- 1Department of Pathophysiology and Key Laboratory of Pathobiology, Ministry of Education, College of Basic Medical Sciences, Jilin University, Changchun, China
- 2Department of Microbiology and Immunology and Greenebaum Comprehensive Cancer Center, School of Medicine, University of Maryland, Baltimore, Baltimore, MD, United States
- 3Department of Plastic Surgery, China-Japan Union Hospital, Jilin University, Changchun, China
Since the first reported spontaneous regression of tumors in patients with streptococcus infection, cancer biological therapy was born and it evolved into today’s immunotherapy over the last century. Although the original strategy was unable to impart maximal therapeutic benefit at the beginning, it laid the foundations for the development of immune checkpoint blockade and CAR-T which are currently used for cancer treatment in the clinics. However, clinical applications have shown that current cancer immunotherapy can cause a series of adverse reactions and are captious for patients with preexisting autoimmune disorders. Salmonellae was first reported to exert antitumor effect in 1935. Until now, numerous studies have proved its potency as an antitumor agent in the near future. In this review, we summarize the currently available data on the antitumor effects of Salmonella, and discussed a possibility of integrating Salmonella into cancer immunotherapy to overcome current obstacles.
Introduction
Using bacteria to treat tumors has long been a controversial subject. Dr. William B. Coley, a surgeon at the New York Hospital, first reported the spontaneous regression of tumors in patients with streptococcus infection at the end of the 19th century (1). In the later 40 years, Coley concentrated on applying the “Coley’s Toxins”, a variety of antitumor mixture of heat-killed Streptococcus pyogenes and Serratia marcescens, to patients with sarcomas, carcinomas, lymphomas, melanomas, and myelomas (2, 3). By simulating symptoms of infection without the risks of bacteremia, “Coley’s Toxins” account for complete tumor regression in many cases (2, 3). Subsequently, Salmonella was first observed a tumor therapeutic effect on animal models from a hemorrhagic allergy experiment reported in 1935 (4). However, because of the concerns about non-reproducible, uncertain, and unpredictable effects in the early trials, the development of chemoradiotherapy and surgical treatment soon rose to prominence and took a dominant position in the following decades for tumor therapy (3). The success of Bacillus Calmette Guerin (BCG) against superficial bladder cancer once again brought attention to the treatment of tumors with bacteria (5, 6). With the development of molecular biology, comprehensive sequencing of bacterial genomes and abundance of gene construction methods have greatly enhanced the plasticity of bacteria as antitumor agents (7). Currently, the antitumor effects of Salmonella have been widely studied pre-clinically, in which Salmonella can target and colonize into tumor tissues, directly kill tumor cells, and cause changes in tumor immune microenvironment to enhance host tumor recognition and elimination (8, 9). Nevertheless, clinical studies showed that the robust antitumor effects of Salmonella have not been fully exhibited in human patients (8), which raises a further claim to the exploration of Salmonella modification, as well as augment other treatment strategies.
Presently, remarkable clinical achievements of immune checkpoint blockade and chimeric antigen receptor T cell therapies (CAR-T) enable cancer immunotherapy as another important antitumor strategy after chemoradiotherapy and surgical treatment (10). However, clinical studies in recent years have also revealed the side effects, cancer heterogeneity and limitations of patient selection in cancer immunotherapy (1, 11). In order to expand the applications, obtain durable therapeutic responses and reduce related adverse events of cancer immunotherapy, more precise treatment, complex combinations of immunomodulatory agents, and further activation of host immune system against tumor cells become the future research direction.
In this review, we summarize the present research on the antitumor effects of Salmonella, and describe the development and limitations of current cancer immunotherapy. We will also consider the advantages of Salmonella in tumor targeting, immune regulation and engineering plasticity. In conclusion, Salmonella is expected to further enhance the consistency, durability and effectiveness of cancer immunotherapy.
Salmonella in Cancer Therapy
Many bacteria have been found the capacity to colonize into tumor microenvironment (TME) (12). Among them, Salmonella typhi first showed a significant tumor therapeutic effect on sarcoma in mouse model (4). The antitumor effect of Salmonella is mainly exerted through bacterial-intrinsic and host immune mechanisms. The attenuation of bacteria, which reduces the bacterial toxicity owing to the secretory factors, may diminish the intrinsic oncolytic activity of bacteria to a certain extent (12, 13). Such loss of intrinsic antitumor capacity may also augment the other capacity of Salmonella, e.g., host immune response against tumors (14). As early as the 19th century, it was discovered that bacteria and their products stimulate the host immune system for inhibiting tumor growth (15, 16). Since the first report about Salmonella inhibiting solid tumor growth in 1930s (4), numerous studies have confirmed the ability of Salmonella to stimulate immune system against cancer (Figure 1) (3, 8). Both innate (17, 18) and adaptive immune responses are augmented (19–21).
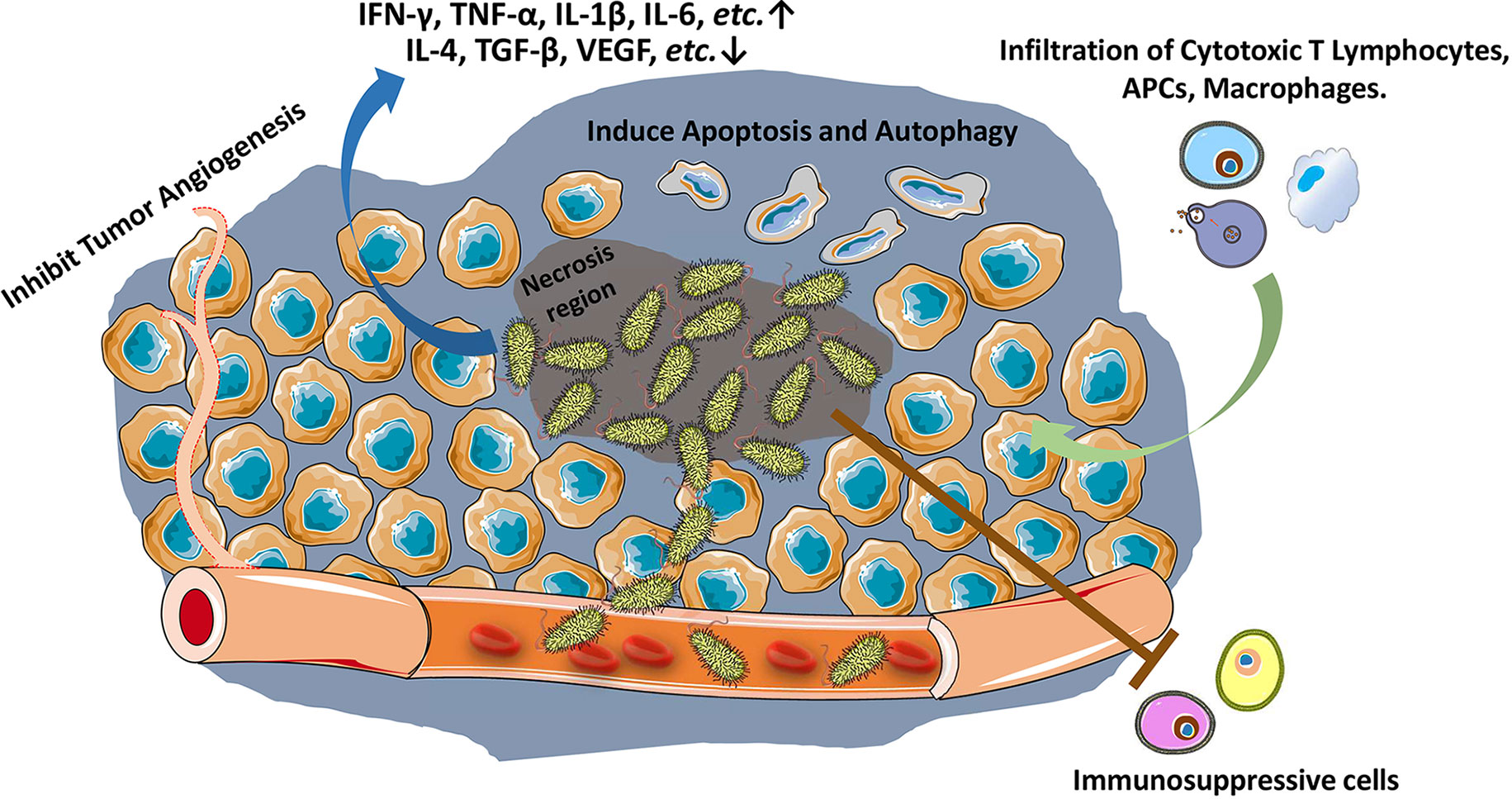
Figure 1 Salmonella stimulates host immune response against tumors. Salmonella accumulates in tumors (especially in necrosis region), inhibits tumor angiogenesis, and induces apoptosis and autophagy in tumor cells. Salmonella increases and activates cytotoxic T lymphocytes, antigen presenting cells (APCs) and macrophages against tumor cells, reduces tumor infiltration of Treg cells, and ablates the immunosuppressive capacity of myeloid-derived suppressor cells (MDSCs) and Tumor-associated macrophages.
Tumor Targeting Capacity
Salmonella has been shown enrich in the tumors > 1,000-fold over in normal tissues and cause robust antitumor effects in animal models (8). Upon administration into animals, the initial levels of Salmonella between tumors and other tissues were not significantly different. Salmonellae in the circulatory system and other tissues were cleared within hours to days, while Salmonella that entered tumors took footing, colonized and grew (22). The tumor-homing ability of bacteria is probably related to the unique immunosuppressive and biochemical environment of tumors (23). Although tumor-targeting mechanism of Salmonella is still not completely understood, it might be related to the following aspects (Figure 2):
Hypoxia in tumors permits bacterial colonization of Salmonella, a facultative anaerobe (8). Salmonellae in tumors migrate away from vasculature and prefer to thrive in the necrotic zones (24). This is also partly due to the availability of nutrients in the necrotic area for bacteria and concealment from the immune system (25). Studies involving both anaerobe and facultative anaerobe showed that the intravenously injected bacteria did not colonize in other non-tumor tissues with hypoxia or inflammatory lesions (26), indicating other potential mechanisms for their tumor homing ability. The genetically modified Salmonella typhimurium strain YB1 possessed an enhanced hypoxia selectivity exhibited a stronger tumor-targeting and antitumor ability than the parental strain SL7207 (27). Chemotaxis toward components in TME is another potential mechanism that accounts for the preferential homing into tumors by Salmonella. Each chemotactic receptor may distinctly guide Salmonella into target tumors, depending on the TME. Kasinskas et al. found that chemotaxis of Salmonella is essential for the initial accumulation in tumors, where the quiescent and necrotic cells are crucial for their survival (28). Further research suggests that responsive chemotactic receptors (aspartate, serine and ribose/galactose receptor) can initiate chemotaxis, penetration and direct Salmonellae toward necrosis (29). Silva-Valenzuela et al. (30, 70) showed that the chemotactic gene cheY and the motility gene motAB of Salmonella are crucial for colonizing tumors. And eutC, an ethanolamine metabolism related gene, also confers a tumor preference of Salmonella (30, 31). In addition, motility (flagella) is also necessary for tumor-targeting ability as mutated Salmonella fail to colonize the tumors (32). Interestingly, Stritzker et al. (33) arrived at an opposite conclusion. Their in vivo studies showed that chemotaxis and motility of Salmonella have no impact on tumor colonization. They suggest that the tumor-trafficking ability may be related to a passive mechanism by bacterial metabolism and host macrophages (33). Furthermore, studies in B cell-deficient mice showed higher accumulation of Salmonellae in tumors and increased tumor growth inhibition. However, B cells are also required for tumor targeting by restricting the dissemination of Salmonella to normal tissues (19). Salmonella can induce large amounts of blood flow into tumor tissues by increasing the secretion of TNF-α, which in turn leads to more Salmonella entering tumor tissues (34). The tumor targeting ability also contributes to another application of Salmonella. Panteli et al. (35) created an engineered fluorescein-secreting Salmonella to detect small tumors and metastases. As a nest for Salmonella accumulation, tumors and metastases constantly export fluorescent biomarker for detection (35). Similarly, Xiong et al. administrated Salmonella carrying a targeting peptide ubiquicidin labeled with fluorescent dye into mouse models, which detected using the multimodal imaging of tumors (36).
Intrinsic Oncolytic Activity
Similar to viruses, Salmonella kills tumor cells using its intrinsic oncolytic activity (37). Active division of tumor cells and the necrosis inside tumors provide a conducive environment for Salmonellae (25). Salmonella accumulating in tumors deprive extracellular nutrients from tumor cells (38), which promotes apoptosis in tumor cells (39). Salmonella influxes into solid tumors up-regulates the production and release of TNF-α, which leads to tumor hemorrhage and Salmonella invasion, and eventually inhibits tumor angiogenesis (34). The vessel destruction by Salmonella would be augmented by high tumor vascularity (40). Subsequent studies showed that Salmonella can also inhibit tumor angiogenesis through AKT/mTOR pathway by suppressing the levels of HIF-1α and VEGF (41). Besides, this anti-angiogenic capacity can be boosted by Salmonella with engineering or a combination with Triptolid (42). In addition to destroying the microenvironment required for tumor growth, Salmonella can also kill tumor cells by secreting or inducing antitumor agents. As the pathogen-associated molecular patterns (PAMPs) of Salmonella, LPS and flagella have been shown antitumor activities in many studies, whose elimination accounts for a loss of therapeutic potency (43–47). LPS from Salmonella can increase TNF-α and the tumor specific response of CD8+ T cells (45). While flagellin from Salmonella can reduce the amount of regulatory T cells (Treg) in TME (43). When flagellin affects tumors, TLR4 and TLR5 were shown to play important role in this process using mouse models (43, 44). An engineered flagellum of Salmonella has been shown to exert strong antitumor effect (47). Salmonella can also inhibit tumors through producing NO in TME, where nitrate reductase secreted by Salmonella converts nitrates and nitrites into NO (48, 49). Autophagy is a defensive mechanism of host cells against infection and intracellular proliferation of pathogens (50). Blockade of Salmonella-induced autophagy was shown improves cytotoxicity of Salmonella, and increases tumor cells apoptosis (51). Lee et al. showed Salmonella induce melanoma cell autophagy by restraining AKT/mTOR pathway (52). However, this study indicated that autophagy might also a pattern for Salmonella induced cell death. They showed that, 3-Methyladenine, an autophagy inhibitor, suppressed the antitumor effect of Salmonella (52). Previous study also found that SipB, a Salmonella protein with membrane fusion activity, causes macrophage cell death by inducing autophagy (53). Hence, the question whether autophagy contributes to or blocks the cell death induced by Salmonella needs to be confirmed by further research. Another advantage with Salmonella therapy is its preference for metastases. Monotherapy with Salmonella suppressed tumor growth and metastasis in nude mice (24). Further investigations showed that Salmonella downregulated the AKT/mTOR pathway in tumor cells and suppressed the expression of matrix metalloprotease MMP9 (54).
Induction of Inflammation
Salmonella induces proinflammatory responses which are critical for its antitumor effects. Similar to Salmonella, E. coli also accumulates in tumors, but does not induce antitumor effects. The reason might be that E. coli colonization in tumors does not induce a same inflammatory response as Salmonella (55). Chirullo et al. reported that, after Salmonella administration, the number of monocytes/macrophages (F4/80+-Ly6C+) and CD8+ T cells was significantly up-regulated (56) in the spleens of mice. The expression of IFN-γ and IL-1β was also up-regulated in tumors after Salmonella administration (18, 19, 21). Calreticulin, a protein related to immunogenic cell death pathway, is increased in tumor tissues after Salmonella infection (56). Likewise, Lee et al. (18) showed that infiltration of macrophages, neutrophils, and CD8+ T cells in mouse tumors was increased after Salmonella infection. IFN-γ and IFN-inducible chemokines CXCL9 and CXCL10 are also shown to be up-regulated (18). TLR4 is critical for this response, as the antitumor activity was not mounted in Salmonella treated TLR4 defective mice (18). Another study showed that the flagellin of Salmonella inhibits the numbers of CD4+CD25+ Treg cells in TME through TLR5 (43). Besides, B cells have been shown to play an important role in regulating IFN-γ expression and adaptive immunity after Salmonella treatment (19, 57). Tumor cells infected with Salmonella secrete more TNF-α (58). Furthermore, Salmonella also elicits transformation of immunosuppressive myeloid-derived suppressor cells (MDSC) into TNF-α-secreting cells, and reduces the infiltration of Treg cells into tumors (59, 60). Some studies also showed that LPS from Salmonella induces systemic or local production of massive TNF-α in leucocytes which activates recruitment of macrophages and neutrophils (45, 61, 62). Salmonella infection actives the NF-κB pathway in lymphocytes, leading to an increased secretion of proinflammatory factors (63). Multiplex suspension arrays in melanoma models revealed that, Salmonella caused a significant up-regulation of proinflammatory factors, such as IL-6, IL-1α, IL-12p70, IL-17, and IL-13, and chemokines, such as G-CSF, GM-CSF, MIP-1α, MIP-1β and etc. in the TME (42). In addition, Salmonella up-regulated the expression of iNOS in tumors, which is attributed to intratumoral myeloid cells (17). Studies showed that the rise in IFN-γ and iNOS might play an important role in the increase of neutrophils, activated CD8+ T cells and natural killer (NK) cells (17, 18). On the other hand, Salmonella also down-regulated the expression of immunosuppressive factors such as ARG-1, IL-4, TGF-β, and VEGF (17, 64). Salmonella also activates inflammasome formation in myeloid macrophages, which activates the expression of caspase-1, NLRP3 and IPAF, and induces apoptosis of tumor cells (55). This process might include two distinct effects: 1) Salmonella directly stimulates macrophages to secrete proinflammatory factor using its flagellin (65); 2)ATP released from Salmonella-damaged tumor cells stimulates macrophages to release inflammasomes (55).
Effects on Immune Cell Infiltration
Salmonella was shown to facilitate the recruitment of both innate and adaptive cells to the tumor (66). Avogadri et al. (2005). observed a large leukocyte infiltration into necrotic tumor areas with a high Salmonella accumulation. Initially (first 2 days), the recruited immune cells mainly consisted of granulocytes. T lymphocytes (both CD4+ and CD8+) and B lymphocytes (CD19+) were recruited at later following Salmonella injection (67). Other studies also indicate the infiltration of lymphocytes, including CD4/8+ T cells and NK cells to the site of tumor after Salmonella infection (67, 68). Chirullo et al. showed that Salmonella up-regulates calreticulin in TME (56), which may contribute to the Cytotoxic T Lymphocytes (CTLs) infiltration in patients with ovarian and non-small cell cancers (69). Moreover, Clay et al. found that polarization of CD4+ T cells in Salmonella infected site is regulated by Treg cells (70). Salmonella enhances the infiltration of neutrophils into the tumor, which has been demonstrated in many researches (46, 48, 68, 71). Some studies showed the antitumor capacity and tumor antigen-presenting function of neutrophils after the administration of Salmonella (72). However, these neutrophil populations are heterogenous, which may exert either positive or negative effects on tumor growth (73). Chen et al. indicated that Triptolide, diterpenoid triepoxide compound purified from Tripterygium wilfordii, augments antitumor effect of Salmonella by reducing neutrophils infiltration in melanoma (42). Whether the subset of neutrophils recruited by Salmonella would exert pro- or antitumor functions is still worth a further investigation. Besides, administration of Salmonella also recruits macrophages into tumor tissue, which then secrete TNF-α and IL-1β for exerting anticancer effects (17, 46).
Sensitization on Tumor Immune Microenvironment
In addition to inducing inflammation, Salmonella also promotes T cell-dependent immune responses. Because of the antigenic determinants of Salmonella on tumor cell surfaces, it allows to CTLs to kill tumor cells (67). Dendritic Cell (DC) present exogenous antigens to T cells through the cross-presentation pathway. Such presentation of tumor peptides is crucial for anti-tumor T cell development (74). Salmonella not only increases the number of active DCs in mouse lymph nodes but also enhances tumor antigen presentation by DCs (59, 75). Saccheri et al. (2010). demonstrated that bacteria-treated melanoma cells can establish functional gap junctions with adjacent DCs. The antigenic peptides from tumor cells can be transferred to DCs through the gap junctions, which then present these peptides to CTLs against tumor cells. During this process, connexin 43 (Cx43), a ubiquitous protein that forms gap junctions and normally lost in melanoma cells, was up-regulated after Salmonella administration (75). Besides, Salmonella was shown reduce the expression of indoleamine 2, 3-dioxygenase 1 (IDO) via inhibiting AKT signaling pathway, which is related to immune tolerance and T cell apoptosis (76). Although Salmonella targets tumors, most of them live in the necrotic areas. Neutrophils surrounding the necrotic areas actually prevent Salmonella from spreading to non-tumor cells. But when those neutrophils are depleted, Salmonella can then breach that barrier and further suppress tumors (77, 78).
The inhibitory effect of tumor immune microenvironment is an important reason for tumor immune-suppression and the poor efficacy of tumor immunotherapy. One study tested if the expression of immune checkpoint PD-L1 was affected by Salmonella on a variety of cancer cell lines. Data from these experiments demonstrated that Salmonella downregulated PD-L1 expression in a p-AKT, p-mTOR and p-p70S6K involved manner. As a result of reduced apoptosis, the T cell numbers rose (79). Salmonella also affects other immunosuppressive cells (60) in the TME. The down-regulation of IDO inhibits the numbers, activation and censorship of Treg cells on tumor immunity (60, 76, 80, 81). Intratumoral injection of attenuated Salmonella produces antitumor effect by redirecting activated TNF-α secreting neutrophils to the tumor site and reducing Treg cells in the draining lymph nodes of the tumor (82). Liu et al. found that tumor suppression by Salmonella is associated with a down-regulation of CD44high and CD4+CD25+ Treg cells and the LPS and Braun lipoprotein of Salmonella were critical for such response (83). The MDSCs are crucial contributors to tumor progression (84). Study from Kainala et al. (2014). showed Salmonella ablates the immunosuppressive capacity of MDSC and Tumor-associated macrophages (17). Chang et al. found that Salmonella can convert the immune suppressive MDSC into TNF-α-secreting cells with characteristics of neutrophils, which accompanied a loss of Treg cells (60). Finally, Kim et al. reported that Salmonella recruits M1-like macrophages and reduces Treg numbers in the tumor tissues, significantly elevating the proportion of M1/M2 macrophages (80).
Therapeutic Combination
Despite its advantages as biotherapeutic, Salmonella in clinical trials did not show a significant effect as it did in animal experiments (8). The immunomodulatory effects of Salmonella suggest that it may work other therapeutics for achieving optimal tumor inhibition and overcome clinical barriers associated with monotherapies.
Several studies indicated that the combination of Salmonella with chemotherapeutics (such as 5-fluorouracil, cisplatin, cyclophosphamide, doxorubicin and etc.) causes a remarkably stronger tumor suppression (85–88). In a study of B-cell non-Hodgkin lymphoma, mice treated with the Salmonella/CHOP (cyclophosphamide, doxorubicin, vincristine, and prednisone) combination significantly delayed tumor growth and prolonged the animal survival compared to monotherapy. Salmonella also improved the side effects caused by chemotherapeutics (86). A similar conclusion was arrived from a study that used the combination of Salmonella and doxorubicin against breast cancer (85). Jia et al. (89) demonstrated that Salmonella strain VNP20009 improves the maximum tolerated dose and low-dose metronomic regimens of cyclophosphamide in a murine melanoma model. The VNP20009/cyclophosphamide combination reduced the circulating levels of vascular endothelial growth factor and tumor micro-vessel density compared to the monotherapy. Interestingly, tumor accumulation of Salmonella is also increased with the combination therapy than of Salmonella alone (89). Likewise, Triptolide and VNP20009 combination also strongly inhibited tumor angiogenesis and increased host immune response, which accompanied a decreased VEGF and neutrophils infiltration (42). Din et al. engineered Salmonella to lyse synchronously at a threshold population density and release encoded drugs, which exerted a better antitumor effect when combined with 5-fluorouracil (87). Salmonella also exerts better antitumor effects when combined with other drugs such as caffeine, valproic acid, gemcitabine, Chinese medicine herbal mixture and (90–94). As to the mechanisms, current studies suggest several possibilities. Salmonella could enhance gap junctional intercellular communication (GJIC) by increasing the expression of Cx43 (75, 95, 96), for promoting antigen presentation by DCs (75). Chang et al. (2013). suggest Salmonella enhanced the chemosensitivity of tumors to cisplatin, in which Cx43 was knocked down (95). A study with gemcitabine indicates that Salmonella strain A1-R pushes cancer cells from a chemo-resistant G0/G1 phase to the chemo-sensitive S phase (97). The same mechanism also appears to operate when cisplatin and paclitaxel with strain A1-R are combined (98, 99). Salmonella could also decrease the expression of P-glycoprotein (P-gp), a plasma membrane multidrug resistance protein, and its drug-efflux capabilities. This activity enhances the antitumor effects of 5-fluorouracil (96). P-gp down-regulation caused a decline of p-AKT, p-mTOR and p-p70s6K levels in tumors. The P-gp loss caused by Salmonella was rescued by recovering p-AKT, highlighting the important role of AKT/mTOR pathway (41, 52, 54, 96). Another study from Mercado-Lubo et al. (2016) indicated that P-gp loss by Salmonella is dependent its type III secretion effector, SipA. This process appears to require caspase-3 (100).
In addition to chemotherapy, Salmonella also enhances the antitumor effects of other therapeutics. Similar to Bifidobacterium and engineered Escherichia (101, 102), Salmonella synergistically augments radiation induced tumor growth inhibition (103–105). Murakami et al. (2015). used Salmonella strain A1-R as an adjuvant treatment after resection of metastases. This improved disease-free survival and lowered tumor burden in mice (106). Studies on the combination with nanomaterials further exert the tumor targeting advantages of Salmonella (107, 108). As a vehicle, Salmonella loads gold nanoparticles to the central tumor hypoxic regions, a restricted area for gold nanoparticles, and eventually enhances efficacy of radiation therapy (107). Another study combining with photothermal therapy also produced a promising result. Chen et al. (108). coated Salmonella strain VNP20009 with polydopamine via oxidation and self-polymerization. After systematic injection of coated Salmonella, near-infrared laser irradiation induced heating of polydopamine successfully restrained tumors in the targeted hypoxic regions (108). With the potential benefits of tumor immunotherapy, combining Salmonella with adoptive T cell therapy may yield a better antitumor effect, in which Salmonella sensitizes tumors to adoptive T cells therapy prevents tumor relapse and eventually eradicates tumors (109). Binder et al. reported that combining Salmonella expressing tumor-specific antigen ovalbumin with anti-PD-L1 antibody rescues antitumor function of endogenous PD-1+CD8+ T cells against long-established melanoma in mice (110).
Clinical Trials
Since the first report in 1893 that patients infected by bacteria shows hemorrhagic necrosis of solid tumors (15, 16), many bacteria capable of colonizing and restraining tumors have been described (12). Salmonella was first shown to have a tumor therapeutic effect in animal models in 1935 (4). Until now several clinical studies on Salmonella have been reported (Table 1). As mentioned above, LPS and TNF-α are important for the therapeutic efficiency of Salmonella against tumors (45). Clinical trials with LPS from Salmonella in cancer patients were carried out since 1991 (111, 112). Engelhardt et al. (1991) intravenously administered escalating doses (from 0.15 ng/kg to 5.0 ng/kg) of purified-LPS from Salmonella abortus equi to cancer patients at 2-week intervals, and observed a strong rise in TNF-α, IL-6 and granulocyte-colony-stimulating factor (G-CSF) levels in serum. Two patients with colorectal cancer showed moderate antitumor activity in this trial (111). A Phase II trial of LPS was aimed at treating patients of colorectal and non-small cell lung cancer. Although LPS showed limited antitumor effects in non-small cell lung cancer, 3 out of 27 patients of colorectal cancer was had objective responses. Two patients with partial remissions were maintained for 7 and 8 months. While One patient with complete tumor remission was maintained for more than 3 years (112). However, the poor tumor response and toxicity limited the further application of purified LPS against tumors. Goto et al. (1996) reported that intradermal administration of LPS improves biological response (113). They showed higher tolerable doses of LPS produced more continuous induction of cytokines than previous intravenous administration. By combining LPS with cyclophosphamide, three out of five patients (ovarian cancer, cervical cancer and brain tumor) showed a significant response (113). A phase I trial using VNP20009 as therapeutic against tumors was first reported in 2002, which showed the safety of intravenous bolus infusions containing 106 to 109 CFU. Despite the high tolerance and colonization in some tumors, VNP20009 didn’t show objective response in patients against metastatic melanoma (114). Another clinical study using VNP20009 to treat multiple tumors in dogs was also carried out, in which 35 out of 41 animals were evaluable for antitumor response (116). A pilot trial further supported tumor colonization and the principle that Salmonella can be used as a delivery vehicle expressing exogenous growth inhibitory gene products into tumors. In this study, TAPET-CD, an attenuated Salmonella expressing the E. coli cytosine deaminase, successfully expressed the gene in 2 of 3 patients after intratumoral injection (115). Later trials used oral route for administering Salmonella. The attenuated Salmonella strain VXM01 was used in a phase I trial against advanced pancreatic cancer (120). VXM01 carries an VERFR-2 expressing plasmid, which aroused VEGFR2 specific T effector response and significant reduction of tumor perfusion, indicating anti-angiogenic activity in patients. These researchers further evaluated the safety of Salmonella in monthly boosting experiments and found a high VERFR-2 specific T cell response. Although patients received VXM01 showed adverse events such as lymphocyte reduction in blood, neutrophils increase and diarrhea, compared with placebo treatment, VXM01 demonstrated safety and efficiency on arousing VERFR-2 specific T cell responses (118). SalpIL2, a Salmonella strain carrying IL-2 expressing plasmid, was reported in a phase I clinical study with amputation and adjuvant doxorubicin for canine appendicular osteosarcoma. The disease-free interval (DFI) of dogs administrated with SalpIL2 was significantly prolonged than doxorubicin alone. Interestingly, the lower dose group showed longer DFI than group treated with highest SalpIL2 (117). However, another phase I trial using IL-2 expressing Salmonella strain, Saltikva, for metastatic gastrointestinal cancer patients showed no significant benefits. Saltikva increased circulating NK cells and NK-T cells, which promises the possibility for a multiple therapeutic strategy using Salmonella and other tumor immunotherapeutics (119). Above all, clinical trials of Salmonella strains against tumors are still in progress (121), which are likely provide a new generation of antitumor drugs through further modifications.
Cancer Immunotherapy and Adverse Effects
Human immune system can recognize and eliminate foreign substances through innate and adaptive immunity to ensure the normal physiological function. Tumor cells have developed their specificity in many ways compared to normal cells, including biochemical composition, antigenic structures, and biologic behaviors (122, 123). These properties make it possible for immune system to recognize and eliminate tumor cells. However, the heterogeneity of tumors poses new challenges, such as differences between tumors of different patients, and in tumors of the same patient, and their individual surrounding environments (124). In addition, considering that tumor cells come from the body itself, they can use or enhance the mechanisms of the body’s autoimmune tolerance to evade the immune system in many ways, thus evading growth arrest. In the battle between host immune system and tumor cells, normal and strong autoimmune system can remove abnormal cells in time to ensure body’s normal physiological function. But, when tumor cells gained the ability to escape, host immune system cannot clear them. Therefore, tumor immunotherapy aimed at enhancing body’s antitumor immunity or reducing the immune evasion of tumor cells, will enable the host to tilt such imbalance (125).
Development of Cancer Immunotherapy
The advent of smallpox vaccine in 1796 paved the way for subsequent immunogenic interventions (126). Dr William Coley, known as the “father of immunotherapy”, used “Coley’s toxins,” a mixture of live and inactivated Streptococcus Pyogenes and Serratia Marcescens to treat tumor patients, and found that this kind of toxins can be used to cope with various malignancies (15). Furthermore, a substantial relationship between tumorigenesis and immune system is revealed by the spontaneous regression of some patients with malignant tumor, and by the higher risk of cancer incidence in immunosuppressed populations (122). However, due to the limitation of therapeutic effect and poor understanding of therapeutic mechanism, as well as the development of chemo-, and radio-, therapies and surgery, immune therapeutic strategies to block tumors did not attract much attention in the following decades. After a long time, the effectiveness of tumor immunotherapy was established through the use of BCG against superficial bladder cancer (5). BCG can indirectly increase the expression of tumor antigens in tumor cells and extensively induce the expression of a large number of cytokines, thus enhancing the antitumor activity mediated by cytotoxic T lymphocytes, NK cells, neutrophils and macrophages (127). Besides, the possibility of TNF as an immunotherapy for tumors was suggested (128). Unfortunately, systematic treatment of TNF leads to severe toxicity, making it less likely to be used as a cancer therapeutic (129). Similarly, the USFDA approved IFN-α for treatment for hairy cell leukemia and IL-2 for metastatic kidney cancer and metastatic melanoma in 1986 and 1992 respectively, which have been shown to enhance the production of T cells in patients. However, subsequent clinical applications found the short duration of IFN-α in patients, and IL-2 could cause emergent therapeutic toxicity, which led to their gradual withdrawal from tumor therapy (11, 122). Sipuleucel-T (Provenge) is the first therapeutic autologous vaccine approved by the USFDA in 2010, which is used to treat symptomatic castration-resistant metastatic prostate cancer (122). The patient’s own DC cells were incubated in vitro with PA2024, a fusion protein constructed by prostatic acid phosphatase (PAP) and granulocyte macrophage colony stimulating factor (GM-CSF), and then re-infused back. Although Sipuleucel-T failed to prevent tumor progression, it extended survival time by an average of 4 months (130). Unlike modification of DC cells by Sipuleucel-T, Adoptive T cell therapy (including TIL, TCR-T and CAR-T) involves the isolation, modification and amplification of tumor-specific T cells in vitro, which are then infused back into patients to fight tumor cells, which has shown promising results in clinical trials for both hematologic and solid tumors (131). Among them, CAR-T was approved by the USFDA for relapsed and refractory B-cell acute lymphoblastic leukemia in pediatric and young adult patients (122). However, the death risk from cytokine storms and brain edema associated with treatment cannot be ignored at the same time (122). In addition, although adoptive cell therapy can achieve objective therapeutic effects, financial cost is another factor that needs to be controlled in the process of practical clinical promotion. For example, Sipuleucel-T requires about US $ 93,000 per one treatment period from collecting patients’ DC cells, transferring cells to laboratory for cultivation and modification in vitro, to reinfusing cells to patients. Its expensiveness undoubtedly limits the benefits of this kind of strategy for a large number of patients (132). In addition to adoptive cell therapy, as another pillar of today’s tumor immunotherapy, the development of immune checkpoint blockade opened a new door for tumor treatment. Persistent inflammation damages a body. To control the negative effects of excessive immune responses, many mechanisms limit them and control the degree of inflammation. The immune checkpoint is one such important feedback regulatory mechanism. Through a blockade of immune checkpoint protein, this strategy inhibits intrinsic down-regulation of immunity and mainly sustains the immune activity of T cells against tumor cells (133). In 2011, the first immune checkpoint drug anti-CTLA4 was approved by the USFDA for the treatment of metastatic melanoma (134). Subsequently, monoclonal antibodies capable of blocking PD-1 and PD-L1 have been successively developed for many types of cancers (133) (Table 2). In addition, drugs targeting other immune checkpoints are being continuously researched and developed (135). Oncolytic virus therapy is another promising approach for cancer immunotherapy. The understanding of viral therapy for tumors first emerged in the early 20th century, where tumor recession was noticed in patients who acquired viral diseases. The treatment of cervical cancer with rabies virus also suggests the possibility oncolytic viruses as a therapeutic (136). Viruses used for such therapies have been genetically attenuated to prevent their virulence to normal cells, while remaining tumor targeting and lysing capacities. These oncolytic viruses can carry antitumor agents into TME, which further enhances effectiveness (137). In 2015, the USFDA approved the first oncolytic virus antitumor drug, Talimogene Laherparepvec for melanoma (137). Currently, other clinical trials are being carried out for targeting a variety of different tumors, which are likely to provide more avenues for the expansion of tumor immunotherapy.
Following Adverse Events
In the scope of cancer immunotherapy, immune checkpoint blockade can effectively activate tumor-specific T cells and provokes the development of antitumor microenvironment. Immune checkpoint, CTLA-4 and its ligands B7-1 and B7-2 are mainly concentrated in human and mouse secondary lymphocyte organs, while PD-1 is more widely expressed in non-lymphocyte T cells, and its ligand PD-L1 is also more widely expressed in peripheral inflammatory tissue sites, making it a key factor in regulating peripheral effecter T cell responses (138, 139). Accordingly, PD-1 plays a critical role in regulating CD8+T cell exhaustion than CTLA-4, which is better at inactivating and initiating T cells (140–142). In addition, CTLA-4 and PD-1 are also widely found in B cells, NK cells, DC cells, monocytes, granulocytes, NKT cells and other immune-related cells (138, 139). Therefore, the effects of immune checkpoints on immune system are extensive, which is bound to involve a number of complex mechanisms requiring further research in the future. The immune system recognizes not only exogenous pathogens, but also some antigens in normal tissue cells. Therefore, immune tolerance, as a protective measure, prevents the pathogenic autoimmune responses caused by the excessive immune to native tissues, symbiotic organisms and harmless environmental antigens. These responses include allergic diseases (such as urticaria, bronchial asthma and anaphylactic shock) and other autoimmune diseases might damage host (143). However, immune checkpoint blockade might increase the risk of off-tumor inflammatory responses and disturb the immune tolerance regulation in patients (143). Clinical studies have shown that some patients who received immune checkpoint blockade, developed immune-related adverse events (irAEs). In some cases, it was life-threatening (144). The irAEs caused by immune checkpoint blockade are varied, such as rash, colitis, hepatotoxicity, pneumonia, and pituitary inflammation. More seriously, irAEs could cause permanent damage to some organs, such as insulin and adrenal corticosteroid deficiency (145–148). In addition, block different immune checkpoint are associated with different types of irAEs. For example, patients who received CTLA-4 blockade were more likely to experience pituitary inflammation and colitis, while those treated with PD-1 antibodies were more likely to develop thyroid dysfunction (147). Current statistics show that PD-L1 blockade has the lowest probability (12-24%), while CTLA-4 blockade has the highest probability (60-85%) of producing irAEs. Combined blockade of both PD-1 and CTLA-4 resulted in more serious adverse reactions (143). Patients with preexisting autoimmune diseases are often excluded from checkpoint blockade therapy. However, clinical analyses show that not all preexisting autoimmune diseases patients had an aggravating autoimmune disease after immune checkpoint blockade (149, 150). In a study of Ipilimumab in cancer patients with different preexisting autoimmune diseases (6 with rheumatoid arthritis, 5 with psoriasis, 6 with inflammatory bowel disease, 2 with systemic lupus erythematosus, 2 with multiple sclerosis, 2 with autoimmune thyroiditis, and 7 with other conditions), only eight from them developed worsening autoimmune diseases, while cortisol intervention effectively inhibited such adverse reactions (149). Similarly, another retrospective study exploring the impact of a PD-1 inhibitor on preexisting autoimmune disease patients showed that only 52 of 119 patients had autoimmune disease progression. Patients also experienced relative relief of adverse reactions after receiving the intervention of corticosteroids and steroid-sparing agents (150). It is not yet understood under what circumstances the incidence of irAEs will increase, which autoimmune diseases are more likely to be induced by immune checkpoint inhibitors, and whether different autoimmune diseases require different defenses to induce them. With the widespread use of checkpoint blockade drugs, we are likely to see more unforeseen adverse conditions in preclinical studies. Therefore, how to determine the maximum dose to balance the negative effects from adverse events in patients, and how to reduce the adverse events caused by immune checkpoint blockade is an important direction for the development of tumor immunotherapy in the future.
Currently, the possible mechanisms of irAEs include the following aspects: 1. enhance autoreactive T and B cells, or inhibit the activity of Treg cells to induce the preexisting subclinical autoimmune condition; 2. Directly trigger new autoimmunity or inflammation; 3. Disturb the balance of gastrointestinal symbiotic bacteria and causes gastrointestinal disruption; 4. Damage normal tissues around tumor tissues, or directly affect tissues with high expression of immune checkpoint, such as hypophysis (143). Therefore, one of the key issues that needs to be addressed in immunosuppressive therapy is how to isolate irAEs-related adverse inflammation from tumor immunity enhanced by immune checkpoint blockade, which might be influenced by drug toxicity, tumor types, and different type of irAEs diseases (143). Treatment with CAR-T therapy also resulted in irAEs in some cancer patients. The combination anti-IL-6 receptor can effectively inhibit it during the CAR-T therapy (151).
However, unlike immune checkpoint blockade, the irAEs induced by CAR-T were mainly attributed to the IL-6-induced cytokine storm. In contrast, possible mechanisms of irAEs discussed above, and the causes of irAEs caused by immune checkpoint blockade are more extensive.
Integration of Salmonella Into Combination Immunotherapy
Cancer immunotherapy has made remarkable achievements and become another important antitumor strategy after chemoradiotherapy and surgery. However, similar to other therapies, in addition to the excellent antitumor effects, many side effects and limitations have been observed in some patients who received immunotherapies. For example, some patients with cancer immunotherapy were found to develop autoimmune reactions, cytokine release syndrome and vascular leak syndrome. Some severe cases are even fatal for patients (11). Patients with preexisting autoimmune disorders are often excluded from the treatment for immune checkpoint blockade (149). A rational integration of other approaches is expected to enhance the antitumor therapeutic effects and reduce immune-related side effects caused by cancer immunotherapy. In recent years, some studies showed that, in addition to their own antitumor toxicity, oncolytic virus can also serve as engineering platforms for immunotherapy (152). Engineered oncolytic virus were shown selectively replicate, destroy tumor tissues and enhance host antitumor immunity (137). The USFDA has approved an oncolytic antitumor drug “Talimogene laherparepvec (Imlygic)” in 2015, which is derived from herpes simplex virus type 1 and indicated for patients with melanoma recurrent after initial surgery (11). Similarly, the antitumor effects of bacteria were also being explored widely (12). For example, Salmonella has been used as promising antitumor agent and deliver cytotoxic agents for its ability in tumors targeting, cytotoxicity, reversal of immune tolerance (8). Therefore, a combination of Salmonella with other immunotherapeutic could further exert its anticancer effects and reduce the therapeutic resistance and side effects during current cancer immunotherapy (Table 3).
Delivery for Antitumor Immunomodulators
With the development of drug delivery systems and higher targeting requirement for antitumor drugs, an increasing number of studies are employing nanoparticles, scaffolds and hydrogels to improve the efficacy and safety of cancer immunotherapy (11). Because of tumor targeting, intrinsic oncolytic and host immune response augmenting abilities, Salmonella has been widely studied as a drug carrier for oncotherapy. Gene-editing techniques enable tumor-targeting Salmonella to interfere genes expression in tumor cells or macrophages, further suppressing the tumor growth. It also enables Salmonella to directly express (or secrete) tumor antigens, cytokines, antibodies and etc., which affects TME and the antitumor ability of host immune system (8).
Many genes that promote tumor development have been found. Mutations in some of them are directly associated with tumorigenesis. Engineered Salmonella, as a kind of potential tumor gene therapy platform, could carry target genes into patients’ tumor cells, affecting the expression of cancer-related genes. For example, TP53 is a widely mutated tumor suppressor in cancers, which prevents an elimination of damaged cells. Loss of the p53 protein is one of the reasons for resistance to chemotherapeutics (153). Salmonella carrying a eukaryotic expression wild-type p53 plasmid to colonize tumor sites restored its expression of in tumor cells effectively in inhibited tumor growth and regained the sensitivity to chemotherapeutics (154, 155). In addition, Salmonella carrying eukaryotic expression plasmids that express shRNAs which target oncogenic products like STAT3, Survivin, Bcl-2, and HIF-1 strongly suppressed tumor growth in xenograft models (156–159). The use of Salmonella to overexpress tumor antigens in cancer cells, such as Legumain, PCSA, AFP, etc., has also been shown to inhibit tumor growth effectively (160–162). Similarly, genetically modified Salmonella successfully induced tumor cells to secrete immune-related cytokines, such as IL-12, IL-4, and IL-18. These cytokines modify the host immune responses and inhibit tumor growth (163, 164). For Salmonella caring prokaryotic expression system, direct expression or secretion of target proteins might be more effective for influencing the TME. Studies on osteosarcoma treated with an IL-2 secreting Salmonella have shown the tumor repression effect in mice model (165). A pre-clinical study of Canine osteosarcoma treated with Salmonella expressing IL-2 has also shown a prolongation of disease-free interval (117). IL-2 secreting Salmonella increased circulating NK cells and NK-T cells in patients with metastatic gastrointestinal cancer (119). In addition to IL-2 (approved for metastatic kidney cancer and metastatic melanoma in 1992 and 1998), IFN-α has been approved by the USFDA for the treatment with hairy cell leukemia (1986) (11), and TNF (tasonermin) was licensed in Europe for irresectable soft tissue sarcoma in the 1999. However, due to the short effectiveness and serious adverse reactions, they are not used in clinical treatment nowadays (11, 122). Studies using IFN-γ or TNF-α secreting Salmonella have found significant inhibitory effects on the growth of melanoma in mice, demonstrating the possibility for further use of this type of therapy (166, 167). Flagellin, also exhibited strong antitumor effects (43, 44). Salmonella secreting flagellin B inhibits tumor growth by regulating TME via TLR5 and shifts macrophages from M2-like suppressive activities to M1 phenotypes in the microenvironment. Besides, flagellin diminished the numbers of Treg cells in the TME (46, 47). Binder et al. (2016). constructed an antigen (SIINF) producing Salmonella, which satisfied the high-antigen expression requirement of adoptively transferred T cells and prevented tumor relapse of a fibrosarcoma which has low antigen expression (109). In addition, genetic programming may further enhance the delivery ability of Salmonella. Sreyan et al. (2019) engineered a quorum-sensing E. coli to specifically lyse upon tumor accumulation (168). Based on the tumor-targeting ability, quorum sensing lysis was a promising strategy to enhance Salmonella releasing anti-tumor agents.
Combination With Immune Checkpoint Blockade
The complete activation of T cells requires two different signals. One is the contact of antigens and major histocompatibility complex (MHC), respectively on the cell surface of antigen presenting cells (APCs) with the T cell receptor, which produce antigen specific T-cells. The others are antigen independent co-signaling molecules, including co-stimulators and co-inhibitors, which are also known as immune checkpoint (134). The presence of immune checkpoint is conducive to controlling and regulating the persistence of the immune response and preventing the pathogenic autoimmune responses (143). The blockade of immune checkpoint permits the killing of tumor cells by unleashing killer T cells. Since the first CTLA-4 block drug, Ipilimumab, was approved for metastatic melanoma in 2011 (134), immune checkpoint blockade has shown impressive durable responses and therapeutic effects, elevating the development of cancer immunotherapy to a new level. However, as described in the above, the irAEs generated due to immune checkpoint blockade pose a potential hazard for the long-term clinical use of this therapy. The fact that only some patients benefit from such treatment adds to the barriers to its wider use (169). In terms of blocking PD-1/PD-L1, the infiltration of T cells into tumors and the expression of PD-L1 will largely affect the therapeutic effect of immune checkpoint inhibitors. However, clinical studies also found that some PD-L1 positive patients had poor feedback on immune checkpoint blockade, while others patients with negative PD-L1 were also able to respond (169), which provides a new requirement for further understanding the role of immune checkpoint in tumor immunotherapy to improve this strategy.
PD-L1 expression in tumor tissues can be induced by IFN-γ, secreted by tumor-specific T cells after recognizing tumor cells. The up-regulation of PD-L1 is not only limited to tumor cells in the TME (170–172). This physiological induction of PD-L1 leads to the escape of tumor cells from T cell response and immune tolerance (169). Theoretically, for patients with high PD-L1 expression, blocking PD-1/PD-L1 should be able to eliminate the immune tolerance and achieve the therapeutic purpose. However, clinical data analyses showed that some patients lacked T cell infiltration could still not be effectively treated, although the expression of PD-L1 has been down-regulated (169). In fact, most patients with no response to PD-1/PD-L1 blockade show little T cell infiltration and negative PD-L1 expression (170, 172). However, clinical trials in melanoma have shown that the combination of Nivolumab (αPD-1) and Ipilimumab (αCTLA-4) can restore the therapeutic effects of Nivolumab and extend progression-free survival in patients with negative PD-L1, compared with those treated with Nivolumab alone (173, 174). The enhanced effect of PD-1/PD-L1 blockade is attributed to CTLA-4 inhibition by Ipilimumab, which lead to a marked diversification of the peripheral TCR repertoire and increased infiltration of T cells into tumor tissue (174). Interestingly, T cell infiltration increased by anti-CTLA-4, could also cause PD-L1 up-regulation through IFN-γ induction (169). The effect of Nivolumab in the combined treatment group may offset the negative effect of Ipilimumab on inducing PD-L1 after increasing T cell tumor invasion.
Currently, the success of Salmonella in animal studies has not been fully replicated in clinical studies (8). The reasons for such weaker response are manifold, such as the biological differences between human and animals, optimization of bacterial administration (routes, numbers, genetic modifications) and etc. Among them, the relationship between Salmonella and immune checkpoint has not received sufficient attention. Chen et al. found the down-regulation of PD-L1 expression in a variety of mouse and human tumor cells treated with Salmonella, affects apoptosis of co-cultured PD-1-expression cells (79). However, PD-L1 is also expressed in other non-tumor cells in TME, such as stromal cells, myeloid-derived cells and infiltrating immune cells (169, 175). Among them, a study on colon cancer found that PD-L1 expressed by mesenchymal stromal cells could decrease T cells proliferation, activation, and promote tumor growth (175). Notably, in addition to tumor cells, many studies have found that Salmonella induces the expression of PD-L1 in different cell types. Salmonella induces PD-L1 in B cells, which might use this mechanism to evade cytotoxic effector response and enable B cells as a reservoir for the bacteria (176). Further studies showed that the up-regulated PD-L1 in B cells (induced by Salmonella) impaired T cell response (177). Similarly, Salmonella also induces the expression of PD-L1 in DCs and CD4+ T cells (178, 179). The PD-L1 up-regulation is associated with the Pathogenicity Island2 of Salmonella (180). The above confounding observations raise, whether the PD-1/PD-L1 pathway positive or negative role in Salmonella mediated tumor therapy. Recently, many studies on the combination of Salmonella and immune checkpoint blockade were reported (110, 181–183). Binder et al. (2013) constructed an antigen-producing Salmonella against long-established immunogenic melanomas. It is worth noting that, 80% tumor rejection and expansion of tumor-specific CD8+ T cells were observed after the treatment combining antigen (ovalbumin) producing Salmonella with anti-PD-L1 antibody (110). Besides, in two other studies focusing on Salmonella carrying PD-1 siRNA, Zhao et al. indicated a promising antitumor strategy that integrating immune checkpoint blockade into Salmonella (182, 183). However, a study on combining anti-PD-1 antibody with Salmonella carrying IDO siRNA against colorectal tumors showed no additional tumor growth inhibition than group of Salmonella alone (181), suggesting that the synergistic antitumor effect of Salmonella and immune checkpoint inhibiting needs to be further explored.
Conclusions and Future Perspectives
In recent years, the success of immune checkpoint blockade and CAR-T have established immunotherapy as a major cancer treatment (10). In addition, BCG, oncolytic virus and other immune-related remedies are approved for the treatment of certain tumors (5, 137, 152). The anti-tumor ability of cancer immunotherapy involves many factors, including the expression of tumor antigens, infiltration of effective immune cells into TME, levels of inflammatory factors and immunosuppression signals (125, 126, 132, 135). Current clinical reports also indicate the limitations of single agent immunotherapy and the more effective combinations are needed for augmenting immunotherapies (140, 141). Besides, the non-tumor-related immune effects of cancer immunotherapy also lead to a large number of adverse reactions, which puts forward higher requirements for the further development and application of cancer immunotherapy (143). The rapid development of Salmonella has demonstrated its potential as a novel immunotherapy for cancer. Although reported clinical trials have not yielded exciting results (8), the proven tumor targeting, immune regulating ability and engineering plasticity indicate that Salmonella still has great potential for further development. For example: 1. Salmonella can colonize and grow in tumors. If we take advantage of this and combine with other therapeutics may help reduce the frequency of treatment and prolong the effectiveness of a single treatment; 2. Although the elimination of its toxicity significantly weakens the intrinsic oncolytic activity of Salmonella, the use of biochemical techniques to restore or even enhance oncolytic activity may be a solution to the poor clinical efficacy of Salmonella; 3. Salmonella has been shown to enhance the sensitivity of tumors to chemotherapy in several ways. Future research should focus on adjuvant and combination therapies; 4. The development of tracer method of Salmonella is expected to provide an in vivo detection of tumor sites and metastasis; 5. Integrating the tumor targeting of Salmonella is expected to enhance the tumor specificity of PD-1/PD-L1 blockade, and reduce non-tumor related immune regulation. The enhancement of tumor specificity is expected to minimize a series of clinical adverse effects caused by immune checkpoint blockade; 6. The induction of immune cell infiltration by Salmonella in TME may improve the poor response to cancer immunotherapy in some patients with low immune cell infiltration. It may also convert immunosuppressive signals in TME of some patients; 7. The plasticity of Salmonella as attenuated engineered bacteria make it promising tool for multiple anti-tumor strategies simultaneously. For example, the advantages of combining PD-1 and CTLA-4 antibody may be achieved through engineered-Salmonella secreting both of them simultaneously.
Although the cancer treatment of Salmonella holds great promise, we cannot ignore the problems that need to be solved in the future therapies. These include comprehensive consideration of clinical safety, optimization of dose and interval of administration, timely elimination of bacteria after administration, concerns about antibiotic use, and quality assurance of mass production of live bacteria etc (8). However, more research of Salmonella is needed to further explore the modification and treatment strategies. In the context of clinical limitations and side effects of current cancer immunotherapy, the tumor-homing ability and influence on host immune system raises the possibility for the intervention with Salmonella. It is expected that bacterial treatment against cancer will likely flourish in the near future, expanding the frontiers of cancer immunotherapy.
Author Contributions
DW and XW designed and wrote this manuscript. DK, BG, and LZ provided critical comments, concepts, and insights. All authors contributed to the article and approved the submitted version.
Funding
LZ is supported by the National Natural Science Foundation of China (grant numbers 81773217), Research Fund of Jilin Provincial Science and Technology Department (grant numbers 20200404120YY and 20190701065GH), Jilin Province Health Technology Innovation Project (grant numbers 2019J030), the Fundamental Research Funds for the Central Universities, JLU and Chunhui international research project of Ministry of Education. DK is supported by the Cigarette Restitution Fund of the University of Maryland Greenebaum Cancer Center.
Conflict of Interest
The authors declare that the research was conducted in the absence of any commercial or financial relationships that could be construed as a potential conflict of interest.
Abbreviations
CAR-T, chimeric antigen receptor T cell therapies; BCG, Bacillus Calmette Guerin; TME, tumor microenvironment; TNF, tumor necrosis factor; HIF, hypoxia inducible factor; VEGF, vascular endothelial growth factor; VEGFR, VEGF receptor; PAMPs, pathogen-associated molecular patterns; LPS, lipopolysaccharide; Treg, regulatory T cells; TLR, Toll-like receptor; NO, nitric oxide; iNOS, inducible NO synthase; MMP, matrix metalloproteinase; IFN, interferon; IL, interleukin; CXCL, chemokine ligand; MDSC, myeloid-derived suppressor cell; CSF, colony stimulating factor; MIP, macrophage inflammatory protein; NK, natural killer; ARG, arginase; TGF, transforming growth factor; NLRP3, NLR family pyrin domain containing 3; IPAF, interstitial pneumonia with autoimmune features; ATP, adenosine triphosphate; CTLs, cytotoxic T lymphocytes; DC, dendritic cell; Cx43, connexin 43; IDO, indoleamine 2, 3-dioxygenase; GJIC, gap junctional intercellular communication; DFI, Disease-free interval; PAP, prostatic acid phosphatase; TIL, tumor infiltrating lymphocyte; TCR, T cell receptor; TCR-T, TCR T cell therapies; irAEs, immune-related adverse events; MHC, major histocompatibility complex; APC, antigen presenting cell.
References
1. Guo Y, Chen Y, Liu X, Min JJ, Tan W, Zheng JH. Targeted cancer immunotherapy with genetically engineered oncolytic Salmonella typhimurium. Cancer Lett (2020) 469:102–10. doi: 10.1016/j.canlet.2019.10.033
2. Kramer MG, Masner M, Ferreira FA, Hoffman RM. Bacterial Therapy of Cancer: Promises, Limitations, and Insights for Future Directions. Front Microbiol (2018) 9:16. doi: 10.3389/fmicb.2018.00016
3. Chorobik P, Czaplicki D, Ossysek K, Bereta J. Salmonella and cancer: from pathogens to therapeutics. Acta Biochim Pol (2013) 60(3):285–97. doi: 10.18388/abp.2013_1984
4. Shwartzman G. Hemorrhagic Necrosis and Regression Sarcoma 180. Science (1935) 82(2122):201. doi: 10.1126/science.82.2122.201
5. Old LJ, Clarke DA, Benacerraf B. Effect of Bacillus Calmette-Guerin infection on transplanted tumours in the mouse. Nature (1959) 184(Suppl 5):291–2. doi: 10.1038/184291a0
6. Sieow BF, Wun KS, Yong WP, Hwang IY, Chang MW. Tweak to Treat: Reprograming Bacteria for CancerTreatment. Trends Cancer (2020) S2405-8033(20)30303-4. doi: 10.1016/j.trecan.2020.11.004
7. Felgner S, Kocijancic D, Frahm M, Weiss S. Bacteria in Cancer Therapy: Renaissance of an Old Concept. Int J Microbiol (2016) 2016:8451728. doi: 10.1155/2016/8451728
8. Liang K, Liu Q, Li P, Luo H, Wang H, Kong Q. Genetically engineered Salmonella Typhimurium: Recent advances in cancer therapy. Cancer Lett (2019) 448:168–81. doi: 10.1016/j.canlet.2019.01.037
9. Kaimala S, Al-Sbiei A, Cabral-Marques O, Fernandez-Cabezudo MJ, Al-Ramadi BK. Attenuated Bacteria as Immunotherapeutic Tools for Cancer Treatment. Front Oncol (2018) 8:136. doi: 10.3389/fonc.2018.00136
10. Yang Y. Cancer immunotherapy: harnessing the immune system to battle cancer. J Clin Invest (2015) 125(9):3335–7. doi: 10.1172/JCI83871
11. Zhao Z, Zheng L, Chen W, Weng W, Song J, Ji J. Delivery strategies of cancer immunotherapy: recent advances and future perspectives. J Hematol Oncol (2019) 12(1):126. doi: 10.1186/s13045-019-0817-3
12. Nallar SC, Xu DQ, Kalvakolanu DV. Bacteria and genetically modified bacteria as cancer therapeutics: Current advances and challenges. Cytokine (2017) 89:160–72. doi: 10.1016/j.cyto.2016.01.002
13. Dang LH, Bettegowda C, Huso DL, Kinzler KW, Vogelstein B. Combination bacteriolytic therapy for the treatment of experimental tumors. Proc Natl Acad Sci USA (2001) 98(26):15155–60. doi: 10.1073/pnas.251543698
14. Forbes NS. Engineering the perfect (bacterial) cancer therapy. Nat Rev Cancer (2010) 10(11):785–94. doi: 10.1038/nrc2934
15. Coley WB. The Treatment of Inoperable Sarcoma by Bacterial Toxins (the Mixed Toxins of the Streptococcus erysipelas and the Bacillus prodigiosus). Proc R Soc Med (1910) 3(Surg Sect):1–48. doi: 10.1177/003591571000301601
16. Nauts HC, Swift WE, Coley BL. The treatment of malignant tumors by bacterial toxins as developed by the late William B. Coley, M.D., reviewed in the light of modern research. Res Cancer Res (1946) 6:205–16.
17. Kaimala S, Mohamed YA, Nader N, Issac J, Elkord E, Chouaib S, et al. Salmonella-mediated tumor regression involves targeting of tumor myeloid suppressor cells causing a shift to M1-like phenotype and reduction in suppressive capacity. Cancer Immunol Immunother (2014) 63(6):587–99. doi: 10.1007/s00262-014-1543-x
18. Lee CH, Wu CL, Shiau AL. Toll-like receptor 4 mediates an antitumor host response induced by Salmonella choleraesuis. Clin Cancer Res (2008) 14(6):1905–12. doi: 10.1158/1078-0432.CCR-07-2050
19. Lee CH, Hsieh JL, Wu CL, Hsu HC, Shiau AL. B cells are required for tumor-targeting Salmonella in host. Appl Microbiol Biotechnol (2011) 92(6):1251–60. doi: 10.1007/s00253-011-3386-0
20. Stern C, Kasnitz N, Kocijancic D, Trittel S, Riese P, Guzman CA, et al. Induction of CD4(+) and CD8(+) anti-tumor effector T cell responses by bacteria mediated tumor therapy. Int J Cancer (2015) 137(8):2019–28. doi: 10.1002/ijc.29567
21. Lee CH, Hsieh JL, Wu CL, Hsu PY, Shiau AL. T cell augments the antitumor activity of tumor-targeting Salmonella. Appl Microbiol Biotechnol (2011) 90(4):1381–8. doi: 10.1007/s00253-011-3180-z
22. Zhou S, Gravekamp C, Bermudes D, Liu K. Tumour-targeting bacteria engineered to fight cancer. Nat Rev Cancer (2018) 18(12):727–43. doi: 10.1038/s41568-018-0070-z
23. Forbes NS, Munn LL, Fukumura D, Jain RK. Sparse initial entrapment of systemically injected Salmonella typhimurium leads to heterogeneous accumulation within tumors. Cancer Res (2003) 63(17):5188–93.
24. Ganai S, Arenas RB, Sauer JP, Bentley B, Forbes NS. In tumors Salmonella migrate away from vasculature toward the transition zone and induce apoptosis. Cancer Gene Ther (2011) 18(7):457–66. doi: 10.1038/cgt.2011.10
25. Sznol M, Lin SL, Bermudes D, Zheng LM, King I. Use of preferentially replicating bacteria for the treatment of cancer. J Clin Invest (2000) 105(8):1027–30. doi: 10.1172/JCI9818
26. Yu YA, Zhang Q, Szalay AA. Establishment and characterization of conditions required for tumor colonization by intravenously delivered bacteria. Biotechnol Bioeng (2008) 100(3):567–78. doi: 10.1002/bit.21785
27. Yu B, Yang M, Shi L, Yao Y, Jiang Q, Li X. Explicit hypoxia targeting with tumor suppression by creating an “obligate” anaerobic Salmonella Typhimurium strain. Sci Rep (2012) 2:436. doi: 10.1038/srep00436
28. Kasinskas RW, Forbes NS. Salmonella typhimurium specifically chemotax and proliferate in heterogeneous tumor tissue in vitro. Biotechnol Bioeng (2006) 94(4):710–21. doi: 10.1002/bit.20883
29. Kasinskas RW, Forbes NS. Salmonella typhimurium lacking ribose chemoreceptors localize in tumor quiescence and induce apoptosis. Cancer Res (2007) 67(7):3201–9. doi: 10.1158/0008-5472.CAN-06-2618
30. Silva-Valenzuela CA, Desai PT, Molina-Quiroz RC, Pezoa D, Zhang Y, Porwollik S, et al. Solid tumors provide niche-specific conditions that lead to preferential growth of Salmonella. Oncotarget (2016) 7(23):35169–80. doi: 10.18632/oncotarget.9071
31. Anderson CJ, Clark DE, Adli M, Kendall MM. Ethanolamine Signaling Promotes Salmonella Niche Recognition and Adaptation during Infection. PloS Pathog (2015) 11(11):e1005278. doi: 10.1371/journal.ppat.1005278
32. Toley BJ, Forbes NS. Motility is critical for effective distribution and accumulation of bacteria in tumor tissue. Integr Biol (2012) 4(2):165–76. doi: 10.1039/c2ib00091a
33. Stritzker J, Weibel S, Seubert C, Götz A, Tresch A, van Rooijen N, et al. Enterobacterial tumor colonization in mice depends on bacterial metabolism and macrophages but is independent of chemotaxis and motility. Int J Med Microbiol (2010) 300(7):449–56. doi: 10.1016/j.ijmm.2010.02.004
34. Leschner S, Westphal K, Dietrich N, Viegas N, Jablonska J, Lyszkiewicz M, et al. Tumor invasion of Salmonella enterica serovar Typhimurium is accompanied by strong hemorrhage promoted by TNF-alpha. PloS One (2009) 4(8):e6692. doi: 10.1371/journal.pone.0006692
35. Panteli JT, Van Dessel N, Forbes NS. Detection of tumors with fluoromarker-releasing bacteria. Int J Cancer (2020) 146(1):137–49. doi: 10.1002/ijc.32414
36. Xiong S, Qi Z, Ni J, Zhong J, Cao L, Yang K. Attenuated Salmonella typhimurium-mediated tumour targeting imaging based on peptides. Biomater Sci (2020) 8(13):3712–9. doi: 10.1039/D0BM00013B
37. Bartee MY, Dunlap KM, Bartee E. Tumor-Localized Secretion of Soluble PD1 Enhances Oncolytic Virotherapy. Cancer Res (2017) 77(11):2952–63. doi: 10.1158/0008-5472.CAN-16-1638
38. Mi Z, Feng ZC, Li C, Yang X, Ma MT, Rong PF. Salmonella-Mediated Cancer Therapy: An Innovative Therapeutic Strategy. J Cancer (2019) 10(20):4765–76. doi: 10.7150/jca.32650
39. Kim BJ, Forbes NS. Single-Cell Analysis Demonstrates How Nutrient Deprivation Creates Apoptotic and Quiescent Cell Populations in Tumor Cylindroids. Biotechnol Bioeng (2008) 101(4):797–810. doi: 10.1002/bit.21985
40. Liu F, Zhang L, Hoffman RM, Zhao M. Vessel destruction by tumor-targeting Salmonella typhimurium A1-R is enhanced by high tumor vascularity. Cell Cycle (2010) 9(22):4518–24. doi: 10.4161/cc.9.22.13744
41. Tu DG, Chang WW, Lin ST, Kuo CY, Tsao YT, Lee CH. Salmonella inhibits tumor angiogenesis by downregulation of vascular endothelial growth factor. Oncotarget (2016) 7(25):37513–23. doi: 10.18632/oncotarget.7038
42. Chen J, Qiao Y, Tang B, Chen G, Liu X, Yang B, et al. Modulation of Salmonella Tumor-Colonization and Intratumoral Anti-angiogenesis by Triptolide and Its Mechanism. Theranostics (2017) 7(8):2250–60. doi: 10.7150/thno.18816
43. Cai Z, Sanchez A, Shi Z, Zhang T, Liu M, Zhang D. Activation of Toll-like receptor 5 on breast cancer cells by flagellin suppresses cell proliferation and tumor growth. Cancer Res (2011) 71(7):2466–75. doi: 10.1158/0008-5472.CAN-10-1993
44. Sfondrini L, Rossini A, Besusso D, Merlo A, Tagliabue E, Mènard S, et al. Antitumor activity of the TLR-5 ligand flagellin in mouse models of cancer. J Immunol (2006) 176(11):6624–30. doi: 10.4049/jimmunol.176.11.6624
45. Kocijancic D, Leschner S, Felgner S, Komoll RM, Frahm M, Pawar V. Therapeutic benefit of Salmonella attributed to LPS and TNF-alpha is exhaustible and dictated by tumor susceptibility. Oncotarget (2017) 8(22):36492–508. doi: 10.18632/oncotarget.16906
46. Zheng JH, Nguyen VH, Jiang SN, Park SH, Tan W, Hong SH. Two-step enhanced cancer immunotherapy with engineered Salmonella typhimurium secreting heterologous flagellin. Sci Transl Med (2017) 9(376):eaak9537. doi: 10.1126/scitranslmed.aak9537
47. Felgner S, Spöring I, Pawar V, Kocijancic D, Preusse M, Falk C, et al. The immunogenic potential of bacterial flagella for Salmonella-mediated tumor therapy. Int J Cancer (2020) 147(2):448–60. doi: 10.1002/ijc.32807
48. Barak Y, Schreiber F, Thorne SH, Contag CH, Debeer D, Matin A. Role of nitric oxide in Salmonella typhimurium-mediated cancer cell killing. BMC Cancer (2010) 10:146. doi: 10.1186/1471-2407-10-146
49. Spector MP, Garcia Del Portillo F, Bearson SMD, Mahmud A, Magut M, Finlay BB, et al. The rpoS-dependent starvation-stress response locus stiA encodes a nitrate reductase (narZYWV) required for carbon-starvation-inducible thermotolerance and acid tolerance in Salmonella typhimurium. Microbiology (1999) 145(Pt 11):3035–45. doi: 10.1099/00221287-145-11-3035
50. Casanova JE. Bacterial Autophagy: Offense and Defense at the Host-Pathogen Interface. Cell Mol Gastroenterol Hepatol (2017) 4(2):237–43. doi: 10.1016/j.jcmgh.2017.05.002
51. Liu B, Jiang Y, Dong T, Zhao M, Wu J, Li L, et al. Blockage of autophagy pathway enhances Salmonella tumor-targeting. Oncotarget (2016) 7(16):22873–82. doi: 10.18632/oncotarget.8251
52. Lee CH, Lin ST, Liu JJ, Chang WW, Hsieh JL, Wang WK. Salmonella induce autophagy in melanoma by the downregulation of AKT/mTOR pathway. Gene Ther (2014) 21(3):309–16. doi: 10.1038/gt.2013.86
53. Hernandez LD, Pypaert M, Flavell RA, Galán JE. A Salmonella protein causes macrophage cell death by inducing autophagy. J Cell Biol (2003) 163(5):1123–31. doi: 10.1083/jcb.200309161
54. Tsao YT, Kuo YC, Cheng SP, Lee CH. Downregulations of AKT/mTOR Signaling Pathway for Salmonella-Mediated Suppression of Matrix Metalloproteinases-9 Expression in Mouse Tumor Models. Int J Mol Sci (2018) 19(6):1630. doi: 10.3390/ijms19061630
55. Phan TX, Nguyen VH, Duong MT, Hong Y, Choy HE, Min JJ. Activation of inflammasome by attenuated Salmonella typhimurium in bacteria-mediated cancer therapy. Microbiol Immunol (2015) 59(11):664–75. doi: 10.1111/1348-0421.12333
56. Chirullo B, Ammendola S, Leonardi L, Falcini R, Petrucci P, Pistoia C, et al. Attenuated mutant strain of Salmonella Typhimurium lacking the ZnuABC transporter contrasts tumor growth promoting anti-cancer immune response. Oncotarget (2015) 6(19):17648–60. doi: 10.18632/oncotarget.3893
57. Barr TA, Brown S, Mastroeni P, Gray D. B cell intrinsic MyD88 signals drive IFN-gamma production from T cells and control switching to IgG2c. J Immunol (2009) 183(2):1005–12. doi: 10.4049/jimmunol.0803706
58. dos Santos SA, de Andrade DRJ, de Andrade DR. TNF-alpha production and apoptosis in hepatocytes after Listeria monocytogenes and Salmonella Typhimurium invasion. Rev Inst Med Trop Sao Paulo (2011) 53(2):107–12. doi: 10.1590/S0036-46652011000200009
59. Avogadri F, Mittal D, Saccheri F, Sarrafiore M, Ciocca M, Larghi P, et al. Intra-tumoral Salmonella typhimurium induces a systemic anti-tumor immune response that is directed by low-dose radiation to treat distal disease. Eur J Immunol (2008) 38(7):1937–47. doi: 10.1002/eji.200738035
60. Chang SY, Kim YJ, Ko HJ. Potential therapeutic anti-tumor effect of a Salmonella-based vaccine. Hum Vaccin Immunother (2013) 9(8):1654–60. doi: 10.4161/hv.24917
61. Schafer R, Nacy CA, Eisenstein TK. Induction of activated macrophages in C3H/HeJ mice by avirulent Salmonella. J Immunol (1988) 140(5):1638–44.
62. Pawelek JM, Low KB, Bermudes D. Tumor-targeted Salmonella as a novel anticancer vector. Cancer Res (1997) 57(20):4537–44.
63. Johannessen M, Askarian F, Sangvik M, Sollid JE. Bacterial interference with canonical NFkappaB signalling. Microbiology (2013) 159(Pt 10):2001–13. doi: 10.1099/mic.0.069369-0
64. Hernandez-Luna MA, Luria-Perez R. Cancer Immunotherapy: Priming the Host Immune Response with Live Attenuated Salmonella enterica. J Immunol Res (2018) 2018:2984247. doi: 10.1155/2018/2984247
65. Franchi L, Amer A, Body-Malapel M, Kanneganti TD, Ozören N, Jagirdar R, et al. Cytosolic flagellin requires Ipaf for activation of caspase-1 and interleukin 1beta in salmonella-infected macrophages. Nat Immunol (2006) 7(6):576–82. doi: 10.1038/ni1346
66. Pangilinan CR, Lee CH. Salmonella-Based Targeted Cancer Therapy: Updates on A Promising and Innovative Tumor Immunotherapeutic Strategy. Biomedicines (2019) 7(2):36. doi: 10.3390/biomedicines7020036
67. Avogadri F, Martinoli C, Petrovska L, Chiodoni C, Transidico P, Bronte V, et al. Cancer immunotherapy based on killing of Salmonella-infected tumor cells. Cancer Res (2005) 65(9):3920–7. doi: 10.1158/0008-5472.CAN-04-3002
68. Grille S, Moreno M, Bascuas T, Marqués JM, Muñoz N, Lens D, et al. Salmonella enterica serovar Typhimurium immunotherapy for B-cell lymphoma induces broad anti-tumour immunity with therapeutic effect. Immunology (2014) 143(3):428–37. doi: 10.1111/imm.12320
69. Stoll G, Iribarren K, Michels J, Leary A, Zitvogel L, Cremer I, et al. Calreticulin expression: Interaction with the immune infiltrate and impact on survival in patients with ovarian and non-small cell lung cancer. Oncoimmunology (2016) 5(7):e1177692. doi: 10.1080/2162402X.2016.1177692
70. Clay SL, Bravo-Blas A, Wall DM, MacLeod MKL, Milling SWF. Regulatory T cells control the dynamic and site-specificpolarization of total CD4 T cells following Salmonella infection. MucosalImmunol (2020) 13(6):946–57. doi: 10.1038/s41385-020-0299-1
71. Li CX, Yu B, Shi L, Geng W, Lin QB, Ling CC, et al. ‘Obligate’ anaerobic Salmonella strain YB1 suppresses liver tumor growth and metastasis in nude mice. Oncol Lett (2017) 13(1):177–83. doi: 10.3892/ol.2016.5453
72. Vendrell A, Gravisaco MJ, Goin JC, Pasetti MF, Herschllik L, De Toro J, et al. Therapeutic effects of Salmonella typhi in a mouse model of T-cell lymphoma. J Immunother (2013) 36(3):171–80. doi: 10.1097/CJI.0b013e3182886d95
73. Granot Z. Neutrophils as a Therapeutic Target in Cancer. Front Immunol (2019) 10:1710. doi: 10.3389/fimmu.2019.01710
74. Wculek SK, Cueto FJ, Mujal AM, Melero I, Krummel MF, Sancho D. Dendritic cells in cancer immunology and immunotherapy. Nat Rev Immunol (2020) 20(1):7–24. doi: 10.1038/s41577-019-0210-z
75. Saccheri F, Pozzi C, Avogadri F, Barozzi S, Faretta M, Fusi P, et al. Bacteria-induced gap junctions in tumors favor antigen cross-presentation and antitumor immunity. Sci Transl Med (2010) 2(44):44ra57. doi: 10.1126/scitranslmed.3000739
76. Kuan YD, Lee CH. Salmonella overcomes tumor immune tolerance by inhibition of tumor indoleamine 2, 3-dioxygenase 1 expression. Oncotarget (2016) 7(1):374–85. doi: 10.18632/oncotarget.6258
77. Westphal K, Leschner S, Jablonska J, Loessner H, Weiss S. Containment of tumor-colonizing bacteria by host neutrophils. Cancer Res (2008) 68(8):2952–60. doi: 10.1158/0008-5472.CAN-07-2984
78. Abi Abdallah DS, Egan CE, Butcher BA, Denkers EY. Mouse neutrophils are professional antigen-presenting cells programmed to instruct Th1 and Th17 T-cell differentiation. Int Immunol (2011) 23(5):317–26. doi: 10.1093/intimm/dxr007
79. Chen MC, Pangilinan CR, Lee CH. Salmonella Breaks Tumor Immune Tolerance by Downregulating Tumor Programmed Death-Ligand 1 Expression. Cancers (Basel) (2019) 12(1):57. doi: 10.3390/cancers12010057
80. Kim JE, Phan TX, Nguyen VH, Dinh-Vu HV, Zheng JH, Yun M, et al. Salmonella typhimurium Suppresses Tumor Growth via the Pro-Inflammatory Cytokine Interleukin-1 beta. Theranostics (2015) 5(12):1328–42. doi: 10.7150/thno.11432
81. Wang WK, Lu MF, Kuan YD, Lee CH. The treatment of mouse colorectal cancer by oral delivery tumor-targeting Salmonella. Am J Cancer Res (2015) 5(7):2222–8.
82. Vendrell A, Gravisaco MJ, Pasetti MF, Croci M, Colombo L, Rodríguez C, et al. A novel Salmonella Typhi-based immunotherapy promotes tumor killing via an antitumor Th1-type cellular immune response and neutrophil activation in a mouse model of breast cancer. Vaccine (2011) 29(4):728–36. doi: 10.1016/j.vaccine.2010.11.017
83. Liu T, Chopra AK. An enteric pathogen Salmonella enterica serovar Typhimurium suppresses tumor growth by downregulating CD44(high) and CD4T regulatory (T-reg) cell expression in mice: the critical role of lipopolysaccharide and Braun lipoprotein in modulating tumor growth. Cancer Gene Ther (2010) 17(2):97–108. doi: 10.1038/cgt.2009.58
84. Gabrilovich DI. Myeloid-Derived Suppressor Cells. Cancer Immunol Res (2017) 5(1):3–8. doi: 10.1158/2326-6066.CIR-16-0297
85. Saltzman D, Augustin L, Leonard A, Mertensotto M, Schottel J. Low dose chemotherapy combined with attenuated Salmonella decreases tumor burden and is less toxic than high dose chemotherapy in an autochthonous murine model of breast cancer. Surgery (2018) 163(3):509–14. doi: 10.1016/j.surg.2017.09.036
86. Bascuas T, Moreno M, Grille S, Chabalgoity JA. Salmonella Immunotherapy Improves the Outcome of CHOP Chemotherapy in Non-Hodgkin Lymphoma-Bearing Mice. Front Immunol (2018) 9:7. doi: 10.3389/fimmu.2018.00007
87. Din MO, Danino T, Prindle A, Skalak M, Selimkhanov J, Allen K, et al. Synchronized cycles of bacterial lysis for in vivo delivery. Nature (2016) 536(7614):81–5. doi: 10.1038/nature18930
88. Lee CH, Wu CL, Tai YS, Shiau AL. Systemic administration of attenuated Salmonella choleraesuis in combination with cisplatin for cancer therapy. Mol Ther (2005) 11(5):707–16. doi: 10.1016/j.ymthe.2005.01.008
89. Jia LJ, Wei DP, Sun QM, Jin GH, Li SF, Huang Y, et al. Tumor-targeting Salmonella typhimurium improves cyclophosphamide chemotherapy at maximum tolerated dose and low-dose metronomic regimens in a murine melanoma model. Int J Cancer (2007) 121(3):666–74. doi: 10.1002/ijc.22688
90. Hiroshima Y, Zhang Y, Murakami T, Maawy A, Miwa S, Yamamoto M, et al. Efficacy of tumor-targeting Salmonella typhimurium A1-R in combination with anti-angiogenesis therapy on a pancreatic cancer patient-derived orthotopic xenograft (PDOX) and cell line mouse models. Oncotarget (2014) 5(23):12346–57. doi: 10.18632/oncotarget.2641
91. Igarashi K, Kawaguchi K, Zhao M, Kiyuna T, Miyake K, Miyake M, et al. Exquisite Tumor Targeting by Salmonella A1-R in Combination with Caffeine and Valproic Acid Regresses an Adult Pleomorphic Rhabdomyosarcoma Patient-Derived Orthotopic Xenograft Mouse Model. Transl Oncol (2020) 13(2):393–400. doi: 10.1016/j.tranon.2019.10.005
92. Zhang Y, Zhang N, Su S, Hoffman RM, Zhao M. Salmonella typhimurium A1-R tumor targeting in immunocompetent mice is enhanced by a traditional Chinese medicine herbal mixture. Anticancer Res (2013) 33(5):1837–43.
93. Kawaguchi K, Igarashi K, Murakami T, Chmielowski B, Kiyuna T, Zhao M, et al. Tumor-targeting Salmonella typhimurium A1-R combined with temozolomide regresses malignant melanoma with a BRAF-V600E mutation in a patient-derived orthotopic xenograft (PDOX) model. Oncotarget (2016) 7(52):85929–36. doi: 10.18632/oncotarget.13231
94. Murakami T, DeLong J, Eilber FC, Zhao M, Zhang Y, Zhang N, et al. Tumor-targeting Salmonella typhimurium A1-R in combination with doxorubicin eradicate soft tissue sarcoma in a patient-derived orthotopic xenograft (PDOX) model. Oncotarget (2016) 7(11):12783–90. doi: 10.18632/oncotarget.7226
95. Chang WW, Lai CH, Chen MC, Liu CF, Kuan YD, Lin ST, et al. Salmonella enhance chemosensitivity in tumor through connexin 43 upregulation. Int J Cancer (2013) 133(8):1926–35. doi: 10.1002/ijc.28155
96. Yang CJ, Chang WW, Lin ST, Chen MC, Lee CH. Salmonella Overcomes Drug Resistance in Tumor through P-glycoprotein Downregulation. Int J Med Sci (2018) 15(6):574–9. doi: 10.7150/ijms.23285
97. Kawaguchi K, Miyake K, Zhao M, Kiyuna T, Igarashi K, Miyake M, et al. Tumor targeting Salmonella typhimurium A1-R in combination with gemcitabine (GEM) regresses partially GEM-resistant pancreatic cancer patient-derived orthotopic xenograft (PDOX) nude mouse models. Cell Cycle (2018) 17(16):2019–26. doi: 10.1080/15384101.2018.1480223
98. Igarashi K, Kawaguchi K, Kiyuna T, Miyake K, Miyake M, Li S, et al. Tumor-targeting Salmonella typhimurium A1-R combined with recombinant methioninase and cisplatinum eradicates an osteosarcoma cisplatinum-resistant lung metastasis in a patient-derived orthotopic xenograft (PDOX) mouse model: decoy, trap and kill chemotherapy moves toward the clinic. Cell Cycle (2018) 17(6):801–9. doi: 10.1080/15384101.2018.1431596
99. Yano S, Takehara K, Zhao M, Tan Y, Han Q, Li S, et al. Tumor-specific cell-cycle decoy by Salmonella typhimurium A1-R combined with tumor-selective cell-cycle trap by methioninase overcome tumor intrinsic chemoresistance as visualized by FUCCI imaging. Cell Cycle (2016) 15(13):1715–23. doi: 10.1080/15384101.2016.1181240
100. Mercado-Lubo R, Zhang Y, Zhao L, Rossi K, Wu X, Zou Y, et al. A Salmonella nanoparticle mimic overcomes multidrug resistance in tumours. Nat Commun (2016) 7:12225. doi: 10.1038/ncomms12225
101. Zhu H, Li Z, Mao S, Ma B, Zhou S, Deng L, et al. Antitumor effect of sFlt-1 gene therapy system mediated by Bifidobacterium Infantis on Lewis lung cancer in mice. Cancer Gene Ther (2011) 18(12):884–96. doi: 10.1038/cgt.2011.57
102. Jiang SN, Phan TX, Nam TK, Nguyen VH, Kim HS, Bom HS, et al. Inhibition of tumor growth and metastasis by a combination of Escherichia coli-mediated cytolytic therapy and radiotherapy. Mol Ther (2010) 18(3):635–42. doi: 10.1038/mt.2009.295
103. Platt J, Sodi S, Kelley M, Rockwell S, Bermudes D, Low KB, et al. Antitumour effects of genetically engineered Salmonella in combination with radiation. Eur J Cancer (2000) 36(18):2397–402. doi: 10.1016/S0959-8049(00)00336-1
104. Yoon WS, Kim S, Seo S, Park Y. Salmonella typhimurium with gamma-radiation induced H2AX phosphorylation and apoptosis in melanoma. Biosci Biotechnol Biochem (2014) 78(6):1082–5. doi: 10.1080/09168451.2014.905173
105. Liu X, Jiang S, Piao L, Yuan F. Radiotherapy combined with an engineered Salmonella typhimurium inhibits tumor growth in a mouse model of colon cancer. Exp Anim (2016) 65(4):413–8. doi: 10.1538/expanim.16-0033
106. Murakami T, Hiroshima Y, Zhao M, Zhang Y, Chishima T, Tanaka K, et al. Adjuvant treatment with tumor-targeting Salmonella typhimurium A1-R reduces recurrence and increases survival after liver metastasis resection in an orthotopic nude mouse model. Oncotarget (2015) 6(39):41856–62. doi: 10.18632/oncotarget.6170
107. Kefayat A, Ghahremani F, Motaghi H, Rostami S, Mehrgardi MA. Alive attenuated Salmonella as a cargo shuttle for smart carrying of gold nanoparticles to tumour hypoxic regions. J Drug Target (2019) 27(3):315–24. doi: 10.1080/1061186X.2018.1523417
108. Chen W, Wang Y, Qin M, Zhang X, Zhang Z, Sun X, et al. Bacteria-Driven Hypoxia Targeting for Combined Biotherapy and Photothermal Therapy. ACS Nano (2018) 12(6):5995–6005. doi: 10.1021/acsnano.8b02235
109. Binder DC, Arina A, Wen DC, Tu T, Zhao M, Hoffman RM, et al. Tumor relapse prevented by combining adoptive T cell therapy with Salmonella typhimurium. Oncoimmunology (2016) 5(6):e1130207. doi: 10.1080/2162402X.2015.1130207
110. Binder DC, Engels B, Arina A, Yu P, Slauch JM, Fu YX, et al. Antigen-specific bacterial vaccine combined with anti-PD-L1 rescues dysfunctional endogenous T cells to reject long-established cancer. Cancer Immunol Res (2013) 1(2):123–33. doi: 10.1158/2326-6066.CIR-13-0058
111. Engelhardt R, Mackensen A, Galanos C. Phase I trial of intravenously administered endotoxin (Salmonella abortus equi) in cancer patients. Cancer Res (1991) 51(10):2524–30.
112. Otto F, Schmid P, Mackensen A, Wehr U, Seiz A, Braun M, et al. Phase II trial of intravenous endotoxin in patients with colorectal and non-small cell lung cancer. Eur J Cancer (1996) 32A(10):1712–8. doi: 10.1016/0959-8049(96)00186-4
113. Goto S, Sakai S, Kera J, Suma Y, Soma GI, Takeuchi S. Intradermal administration of lipopolysaccharide in treatment of human cancer. Cancer Immunol Immunother (1996) 42(4):255–61. doi: 10.1007/s002620050279
114. Toso JF, Gill VJ, Hwu P, Marincola FM, Restifo NP, Schwartzentruber DJ, et al. Phase I study of the intravenous administration of attenuated Salmonella typhimurium to patients with metastatic melanoma. J Clin Oncol (2002) 20(1):142–52. doi: 10.1200/JCO.2002.20.1.142
115. Nemunaitis J, Cunningham C, Senzer N, Kuhn J, Cramm J, Litz C, et al. Pilot trial of genetically modified, attenuated Salmonella expressing the E. coli cytosine deaminase gene in refractory cancer patients. Cancer Gene Ther (2003) 10(10):737–44. doi: 10.1038/sj.cgt.7700634
116. Thamm DH, Kurzman ID, King I, Li Z, Sznol M, Dubielzig RR, et al. Systemic administration of an attenuated, tumor-targeting Salmonella typhimurium to dogs with spontaneous neoplasia: phase I evaluation. Clin Cancer Res (2005) 11(13):4827–34. doi: 10.1158/1078-0432.CCR-04-2510
117. Fritz SE, Henson MS, Greengard E, Winter AL, Stuebner KM, Yoon U, Wilk VL, et al. A phase I clinical study to evaluate safety of orally administered, genetically engineered Salmonella enterica serovar Typhimurium for canine osteosarcoma. Vet Med Sci (2016) 2(3):179–90. doi: 10.1002/vms3.32
118. Schmitz-Winnenthal FH, Hohmann N, Schmidt T, Podola L, Friedrich T, Lubenau H, et al. A phase 1 trial extension to assess immunologic efficacy and safety of prime-boost vaccination with VXM01, an oral T cell vaccine against VEGFR2, in patients with advanced pancreatic cancer. Oncoimmunology (2018) 7(4):e1303584. doi: 10.1080/2162402X.2017.1303584
119. Gniadek TJ, Augustin L, Schottel J, Leonard A, Saltzman D, Greeno E, et al. Dose Escalation, Single Dose Trial of Oral Attenuated Salmonella typhimurium Containing Human IL-2 in Patients With Metastatic Gastrointestinal Cancers. J Immunother (2020) 43(7):217–21. doi: 10.1097/CJI.0000000000000325
120. Schmitz-Winnenthal FH, Hohmann N, Niethammer AG, Friedrich T, Lubenau H, Springer M, et al. Anti-angiogenic activity of VXM01, an oral T-cell vaccine against VEGF receptor 2, in patients with advanced pancreatic cancer: A randomized, placebo-controlled, phase 1 trial. Oncoimmunology (2015) 4(4):e1001217. doi: 10.1080/2162402X.2014.1001217
121. U.S. National Institutes of Health. Clinicaltrials.gov. Multiple Myeloma Trial of Orally Administered Salmonella Based Survivin Vaccine (MAPSS). Available at: https://clinicaltrials.gov/ct2/show/NCT03762291 (Accessed 23 April 2019).
122. Abbott M, Ustoyev Y. Cancer and the Immune System: The History and Background of Immunotherapy. Semin Oncol Nurs (2019) 35(5):150923. doi: 10.1016/j.soncn.2019.08.002
123. Hanahan D, Weinberg RA. Hallmarks of cancer: the next generation. Cell (2011) 144(5):646–74. doi: 10.1016/j.cell.2011.02.013
124. Parsons BL. Multiclonal tumor origin: Evidence and implications. Mutat Res (2018) 777:1–18. doi: 10.1016/j.mrrev.2018.05.001
125. Hegde PS, Chen DS. Top 10 Challenges in Cancer Immunotherapy. Immunity (2020) 52(1):17–35. doi: 10.1016/j.immuni.2019.12.011
126. Olszanski AJ. Principles of immunotherapy. J Natl Compr Canc Netw (2015) 13(5 Suppl):670–2. doi: 10.6004/jnccn.2015.0199
127. Fuge O, Vasdev N, Allchorne P, Green JS. Immunotherapy for bladder cancer. Res Rep Urol (2015) 7:65–79. doi: 10.2147/RRU.S63447
128. Carswell EA, Old LJ, Kassel RL, Green S, Fiore N, Williamson B. An endotoxin-induced serum factor that causes necrosis of tumors. Proc Natl Acad Sci U.S.A. (1975) 72(9):3666–70. doi: 10.1073/pnas.72.9.3666
129. Balkwill F. Tumour necrosis factor and cancer. Nat Rev Cancer (2009) 9(5):361–71. doi: 10.1038/nrc2628
130. Moussa M, Papatsoris A, Abou Chakra M, Sryropoulou D, Dellis A. Pharmacotherapeutic strategies for castrate-resistant prostate cancer. Expert Opin Pharmacother (2020) 21(12):1431–48. doi: 10.1080/14656566.2020.1767069
131. Met Ö, Jensen KM, Chamberlain CA, Donia M, Svane IM. Principles of adoptive T cell therapy in cancer. Semin Immunopathol (2019) 41(1):49–58. doi: 10.1007/s00281-018-0703-z
132. Lesterhuis WJ, Haanen JB, Punt CJ. Cancer immunotherapy–revisited. Nat Rev Drug Discovery (2011) 10(8):591–600. doi: 10.1038/nrd3500
133. Abril-Rodriguez G, Ribas A. SnapShot: Immune Checkpoint Inhibitors. Cancer Cell (2017) 31(6):848–848.e1. doi: 10.1016/j.ccell.2017.05.010
134. Qin S, Xu L, Yi M, Yu S, Wu K, Luo S. Novel immune checkpoint targets: moving beyond PD-1 and CTLA-4. Mol Cancer (2019) 18(1):155. doi: 10.1186/s12943-019-1091-2
135. Sharpe AH. Introduction to checkpoint inhibitors and cancer immunotherapy. Immunol Rev (2017) 276(1):5–8. doi: 10.1111/imr.12531
136. Fukuhara H, Ino Y, Todo T. Oncolytic virus therapy: A new era of cancer treatment at dawn. Cancer Sci (2016) 107(10):1373–9. doi: 10.1111/cas.13027
137. Lawler SE, Speranza MC, Cho CF, Chiocca EA. Oncolytic Viruses in Cancer Treatment: A Review. JAMA Oncol (2017) 3(6):841–9. doi: 10.1001/jamaoncol.2016.2064
138. Sharpe AH, Pauken KE. The diverse functions of the PD1 inhibitory pathway. Nat Rev Immunol (2018) 18(3):153–67. doi: 10.1038/nri.2017.108
139. Schildberg FA, Klein SR, Freeman GJ, Sharpe AH. Coinhibitory Pathways in the B7-CD28 Ligand-Receptor Family. Immunity (2016) 44(5):955–72. doi: 10.1016/j.immuni.2016.05.002
140. Wei SC, Levine JH, Cogdill AP, Zhao Y, Anang NAS, Andrews MC, et al. Distinct Cellular Mechanisms Underlie Anti-CTLA-4 and Anti-PD-1 Checkpoint Blockade. Cell (2017) 170(6):1120–33.e17. doi: 10.1016/j.cell.2017.07.024
141. Twyman-Saint Victor C, Rech AJ, Maity A, Rengan R, Pauken C, Stelekati E, et al. Radiation and dual checkpoint blockade activate non-redundant immune mechanisms in cancer. Nature (2015) 520(7547):373–7. doi: 10.1038/nature14292
142. Sotomayor EM, Borrello I, Tubb E, Allison JP, Levitsky HI. In vivo blockade of CTLA-4 enhances the priming of responsive T cells but fails to prevent the induction of tumor antigen-specific tolerance. Proc Natl Acad Sci USA (1999) 96(20):11476–81. doi: 10.1073/pnas.96.20.11476
143. Pauken KE, Dougan M, Rose NR, Lichtman AH, Sharpe AH. Adverse Events Following Cancer Immunotherapy: Obstacles and Opportunities. Trends Immunol (2019) 40(6):511–23. doi: 10.1016/j.it.2019.04.002
144. Wang DY, Salem JE, Cohen JV, Chandra S, Menzer C, Ye F, et al. Fatal Toxic Effects Associated With Immune Checkpoint Inhibitors: A Systematic Review and Meta-analysis. JAMA Oncol (2018) 4(12):1721–8. doi: 10.1001/jamaoncol.2018.3923
145. Haanen JBAG, Carbonnel F, Robert C, Kerr KM, Peters S, Larkin J, et al. Management of toxicities from immunotherapy: ESMO Clinical Practice Guidelines for diagnosis, treatment and follow-up. Ann Oncol (2018) 29(Suppl 4):iv264–6. doi: 10.1093/annonc/mdy162
146. Postow MA, Sidlow R, Hellmann MD. Immune-Related Adverse Events Associated with Immune Checkpoint Blockade. N Engl J Med (2018) 378(2):158–68. doi: 10.1056/NEJMra1703481
147. Boutros C, Tarhini A, Routier E, Lambotte O, Ladurie FL, Carbonnel F, et al. Safety profiles of anti-CTLA-4 and anti-PD-1 antibodies alone and in combination. Nat Rev Clin Oncol (2016) 13(8):473–86. doi: 10.1038/nrclinonc.2016.58
148. Weber JS, Kahler KC, Hauschild A. Management of immune-related adverse events and kinetics of response with ipilimumab. J Clin Oncol (2012) 30(21):2691–7. doi: 10.1200/JCO.2012.41.6750
149. Johnson DB, Sullivan RJ, Ott PA, Carlino MS, Khushalani NI, Ye F, et al. Ipilimumab Therapy in Patients With Advanced Melanoma andPreexisting Autoimmune Disorders. JAMA Oncol (2016)2(2):234–40. doi: 10.1001/jamaoncol.2015.4368
150. Menzies AM, Johnson DB, Ramanujam S, Atkinson VG, Wong ANM, Park JJ, et al. Anti-PD-1 therapy in patients with advanced melanoma and preexistingautoimmune disorders or major toxicity with ipilimumab. Ann Oncol(2017) 28(2):368–76. doi: 10.1093/annonc/mdw443
151. June CH, O'Connor RS, Kawalekar OU, Ghassemi S, Milone MC. CAR T cell immunotherapy for human cancer. Science (2018) 359(6382):1361–5. doi: 10.1126/science.aar6711
152. Twumasi-Boateng K, Pettigrew JL, Kwok YYE, Bell JC, Nelson BH. Oncolytic viruses as engineering platforms for combination immunotherapy (vol 18, pg 419, 2018). Nat Rev Cancer (2018) 18(8):526–6. doi: 10.1038/s41568-018-0019-2
153. Kanapathipillai M. Treating p53 Mutant Aggregation-Associated Cancer. Cancers (Basel) (2018) 10(6):154. doi: 10.3390/cancers10060154
154. Li X, Li Y, Hu J, Wang B, Zhao L, Ji K, et al. Plasmid-based E6-specific siRNA and co-expression of wild-type p53 suppresses the growth of cervical cancer in vitro and in vivo. Cancer Lett (2013) 335(1):242–50. doi: 10.1016/j.canlet.2013.02.034
155. Jiang T, Zhou C, Gu J, Liu Y, Zhao L, Li W, et al. Enhanced therapeutic effect of cisplatin on the prostate cancer in tumor-bearing mice by transfecting the attenuated Salmonella carrying a plasmid co-expressing p53 gene and mdm2 siRNA. Cancer Lett (2013) 337(1):133–42. doi: 10.1016/j.canlet.2013.05.028
156. Zhang L, Gao L, Li Y, Lin G, Shao Y, Ji K, et al. Effects of plasmid-based Stat3-specific short hairpin RNA and GRIM-19 on PC-3M tumor cell growth. Clin Cancer Res (2008) 14(2):559–68. doi: 10.1158/1078-0432.CCR-07-1176
157. Gao L, Zhang L, Hu J, Li F, Shao Y, Zhao D, et al. Down-regulation of signal transducer and activator of transcription 3 expression using vector-based small interfering RNAs suppresses growth of human prostate tumor in vivo. Clin Cancer Res (2005) 11(17):6333–41. doi: 10.1158/1078-0432.CCR-05-0148
158. Yang N, Zhu X, Chen L, Li S, Ren D. Oral administration of attenuated S. typhimurium carrying shRNA-expressing vectors as a cancer therapeutic. Cancer Biol Ther (2008) 7(1):145–51. doi: 10.4161/cbt.7.1.5195
159. Gu J, Li Y, Zeng J, Wang B, Ji K, Tang Y, et al. Knockdown of HIF-1alpha by siRNA-expressing plasmid delivered by attenuated Salmonella enhances the antitumor effects of cisplatin on prostate cancer. Sci Rep (2017) 7(1):7546. doi: 10.1038/s41598-017-07973-4
160. Blache CA, Manuel ER, Kaltcheva TI, Wong AN, Ellenhorn JD, Blazar BR, et al. Systemic delivery of Salmonella typhimurium transformed with IDO shRNA enhances intratumoral vector colonization and suppresses tumor growth. Cancer Res (2012) 72(24):6447–56. doi: 10.1158/0008-5472.CAN-12-0193
161. Ahmad S, Casey G, Cronin M, Rajendran S, Sweeney P, Tangney M, et al. Induction of effective antitumor response after mucosal bacterial vector mediated DNA vaccination with endogenous prostate cancer specific antigen. J Urol (2011) 186(2):687–93. doi: 10.1016/j.juro.2011.03.139
162. Lewēn S, Zhou H, Hu HD, Cheng T, Markowitz D, Reisfeld RA, et al. A Legumain-based minigene vaccine targets the tumor stroma and suppresses breast cancer growth and angiogenesis. Cancer Immunol Immunother (2008) 57(4):507–15. doi: 10.1007/s00262-007-0389-x
163. Yuhua L, Kunyuan G, Hui C, Yongmei X, Chaoyang S, Xun T, et al. Oral cytokine gene therapy against murine tumor using attenuated Salmonella typhimurium. Int J Cancer (2001) 94(3):438–43. doi: 10.1002/ijc.1489
164. Agorio C, Schreiber F, Sheppard M, Mastroeni P, Fernandez M, Martinez MA, et al. Live attenuated Salmonella as a vector for oral cytokine gene therapy in melanoma. J Gene Med (2007) 9(5):416–23. doi: 10.1002/jgm.1023
165. Sorenson BS, Banton KL, Frykman NL, Leonard AS, Saltzman DA. Attenuated Salmonella typhimurium with IL-2 gene reduces pulmonary metastases in murine osteosarcoma. Clin Orthop Relat Res (2008) 466(6):1285–91. doi: 10.1007/s11999-008-0243-2
166. Yoon W, Park YC, Kim J, Chae YS, Byeon JH, Min SH, et al. Application of genetically engineered Salmonella typhimurium for interferon-gamma-induced therapy against melanoma. Eur J Cancer (2017) 70:48–61. doi: 10.1016/j.ejca.2016.10.010
167. Yoon WS, Chae YS, Hong J, Park YK. Antitumor therapeutic effects of a genetically engineered Salmonella typhimurium harboring TNF-alpha in mice. Appl Microbiol Biotechnol (2011) 89(6):1807–19. doi: 10.1007/s00253-010-3006-4
168. Chowdhury S, Castro S, Coker C, Hinchliffe TE, Arpaia N, Danino T. Programmable bacteria induce durable tumor regression and systemic antitumor immunity. Nat Med (2019) 25(7):1057–63. doi: 10.1038/s41591-019-0498-z
169. Ribas A, Hu-Lieskovan S. What does PD-L1 positive or negative mean? J Exp Med (2016) 213(13):2835–40. doi: 10.1084/jem.20161462
170. Tumeh PC, Harview CL, Yearley JH, Shintaku IP, Taylor EJ, Robert L, et al. PD-1 blockade induces responses by inhibiting adaptive immune resistance. Nature (2014) 515(7528):568–71. doi: 10.1038/nature13954
171. McDermott DF, Sosman JA, Sznol M, Massard C, Gordon MS, Hamid O, et al. Atezolizumab, an Anti-Programmed Death-Ligand 1 Antibody, in Metastatic Renal Cell Carcinoma: Long-Term Safety, Clinical Activity, and Immune Correlates From a Phase Ia Study. J Clin Oncol (2016) 34(8):833–42. doi: 10.1200/JCO.2015.63.7421
172. Herbst RS, Soria JC, Kowanetz M, Fine GD, Hamid O, Gordon MS, et al. Predictive correlates of response to the anti-PD-L1 antibody MPDL3280A in cancer patients. Nature (2014) 515(7528):563–7. doi: 10.1038/nature14011
173. Larkin J, Chiarion-Sileni V, Gonzalez R, Grob JJ, Cowey CL, Lao CD, et al. Combined Nivolumab and Ipilimumab or Monotherapy in UntreatedMelanoma. N Engl J Med (2015)373(1):23–34. doi: 10.1056/NEJMoa1504030
174. Postow MA, Chesney J, Pavlick AC, Robert C, Grossmann K, McDermott D, et al. Nivolumab and ipilimumab versus ipilimumab in untreated melanoma. N Engl J Med (2015) 372(21):2006–17. doi: 10.1056/NEJMoa1414428
175. O’Malley G, Treacy O, Lynch K, Naicker SD, Leonard NA, Lohan P, et al. Stromal Cell PD-L1 Inhibits CD8(+) T-cell Antitumor Immune Responses and Promotes Colon Cancer. Cancer Immunol Res (2018) 6(11):1426–41. doi: 10.1158/2326-6066.CIR-17-0443
176. Lopez-Medina M, Perez-Lopez A, Alpuche-Aranda C, Ortiz-Navarrete V. Salmonella induces PD-L1 expression in B cells. Immunol Lett (2015) 167(2):131–40. doi: 10.1016/j.imlet.2015.08.004
177. Lopez-Medina M, Perez-Lopez A, lpuche-Aranda C, Ortiz-Navarrete V, Lopez-Medina M, Lopez-Medina M. Salmonella modulates B cell biology to evade CD8(+) T cell-mediated immune responses. Front Immunol (2014) 5:586. doi: 10.3389/fimmu.2014.00586
178. Newland SA, Phillips JM, Mastroeni P, Azuma M, Zaccone P, Cooke A. PD-L1 blockade overrides Salmonella typhimurium-mediated diabetes prevention in NOD mice: no role for Tregs. Eur J Immunol (2011) 41(10):2966–76. doi: 10.1002/eji.201141544
179. Srinivasan A, Nanton M, Griffin A, McSorley SJ. Culling of activated CD4 T cells during typhoid is driven by Salmonella virulence genes. J Immunol (2009) 182(12):7838–45. doi: 10.4049/jimmunol.0900382
180. Sahler JM, Eade CR, Altier C, March JC. Salmonella enterica Serovar Typhimurium Increases Functional PD-L1 Synergistically with Gamma Interferon in Intestinal Epithelial Cells via Salmonella Pathogenicity Island 2. Infect Immun (2018) 86(5):e00674–17. doi: 10.1128/IAI.00674-17
181. Phan T, Nguyen VH, D'Alincourt MS, Manuel ER, Kaltcheva T, Tsai W, et al. Salmonella-mediated therapy targeting indoleamine 2, 3-dioxygenase 1 (IDO) activates innate immunity and mitigates colorectal cancer growth. Cancer Gene Ther (2020) 27(3-4):235–45. doi: 10.1038/s41417-019-0089-7
182. Zhao T, Feng Y, Guo M, Zhang C, Wu Q, Chen J, et al. Combination of attenuated Salmonella carrying PD-1 siRNA with nifuroxazide for colon cancer therapy. J Cell Biochem (2020) 121(2):1973–85. doi: 10.1002/jcb.29432
Keywords: Salmonella, cancer immunotherapy, tumor microenvironment, combination therapy, bacterial therapy
Citation: Wang D, Wei X, Kalvakolanu DV, Guo B and Zhang L (2021) Perspectives on Oncolytic Salmonella in Cancer Immunotherapy—A Promising Strategy. Front. Immunol. 12:615930. doi: 10.3389/fimmu.2021.615930
Received: 10 October 2020; Accepted: 11 January 2021;
Published: 25 February 2021.
Edited by:
Basel K. Al-Ramadi, United Arab Emirates University, United Arab EmiratesReviewed by:
Che-Hsin Lee, National Sun Yat-sen University, TaiwanZong Sheng Guo, University of Pittsburgh, United States
Copyright © 2021 Wang, Wei, Kalvakolanu, Guo and Zhang. This is an open-access article distributed under the terms of the Creative Commons Attribution License (CC BY). The use, distribution or reproduction in other forums is permitted, provided the original author(s) and the copyright owner(s) are credited and that the original publication in this journal is cited, in accordance with accepted academic practice. No use, distribution or reproduction is permitted which does not comply with these terms.
*Correspondence: Baofeng Guo, Z2JmQGpsdS5lZHUuY24=; Ling Zhang, emhhbmdsaW5nM0BqbHUuZWR1LmNu