly expressed in hematopoietic tissues and plays a significant role in the development of hematopoietic lineages (5).
Hematopoietic stem cells (HSCs) are one of the adult stem cells and can differentiate into various mature blood cells (79). Compared with the control group, Tcptp knockout resulted in a nine-fold increase of the HSC number in the bone marrow (80). Lymphoid and myeloid precursors were also more abundant in Tcptp−/− mice compared with the wide type controls (45). Bourdeau et al. also demonstrated that this effect could be reproduced by TCPTP inhibiting agents and interleukin-18 (IL-18) signaling pathway involved in this process (80). The above results implicated that TCPTP plays an important role in the regulation of HSC proliferation.
Tcptp−/− mice exhibited increased splenic macrophage populations and yielded four times of the macrophage colony-forming unit (CFU-M) number (67). TCPTP is also involved in myeloid progenitor development. Tcptp−/− mice had 4 times of the granulocyte/macrophage precursors (GMPs) compared with wildtype mice and CSF-1/CSF-1R signaling may involve in this process (67).
Tcptp−/− mice were reported to suffer from severe anemia which could contribute to their early lethality. You-Ten et al. reported a failure to initiate hematopoietic function in the bone marrow of Tcptp−/− animals after 2–3 weeks (72). What’s more, the deficiency of bone marrow stromal cell numbers, the impairment of remaining stromal cells, and the inadequate cytokines production by the bone marrow microenvironment could be the possible explanations for the defective hematopoiesis in Tcptp−/− mice (72).
Tcptp−/− mice exhibited specific defects of B cell lymphopoiesis in the bone marrow, however, T cell development in the thymus was not significantly affected (72). The defects of B cell lymphopoiesis were characterized by fewer pre-B cell colonies and impaired transition to the immature B-cell stage (81). Bourdeau et al. have found that bone marrow stromal cells from Tcptp−/− mice could secrete higher levels of IFN-γ resulting in a 2-fold reduction in the mitotic index on IL-7 stimulation of Tcptp−/− pre-B cells (81).
Recent studies using gene knockout mice have emphasized the importance of TCPTP in bone metabolism. Tcptp−/− BALB/c mice showed significantly reduced femoral length and width, as reflected by the large volume of unabsorbed cartilage at the epiphysis by 14 days of age (82); however the latter was no longer evident at 21 days of age (82). Besides, Tcptp−/− mice have a higher incidence of synovitis in the knee joint (83). Loh et al. found that the runted body of neuronal cell-specific Tcptp knockout mice is associated with decreased circulating growth hormone (84). However, the precise mechanisms that underlie the bone development in Tcptp−/− mice still need further investigation.
Insulin signaling in osteoblast inhibits the expression of osteoprotegerin (OPG), an osteoclastogenesis inhibitory factor (85). TCPTP expressed in osteoblasts regulates insulin receptor phosphorylation, thus activating insulin signaling (86). A classical coculture assay revealed that osteoblasts lacking TCPTP induced more tartrate-resistant acid phosphatase (TRAP) positive osteoclasts than wild-type osteoblasts did (86). Accordingly, osteoclast activity was increased in osteoblasts-specific Tcptp−/− mice which was proved by increased serum levels of CTx, a marker of bone resorption (86).
As shown in Figure 1, TCPTP could influence bone metabolism by regulating the biofunction of macrophages, T cells, and B cells. Besides, TCPTP in osteoblasts and bone marrow stem cells also regulates bone metabolism. The potential roles of TCPTP in osteoimmunology are discussed below.
Macrophages, a significant component of the non-specific immunity, are crucial regulators in bone metabolism and can be regulated by TCPTP (77, 87, 88). The potential influences of TCPTP on osteoimmunology through macrophages can be expounded from four aspects. Firstly, TCPTP negatively regulates macrophages development (45). Tcptp−/− animals exhibited significantly impaired bone marrow microenvironment (including impairment of erythropoiesis, the decline of pre-B and mature B cells, and reduced stromal cells) and increased macrophage numbers (89). Secondly, TCPTP participates in the process of macrophage polarization. M1 and M2 macrophages, the two major phenotypes of macrophages, are pro-inflammatory and anti-inflammatory respectively. A previous study reported that the increased M1/M2 ratio finally promoted osteoclastogenesis (90), and TCPTP could reverse diabetes-mediated high M1/M2 polarization in mice (91). This can be attributed to the fact that macrophages from Tcptp−/− mice are inherently hypersensitive to lipopolysaccharide and IFN-γ stimulation, which are two main cytokines that induce M1 differentiation (73, 92). Thirdly, the colony-stimulating factor 1 (CSF-1)/colony-stimulating factor 1 receptor (CSF-1R) signaling has been proven to be downregulated by TCPTP in macrophages. After CSF-1 stimulation, tyrosine phosphorylation of the CSF-1R markedly increased, and the activation of extracellular regulated protein kinases (ERK) was enhanced in Tcptp−/− macrophages (67). Zhang et al. ascertained that TCPTP inhibited alveolar bone resorption in diabetic periodontitis via dephosphorylating CSF-1R at the Y807 site, thereby prohibiting osteoclast differentiation (93). Fourthly, the dephosphorylating of c-Jun N-terminal kinase (JNK) in macrophages is another means how TCPTP protects against inflammatory response and bone loss caused by inflammasome-mediated interleukin-1β secretion (76). These studies suggest that TCPTP may be a potential treatment target against inflammatory bone loss induced by macrophage-related disorders, for example, periodontitis and synovitis (Figure 2A).
Figure 2 TCPTP-related signaling pathways in macrophages, B cells, and T cells. (A) TCPTP downregulates the IFN-γ signaling, the CSF-1/CSF-1R signaling, and inflammasome activation in macrophages. (B) TCPTP drives B cell maturation via suppressing the IFN-γ signaling and prohibits B cell proliferation by downregulating the IL-21 signaling. (C) TCPTP inhibits IL-6-driven pathogenic loss of Foxp3 after Tregs have acquired RORγt expression through dephosphorylation of STAT3. TCPTP negatively regulates the IL-2 receptor signaling by JAK1, JAK3, and STAT5 dephosphorylation, which is an important way related to Treg differentiation. TCPTP inhibits TCR activation and hyperphosphorylation of Lck to inhibit inflammatory reactions and cell differentiation. TCPTP, T cell protein tyrosine phosphatase; JAK1, Janus activated kinase 1; JAK2, Janus activated kinase 2; JAK3, Janus activated kinase 3; STAT1, signal transducer and activator of transcription1; STAT3, signal transducer and activator of transcription3; STAT5, signal transducer and activator of transcription5; IFN-γ, interferon-γ; IFNGR, interferon-γ receptor; CSF-1, colony-stimulating factor 1; CSF-1R, colony-stimulating factor 1 receptor; DAMPs, damage-associated molecular pattern molecules; TLR, toll-like receptor; JNK, c-Jun N-terminal kinase; IL-21, interleukin-21; IL-21R, interleukin-21R; IL-6, interleukin-6; IL-6R, interleukin-6R; TCR, T cell receptor.
Undoubtedly, T cells play a critical role in bone homeostasis, wherein the effects of TCPTP on T cell function cannot be neglected (Figure 2C). TCPTP negatively regulates T cell activation (94, 95). Tcptp−/− T cells show enhanced cell activity via the reduction of T cell receptor (TCR) threshold and hyperphosphorylation of the activated tyrosine residue of Lck (75). Furthermore, TCPTP regulates T cell differentiation (91, 96, 97). TCPTP regulated the activation and differentiation of T cells in colonic inflammation (96). Wiede et al. showed that TCPTP attenuates the activity of the STAT5 signaling to regulate αβ TCR versus γδ TCR T cell development (97). Li et al. reported that TCPTP overexpression reversed the high Th1/Treg and Th17/Treg ratios in epididymal white adipose tissue of diabetic mice (91). Spalinger et al. showed that Tcptp−/− CD4+ T cell reinfusion led to a nearly 3-fold increase in the frequency of Th1 cells, a 2-fold increase in the frequency of Th17 cells, and by contrast, a 3-fold decrease in the frequency of Tregs in colitis animal models (74). In addition, the inhibition of the interleukin-2 (IL-2)/IL-2 receptor pathway mediated by JAK1, JAK3, and STAT5 dephosphorylation was found to be the mechanism of how TCPTP drives Treg differentiation possibly (98). As illustrated above, various cytokines (e.g., IFN-γ and IL-6 from Th1 cells) released from stimulated T cells bridge the two-way communications in osteoimmune responses. These two cytokines, which are closely associated with osteoimmunology, can be regulated by TCPTP. Therefore, the interactions between the two cytokines and TCPTP in bone metabolism were discussed in detail below.
IFN-γ is a classical cytokine secreted from Th1 cells. On the one hand, IFN-γ promotes osteoclastogenesis via promoting the fusion of mononucleated pre-osteoclasts in the late period of osteoclastogenesis directly and stimulating the secretion of osteoclastic factors (such as RANKL and TNF-α)indirectly (9, 99, 100). By contrast, IFN-γ can also intensively suppress osteoclastogenesis by degrading tumor necrosis factor receptor-associated factor 6 and inhibiting the RANK/RANKL signaling pathway (6). TCPTP downregulates the IFN-γ signaling through JAK1, STAT1, and STAT3 dephosphorylation (52, 55, 59, 92). So, a rational thread of targeting TCPTP to suppress IFN-γ activity is expected to regulate osteoclastogenesis, and this hypothesis still needs more validations.
IL-6 is an inflammatory cytokine that exerts pathological effects on inflammatory bone loss (101, 102), directly supporting osteoclast formation, stimulating osteoclast differentiation, and accelerating bone resorption (103, 104). IL-6 is associated with Th17 cell differentiation (105), and abnormalities in the IL-6 signaling can disturb the Th17/Treg balance and influence bone homeostasis indirectly (106). Concerning the regulation of IL-6 by TCPTP, TCPTP curbs both IL-6 secretion and signaling. Aradi et al. confirmed that TCPTP silencing significantly increased IL-6 secretion from synovial fibroblasts in rheumatoid arthritis animals (53). TCPTP also dephosphorylate STAT3 at the Y705 site, thereby suppressing IL-6 signaling (107, 108). Besides, TCPTP inhibits the IL-6-driven pathogenic loss of Foxp3 after Tregs have acquired RORγt expression through dephosphorylation of STAT3 (109). Therefore, TCPTP can be expected to inhibit bone resorption through inhibiting IL-6 generation and IL-6 signaling directly or reversing IL-6-induced Th17/Treg imbalance indirectly.
B lymphocytes play an extremely vital role in both immune responses and bone metabolism, but the detailed relationship between B lymphocytes and osteoclastogenesis remains some points for debate. Physiologically, B cells produce osteoprotegerin to inhibit osteoclastogenesis but stimulate osteoclastogenesis via the RANK/RANKL axis in the pathological state (22, 23, 110). Recently, increasing evidence tended to support that B cell development and proliferation were crucially affected by TCPTP (Figure 2B). An impaired transition from pre-B to immature B cell was found in Tcptp−/− mice (45, 89), which might be associated with the abnormally increased release of IFN-γ and enhanced STAT1 phosphorylation in the pre-B cell compartment (81). With interleukin-21-induced hyperactivity of the STAT-3 signaling in Tcptp−/− mice, B cell proliferation was simultaneously boosted (111). These results ascertain TCPTP as an important regulator of B cells in bone homeostasis. However, concerning the complexity of the two-way effect between B lymphocytes and bone homeostasis or between TCPTP and B lymphocytes, the specific effect and the mechanisms behind each regulation should be confirmed in further explorations.
TCPTP that is expressed in osteoblasts and bone marrow stem cells participates in the regulation of bone metabolism (Figure 1). Zee et al. demonstrated that TCPTP deficiency in osteoblasts enhanced the activity of osteoclasts by activating insulin signaling and inhibiting the expression of OPG in vitro (86). Our previous study found that TCPTP improved the osteogenic differentiation ability via ERK dephosphorylation in rat bone marrow stem cells in high glucose conditions (112). However, to our knowledge, no specific studies have reported the impacts of TCPTP in bone-related cells on immune cells which may be a novel aspect in the future, and the underlying mechanism also needs further exploration.
TCPTP exerts an anti-inflammatory role in innate and adaptive immunity (113). As described in Section TCPTP and Immunomodulation, Tcptp knockout in systemic or specific immune cells brings about serious immune disorders. TCPTP deficiency also leads to subchondral bone loss and spontaneous synovitis mediated by excessive inflammatory cytokines (83). Loss-of-function variants of TCPTP increase the risk of rheumatoid arthritis due to Treg cell dysfunction (114). Therefore, using TCPTP agonists to overexpress or activate TCPTP seems to be a rational strategy against inflammatory bone resorption. This proposal can be supported by other studies. Zhang P. et al. found that TCPTP alleviated inflammatory responses and bone resorption in periodontal tissues via the JAK/STAT pathway in human oral keratinocytes and type 2 diabetes mellitus (T2DM) db/db mice (115). Zhang D.J. et al. demonstrated that TCPTP inhibited alveolar bone resorption in T2DM C57BL/6 wild-type mice via dephosphorylating CSF1 receptor (93). Consistently, our previous study series ascertained that TCPTP improves implant osseointegration in T2DM rats via ERK dephosphorylation (112). It is obvious that TCPTP could work as an effective potential target for preventing and treating inflammatory bone resorption. However, concerning the ubiquitous distribution and wide regulation of TCPTP related to glucose metabolism, immunoregulation, oncogenesis, and other various life processes in a cell or tissue-specific way, designing specific agents targeting TCPTP in specific cells, tissues or organs is a promising research direction and a novel aspect of osteoimmunology in the future.
TCPTP bridges the two-way communication between immune cells and bone cells and it could work as a potential target for the prevention and treatment of inflammatory bone diseases, such as periodontitis, synovitis, and osteoarthritis. The proposal of identifying whether TCPTP can be used as an independent therapeutic target is feasible for the development of TCPTP agonists binding to a target of interest. This review provides clear insights into the potential roles of TCPTP in osteoimmunology, which may pave the way for further bone studies on osteoimmunological aspects.
Y-NW, SL, TJ, YF, and DZ made substantial contributions to conception, design, and acquisition of data. Y-NW, SL, WZ, and XX drafted the initial manuscript. XX and DZ critically reviewed it for important intellectual content. All authors contributed to the article and approved the submitted version.
This work was supported by the National Natural Science Foundation of China (no. 82071148; no. 82001055), the Construction Engineering Special Fund of Taishan Scholars (TS201511106), the Key Project of Chinese National Programs for Research and Development (2016YFC1102705), the Shandong Provincial Natural Science Foundation (ZR2020QH158), the Fundamental Research Funds of Shandong University (2018GN024), the China Postdoctoral Science Foundation (2019M662371), and the Youth scientific research funds of School of Stomatology, Shandong University (2019QNJJ03).
The authors declare that the research was conducted in the absence of any commercial or financial relationships that could be construed as a potential conflict of interest.
1. Orsolini G, Bertoldi I, Rossini M. Osteoimmunology in rheumatoid and psoriatic arthritis: potential effects of tofacitinib on bone involvement. Clin Rheumatol (2020) 39:727–36. doi: 10.1007/s10067-020-04930-x
2. Gruber R. Osteoimmunology: Inflammatory osteolysis and regeneration of the alveolar bone. J Clin Periodontol (2019) 46 Suppl 21:52–69. doi: 10.1111/jcpe.13056
3. Chen Z, Bozec A, Ramming A, Schett G. Anti-inflammatory and immune-regulatory cytokines in rheumatoid arthritis. Nat Rev Rheumatol (2019) 15:9–17. doi: 10.1038/s41584-018-0109-2
5. Cool DE, Tonks NK, Charbonneau H, Walsh KA, Fischer EH, Krebs EG. cDNA isolated from a human T-cell library encodes a member of the protein-tyrosine-phosphatase family. Proc Natl Acad Sci USA (1989) 86:5257–61. doi: 10.1073/pnas.86.14.5257
6. Takayanagi H, Ogasawara K, Hida S, Chiba T, Murata S, Sato K, et al. T-cell-mediated regulation of osteoclastogenesis by signalling cross-talk between RANKL and IFN-gamma. Nature (2000) 408:600–5. doi: 10.1038/35046102
7. Tang M, Tian L, Luo G, Yu X. Interferon-Gamma-Mediated Osteoimmunology. Front Immunol (2018) 9:1508:1508. doi: 10.3389/fimmu.2018.01508
8. Sato K, Suematsu A, Okamoto K, Yamaguchi A, Morishita Y, Kadono Y, et al. Th17 functions as an osteoclastogenic helper T cell subset that links T cell activation and bone destruction. J Exp Med (2006) 203:2673–82. doi: 10.1084/jem.20061775
9. Kim JW, Lee MS, Lee CH, Kim HY, Chae SU, Kwak HB, et al. Effect of interferon-γ on the fusion of mononuclear osteoclasts into bone-resorbing osteoclasts. BMB Rep (2012) 45:281–6. doi: 10.5483/bmbrep.2012.45.5.281
10. Yan Y, Liu F, Kou X, Liu D, Yang R, Wang X, et al. T Cells Are Required for Orthodontic Tooth Movement. J Dent Res (2015) 94:1463–70. doi: 10.1177/0022034515595003
11. Marahleh A, Kitaura H, Ohori F, Kishikawa A, Ogawa S, Shen WR, et al. TNF-α Directly Enhances Osteocyte RANKL Expression and Promotes Osteoclast Formation. Front Immunol (2019) 10:2925:2925. doi: 10.3389/fimmu.2019.02925
12. Palmqvist P, Lundberg P, Persson E, Johansson A, Lundgren I, Lie A, et al. Inhibition of hormone and cytokine-stimulated osteoclastogenesis and bone resorption by interleukin-4 and interleukin-13 is associated with increased osteoprotegerin and decreased RANKL and RANK in a STAT6-dependent pathway. J Biol Chem (2006) 281:2414–29. doi: 10.1074/jbc.M510160200
13. Wing K, Yamaguchi T, Sakaguchi S. Cell-autonomous and -non-autonomous roles of CTLA-4 in immune regulation. Trends Immunol (2011) 32:428–33. doi: 10.1016/j.it.2011.06.002
14. Wang J, Jiang H, Qiu Y, Wang Y, Sun G, Zhao J. Effector memory regulatory T cells were most effective at suppressing RANKL but their frequency was downregulated in tibial fracture patients with delayed union. Immunol Lett (2019) 209:21–7. doi: 10.1016/j.imlet.2019.03.018
15. Luo CY, Wang L, Sun C, Li DJ. Estrogen enhances the functions of CD4(+)CD25(+)Foxp3(+) regulatory T cells that suppress osteoclast differentiation and bone resorption in vitro. Cell Mol Immunol (2011) 8:50–8. doi: 10.1038/cmi.2010.54
16. Miossec P, Korn T, Kuchroo VK. Interleukin-17 and type 17 helper T cells. N Engl J Med (2009) 361:888–98. doi: 10.1056/NEJMra0707449
17. Yasuda K, Takeuchi Y, Hirota K. The pathogenicity of Th17 cells in autoimmune diseases. Semin Immunopathol (2019) 41:283–97. doi: 10.1007/s00281-019-00733-8
18. Dey I, Bishayi B. Role of Th17 and Treg cells in septic arthritis and the impact of the Th17/Treg -derived cytokines in the pathogenesis of S. aureus induced septic arthritis in mice. Microb Pathog (2017) 113:248–64. doi: 10.1016/j.micpath.2017.10.033
19. Samuel RO, Ervolino E, de Azevedo Queiroz ÍO, Azuma MM, Ferreira GT, Cintra LTA. Th1/Th2/Th17/Treg Balance in Apical Periodontitis of Normoglycemic and Diabetic Rats. J Endod (2019) 45:1009–15. doi: 10.1016/j.joen.2019.05.003
20. Li JY, Yu M, Tyagi AM, Vaccaro C, Hsu E, Adams J, et al. IL-17 Receptor Signaling in Osteoblasts/Osteocytes Mediates PTH-Induced Bone Loss and Enhances Osteocytic RANKL Production. J Bone Miner Res (2019) 34:349–60. doi: 10.1002/jbmr.3600
21. Kotake S, Udagawa N, Takahashi N, Matsuzaki K, Itoh K, Ishiyama S, et al. IL-17 in synovial fluids from patients with rheumatoid arthritis is a potent stimulator of osteoclastogenesis. J Clin Invest (1999) 103:1345–52. doi: 10.1172/jci5703
22. Li Y, Toraldo G, Li A, Yang X, Zhang H, Qian WP, et al. B cells and T cells are critical for the preservation of bone homeostasis and attainment of peak bone mass in vivo. Blood (2007) 109:3839–48. doi: 10.1182/blood-2006-07-037994
23. Weitzmann MN. T-cells and B-cells in osteoporosis. Curr Opin Endocrinol Diabetes Obes (2014) 21:461–7. doi: 10.1097/med.0000000000000103
24. Rivollier A, Mazzorana M, Tebib J, Piperno M, Aitsiselmi T, Rabourdin-Combe C, et al. Immature dendritic cell transdifferentiation into osteoclasts: a novel pathway sustained by the rheumatoid arthritis microenvironment. Blood (2004) 104:4029–37. doi: 10.1182/blood-2004-01-0041
25. Speziani C, Rivollier A, Gallois A, Coury F, Mazzorana M, Azocar O, et al. Murine dendritic cell transdifferentiation into osteoclasts is differentially regulated by innate and adaptive cytokines. Eur J Immunol (2007) 37:747–57. doi: 10.1002/eji.200636534
26. Madel MB, Ibáñez L, Wakkach A, de Vries TJ, Teti A, Apparailly F, et al. Immune Function and Diversity of Osteoclasts in Normal and Pathological Conditions. Front Immunol (2019) 10:1408:1408. doi: 10.3389/fimmu.2019.01408
27. Dhodapkar KM, Barbuto S, Matthews P, Kukreja A, Mazumder A, Vesole D, et al. Dendritic cells mediate the induction of polyfunctional human IL17-producing cells (Th17-1 cells) enriched in the bone marrow of patients with myeloma. Blood (2008) 112:2878–85. doi: 10.1182/blood-2008-03-143222
28. Kwan Tat S, Padrines M, Théoleyre S, Heymann D, Fortun Y. IL-6, RANKL, TNF-alpha/IL-1: interrelations in bone resorption pathophysiology. Cytokine Growth Factor Rev (2004) 15:49–60. doi: 10.1016/j.cytogfr.2003.10.005
29. Mercier FE, Ragu C, Scadden DT. The bone marrow at the crossroads of blood and immunity. Nat Rev Immunol (2011) 12:49–60. doi: 10.1038/nri3132
30. Taichman RS, Emerson SG. Human osteoblasts support hematopoiesis through the production of granulocyte colony-stimulating factor. J Exp Med (1994) 179:1677–82. doi: 10.1084/jem.179.5.1677
31. Nakamura Y, Arai F, Iwasaki H, Hosokawa K, Kobayashi I, Gomei Y, et al. Isolation and characterization of endosteal niche cell populations that regulate hematopoietic stem cells. Blood (2010) 116:1422–32. doi: 10.1182/blood-2009-08-239194
32. Zhu J, Garrett R, Jung Y, Zhang Y, Kim N, Wang J, et al. Osteoblasts support B-lymphocyte commitment and differentiation from hematopoietic stem cells. Blood (2007) 109:3706–12. doi: 10.1182/blood-2006-08-041384
33. Wu JY, Purton LE, Rodda SJ, Chen M, Weinstein LS, McMahon AP, et al. Osteoblastic regulation of B lymphopoiesis is mediated by Gs{alpha}-dependent signaling pathways. Proc Natl Acad Sci USA (2008) 105:16976–81. doi: 10.1073/pnas.0802898105
34. Yu VW, Saez B, Cook C, Lotinun S, Pardo-Saganta A, Wang YH, et al. Specific bone cells produce DLL4 to generate thymus-seeding progenitors from bone marrow. J Exp Med (2015) 212:759–74. doi: 10.1084/jem.20141843
35. Asada N, Katayama Y, Sato M, Minagawa K, Wakahashi K, Kawano H, et al. Matrix-embedded osteocytes regulate mobilization of hematopoietic stem/progenitor cells. Cell Stem Cell (2013) 12:737–47. doi: 10.1016/j.stem.2013.05.001
36. Azab E, Chandler KB, Uda Y, Sun N, Hussein A, Shuwaikan R, et al. Osteocytes control myeloid cell proliferation and differentiation through Gsα-dependent and -independent mechanisms. FASEB J (2020) 34:10191–211. doi: 10.1096/fj.202000366R
37. Fujiwara Y, Piemontese M, Liu Y, Thostenson JD, Xiong J, O’Brien CA. RANKL (Receptor Activator of NFκB Ligand) Produced by Osteocytes Is Required for the Increase in B Cells and Bone Loss Caused by Estrogen Deficiency in Mice. J Biol Chem (2016) 291:24838–50. doi: 10.1074/jbc.M116.742452
38. Kollet O, Dar A, Shivtiel S, Kalinkovich A, Lapid K, Sztainberg Y, et al. Osteoclasts degrade endosteal components and promote mobilization of hematopoietic progenitor cells. Nat Med (2006) 12:657–64. doi: 10.1038/nm1417
39. Miyamoto K, Yoshida S, Kawasumi M, Hashimoto K, Kimura T, Sato Y, et al. Osteoclasts are dispensable for hematopoietic stem cell maintenance and mobilization. J Exp Med (2011) 208:2175–81. doi: 10.1084/jem.20101890
40. Kiesel JR, Buchwald ZS, Aurora R. Cross-presentation by osteoclasts induces FoxP3 in CD8+ T cells. J Immunol (2009) 182:5477–87. doi: 10.4049/jimmunol.0803897
41. Ibáñez L, Abou-Ezzi G, Ciucci T, Amiot V, Belaïd N, Obino D, et al. Inflammatory Osteoclasts Prime TNFα-Producing CD4(+) T Cells and Express CX(3) CR1. J Bone Miner Res (2016) 31:1899–908. doi: 10.1002/jbmr.2868
42. Bussieres-Marmen S, Hutchins AP, Schirbel A, Rebert N, Tiganis T, Fiocchi C, et al. Characterization of PTPN2 and its use as a biomarker. Methods (2014) 65:239–46. doi: 10.1016/j.ymeth.2013.08.020
43. Tiganis T, Kemp BE, Tonks NK. The protein-tyrosine phosphatase TCPTP regulates epidermal growth factor receptor-mediated and phosphatidylinositol 3-kinase-dependent signaling. J Biol Chem (1999) 274:27768–75. doi: 10.1074/jbc.274.39.27768
44. Sharp RC, Abdulrahim M, Naser ES, Naser SA. Genetic Variations of PTPN2 and PTPN22: Role in the Pathogenesis of Type 1 Diabetes and Crohn’s Disease. Front Cell Infect Microbiol (2015) 5:95. doi: 10.3389/fcimb.2015.00095
45. Doody KM, Bourdeau A, Tremblay ML. T-cell protein tyrosine phosphatase is a key regulator in immune cell signaling: lessons from the knockout mouse model and implications in human disease. Immunol Rev (2009) 228:325–41. doi: 10.1111/j.1600-065X.2008.00743.x
46. Stuible M, Doody KM, Tremblay ML. PTP1B and TC-PTP: regulators of transformation and tumorigenesis. Cancer Metastasis Rev (2008) 27:215–30. doi: 10.1007/s10555-008-9115-1
47. LaFleur MW, Nguyen TH. PTPN2 regulates the generation of exhausted CD8(+) T cell subpopulations and restrains tumor immunity. Nat Immunol (2019) 20:1335–47. doi: 10.1038/s41590-019-0480-4
48. Manguso RT, Pope HW, Zimmer MD, Brown FD, Yates KB, Miller BC, et al. In vivo CRISPR screening identifies Ptpn2 as a cancer immunotherapy target. Nature (2017) 547:413–18. doi: 10.1038/nature23270
49. Wiede F, Lu K-H, Du X, Liang S, Hochheiser K, Dodd GT, et al. PTPN2 phosphatase deletion in T cells promotes anti-tumour immunity and CAR T-cell efficacy in solid tumours. EMBO J (2020) 39:e103637–e37. doi: 10.15252/embj.2019103637
50. Wiede F, Brodnicki TC. T-Cell-Specific PTPN2 Deficiency in NOD Mice Accelerates the Development of Type 1 Diabetes and Autoimmune Comorbidities. Diabetes (2019) 68:1251–66. doi: 10.2337/db18-1362
51. Scharl M, McCole DF, Weber A, Vavricka SR, Frei P, Kellermeier S, et al. Protein tyrosine phosphatase N2 regulates TNFalpha-induced signalling and cytokine secretion in human intestinal epithelial cells. Gut (2011) 60:189–97. doi: 10.1136/gut.2010.216606
52. Scharl M, Hruz P, McCole DF. Protein tyrosine phosphatase non-receptor Type 2 regulates IFN-gamma-induced cytokine signaling in THP-1 monocytes. Inflammation Bowel Dis (2010) 16:2055–64. doi: 10.1002/ibd.21325
53. Aradi B, Kato M, Filkova M, Karouzakis E, Klein K, Scharl M, et al. Protein tyrosine phosphatase nonreceptor type 2: an important regulator of lnterleukin-6 production in rheumatoid arthritis synovial fibroblasts. Arthritis Rheumatol (2015) 67:2624–33. doi: 10.1002/art.39256
54. Parlato M, Nian Q, Charbit-Henrion F, Ruemmele FM, Rodrigues-Lima F, Cerf-Bensussan N. Loss-of-Function Mutation in PTPN2 Causes Aberrant Activation of JAK Signaling Via STAT and Very Early Onset Intestinal Inflammation. Gastroenterology (2020) 159:1968–71. doi: 10.1053/j.gastro.2020.07.040
55. Simoncic PD, Lee-Loy A, Barber DL, Tremblay ML, McGlade CJ. The T cell protein tyrosine phosphatase is a negative regulator of janus family kinases 1 and 3. Curr Biol (2002) 12:446–53. doi: 10.1016/s0960-9822(02)00697-8
56. Krishnan M, McCole DF. T cell protein tyrosine phosphatase prevents STAT1 induction of claudin-2 expression in intestinal epithelial cells. Ann New Y Acad Sci (2017) 1405:116–30. doi: 10.1111/nyas.13439
57. Fukushima A, Loh K, Galic S, Fam B, Shields B, Wiede F, et al. T-cell protein tyrosine phosphatase attenuates STAT3 and insulin signaling in the liver to regulate gluconeogenesis. Diabetes (2010) 59:1906–14. doi: 10.2337/db09-1365
58. Gurzov EN, Tran M, Fernandez-Rojo MA, Merry TL, Zhang X, Xu Y, et al. Hepatic oxidative stress promotes insulin-STAT-5 signaling and obesity by inactivating protein tyrosine phosphatase N2. Cell Metab (2014) 20:85–102. doi: 10.1016/j.cmet.2014.05.011
59. ten Hoeve J, de Jesus Ibarra-Sanchez M, Fu Y, Zhu W, Tremblay M, David M, et al. Identification of a nuclear Stat1 protein tyrosine phosphatase. Mol Cell Biol (2002) 22:5662–68. doi: 10.1128/mcb.22.16.5662-5668.2002
60. Galic S, Klingler-Hoffmann M, Fodero-Tavoletti MT, Puryer MA, Meng TC, Tonks NK, et al. Regulation of insulin receptor signaling by the protein tyrosine phosphatase TCPTP. Mol Cell Biol (2003) 23:2096–108. doi: 10.1128/mcb.23.6.2096-2108.2003
61. Dodd GT, Lee-Young RS, Bruning JC, Tiganis T. TCPTP Regulates Insulin Signaling in AgRP Neurons to Coordinate Glucose Metabolism With Feeding. Diabetes (2018) 67:1246–57. doi: 10.2337/db17-1485
62. Tiganis T, Bennett AM, Ravichandran KS, Tonks NK. Epidermal growth factor receptor and the adaptor protein p52Shc are specific substrates of T-cell protein tyrosine phosphatase. Mol Cell Biol (1998) 18:1622–34. doi: 10.1128/mcb.18.3.1622
63. Stanoev A, Mhamane A, Schuermann KC, Grecco HE, Stallaert W, Baumdick M, et al. and Phosphatases Spatially Established by Vesicular Dynamics Generates a Growth Factor Sensing and Responding Network. Cell Syst (2018) 7:295–309.e11. doi: 10.1016/j.cels.2018.06.006
64. Mattila E, Auvinen K, Salmi M, Ivaska J. The protein tyrosine phosphatase TCPTP controls VEGFR2 signalling. J Cell Sci (2008) 121:3570–80. doi: 10.1242/jcs.031898
65. Persson C, Sävenhed C, Bourdeau A, Tremblay ML, Markova B, Böhmer FD, et al. Site-selective regulation of platelet-derived growth factor beta receptor tyrosine phosphorylation by T-cell protein tyrosine phosphatase. Mol Cell Biol (2004) 24:2190–201. doi: 10.1128/mcb.24.5.2190-2201.2004
66. Kramer F, Dernedde J, Mezheyeuski A, Tauber R, Micke P, Kappert K. Platelet-derived growth factor receptor β activation and regulation in murine myelofibrosis. Haematologica (2020) 105:2083–94. doi: 10.3324/haematol.2019.226332
67. Simoncic PD, Bourdeau A, Lee-Loy A, Rohrschneider LR, Tremblay ML, Stanley ER, et al. T-cell protein tyrosine phosphatase (Tcptp) is a negative regulator of colony-stimulating factor 1 signaling and macrophage differentiation. Mol Cell Biol (2006) 26:4149–60. doi: 10.1128/MCB.01932-05
68. Lees CW, Barrett JC, Parkes M, Satsangi J. New IBD genetics: common pathways with other diseases. Gut (2011) 60:1739–53. doi: 10.1136/gut.2009.199679
69. Marcil V, Mack DR, Kumar V, Faure C, Carlson CS, Beaulieu P, et al. Association between the PTPN2 gene and Crohn’s disease: dissection of potential causal variants. Inflammation Bowel Dis (2013) 19:1149–55. doi: 10.1097/MIB.0b013e318280b181
70. Zhang Q, Li H, Hou S, Yu H, Su G, Deng B, et al. Association of genetic variations in PTPN2 and CD122 with ocular Behcet’s disease. Br J Ophthalmol (2018) 102:996–1002. doi: 10.1136/bjophthalmol-2017-310820
71. Killock D. Genetics: Etiological insight into the genetic association of TCPTP with RA and JIA? Nat Rev Rheumatol (2011) 7:683. doi: 10.1038/nrrheum.2011.167
72. You-Ten KE, Muise ES, Itié A, Michaliszyn E, Wagner J, Jothy S, et al. Impaired bone marrow microenvironment and immune function in T cell protein tyrosine phosphatase-deficient mice. J Exp Med (1997) 186:683–93. doi: 10.1084/jem.186.5.683
73. Heinonen KM, Nestel FP, Newell EW, Charette G, Seemayer TA, Tremblay ML, et al. T-cell protein tyrosine phosphatase deletion results in progressive systemic inflammatory disease. Blood (2004) 103:3457–64. doi: 10.1182/blood-2003-09-3153
74. Spalinger MR, Kasper S, Chassard C, Raselli T, Frey-Wagner I, Gottier C, et al. PTPN2 controls differentiation of CD4(+) T cells and limits intestinal inflammation and intestinal dysbiosis. Mucosal Immunol (2015) 8:918–29. doi: 10.1038/mi.2014.122
75. Wiede F, Shields BJ, Chew SH, Kyparissoudis K, van Vliet C, Galic S, et al. T cell protein tyrosine phosphatase attenuates T cell signaling to maintain tolerance in mice. J Clin Invest (2011) 121:4758–74. doi: 10.1172/JCI59492
76. Spalinger MR, Manzini R, Hering L, Riggs JB, Gottier C, Lang S, et al. PTPN2 Regulates Inflammasome Activation and Controls Onset of Intestinal Inflammation and Colon Cancer. Cell Rep (2018) 22:1835–48. doi: 10.1016/j.celrep.2018.01.052
77. Spalinger MR, Sayoc-Becerra A, Santos AN, Shawki A, Canale V, Krishnan M, et al. PTPN2 Regulates Interactions Between Macrophages and Intestinal Epithelial Cells to Promote Intestinal Barrier Function. Gastroenterology (2020) 159:1763–77. doi: 10.1053/j.gastro.2020.07.004
78. Zheng L, Zhang W, Li A, Liu Y, Yi B, Nakhoul F, et al. PTPN2 Downregulation Is Associated with Albuminuria and Vitamin D Receptor Deficiency in Type 2 Diabetes Mellitus. J Diabetes Res (2018) 2018:3984797. doi: 10.1155/2018/3984797
79. Dzierzak E, Bigas A. Blood Development: Hematopoietic Stem Cell Dependence and Independence. Cell Stem Cell (2018) 22:639–51. doi: 10.1016/j.stem.2018.04.015
80. Bourdeau A, Trop S, Doody KM, Dumont DJ, Tremblay ML. Inhibition of T cell protein tyrosine phosphatase enhances interleukin-18-dependent hematopoietic stem cell expansion. Stem Cells (2013) 31:293–304. doi: 10.1002/stem.1276
81. Bourdeau A, Dube N, Heinonen KM, Theberge JF, Doody KM, Tremblay ML. TC-PTP-deficient bone marrow stromal cells fail to support normal B lymphopoiesis due to abnormal secretion of interferon-{gamma}. Blood (2007) 109:4220–8. doi: 10.1182/blood-2006-08-044370
82. Wiede F, Chew SH, van Vliet C, Poulton IJ, Kyparissoudis K, Sasmono T, et al. Strain-dependent differences in bone development, myeloid hyperplasia, morbidity and mortality in ptpn2-deficient mice. PloS One (2012) 7:e36703. doi: 10.1371/journal.pone.0036703
83. Doody KM, Bussières-Marmen S, Li A, Paquet M, Henderson JE, Tremblay ML. T cell protein tyrosine phosphatase deficiency results in spontaneous synovitis and subchondral bone resorption in mice. Arthritis Rheumatism (2012) 64:752–61. doi: 10.1002/art.33399
84. Loh K, Fukushima A, Zhang X, Galic S, Briggs D, Enriori Pablo J, et al. Elevated Hypothalamic TCPTP in Obesity Contributes to Cellular Leptin Resistance. Cell Metab (2011) 14:684–99. doi: 10.1016/j.cmet.2011.09.011
85. Ferron M, Wei J, Yoshizawa T, Del Fattore A, DePinho RA, Teti A, et al. Insulin signaling in osteoblasts integrates bone remodeling and energy metabolism. Cell (2010) 142:296–308. doi: 10.1016/j.cell.2010.06.003
86. Zee T, Settembre C, Levine RL. Karsenty G. T-cell protein tyrosine phosphatase regulates bone resorption and whole-body insulin sensitivity through its expression in osteoblasts. Mol Cell Biol (2012) 32:1080–88. doi: 10.1128/MCB.06279-11
87. Sinder BP, Pettit AR, McCauley LK. Macrophages: Their Emerging Roles in Bone. J Bone Miner Res (2015) 30:2140–9. doi: 10.1002/jbmr.2735
88. Spalinger MR, Sayoc-Becerra A, Ordookhanian C, Canale V, Santos AN, King SJ, et al. The JAK inhibitor tofacitinib rescues intestinal barrier defects caused by disrupted epithelial-macrophage interactions. J Crohns Colitis (2020) jjaa182. doi: 10.1093/ecco-jcc/jjaa182
89. You-Ten KE, Muise ES, Itié A, Michaliszyn E, Wagner J, Jothy S, et al. Impaired bone marrow microenvironment and immune function in T cell protein tyrosine phosphatase-deficient mice. J Exp Med (1997) 186:683–93. doi: 10.1084/jem.186.5.683
90. He D, Kou X, Luo Q, Yang R, Liu D, Wang X, et al. Enhanced M1/M2 macrophage ratio promotes orthodontic root resorption. J Dent Res (2015) 94:129–39. doi: 10.1177/0022034514553817
91. Li Y, Zhou HM, Wang F, Han L, Liu MH, Li YH, et al. Overexpression of PTPN2 in Visceral Adipose Tissue Ameliorated Atherosclerosis via T Cells Polarization Shift in Diabetic Apoe(-/-) Mice. Cell Physiol Biochem (2018) 46:118–32. doi: 10.1159/000488415
92. Heinonen KM, Bourdeau A, Doody KM, Tremblay ML. Protein tyrosine phosphatases PTP-1B and TC-PTP play nonredundant roles in macrophage development and IFN-gamma signaling. Proc Natl Acad Sci USA (2009) 106:9368–72. doi: 10.1073/pnas.0812109106
93. Zhang D, Jiang Y, Song D, Zhu Z, Zhou C, Dai L, et al. Tyrosine-protein phosphatase non-receptor type 2 inhibits alveolar bone resorption in diabetic periodontitis via dephosphorylating CSF1 receptor. J Cell Mol Med (2019) 23:6690–99. doi: 10.1111/jcmm.14545
94. Rhee I, Veillette A. Protein tyrosine phosphatases in lymphocyte activation and autoimmunity. Nat Immunol (2012) 13:439–47. doi: 10.1038/ni.2246
95. Flosbach M, Oberle SG, Scherer S, Zecha J, von Hoesslin M, Wiede F, et al. PTPN2 Deficiency Enhances Programmed T Cell Expansion and Survival Capacity of Activated T Cells. Cell Rep (2020) 32:107957. doi: 10.1016/j.celrep.2020.107957
96. Pike KA, Tremblay ML. Protein Tyrosine Phosphatases: Regulators of CD4 T Cells in Inflammatory Bowel Disease. Front Immunol (2018) 9:2504:2504. doi: 10.3389/fimmu.2018.02504
97. Wiede F, Dudakov JA, Lu K-H, Dodd GT, Butt T, Godfrey DI, et al. PTPN2 regulates T cell lineage commitment and αβ versus γδ specification. J Exp Med (2017) 214:2733–58. doi: 10.1084/jem.20161903
98. Yi Z, Lin WW, Stunz LL, Bishop GA. The adaptor TRAF3 restrains the lineage determination of thymic regulatory T cells by modulating signaling via the receptor for IL-2. Nat Immunol (2014) 15:866–74. doi: 10.1038/ni.2944
99. Cenci S, Toraldo G, Weitzmann MN, Roggia C, Gao Y, Qian WP, et al. Estrogen deficiency induces bone loss by increasing T cell proliferation and lifespan through IFN-gamma-induced class II transactivator. Proc Natl Acad Sci USA (2003) 100:10405–10. doi: 10.1073/pnas.1533207100
100. Gao Y, Grassi F, Ryan MR, Terauchi M, Page K, Yang X, et al. IFN-gamma stimulates osteoclast formation and bone loss in vivo via antigen-driven T cell activation. J Clin Invest (2007) 117:122–32. doi: 10.1172/jci30074
101. Lazzaro L, Tonkin BA, Poulton IJ, McGregor NE, Ferlin W, Sims NA. IL-6 trans-signalling mediates trabecular, but not cortical, bone loss after ovariectomy. Bone (2018) 112:120–27. doi: 10.1016/j.bone.2018.04.015
102. Kuroyanagi G, Adapala NS, Yamaguchi R, Kamiya N, Deng Z, Aruwajoye O, et al. Interleukin-6 deletion stimulates revascularization and new bone formation following ischemic osteonecrosis in a murine model. Bone (2018) 116:221–31. doi: 10.1016/j.bone.2018.08.011
103. Udagawa N, Takahashi N, Katagiri T, Tamura T, Wada S, Findlay DM, et al. Interleukin (IL)-6 induction of osteoclast differentiation depends on IL-6 receptors expressed on osteoblastic cells but not on osteoclast progenitors. J Exp Med (1995) 182:1461–8. doi: 10.1084/jem.182.5.1461
104. Wu Q, Zhou X, Huang D, Ji Y, Kang F. IL-6 Enhances Osteocyte-Mediated Osteoclastogenesis by Promoting JAK2 and RANKL Activity In Vitro. Cell Physiol Biochem (2017) 41:1360–69. doi: 10.1159/000465455
105. Yamashita T, Iwakura T, Matsui K, Kawaguchi H, Obana M, Hayama A, et al. IL-6-mediated Th17 differentiation through RORγt is essential for the initiation of experimental autoimmune myocarditis. Cardiovasc Res (2011) 91:640–8. doi: 10.1093/cvr/cvr148
106. Aqel SI, Kraus EE, Jena N, Kumari V, Granitto MC, Mao L, et al. Novel small molecule IL-6 inhibitor suppresses autoreactive Th17 development and promotes T(reg) development. Clin Exp Immunol (2019) 196:215–25. doi: 10.1111/cei.13258
107. Zhang Y, Ding H, Wang X, Ye SD. Modulation of STAT3 phosphorylation by PTPN2 inhibits naive pluripotency of embryonic stem cells. FEBS Lett (2018) 592:2227–37. doi: 10.1002/1873-3468.13112
108. Yamamoto T, Sekine Y, Kashima K, Kubota A, Sato N, Aoki N, et al. The nuclear isoform of protein-tyrosine phosphatase TC-PTP regulates interleukin-6-mediated signaling pathway through STAT3 dephosphorylation. Biochem Biophys Res Commun (2002) 297:811–7. doi: 10.1016/s0006-291x(02)02291-x
109. Svensson MN, Doody KM, Schmiedel BJ, Bhattacharyya S, Panwar B, Wiede F, et al. Reduced expression of phosphatase PTPN2 promotes pathogenic conversion of Tregs in autoimmunity. J Clin Invest (2019) 129:1193–210. doi: 10.1172/JCI123267
110. Onal M, Xiong J, Chen X, Thostenson JD, Almeida M, Manolagas SC, et al. Receptor activator of nuclear factor κB ligand (RANKL) protein expression by B lymphocytes contributes to ovariectomy-induced bone loss. J Biol Chem (2012) 287:29851–60. doi: 10.1074/jbc.M112.377945
111. Wiede F, Sacirbegovic F, Leong YA, Yu D, Tiganis T. PTPN2-deficiency exacerbates T follicular helper cell and B cell responses and promotes the development of autoimmunity. J Autoimmun (2017) 76:85–100. doi: 10.1016/j.jaut.2016.09.004
112. Wang YN, Jia T, Zhang J, Lan J, Zhang D, Xu X. PTPN2 improves implant osseointegration in T2DM via inducing the dephosphorylation of ERK. Exp Biol Med (Maywood) (2019) 244:1493–503. doi: 10.1177/1535370219883419
113. Niechcial A, Butter M, Manz S, Obialo N, Bäbler K, van der Lely L, et al. Presence of PTPN2 SNP rs1893217 Enhances the Anti-inflammatory Effect of Spermidine. Inflammation Bowel Dis (2020) 26:1038–49. doi: 10.1093/ibd/izaa013
114. Hsieh WC, Svensson MN, Zoccheddu M, Tremblay ML, Sakaguchi S, Stanford SM, et al. PTPN2 links colonic and joint inflammation in experimental autoimmune arthritis. JCI Insight (2020) 5:e141868. doi: 10.1172/jci.insight.141868
115. Zhang P, Zhang W, Zhang D, Wang M, Aprecio R, Ji N, et al. 25-Hydroxyvitamin D3 -enhanced PTPN2 positively regulates periodontal inflammation through the JAK/STAT pathway in human oral keratinocytes and a mouse model of type 2 diabetes mellitus. J Periodontal Res (2018) 53:467–77. doi: 10.1111/jre.12535
Keywords: T cell protein tyrosine phosphatase (TCPTP), Protein tyrosine phosphatase non-receptor 2 (PTPN2), osteoimmunology, macrophages, T cell, B cell
Citation: Wang Y-N, Liu S, Jia T, Feng Y, Zhang W, Xu X and Zhang D (2021) T Cell Protein Tyrosine Phosphatase in Osteoimmunology. Front. Immunol. 12:620333. doi: 10.3389/fimmu.2021.620333
Received: 22 October 2020; Accepted: 04 January 2021;
Published: 22 February 2021.
Edited by:
Teun J. De Vries, VU University Amsterdam, NetherlandsReviewed by:
Maria-Bernadette Madel, Baylor College of Medicine, United StatesCopyright © 2021 Wang, Liu, Jia, Feng, Zhang, Xu and Zhang. This is an open-access article distributed under the terms of the Creative Commons Attribution License (CC BY). The use, distribution or reproduction in other forums is permitted, provided the original author(s) and the copyright owner(s) are credited and that the original publication in this journal is cited, in accordance with accepted academic practice. No use, distribution or reproduction is permitted which does not comply with these terms.
*Correspondence: Dongjiao Zhang, ZGp6aGFuZzExMDlAMTYzLmNvbQ==
†These authors have contributed equally to this work
Disclaimer: All claims expressed in this article are solely those of the authors and do not necessarily represent those of their affiliated organizations, or those of the publisher, the editors and the reviewers. Any product that may be evaluated in this article or claim that may be made by its manufacturer is not guaranteed or endorsed by the publisher.
Research integrity at Frontiers
Learn more about the work of our research integrity team to safeguard the quality of each article we publish.