- Rotterdam Transplant Group, Department of Internal Medicine, Nephrology and Transplantation, Erasmus MC, Erasmus University Medical Center, Rotterdam, Netherlands
The endothelium plays a key role in acute and chronic rejection of solid organ transplants. During both processes the endothelium is damaged often with major consequences for organ function. Also, endothelial cells (EC) have antigen-presenting properties and can in this manner initiate and enhance alloreactive immune responses. For decades, knowledge about these roles of EC have been obtained by studying both in vitro and in vivo models. These experimental models poorly imitate the immune response in patients and might explain why the discovery and development of agents that control EC responses is hampered. In recent years, various innovative human 3D in vitro models mimicking in vivo organ structure and function have been developed. These models will extend the knowledge about the diverse roles of EC in allograft rejection and will hopefully lead to discoveries of new targets that are involved in the interactions between the donor organ EC and the recipient's immune system. Moreover, these models can be used to gain a better insight in the mode of action of the currently prescribed immunosuppression and will enhance the development of novel therapeutics aiming to reduce allograft rejection and prolong graft survival.
Introduction
The endothelium has an important role in transplantation. First, a well-functioning endothelium is crucial for the health of a transplant as it regulates oxygen and nutrient provision to that organ. Second, endothelial cells (EC) play an active role in allograft rejection (1–3).
The endothelium of the transplant is the first contact site for the recipient's immune system with donor cells. This barrier is important as it regulates the flux of immune cells into and out of the transplanted organ, which is essential for protecting the allograft from pathogens (2, 3). However, in the setting of solid organ transplantation, the endothelial barrier also facilitates influx of alloreactive immune cells that can damage the allograft and subsequently lead to allograft rejection (1, 4, 5). Transendothelial migration of recipient immune cells consists of several steps in which immune cells are attracted, roll along, adhere, and eventually migrate through the endothelium (2, 6). In this cascade of events the expression of specific membrane molecules—selectins, integrins, and cytokine-induced adhesion molecules (CAMs)—on both EC and immune cells play a key role. Upon activation, EC increase the expression of these membrane molecules, along with the release of several pro-inflammatory cytokines and chemokines and the loosening of intercellular vascular endothelial cadherin (VE-cadherin) junctions (4, 5, 7, 8). In organ transplantation, several pathways can lead to such EC activation resulting in enhanced influx of (alloreactive) immune cells.
EC activation takes place during the transplantation procedure, when EC of the allograft are affected by ischemia and reperfusion injury (IRI). The temporary absence of circulation causes hypoxia and leads to activation and injury of the donor endothelium even before explantation of the organ (9–11). Upon reperfusion of the donor organ in the recipient, reactive oxygen species (ROS) are produced causing a second wave of EC injury. This results in apoptosis, necrosis and autophagy of EC. Moreover, EC injury and activation leads to a general immune response and chemotaxis of immune cells (12, 13).
EC activation is also seen in different types of allograft rejection, in which EC can be both target and stimulator of the rejection response, i.e., in both cellular and humoral rejection (1, 5, 14, 15). The earliest rejection event after transplantation is known as hyperacute antibody mediated rejection (ABMR), in which preformed donor-specific antibodies (DSA) recognize human leukocyte antigen (HLA) or ABO antigens on EC. Resting EC express HLA class I molecules, but activated EC highly increase the expression of both HLA class I and HLA class II molecules. Therefore, during IRI, preformed anti-HLA DSA easily recognize and subsequently damage the EC and graft. Other than the IRI pathway, DSA binding itself also triggers the activation of EC (14, 16, 17). However, such hyperacute ABMR events are barely seen nowadays, mainly due to improved pre-transplantation screenings.
At later stages after transplantation acute ABMR can develop in which de novo anti-HLA DSA or non-HLA EC targeted antibodies are involved. These antibodies can cause activation and destruction of the donor endothelium in which either complement dependent cellular cytotoxicity (CDCC) or antibody-dependent cell-mediated cytotoxicity (ADCC) occurs (14, 15, 18, 19).
Also, cytotoxic lymphocytes (CTL) can recognize donor HLA class I and kill donor EC, which is typically seen in T cell mediated rejection (TCMR) (2, 18, 20). These CTL can be activated by antigen presentation of both donor and recipient APCs within secondary lymphoid organs (18).
Moreover, like professional APC, the endothelium itself is also capable of initiating alloreactive T cell responses. The mechanisms through which this occurs are via enhanced expression of donor HLA class I and II on the EC surface, provision of costimulatory signals to lymphocytes, and cytokine production (21, 22). Through direct and indirect pathways EC can activate CD4 or CD8 memory T cells, which can subsequently result in TCMR (2, 18, 23).
More recently, another form of acute allograft rejection has been recognized, in which microvascular inflammation is not caused by classical ABMR or TCMR pathways, but in which NK cells play a dominant role. HLA-I expression on EC is found to directly activate recipient NK cells, which subsequently leads to EC damage (24).
In addition to acute rejection events, it is hypothesized that subclinical EC activation is involved in the development of chronic allograft rejection. However, this mechanism is less well-understood (14, 23, 25).
EC of the transplant receive the first blow during and after transplantation. However, EC possess a great regenerative capacity (26, 27). EC of donor origin have the capacity to proliferate and replace lost or injured cells. In addition to donor EC, also recipient EC can replace the destructed donor EC to restore the continuum of the vessel wall, leading to EC chimerism. It is hypothesized that EC chimerism might lead to reduced immunogenicity of the endothelium (28–34).
It is clear that EC play a key role in organ transplantation, but research gaps are still present, including (i) the precise role of non-HLA endothelial targeted alloantibodies [i.e., MHC class I related chain A (MICA) and anti-endothelial cell antibodies (AECA)] and (ii) the incidence and degree of EC chimerism and relation with immunogenicity.
Much of our current knowledge on the role of EC in alloreactivity comes from in vitro and in vivo research models. In this review we discuss the value and limitations of these research models and introduce novel innovative research models that may enable us to profoundly explore the interaction of immune cells with EC in allograft rejection. This will help us to find new targets to protect the graft EC from damage as well as to explore novel treatments to reduce allograft rejection and prolong graft survival.
Conventional Models for Studying the Role of Endothelial Cells in Allograft Rejection
Conventional Models
The most-used in vitro experimental setup to study the role of EC in allograft rejection is the static 2D co-culture of human umbilical vein endothelial cells (HUVEC) with HLA-mismatched immune cells. Functional analyses are performed by activation, proliferation, transmigration, suppression, and cytotoxicity assays. In these models, cell interactions are studied by flow cytometry, immunofluorescence microscopy, multiplex protein assays, and gene expression analyses (20–22, 35–60). Several agents that specifically block HLA molecules, costimulatory molecules or cytokines have been studied with these methods to investigate their effect on activation, proliferation, and transmigration of immune cells (21, 22, 35, 36, 38, 39, 44, 48, 50, 60). Also, the effect of immunosuppressive drugs on EC and their interaction with immune cells have been examined with these experimental models (22, 45, 52, 61–67).
Animal models have been used to study the role of EC in allograft rejection in a physiological setting. Mostly rodent models are used to transplant organs between major histocompatibility complex (MHC)-mismatched rodent strains. Subsequently, EC—immune cell interactions are studied in blood samples and harvested organs (e.g., the transplanted organ and the secondary lymphoid organs) (21, 29, 40, 42, 68–77). Also, the effect of several immunosuppressive drugs on EC have been investigated within these models, for example to investigate if these drugs can protect EC from IRI injury and reduce EC activation and immune cell infiltration (29, 69, 71, 75).
Limitations of Conventional Models
Despite the breakthroughs that have been achieved with conventional experimental models, novel models that better mimic the human EC—immune cell spatiotemporal interaction in the allograft are needed in the search for effective therapies as conventional models have some limitations: Although easy to work with, HUVEC are not per se the best cell type to study allograft rejection in vitro as EC of different origin are very heterogeneous and differ in their shape, cell-cell connections, membrane molecules and permeability. Especially macrovascular EC, like HUVEC, and microvascular EC are highly divergent. Among EC of different organs—and even within an organ- many differences exist as well (8, 78–83). Also, EC from patients may be affected by their condition (for example aging, comorbidity, and drugs) and therefore behave differently than EC derived from healthy controls. For specific research aims, this issue can be bypassed by using patient derived EC. For example, an EC crossmatch model to study ABMR has been established with the use of transplant donor derived EC and corresponding recipient sera (59, 84).
Furthermore, 2D monolayer cultures lack spatial interaction with other neighboring cell types such as pericytes and smooth muscle cells (SMC) which should also be included in the experimental setup (85). Moreover, the EC glycocalyx protects EC from interaction with immune cells, but the glycocalyx is not represented well in conventional static 2D models (86, 87).
Another issue is that static 2D culture conditions lack physiological perfusion and vessel resistance. The importance of mimicking a physiological perfusion of EC is emphasized by the fact that the level of shear stress along with the flow pattern (laminar or disturbed) highly influences EC morphology, migration, proliferation, gene expression, and permeability (88, 89).
Lack of flow and lack of multicellular spatial interaction are not an issue in animal models. However, limiting factors of animal models are the huge genetic, immunological, and pharmacokinetic differences between animals and humans (37, 90–96). Also, many differences between human and animal surgical transplantation procedures exist (97–100). Therefore, results from these animal models cannot be fully translated to human solid organ transplantation. Also, the ethical dilemmas about animal models are growing worldwide and ethical approval for animal studies has become much stricter in most countries.
Recently, novel innovative models for studying tissue and immune interactions in vitro have been developed. These models may overcome several of the limitations of conventional models and could also be useful for studying the role of EC in allograft rejection. Below an overview of the most promising models is provided. Table 1 summarizes advantages and limitations of both conventional and novel models.
Advanced Experimental Models to Study the Role of Endothelial Cells in Allograft Rejection
2D Microfluidic Culture Systems
When using a 2D co-culture model with an endothelial cell line and immune cells, a few adjustments can highly improve the system. A great step forward toward more physiological conditions can be made when changing from static to dynamic co-culture to mimic vessel perfusion. Conventional culture plates or microfluidic chips can be connected to peristaltic or pulsatile pumps to create a continuous flow of cell medium, serum or plasma (Figure 1) (101–103). These dynamic flow systems have already been successfully used for studying endothelial and immune cell interactions (101, 104, 105). In addition to simulating physiological perfusion, such systems allow studying the effects of abnormal flow, for example to investigate the effect of discontinued flow which occurs in organs during the surgical transplant procedure (102, 103, 106). Other important steps to improve the 2D co-culture system are choosing the microvascular endothelial cell line of the organ of interest and including neighboring cell types in the system, like pericytes or SMC, through a 2D two-layer design (107).
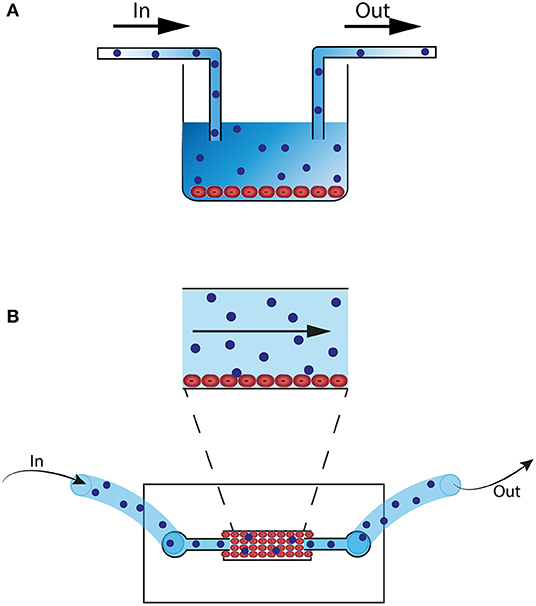
Figure 1. 2D microfluidic in vitro models. (A) Culture well with HUVEC or organ-specific EC (red). The well is connected with a pump (not shown) and flow tubes. Alloreactive lymphocytes (blue) are perfused through the well. (B) 2D Microfluidic chip that can be connected to a pump (not shown) and flow tubes. EC (red) are perfused through the system and cells attach to the bottom of the chip. Next, alloreactive lymphocytes (blue) are perfused through the system.
Humanized Rodent Allograft Models
Humanized rodent models can be a great improvement of the more conventional rodent models as these humanized models can overcome some of the translational problems that come with differences among species. The definition of a humanized rodent allograft model is that human immune cells are injected and human tissue is transplanted in immunodeficient mice (108–110). For studying the role of EC in transplantation it is important that both EC and immune cells are of human origin to closely resemble human transplantation conditions. With this assumption, a few interesting models can be mentioned; First, the transplantation of human skin onto immunodeficient mice and subsequent injection of human peripheral blood mononuclear cells (PBMC) (21, 70, 71). This model is suitable for studying human EC and human immune cells in an in vivo condition and especially the administration of immunosuppressive drugs and its effect on the EC—immune cell interaction would be intriguing to study within this model. However, an important limiting factor of this model is that vascularization of skin transplantation is incomparable with solid organ transplants, as skin transplant vascularization is approximately achieved after 48 hours and completed after 8 up to 21 days (97, 98, 111). Also, vascularization in skin allografts is dominated by recipient neoangiogenesis and regression of donor vasculature, in contrast to human solid organ transplants in which the donor vasculature remains largely intact (111). Another interesting model is the transplantation of human aorta segments into immunodeficient mice and subsequent injection of human PBMC (68). As described above, microvascular EC of solid organs greatly vary from macrovascular EC of an aorta segment. Likewise, the aorta model does not allow for studying the interaction and transmigration of immune cells through microvascular graft EC. Combined transplantation of human skin and human coronary artery in immunodeficient mice has also been performed. In this study subsets of immune cells have been injected to study the effect of specific immune cell populations on the allograft (112).
3D Culture Systems: Organs on a Chip and Organoids (on a Chip)
Organ on a Chip
Another promising transition is the switch from 2D to 3D models. In parallel with the upcoming 3R movement—i.e., reduction, refinement, and replacement of animal models—the bioengineering industry has made big steps forward in producing 3D in vitro models that closely resemble organ function, the so called organ-on-a-chip models. The most developed “organ” on a chip is the vessel-on-a-chip (113, 114). In this model a silicon-based—Polydimethylsiloxane (PDMS)—mold is used in which channels are artificially created with a biopsy punch. The channels are then perfused with EC that will grow into 3D vessel-like structures (Figure 2A) (113, 115–118). PDMS-based molds have limitations however, as this material can absorb certain small molecules, including oxygen and drugs, leading to distorted results (119, 120). Coating the PDMS membrane with a lipid-based solution, before adding the EC, has overcome this problem (119). Recently, the introduction of hydrogel-based molds, the addition of surrounding cells (pericytes or SMC) and the use of the 3D printing technique has resulted in even more realistic vessels in which the effect of shear stress, neighboring cells, and extracellular matrix (ECM) can be studied (121–126). Even naturally occurring neo-angiogenesis and sprouting resulting in self-structured vessels has been made possible (124, 127). These self-structured vessels however, are yet in an early stage as perfusion in self-formed vessels and branches cannot always be assured. Depending on the research purpose either the guided vessel formation or the self-structured vessels may be the best model to choose. These vessel-on-a-chip models can be perfused with cell medium, drugs, serum, plasma or even whole blood to study for example, thrombosis and drug pharmacokinetics and -dynamics (Figure 2A) (114, 115, 123, 128–130). Oxygen can also be added to the perfusion fluid (131). One research group designed a vessel-on-a-chip system as a model for xenotransplantation. Microfluidic chips containing microchannels were constructed with the use of PDMS and mold needles. The channels were perfused with porcine aortic endothelial cells (PAEC) and confluency was confirmed with confocal microscopy. Next, PAEC vessels were perfused with human serum. Confocal microscopy and protein assays were used to investigate the effect of the human serum perfusion on PAEC and complement activation (123). 3D vessel-on-a-chip models would also be a great step forward in the research to the role of EC in allograft rejection for example to study (i) the effect of induced hypoxia and reperfusion on EC (ii) the interaction of EC and alloreactive immune cells (iii) the effect of immunosuppressive drugs on EC activation and on transmigrating immune cells, (iv) EC chimerism.
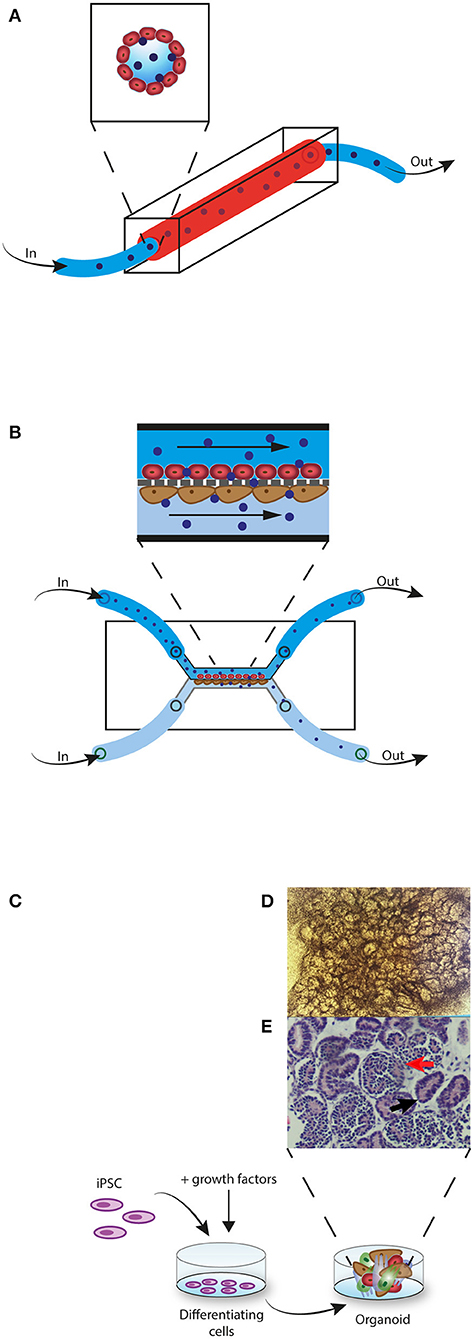
Figure 2. 3D in vitro models. (A) Vessel-on-a-chip model. EC (red) are perfused through a PDMS mold channel. When confluent, alloreactive lymphocytes (blue) are perfused through the vessel. (B) Glomerulus-on-a-chip model. EC (red) are perfused through the upper channel and podocytes (brown) are perfused through the lower channel. The two cell layers are separated by a membrane that imitates the glomerular basement membrane. Next, alloreactive lymphocytes (blue) are perfused through the upper channel. Non-migrated lymphocytes are collected from the upper channel and migrated lymphocytes are collected in the lower channel. (C) Kidney-organoid model. iPSC are induced with growth factors to differentiate into a 3D multicellular structure. (D) Complex structure formation can be monitored with light microscopy. (E) Histological H&E staining of a kidney-organoid shows tubular (black arrow) and glomerular structures (red arrow).
Other than vessels, many organ-on-a-chip models have been generated, including kidney, liver, lung, heart, brain, skin, gut, and lymph nodes (130, 132). Pre-differentiated cells—cell lines or human pluripotent stem cell (hPSC) derived cells—are used in these models. In some of these organ-on-a-chips an endothelial barrier is incorporated in the model and is therefore interesting for studying the role of EC in allograft rejection. For example, a glomerulus on a chip was generated with hPSC-derived podocytes, glomerular microvascular endothelial cells (GMEC) and a glomerular basement membrane (GBM)-like structure (133). Figure 2B shows an example of a glomerulus-on-a-chip model. Also, a 3D vascularized proximal tubulus chip has been established in which a tube formed by proximal tubular epithelial cells (PTEC) and a second tube formed by GMEC are separated by an ECM-like membrane (134). Both glomerulus- and tubulus-on a chip systems have been able to simulate glomerular filtration (133–136). These models would be very interesting in studying the role of EC in transplantation. For example to study (i) how alloreactive immune cells that transmigrate through the endothelium have an effect on the tubular cells or podocytes and by which mechanisms (ii) to discover new drug targets that could interfere with this process (iii) to investigate the effect of currently used immunosuppressive drugs (iv) or to investigate if alloreactive immune cells have a different effect on glomerular EC compared to tubular EC.
The choice for a specific EC cell source in an organ-on-a-chip model comes with certain advantages as well as limitations. Primary EC most closely resemble in vivo conditions, but are limited in culture time as they lose phenotype and functional characteristics after a few passages. Also inter-individual variation occurs within primary cells (137). Immortalized EC cell lines can, in contrast to primary cells, be kept in culture for long term and do not have the issue with between-donor variation. These EC cell lines, however, generally do not resemble well in vivo conditions (137, 138). Circulating progenitor endothelial cells (EPC) have occasionally been used for in vitro differentiation into mature EC, but are limited in their use due to the low yield and the controversy about their identification (139, 140). hPSC have been used for cell differentiation of many different cell lineages, including EC. The ability to expand these stem cells for long term is a great advantage, but a limitation is their immaturity and their phenotypic instability after differentiation (137, 141, 142). Moreover, developed differentiation protocols are generated with the use of a few stem cell lines and application by researchers with other stem cell lines often leads to reproducibility problems.
A new concept of organs-on-a-chip is the connection of individual organ-chips to create a body-on-a-chip (143–145). Especially for drug development and for in vitro modeling of diseases that affect multiple organs, body-on-a-chips would be very useful. The current challenge for this model is to enhance vascularization and innervation of all the organs in the system (143). Also, defining the proportional size of each organ in the system and facilitate maturation of all organs at the same time are remaining challenges (143, 146). Connecting only a few organs instead of creating a whole-body-chip might be sufficient for some research purposes and could reduce some of the described challenges. For studying the role of EC in allograft rejection, it would be interesting to connect a kidney-, liver- or heart-on-a-chip with a lymph-node-on-a-chip and generate vascularization and lymphatic drainage. In cancer research a vascularized and lymphatic drained tumor-on-a-chip has already been developed (147). With such a system, in which the organ-on-chip and lymph-node-on-chip are alloreactive to each other, it would be possible for example, to study the difference between alloreactive T cells that are either activated by the endothelium or activated by DCs in the lymph node and subsequently, if the effect between these two populations within the organ is distinctive. Also, it is well-known that upon damage, EC can detach and circulate through the blood (148, 149). If these circulating endothelial cells (CEC) are also capable of antigen presentation in the circulation or within the lymph node is unknown, but it may be possible to investigate this with such a model as well.
Organoids (on a Chip)
A more complex model of an organ in vitro has been achieved by the establishment of the organoid technology by which hPSC- including induced pluripotent stem cells (iPSC) and embryonic stem cells (ESC)- or adult stem cells (ASC) can be induced to grow into multicellular self-organized 3D organ-like structures (150). Organoids are compared to organs-on-a-chip a more advanced model of true organs as organoids have a higher complexity of (organ) structure and function. Specific signaling molecules of the embryonic development are needed for the differentiation of stem cells into organ-like structures, corresponding to the organ of interest (150–152). Depending on whether hPSC or ASC are used, organoids resemble a fetal-like organ or a matured adult-like organ (151, 153, 154). Other important differences between the various sources of stem cells are: (i) ASC are multipotent and can therefore not be differentiated in all cell types, in contrast to the pluripotent hPSC. (ii) ESC availability is limited as these cells are derived from human embryos, which also gives rise to ethical concerns. (iii) For modeling of genetic diseases the use of iPSC is specifically interesting as iPSC can be derived from patients with the disease of interest (137, 150, 152).
Various types of organoids have been developed, including brain, gut, liver, pancreas, lung, heart, and kidney organoids (150, 152). Figures 2C–E shows an example of kidney organoid formation with iPSC. Diverse platforms for the generation of organoids are used, ranging from culture on a solid ECM base, culturing in suspension with or without ECM proteins, or culturing with an air-liquid interface (151).
For the use of organoids as a platform to study the role of EC in allograft rejection, it is important whether or not EC are present in the organoids. It has been shown that EC can be represented within organoids, but spontaneous development of vascular networks in vitro is absent (155–159). Vascularization has, however, been achieved by the transfer of in vitro grown organoids into immunodeficient mice (152, 157, 158, 160). As animal models are unwanted, it has been suggested that combining the organ-on-a-chip technology with organoids could be an attractive approach to realize in vitro vascularized organoids (161–163). Several attempts to reach this goal have been performed. One study generated kidney organoids on a scaffold in a 3D microfluidic system. This resulted in the outgrowth of organoid-derived EC into a perfusable vascular network within the organoids (164). However, the success of this vascularization and perfusion has been questioned by other researchers (165). Also, vascularization of brain organoids in vitro has been reported but the possibility of in vitro perfusion of the organoid-infiltrating vessels has not been investigated (159). Another reported method to perfuse organoids is the use of 3D printing to create a channel network within cardiac organoids. The channels can be connected to a pump and perfused with oxygenated cell medium. However, the perfusion of HUVEC in the channels to mimic blood vessels resulted in incomplete coverage of the channels (166). Liver organoids with incorporated EC have been generated by co-culturing hPSC-derived pre-hepatic cells together with EC. Although in vitro vascular networks did not occur, hepatic function of the organoids improved by the presence of EC (156–158). This is an intriguing finding and suggests that studying EC function in organoids is worthwhile despite the lack of real vascular networks. Therefore, this model allows the study of the immunogenicity of EC in organoids against alloreactive immune cells by co-culturing alloreactive immune cells with the EC-containing organoids.
Ideally, in vitro vascularized organoid models to study the role of EC in allograft rejection should include organ specific EC. For such models, EC originating from the organoid itself, organ specific primary EC or organ specific EC differentiated from hPSC could be used (159, 164). Organoid vascularization with non-organ specific EC, like HUVEC, may not resemble organ function properly (156–158, 166). For example, glomerular EC have specific characteristics to support filtration which can not be substituted by HUVEC (8). Technical challenges however, could hamper the practical feasibility of such elegant models that include organ specific EC.
Some other limitations of the organoid methodology makes their use as a research model a challenge. First, remaining undifferentiated hPSC within the organoids can transform into tumors/teratomas (167, 168). Second, some organoids, including brain and liver organoids, can be cultured for a long term (up to several months), but other organoids, including kidney organoids, cannot be maintained in culture for more than a few weeks. This could make certain research aims difficult to fulfill (152, 169). Third, the immaturity of hPSC derived organoids could also be a limiting factor (151, 153). Finally, organoids are highly heterogeneous and variation in organoid quality is seen between experiments, between hPSC lines, and between cell batches (169–171).
Despite of these limitations, in vitro perfusable vascularized organoids are a promising research platform to investigate the role of EC in allograft rejection, but optimization of vascularization is needed first. Also, in accordance to the body-on-a-chip development, investigation of connecting multiple organoids-on-a-chip is in progress as well. Such systemic models with various organs represented is a hopeful model for many research areas, including drug development, and transplantation research (162, 172).
Isolation and Characterization of EC for Use in Advanced Experimental Models
Various sources of EC have been used in the above described advanced experimental models. The degree of complexity of isolation and characterization methods differs between various EC sources and may therefore influence the choice for a certain EC source to be used in an advanced experimental model. Primary EC can be commercially purchased or isolated from explanted tissue of biopsy material. Especially in models with disease specific EC, isolation from patient derived tissue is preferred and one should consider which method is most appropriate.
Isolation of conventionally used HUVEC is relatively easy compared to microvascular EC (173, 174). The inner lining of umbilical veins is enzymatically digested with collagenase treatment or mechanically scraped and results in a substantial yield of EC and relatively low contamination with other cell types (173, 175). Isolation of microvascular EC from solid organs requires enzymatic digestion of tissue resulting in a lower degree of EC purity and therefore subsequential EC purification is needed (174, 176, 177). Within an organ, different types of microvascular EC can be present and it may be useful to separate those depending on the research question (8, 178). For example, within the kidney, separation of glomerular and tubulo-interstitial EC can be performed by collagenase treatment of renal cortex and next passing the obtained cell suspension through different pore size sieves, which will separate glomerular EC from tubulointerstitial EC (174, 179). After enzymatic digestion, the obtained cell suspension is often added to a gelatin-coated culture flask to allow for EC adhesion, expansion and purification. Non-adherent cell types are removed upon the first cell culture medium refreshment (173, 175–177, 179). EC can be visually recognized by their typical cobblestone appearance, which allows for EC purification by manual weeding (173–175). Another method that can be used to remove fibroblasts and mesenchymal cell contamination is with a DiI-conjugated acetylated low-density lipoproteins (DiI-AC-LDL) assay. EC take up DiI-AC-LDL whereas fibroblasts and mesenchymal cells do not. After incubation of cell cultures with DiI-AC-LDL, uptake assessment and EC purification can be performed by fluorescence microscopy along with manual weeding or by flow cytometric cell sorting (176, 179). A third EC purification method is based on positive or negative cell selection with the use of magnetic beads, for which most often anti-platelet endothelial cell adhesion molecule (PECAM) beads, anti-von Willebrand factor (vWF) beads or Ulex europaeus agglutinin-1 (UEA-1) beads are used. This magnetic purification step can be performed immediately after enzymatic tissue digestion before the cells are added to a culture flask or the purification can be done or repeated after a certain period of cell culture (174, 176, 177, 179). The obtained primary EC can be used in an experimental model or can be transformed into immortalized EC lines by viral transduction and subcloning. Immortalized EC should then be assessed for the persistence of EC characteristics, as the immortalization process often leads to diminished expression of EC membrane molecules (180–182).
EC can also be acquired through differentiation of hPSC. The protocol for EC differentiation most often includes bone morphogenic protein-4 (BMP4), fibroblast growth factor 2 (FGF2) and vascular endothelial growth factor (VEGF) (141, 142, 159, 183, 184). During the differentiation process, it may be required to perform a purification step based on the expression of EC specific markers, for example PECAM, VE-cadherin, kinase insert domain receptor (KDR) and neuropilin-1 (NPR1) are used (141, 142, 184). However, it has been reported that the generation of a high purity EC population is possible without cell sorting (159, 183). Successful EC differentiation can subsequently be confirmed by assessment of general EC characteristics, including cobblestone appearance, DiI-AC-LDL uptake and expression of PECAM, VE-cadherin, and vWf (141, 142, 183).
Conclusion
We have provided an overview of innovative research models that will greatly improve the study of EC in solid organ transplantation. The first step in improving the relevance of research models is the transition from 2D static to 2D dynamic culture models and from conventional animal models to humanized rodent models. Further progress can be made with the implementation of 3D in vitro research models. Substantial translational limitations of both 2D models and animal models can be overcome with the use of these models. Implementation of the 3D models will therefore lead to increased knowledge about the role of EC in allograft rejection and to the discovery of new targets for drug development. Moreover, new insights about the effect of currently used immunosuppressive drugs on the EC- immune system interaction can be obtained.
Although some 3D models are still in early developmental stage, their upcoming use in various research fields will soon redress current restraints. Other research areas focusing on EC will also benefit by using these models, for example the study of EC in cardiovascular diseases. Further, the proposed models are likewise feasible for wider applications, including transplantation research in general and other research areas (e.g., immunology, cancer, and pharmacology research).
Author Contributions
DP writing original draft. DP, MH, DH, and CB editing. MH, DH, and CB supervision. All authors contributed to the article and approved the submitted version.
Conflict of Interest
The authors declare that the research was conducted in the absence of any commercial or financial relationships that could be construed as a potential conflict of interest.
References
1. Abrahimi P, Liu R, Pober JS. Blood vessels in allotransplantation. Am J Transplant. (2015) 15:1748–54. doi: 10.1111/ajt.13242
2. Jaehyuk C, David RE, Kian Peng K, Stephen LS, Jordan SP. T Lymphocyte–endothelial cell interactions. Annu Rev Immunol. (2004) 22:683–709. doi: 10.1146/annurev.immunol.22.012703.104639
3. Félétou M. The endothelium, part i: multiple functions of the endothelial cells – focus on endothelium-derived vasoactive mediators. Colloq Ser Integr Syst Physiol Mol Funct. (2011) 3:1–306. doi: 10.4199/C00031ED1V01Y201105ISP019
4. Kariya T, Ueta H, Xu X-D, Koga D, Ezaki T, Yu E, et al. Direct evidence for activated CD8+ T cell transmigration across portal vein endothelial cells in liver graft rejection. J Gastroenterol. (2016) 51:985–98. doi: 10.1007/s00535-016-1169-1
5. Valujskikh A, Heeger PS. Emerging roles of endothelial cells in transplant rejection. Curr Opin Immunol. (2003) 15:493–8. doi: 10.1016/S0952-7915(03)00110-9
6. Vestweber D. How leukocytes cross the vascular endothelium. Nat Rev Immunol. (2015) 15:692–704. doi: 10.1038/nri3908
7. Muller WA. How endothelial cells regulate transmigration of leukocytes in the inflammatory response. Am J Pathol. (2014) 184:886–96. doi: 10.1016/j.ajpath.2013.12.033
8. Jourde-Chiche N, Fakhouri F, Dou L, Bellien J, Burtey S, Frimat M, et al. Endothelium structure and function in kidney health and disease. Nat Rev Nephrol. (2019) 15:87–108. doi: 10.1038/s41581-018-0098-z
9. Jassem W, Koo DDH, Cerundolo L, Rela M, Heaton ND, Fuggle SV. Leukocyte infiltration and inflammatory antigen expression in cadaveric and living-donor livers before transplant1. Transplantation. (2003) 75:2001–7. doi: 10.1097/01.TP.0000061605.30685.03
10. Koo DD, Welsh KI, McLaren AJ, Roake JA, Morris PJ, Fuggle SV. Cadaver versus living donor kidneys: impact of donor factors on antigen induction before transplantation. Kidney Int. (1999) 56:1551–9. doi: 10.1046/j.1523-1755.1999.00657.x
11. Baan C, van Gelder T, Peeters A, Mol W, Niesters H, Weimar W, et al. Living kidney donors and hypoxia-inducible factor-1α. Transplantation. (2003) 75:570–1. doi: 10.1097/01.TP.0000034241.55602.AD
12. Salvadori M, Rosso G, Bertoni E. Update on ischemia-reperfusion injury in kidney transplantation: pathogenesis and treatment. World J Transplant. (2015) 5:52–67. doi: 10.5500/wjt.v5.i2.52
13. Cardinal H, Dieudé M, Hébert MJ. Endothelial dysfunction in kidney transplantation. Front Immunol. (2018) 9:1130. doi: 10.3389/fimmu.2018.01130
14. Cross AR, Glotz D, Mooney N. The role of the endothelium during antibody-mediated rejection: from victim to accomplice. Front Immunol. (2018) 9:106. doi: 10.3389/fimmu.2018.00106
15. Al-Lamki RS, Bradley JR, Pober JS. Endothelial cells in allograft rejection. Transplantation. (2008) 86:1340–8. doi: 10.1097/TP.0b013e3181891d8b
16. Collins AB, Schneeberger EE, Pascual MA, Saidman SL, Williams WW, Tolkoff-Rubin N, et al. Complement activation in acute humoral renal allograft rejection: diagnostic significance of C4d deposits in peritubular capillaries. J Am Soc Nephrol. (1999) 10:2208–14.
17. Jeannet M, Pinn VW, Flax MH, Winn HJ, Russell PS. Humoral antibodies in renal allotransplantation in man. N Engl J Med. (1970) 282:111–7. doi: 10.1056/NEJM197001152820301
18. Nankivell BJ, Alexander SI. Rejection of the kidney allograft. N Engl J Med. (2010) 363:1451–62. doi: 10.1056/NEJMra0902927
19. Taflin C, Charron D, Glotz D, Mooney N. Immunological function of the endothelial cell within the setting of organ transplantation. Immunol Lett. (2011) 139:1–6. doi: 10.1016/j.imlet.2011.04.014
20. Krupnick AS, Kreisel D, Popma SH, Balsara KR, Szeto WY, Krasinskas AM, et al. Mechanism of T cell-mediated endothelial apoptosis. Transplantation. (2002) 74:871–6. doi: 10.1097/00007890-200209270-00022
21. Shiao SL, McNiff JM, Pober JS. Memory T cells and their costimulators in human allograft injury. J Immunol. (2005) 175:4886–96. doi: 10.4049/jimmunol.175.8.4886
22. Dengler TJ, Pober JS. Human vascular endothelial cells stimulate memory but not naive CD8 <sup> + </sup> T cells to differentiate into CTL retaining an early activation phenotype. J Immunol. (2000) 164:5146–55. doi: 10.4049/jimmunol.164.10.5146
23. Denton MD, Davis SF, Baum MA, Melter M, Reinders ME, Exeni A, et al. The role of the graft endothelium in transplant rejection: evidence that endothelial activation may serve as a clinical marker for the development of chronic rejection. Pediatr Transplant. (2000) 4:252–60. doi: 10.1034/j.1399-3046.2000.00031.x
24. Koenig A, Chen C-C, Marçais A, Barba T, Mathias V, Sicard A, et al. Missing self triggers NK cell-mediated chronic vascular rejection of solid organ transplants. Nat Commun. (2019) 10:5350. doi: 10.1038/s41467-019-13113-5
25. Bruneau S, Wedel J, Fakhouri F, Nakayama H, Boneschansker L, Irimia D, et al. Translational implications of endothelial cell dysfunction in association with chronic allograft rejection. Pediatr Nephrol. (2016) 31:41–51. doi: 10.1007/s00467-015-3094-6
26. Vanhoutte PM. Regeneration of the endothelium in vascular injury. Cardiovasc Drugs Ther. (2010) 24:299–303. doi: 10.1007/s10557-010-6257-5
27. McDonald AI, Shirali AS, Aragón R, Ma F, Hernandez G, Vaughn DA, et al. Endothelial regeneration of large vessels is a biphasic process driven by local cells with distinct proliferative capacities. Cell Stem Cell. (2018) 23:210–25.e6. doi: 10.1016/j.stem.2018.07.011
28. Koopmans M, Kremer Hovinga IC, Baelde HJ, de Heer E, Bruijn JA, Bajema IM. Endothelial chimerism in transplantation: Looking for needles in a haystack. Transplantation. (2006) 82(Suppl. 1):S25–9. doi: 10.1097/01.tp.0000231446.41051.98
29. Xu W, Baelde HJ, Lagaaij EL, De Heer E, Paul LC, Bruijn JA. Endothelial cell chimerism after renal transplantation in a rat model. Transplantation. (2002) 74:1316–20. doi: 10.1097/00007890-200211150-00020
30. Lagaaij EL, Cramer-Knijnenburg GF, van Kemenade FJ, van Es LA, Bruijn JA, van Krieken JH. Endothelial cell chimerism after renal transplantation and vascular rejection. Lancet. (2001) 357:33–7. doi: 10.1016/S0140-6736(00)03569-8
31. Pons JA, Yélamos J, Ramírez P, Oliver-Bonet M, Sánchez A, Rodríguez-Gago M, et al. Endothelial cell chimerism does not influence allograft tolerance in liver transplant patients after withdrawal of immunosuppression. Transplantation. (2003) 75:1045–7. doi: 10.1097/01.TP.0000058472.71775.7D
32. van Poelgeest EP, Baelde HJ, Lagaaij EL, Sijpkens YVOWJ, de Heer E, Bruijn JANA, et al. Endothelial cell chimerism occurs more often and earlier in female than in male recipients of kidney transplants. Kidney Int. (2005) 68:847–53. doi: 10.1111/j.1523-1755.2005.00466.x
33. Chen CC, Pouliquen E, Broisat A, Andreata F, Racapé M, Bruneval P, et al. Endothelial chimerism and vascular sequestration protect pancreatic islet grafts from antibody-mediated rejection. J Clin Invest. (2018) 128:219–32. doi: 10.1172/JCI93542
34. Tanaka Y, Haga H, Egawa H, Okuno T, Miyagawa-Hayashino A, Tsuruyama T, et al. Intragraft expression of recipient-type ABO blood group antigens: long-term follow-up and histological features after liver transplantation. Liver Transpl. (2005) 11:547–54. doi: 10.1002/lt.20415
35. Epperson DE, Pober JS. Antigen-presenting function of human endothelial cells. Direct activation of resting CD8 T cells. J Immunol. (1994) 153:5402–12.
36. Savage CO, Hughes CC, McIntyre BW, Picard JK, Pober JS. Human CD4+ T cells proliferate to HLA-DR+ allogeneic vascular endothelium. Identification of accessory interactions. Transplantation. (1993) 56:128–34. doi: 10.1097/00007890-199307000-00024
37. Denton MD, Geehan CS, Alexander SI, Sayegh MH, Briscoe DM. Endothelial cells modify the costimulatory capacity of transmigrating leukocytes and promote CD28-mediated CD4(+) T cell alloactivation. J Exp Med. (1999) 190:555–66. doi: 10.1084/jem.190.4.555
38. Lahdou I, Engler C, Mehrle S, Daniel V, Sadeghi M, Opelz G, et al. Role of human corneal endothelial cells in T-cell–mediated alloimmune attack in vitro. Invest Ophthalmol Vis Sci. (2014) 55:1213–21. doi: 10.1167/iovs.13-11930
39. Page C, Thompson C, Yacoub M, Rose M. Human endothelial stimulation of allogeneic T cells via a CTLA-4 independent pathway. Transpl Immunol. (1994) 2:342–7. doi: 10.1016/0966-3274(94)90013-2
40. Valujskikh A, Lantz O, Celli S, Matzinger P, Heeger PS. Cross-primed CD8+ T cells mediate graft rejection via a distinct effector pathway. Nat Immunol. (2002) 3:844–51. doi: 10.1038/ni831
41. Biedermann BC, Pober JS. Human endothelial cells induce and regulate cytolytic T cell differentiation. J Immunol. (1998) 161:4679–87.
42. Kapessidou Y, Habran C, Buonocore S, Flamand V, Barvais L, Goldman M, et al. The replacement of graft endothelium by recipient-type cells conditions allograft rejection mediated by indirect pathway CD4+ T cells. Transplantation. (2006) 82:582–91. doi: 10.1097/01.tp.0000184444.93108.d1
43. Manavalan JS, Kim-Schulze S, Scotto L, Naiyer AJ, Vlad G, Colombo PC, et al. Alloantigen specific CD8+CD28- FOXP3+ T suppressor cells induce ILT3+ ILT4+ tolerogenic endothelial cells, inhibiting alloreactivity. Int Immunol. (2004) 16:1055–68. doi: 10.1093/intimm/dxh107
44. Kunitomi A, Hori T, Imura A, Uchiyama T. Vascular endothelial cells provide T cells with costimulatory signals via the OX40/gp34 system. J Leukoc Biol. (2000) 68:111–8. doi: 10.1189/jlb.68.1.111
45. Huang EH, Gabler DM, Krecic ME, Gerber N, Ferguson RM, Orosz CG. Differential effects of gallium nitrate on T lymphocyte and endothelial cell activation. Transplantation. (1994) 58:1216–22. doi: 10.1097/00007890-199412150-00014
46. Lim WC, Olding M, Healy E, Millar TM. Human endothelial cells modulate CD4(+) T cell populations and enhance regulatory T cell suppressive capacity. Front Immunol. (2018) 9:565. doi: 10.3389/fimmu.2018.00565
47. Taflin C, Favier B, Baudhuin J, Savenay A, Hemon P, Bensussan A, et al. Human endothelial cells generate Th17 and regulatory T cells under inflammatory conditions. Proc Natl Acad Sci USA. (2011) 108:2891–6. doi: 10.1073/pnas.1011811108
48. Klingenberg R, Autschbach F, Gleissner C, Giese T, Wambsganss N, Sommer N, et al. Endothelial inducible costimulator ligand expression is increased during human cardiac allograft rejection and regulates endothelial cell-dependent allo-activation of CD8+ T cells in vitro. Eur J Immunol. (2005) 35:1712–21. doi: 10.1002/eji.200425727
49. Kreisel D, Krupnick AS, Gelman AE, Engels FH, Popma SH, Krasinskas AM, et al. Non-hematopoietic allograft cells directly activate CD8+ T cells and trigger acute rejection: an alternative mechanism of allorecognition. Nat Med. (2002) 8:233–9. doi: 10.1038/nm0302-233
50. Lion J, Taflin C, Cross AR, Robledo-Sarmiento M, Mariotto E, Savenay A, et al. HLA class II antibody activation of endothelial cells promotes Th17 and disrupts regulatory T lymphocyte expansion. Am J Transplant. (2016) 16:1408–20. doi: 10.1111/ajt.13644
51. Chen WJ, Hu XF, Yan M, Zhang WY, Mao XB, Shu YW. Human umbilical vein endothelial cells promote the inhibitory activation of CD4(+)CD25(+)Foxp3(+) regulatory T cells via PD-L1. Atherosclerosis. (2016) 244:108–12. doi: 10.1016/j.atherosclerosis.2015.11.002
52. Werner I, Bogert NV, Stock UA, Moritz A, Beiras-Fernandez A. Dose/time-dependent modulation of the endothelial function through induction agents: non-depleting versus depleting agents. Transplant Proc. (2014) 46:2953–6. doi: 10.1016/j.transproceed.2014.06.055
53. He S, Li M, Ma X, Lin J, Li D. CD4+CD25+Foxp3+ regulatory T cells protect the proinflammatory activation of human umbilical vein endothelial cells. Arterioscler Thromb Vasc Biol. (2010) 30:2621–30. doi: 10.1161/ATVBAHA.110.210492
54. Suárez Y, Shepherd BR, Rao DA, Pober JS. Alloimmunity to human endothelial cells derived from cord blood progenitors. J Immunol. (2007) 179:7488–96. doi: 10.4049/jimmunol.179.11.7488
55. Cross AR, Lion J, Poussin K, Assayag M, Taupin JL, Glotz D, et al. HLA-DQ alloantibodies directly activate the endothelium and compromise differentiation of FoxP3 <sup>high</sup> regulatory T lymphocytes. Kidney Int. (2019) 96:689–98. doi: 10.1016/j.kint.2019.04.023
56. Racape M, Sykes G, Hidalgo LG. OR4 Development of an in vitro model of human antibody mediated rejection. Hum Immunol. (2017) 78:4–5. doi: 10.1016/j.humimm.2017.06.010
57. Jane-Wit D, Manes TD, Yi T, Qin L, Clark P, Kirkiles-Smith NC, et al. Alloantibody and complement promote T cell-mediated cardiac allograft vasculopathy through noncanonical nuclear factor-κB signaling in endothelial cells. Circulation. (2013) 128:2504–16. doi: 10.1161/CIRCULATIONAHA.113.002972
58. Valenzuela NM, Thomas KA, Mulder A, Parry GC, Panicker S, Reed EF. Complement-mediated enhancement of monocyte adhesion to endothelial cells by HLA antibodies, and blockade by a specific inhibitor of the classical complement cascade, TNT003. Transplantation. (2017) 101:1559–72. doi: 10.1097/TP.0000000000001486
59. Delville M, Lamarthée B, Pagie S, See SB, Rabant M, Burger C, et al. Early acute microvascular kidney transplant rejection in the absence of anti-HLA antibodies is associated with preformed IgG antibodies against diverse glomerular endothelial cell antigens. J Am Soc Nephrol. (2019) 30:692–709. doi: 10.1681/ASN.2018080868
60. Roth SJ, Carr MW, Rose SS, Springer TA. Characterization of transendothelial chemotaxis of T lymphocytes. J Immunol Methods. (1995) 188:97–116. doi: 10.1016/0022-1759(95)00208-1
61. Lion J, Burbach M, Cross A, Poussin K, Taflin C, Kaveri S, et al. Endothelial cell amplification of regulatory T cells is differentially modified by immunosuppressors and intravenous immunoglobulin. Front Immunol. (2017) 8:1761. doi: 10.3389/fimmu.2017.01761
62. Murphy LLS, Hughes CCW. Endothelial cells stimulate T cell NFAT nuclear translocation in the presence of cyclosporin A: involvement of the wnt/glycogen synthase kinase-3β pathway. J Immunol. (2002) 169:3717–25. doi: 10.4049/jimmunol.169.7.3717
63. Blaheta RA, Leckel K, Wittig B, Zenker D, Oppermann E, Harder S, et al. Inhibition of endothelial receptor expression and of T-cell ligand activity by mycophenolate mofetil. Transplant Immunol. (1998) 6:251–9. doi: 10.1016/S0966-3274(98)80015-4
64. Olejarz W, Bryk D, Zapolska-Downar D, Malecki M, Stachurska A, Sitkiewicz D. Mycophenolic acid attenuates the tumour necrosis factor-α-mediated proinflammatory response in endothelial cells by blocking the MAPK/NF-κB and ROS pathways. Eur J Clin Invest. (2014) 44:54–64. doi: 10.1111/eci.12191
65. Suzuki M, Hashizume M, Yoshida H, Mihara M. Anti-inflammatory mechanism of tocilizumab, a humanized anti-IL-6R antibody: effect on the expression of chemokine and adhesion molecule. Rheumatol Int. (2009) 30:309–15. doi: 10.1007/s00296-009-0953-0
66. Huang Y, Liu Z, Huang H, Liu H, Li L. Effects of mycophenolic acid on endothelial cells. Int Immunopharmacol. (2005) 5:1029–39. doi: 10.1016/j.intimp.2005.01.015
67. Blaheta RA, Hailer NP, Brude N, Wittig B, Leckel K, Oppermann E, et al. In vitro analysis of verapamil-induced immunosuppression: potent inhibition of T cell motility and lymphocytic transmigration through allogeneic endothelial cells1. Transplantation. (2000) 69:588–97. doi: 10.1097/00007890-200002270-00021
68. Lorber MI, Wilson JH, Robert ME, Schechner JS, Kirkiles N, Qian H-Y, et al. Human allogeneic vascular rejection after arterial transplantation and peripheral lymphoid reconstitution in severe combined immunodeficient mice1. Transplantation. (1999) 67:897–903. doi: 10.1097/00007890-199903270-00018
69. Dragun D, Böhler T, Nieminen-Kelhä M, Waiser J, Schneider W, Haller H, et al. FTY720-induced lymphocyte homing modulates post-transplant preservation/reperfusion injury. Kidney Int. (2004) 65:1076–83. doi: 10.1111/j.1523-1755.2004.00478.x
70. Murray AG, Petzelbauer P, Hughes CC, Costa J, Askenase P, Pober JS. Human T-cell-mediated destruction of allogeneic dermal microvessels in a severe combined immunodeficient mouse. Proc Natl Acad Sci USA. (1994) 91:9146–50. doi: 10.1073/pnas.91.19.9146
71. Murray AG, Schechner JS, Epperson DE, Sultan P, McNiff JM, Hughes CC, et al. Dermal microvascular injury in the human peripheral blood lymphocyte reconstituted-severe combined immunodeficient (HuPBL-SCID) mouse/skin allograft model is T cell mediated and inhibited by a combination of cyclosporine and rapamycin. Am J Pathol. (1998) 153:627–38. doi: 10.1016/S0002-9440(10)65604-0
72. Kreisel D, Krupnick AS, Balsara KR, Riha M, Gelman AE, Popma SH, et al. Mouse vascular endothelium activates CD8 <sup> + </sup> T lymphocytes in a B7-dependent fashion. J Immunol. (2002) 169:6154–61. doi: 10.4049/jimmunol.169.11.6154
73. Fukami N, Ramachandran S, Narayanan K, Liu W, Nath DS, Jendrisak M, et al. Mechanism of accommodation in a sensitized human leukocyte antigen transgenic murine cardiac transplant model. Transplantation. (2012) 93:364–72. doi: 10.1097/TP.0b013e3182406a6b
74. von Rossum A, Rey K, Enns W, Manku S, Cheema R, MacEwan GE, et al. Graft-derived IL-6 amplifies proliferation and survival of effector T cells that drive alloimmune-mediated vascular rejection. Transplantation. (2016) 100:2332–41. doi: 10.1097/TP.0000000000001227
75. Hamano K, Ito H, Shirasawa B, Gohra H, Katoh T, Fujimura Y, et al. Correlations among expression of intercellular adhesion molecule 1, cellular infiltration, and coronary arteriosclerosis during chronic rejection using the rat heart transplantation model. Eur Surg Res. (1998) 30:235–42. doi: 10.1159/000008582
76. Tang ML, Hale LP, Steeber DA, Tedder TF. L-selectin is involved in lymphocyte migration to sites of inflammation in the skin: delayed rejection of allografts in L-selectin-deficient mice. J Immunol. (1997) 158:5191–9.
77. Rothermel AL, Wang Y, Schechner J, Mook-Kanamori B, Aird WC, Pober JS, et al. Endothelial cells present antigens in vivo. BMC Immunol. (2004) 5:5. doi: 10.1186/1471-2172-5-5
78. Tan PH, Chan C, Xue SA, Dong R, Ananthesayanan B, Manunta M, et al. Phenotypic and functional differences between human saphenous vein (HSVEC) and umbilical vein (HUVEC) endothelial cells. Atherosclerosis. (2004) 173:171–83. doi: 10.1016/j.atherosclerosis.2003.12.011
79. Muczynski KA, Ekle DM, Coder DM, Anderson SK. Normal human kidney HLA-DR–expressing renal microvascular endothelial cells: characterization, isolation, and regulation of MHC Class II expression. J Am Soc Nephrol. (2003) 14:1336–48. doi: 10.1097/01.ASN.0000061778.08085.9F
80. Page C, Rose M, Yacoub M, Pigott R. Antigenic heterogeneity of vascular endothelium. Am J Pathol. (1992) 141:673–83.
81. Nolan DJ, Ginsberg M, Israely E, Palikuqi B, Poulos MG, James D, et al. Molecular signatures of tissue-specific microvascular endothelial cell heterogeneity in organ maintenance and regeneration. Dev Cell. (2013) 26:204–19. doi: 10.1016/j.devcel.2013.06.017
82. Kinjo T, Takashi M, Miyake K, Nagura H. Phenotypic heterogeneity of vascular endothelial cells in the human kidney. Cell Tissue Res. (1989) 256:27–34. doi: 10.1007/BF00224715
83. Chi JT, Chang HY, Haraldsen G, Jahnsen FL, Troyanskaya OG, Chang DS, et al. Endothelial cell diversity revealed by global expression profiling. Proc Natl Acad Sci USA. (2003) 100:10623–8. doi: 10.1073/pnas.1434429100
84. Canet E, Devallière J, Gérard N, Karam G, Giral M, Charreau B, et al. Profiling posttransplant circulating antibodies in kidney transplantation using donor endothelial cells. Transplantation. (2012) 93:257–64. doi: 10.1097/TP.0b013e31823ec0ec
85. Kloc M, Kubiak JZ, Li XC, Ghobrial RM. Pericytes, microvasular dysfunction, and chronic rejection. Transplantation. (2015) 99:658–67. doi: 10.1097/TP.0000000000000648
86. Dragovich MA, Genemaras K, Dailey HL, Jedlicka S, Frank Zhang X. Dual regulation of l-selectin-mediated leukocyte adhesion by endothelial surface glycocalyx. Cell Mol Bioeng. (2016) 10:102–13. doi: 10.1007/s12195-016-0463-6
87. Yilmaz O, Afsar B, Ortiz A, Kanbay M. The role of endothelial glycocalyx in health and disease. Clin Kidney J. (2019) 12:611–9. doi: 10.1093/ckj/sfz042
88. Li YSJ, Haga JH, Chien S. Molecular basis of the effects of shear stress on vascular endothelial cells. J Biomech. (2005) 38:1949–71. doi: 10.1016/j.jbiomech.2004.09.030
89. Chien S. Effects of disturbed flow on endothelial cells. Ann Biomed Eng. (2008) 36:554–62. doi: 10.1007/s10439-007-9426-3
90. Choo JK, Seebach JD, Nickeleit V, Shimizu A, Lei H, Sachs DH, et al. Species differences in the expression of major histocompatibility complex class II antigens on coronary artery endothelium: implications for cell-mediated xenoreactivity1. Transplantation. (1997) 64:1315–22. doi: 10.1097/00007890-199711150-00014
91. Chinwalla AT, Cook LL, Delehaunty KD, Fewell GA, Fulton LA, Fulton RS, et al. Initial sequencing and comparative analysis of the mouse genome. Nature. (2002) 420:520–62. doi: 10.1038/nature01262
92. Springer MS, Murphy WJ. Mammalian evolution and biomedicine: new views from phylogeny. Biol Rev. (2007) 82:375–92. doi: 10.1111/j.1469-185X.2007.00016.x
93. Swearengen JR. Choosing the right animal model for infectious disease research. Anim Models Exp Med. (2018) 1:100–8. doi: 10.1002/ame2.12020
94. Dehoux JP, Gianello P. The importance of large animal models in transplantation. Front Biosci. (2007) 12:4864–80. doi: 10.2741/2434
95. Martignoni M, Groothuis GMM, de Kanter R. Species differences between mouse, rat, dog, monkey and human CYP-mediated drug metabolism, inhibition and induction. Expert Opin Drug Metab Toxicol. (2006) 2:875–94. doi: 10.1517/17425255.2.6.875
96. Mordenti J. Dosage regimen design for pharmaceutical studies conducted in animals. J Pharm Sci. (1986) 75:852–7. doi: 10.1002/jps.2600750906
98. Haramati J, Soppe C, Zúñiga MC. A rapid method for skin grafting in mice that greatly enhances graft and recipient survival. Transplantation. (2007) 84:1364–7. doi: 10.1097/01.tp.0000289994.13139.f4
99. Westhofen S, Jelinek M, Dreher L, Biermann D, Martin J, Vitzhum H, et al. The heterotopic heart transplantation in mice as a small animal model to study mechanical unloading – Establishment of the procedure, perioperative management and postoperative scoring. PLoS ONE. (2019) 14:e0214513. doi: 10.1371/journal.pone.0214513
100. Chong AS, Alegre ML, Miller ML, Fairchild RL. Lessons and limits of mouse models. Cold Spring Harb Perspect Med. (2013) 3:a015495. doi: 10.1101/cshperspect.a015495
101. Conant CG, Schwartz MA, Ionescu-Zanetti C. Well plate—coupled microfluidic devices designed for facile image-based cell adhesion and transmigration assays. J Biomol Screen. (2009) 15:102–6. doi: 10.1177/1087057109353789
102. Chau L, Doran M, Cooper-White J. A novel multishear microdevice for studying cell mechanics. Lab Chip. (2009) 9:1897–902. doi: 10.1039/b823180j
103. Ostrowski MA, Huang NF, Walker TW, Verwijlen T, Poplawski C, Khoo AS, et al. Microvascular endothelial cells migrate upstream and align against the shear stress field created by impinging flow. Biophys J. (2014) 106:366–74. doi: 10.1016/j.bpj.2013.11.4502
104. Yoshida N, Yoshikawa T, Nakamura Y, Takenaka S, Sakamoto K, Manabe H, et al. Methylprednisolone inhibits neutrophil-endothelial cell interactions induced by interleukin-1beta under flow conditions. Life Sci. (1997) 60:2341–7. doi: 10.1016/S0024-3205(97)00290-7
105. Cinamon G, Grabovsky V, Winter E, Franitza S, Feigelson S, Shamri R, et al. Novel chemokine functions in lymphocyte migration through vascular endothelium under shear flow. J Leukoc Biol. (2001) 69:860–6. doi: 10.1189/jlb.69.6.860
106. Tovar-Lopez F, Thurgood P, Gilliam C, Nguyen N, Pirogova E, Khoshmanesh K, et al. A microfluidic system for studying the effects of disturbed flow on endothelial cells. Front Bioeng Biotechnol. (2019) 7:81. doi: 10.3389/fbioe.2019.00081
107. Chen L-J, Raut B, Nagai N, Abe T, Kaji H. Prototyping a versatile two-layer multi-channel microfluidic device for direct-contact cell-vessel co-culture. Micromachines. (2020) 11:79. doi: 10.3390/mi11010079
108. Brehm MA, Shultz LD. Human allograft rejection in humanized mice: a historical perspective. Cell Mol Immunol. (2012) 9:225–31. doi: 10.1038/cmi.2011.64
109. Nicole CW, Laurie LK, Sonal J, Ken-Edwin A, Dale LG, Michael AB, et al. Humanized mouse models of clinical disease. Annu Rev Pathol Mech Dis. (2017) 12:187–215. doi: 10.1146/annurev-pathol-052016-100332
110. Hogenes M, Huibers M, Kroone C, de Weger R. Humanized mouse models in transplantation research. Transplant Rev. (2014) 28:103–10. doi: 10.1016/j.trre.2014.02.002
111. Capla JM, Ceradini DJ, Tepper OM, Callaghan MJ, Bhatt KA, Galiano RD, et al. Skin graft vascularization involves precisely regulated regression and replacement of endothelial cells through both angiogenesis and vasculogenesis. Plast Reconstr Surg. (2006) 117:836–44. doi: 10.1097/01.prs.0000201459.91559.7f
112. Kirkiles-Smith NC, Harding MJ, Shepherd BR, Fader SA, Yi T, Wang Y, et al. Development of a humanized mouse model to study the role of macrophages in allograft injury. Transplantation. (2009) 87:189–97. doi: 10.1097/TP.0b013e318192e05d
113. Hu C, Chen Y, Tan MJA, Ren K, Wu H. Microfluidic technologies for vasculature biomimicry. Analyst. (2019) 144:4461–71. doi: 10.1039/C9AN00421A
114. Caballero D, Blackburn SM, de Pablo M, Samitier J, Albertazzi L. Tumour-vessel-on-a-chip models for drug delivery. Lab Chip. (2017) 17:3760–71. doi: 10.1039/C7LC00574A
115. Mathur T, Singh KA, NK RP, Tsai SH, Hein TW, Gaharwar AK, et al. Organ-on-chips made of blood: endothelial progenitor cells from blood reconstitute vascular thromboinflammation in vessel-chips. Lab Chip. (2019) 19:2500–11. doi: 10.1039/C9LC00469F
116. Kim J, Chung M, Kim S, Jo DH, Kim JH, Jeon NL. Engineering of a Biomimetic Pericyte-Covered 3D Microvascular Network. PLoS ONE. (2015) 10:e0133880. doi: 10.1371/journal.pone.0133880
117. Haase K, Gillrie MR, Hajal C, Kamm RD. Pericytes contribute to dysfunction in a human 3D model of placental microvasculature through VEGF-Ang-Tie2 signaling. Adv Sci. (2019) 6:1900878. doi: 10.1002/advs.201900878
118. Abdelgawad M, Wu C, Chien WY, Geddie WR, Jewett MAS, Sun Y. A fast and simple method to fabricate circular microchannels in polydimethylsiloxane (PDMS). Lab Chip. (2011) 11:545–51. doi: 10.1039/C0LC00093K
119. van Meer BJ, de Vries H, Firth KSA, van Weerd J, Tertoolen LGJ, Karperien HBJ, et al. Small molecule absorption by PDMS in the context of drug response bioassays. Biochem Biophys Res Commun. (2017) 482:323–8. doi: 10.1016/j.bbrc.2016.11.062
120. Kuncova-Kallio J, Kallio PJ. PDMS and its Suitability for Analytical Microfluidic Devices. Conf Proc IEEE Eng Med Biol Soc. (2006) 2006:2486–9. doi: 10.1109/IEMBS.2006.260465
121. Schöneberg J, De Lorenzi F, Theek B, Blaeser A, Rommel D, Kuehne AJC, et al. Engineering biofunctional in vitro vessel models using a multilayer bioprinting technique. Sci Rep. (2018) 8:10430. doi: 10.1038/s41598-018-28715-0
123. Sfriso R, Zhang S, Bichsel CA, Steck O, Despont A, Guenat OT, et al. 3D artificial round section micro-vessels to investigate endothelial cells under physiological flow conditions. Sci Rep. (2018) 8:5898. doi: 10.1038/s41598-018-24273-7
124. Lee E, Takahashi H, Pauty J, Kobayashi M, Kato K, Kabara M, et al. A 3D in vitro pericyte-supported microvessel model: visualisation and quantitative characterisation of multistep angiogenesis. J Mater Chem B. (2018) 6:1085–94. doi: 10.1039/C7TB03239K
125. Miri AK, Mostafavi E, Khorsandi D, Hu S-K, Malpica M, Khademhosseini A. Bioprinters for organs-on-chips. Biofabrication. (2019) 11:042002. doi: 10.1088/1758-5090/ab2798
126. Campisi M, Shin Y, Osaki T, Hajal C, Chiono V, Kamm RD. 3D self-organized microvascular model of the human blood-brain barrier with endothelial cells, pericytes and astrocytes. Biomaterials. (2018) 180:117–29. doi: 10.1016/j.biomaterials.2018.07.014
127. Zheng Y, Chen J, Craven M, Choi NW, Totorica S, Diaz-Santana A, et al. In vitro microvessels for the study of angiogenesis and thrombosis. Proc Natl Acad Sci USA. (2012) 109:9342–7. doi: 10.1073/pnas.1201240109
128. Price GM, Wong KHK, Truslow JG, Leung AD, Acharya C, Tien J. Effect of mechanical factors on the function of engineered human blood microvessels in microfluidic collagen gels. Biomaterials. (2010) 31:6182–9. doi: 10.1016/j.biomaterials.2010.04.041
129. Tsai M, Kita A, Leach J, Rounsevell R, Huang JN, Moake J, et al. In vitro modeling of the microvascular occlusion and thrombosis that occur in hematologic diseases using microfluidic technology. J Clin Invest. (2012) 122:408–18. doi: 10.1172/JCI58753
130. Ronaldson-Bouchard K, Vunjak-Novakovic G. Organs-on-a-chip: a fast track for engineered human tissues in drug development. Cell Stem Cell. (2018) 22:310–24. doi: 10.1016/j.stem.2018.02.011
131. Brennan MD, Rexius-Hall ML, Elgass LJ, Eddington DT. Oxygen control with microfluidics. Lab Chip. (2014) 14:4305–18. doi: 10.1039/C4LC00853G
132. Gosselin EA, Eppler HB, Bromberg JS, Jewell CM. Designing natural and synthetic immune tissues. Nat Mater. (2018) 17:484–98. doi: 10.1038/s41563-018-0077-6
133. Musah S, Dimitrakakis N, Camacho DM, Church GM, Ingber DE. Directed differentiation of human induced pluripotent stem cells into mature kidney podocytes and establishment of a glomerulus chip. Nat Protoc. (2018) 13:1662–85. doi: 10.1038/s41596-018-0007-8
134. Lin NYC, Homan KA, Robinson SS, Kolesky DB, Duarte N, Moisan A, et al. Renal reabsorption in 3D vascularized proximal tubule models. Proc Natl Acad Sci USA. (2019) 116:5399–404. doi: 10.1073/pnas.1815208116
135. Vedula EM, Alonso JL, Arnaout MA, Charest JL. A microfluidic renal proximal tubule with active reabsorptive function. PLoS ONE. (2017) 12:e0184330. doi: 10.1371/journal.pone.0184330
136. Zhou M, Zhang X, Wen X, Wu T, Wang W, Yang M, et al. Development of a functional glomerulus at the organ level on a chip to mimic hypertensive nephropathy. Sci Rep. (2016) 6:31771. doi: 10.1038/srep31771
137. Wu Q, Liu J, Wang X, Feng L, Wu J, Zhu X, et al. Organ-on-a-chip: recent breakthroughs and future prospects. BioMed Eng Online. (2020) 19:9. doi: 10.1186/s12938-020-0752-0
138. Lidington EA, Moyes DL, McCormack AM, Rose ML. A comparison of primary endothelial cells and endothelial cell lines for studies of immune interactions. Transplant Immunol. (1999) 7:239–46. doi: 10.1016/S0966-3274(99)80008-2
139. Lin Y, Weisdorf DJ, Solovey A, Hebbel RP. Origins of circulating endothelial cells and endothelial outgrowth from blood. J Clin Invest. (2000) 105:71–7. doi: 10.1172/JCI8071
140. Yoder MC. Human endothelial progenitor cells. Cold Spring Harb Perspect Med. (2012) 2:a006692. doi: 10.1101/cshperspect.a006692
141. Jahan B, McCloskey KE. Differentiation and expansion of endothelial cells requires pre-optimization of KDR+ expression kinetics. Stem Cell Res. (2020) 42:101685. doi: 10.1016/j.scr.2019.101685
142. Yoder MC. Differentiation of pluripotent stem cells into endothelial cells. Curr Opin Hematol. (2015) 22:252–7. doi: 10.1097/MOH.0000000000000140
143. Park D, Lee J, Chung JJ, Jung Y, Kim SH. Integrating organs-on-chips: multiplexing, scaling, vascularization, and innervation. Trends Biotechnol. (2020) 38:99–112. doi: 10.1016/j.tibtech.2019.06.006
144. Novak R, Ingram M, Marquez S, Das D, Delahanty A, Herland A, et al. Robotic fluidic coupling and interrogation of multiple vascularized organ chips. Nat Biomed Eng. (2020) 4:407–20. doi: 10.1038/s41551-019-0497-x
145. Miller PG, Shuler ML. Design and demonstration of a pumpless 14 compartment microphysiological system. Biotechnol Bioeng. (2016) 113:2213–27. doi: 10.1002/bit.25989
146. Peck RW, Hinojosa CD, Hamilton GA. Organs-on-Chips in clinical pharmacology: putting the patient into the center of treatment selection and drug development. Clin Pharmacol Ther. (2020) 107:181–5. doi: 10.1002/cpt.1688
147. Cao X, Ashfaq R, Cheng F, Maharjan S, Li J, Ying G, et al. A tumor-on-a-chip system with bioprinted blood and lymphatic vessel pair. Adv Funct Mater. (2019) 29:1807173. doi: 10.1002/adfm.201807173
148. Farinacci M, Krahn T, Dinh W, Volk HD, Düngen HD, Wagner J, et al. Circulating endothelial cells as biomarker for cardiovascular diseases. Res Pract Thromb Haemost. (2018) 3:49–58. doi: 10.1002/rth2.12158
149. Boos CJ, Lip GYH, Blann AD. Circulating endothelial cells in cardiovascular disease. J Am Coll Cardiol. (2006) 48:1538–47. doi: 10.1016/j.jacc.2006.02.078
150. Clevers H. Modeling development and disease with organoids. Cell. (2016) 165:1586–97. doi: 10.1016/j.cell.2016.05.082
151. Rossi G, Manfrin A, Lutolf MP. Progress and potential in organoid research. Nat Rev Genet. (2018) 19:671–87. doi: 10.1038/s41576-018-0051-9
152. Shankar AS, Hoorn EJ, Gribnau J, Baan CC, Hoogduijn MJ. Current state of renal regenerative therapies. Transplantation. (2019) 103:250–61. doi: 10.1097/TP.0000000000002547
153. Combes AN, Zappia L, Er PX, Oshlack A, Little MH. Single-cell analysis reveals congruence between kidney organoids and human fetal kidney. Genome Med. (2019) 11:3. doi: 10.1186/s13073-019-0615-0
154. Luo C, Lancaster MA, Castanon R, Nery JR, Knoblich JA, Ecker JR. Cerebral organoids recapitulate epigenomic signatures of the human fetal brain. Cell Rep. (2016) 17:3369–84. doi: 10.1016/j.celrep.2016.12.001
155. Takasato M, Er PX, Chiu HS, Maier B, Baillie GJ, Ferguson C, et al. Kidney organoids from human iPS cells contain multiple lineages and model human nephrogenesis. Nature. (2015) 526:564–8. doi: 10.1038/nature15695
156. Pettinato G, Lehoux S, Ramanathan R, Salem MM, He L-X, Muse O, et al. Generation of fully functional hepatocyte-like organoids from human induced pluripotent stem cells mixed with Endothelial Cells. Sci Rep. (2019) 9:8920. doi: 10.1038/s41598-019-45514-3
157. Takebe T, Sekine K, Enomura M, Koike H, Kimura M, Ogaeri T, et al. Vascularized and functional human liver from an iPSC-derived organ bud transplant. Nature. (2013) 499:481–4. doi: 10.1038/nature12271
158. Takebe T, Zhang R-R, Koike H, Kimura M, Yoshizawa E, Enomura M, et al. Generation of a vascularized and functional human liver from an iPSC-derived organ bud transplant. Nat Protoc. (2014) 9:396–409. doi: 10.1038/nprot.2014.020
159. Pham MT, Pollock KM, Rose MD, Cary WA, Stewart HR, Zhou P, et al. Generation of human vascularized brain organoids. Neuroreport. (2018) 29:588–93. doi: 10.1097/WNR.0000000000001014
160. van den Berg CW, Ritsma L, Avramut MC, Wiersma LE, van den Berg BM, Leuning DG, et al. Renal subcapsular transplantation of PSC-derived kidney organoids induces neo-vasculogenesis and significant glomerular and tubular maturation in vivo. Stem Cell Rep. (2018) 10:751–65. doi: 10.1016/j.stemcr.2018.01.041
161. McGuigan AP, Sefton MV. Vascularized organoid engineered by modular assembly enables blood perfusion. Proc Natl Acad Sci USA. (2006) 103:11461–6. doi: 10.1073/pnas.0602740103
162. Park SE, Georgescu A, Huh D. Organoids-on-a-chip. Science. (2019) 364:960–5. doi: 10.1126/science.aaw7894
163. Schneeberger K, Spee B, Costa P, Sachs N, Clevers H, Malda J. Converging biofabrication and organoid technologies: the next frontier in hepatic and intestinal tissue engineering? Biofabrication. (2017) 9:013001. doi: 10.1088/1758-5090/aa6121
164. Homan KA, Gupta N, Kroll KT, Kolesky DB, Skylar-Scott M, Miyoshi T, et al. Flow-enhanced vascularization and maturation of kidney organoids in vitro. Nat Methods. (2019) 16:255–62. doi: 10.1038/s41592-019-0325-y
165. Koning M, van den Berg CW, Rabelink TJ. Stem cell-derived kidney organoids: engineering the vasculature. Cell Mol Life Sci. (2019) 77:2257–73. doi: 10.1007/s00018-019-03401-0
166. Skylar-Scott MA, Uzel SGM, Nam LL, Ahrens JH, Truby RL, Damaraju S, et al. Biomanufacturing of organ-specific tissues with high cellular density and embedded vascular channels. Sci Adv. (2019) 5:eaaw2459. doi: 10.1126/sciadv.aaw2459
167. Lee MO, Moon SH, Jeong HC, Yi JY, Lee TH, Shim SH, et al. Inhibition of pluripotent stem cell-derived teratoma formation by small molecules. Proc Natl Acad Sci USA. (2013) 110:E3281–90. doi: 10.1073/pnas.1303669110
168. Ben-David U, Benvenisty N. The tumorigenicity of human embryonic and induced pluripotent stem cells. Nat Rev Cancer. (2011) 11:268–77. doi: 10.1038/nrc3034
169. Quadrato G, Nguyen T, Macosko EZ, Sherwood JL, Min Yang S, Berger DR, et al. Cell diversity and network dynamics in photosensitive human brain organoids. Nature. (2017) 545:48–53. doi: 10.1038/nature22047
170. Phipson B, Er PX, Combes AN, Forbes TA, Howden SE, Zappia L, et al. Evaluation of variability in human kidney organoids. Nat Methods. (2019) 16:79–87. doi: 10.1038/s41592-018-0253-2
171. Subramanian A, Sidhom EH, Emani M, Vernon K, Sahakian N, Zhou Y, et al. Single cell census of human kidney organoids shows reproducibility and diminished off-target cells after transplantation. Nat Commun. (2019) 10:5462. doi: 10.1038/s41467-019-13382-0
172. Yu F, Hunziker W, Choudhury D. Engineering microfluidic organoid-on-a-chip platforms. Micromachines. (2019) 10:165. doi: 10.3390/mi10030165
173. Baudin B, Bruneel A, Bosselut N, Vaubourdolle M. A protocol for isolation and culture of human umbilical vein endothelial cells. Nat Protoc. (2007) 2:481–5. doi: 10.1038/nprot.2007.54
174. McGinn S, Poronnik P, Gallery ED, Pollock CA. A method for the isolation of glomerular and tubulointerstitial endothelial cells and a comparison of characteristics with the human umbilical vein endothelial cell model. Nephrology. (2004) 9:229–37. doi: 10.1111/j.1440-1797.2004.00254.x
175. Medina-Leyte DJ, Domínguez-Pérez M, Mercado I, Villarreal-Molina MT, Jacobo-Albavera L. Use of human umbilical vein endothelial cells (HUVEC) as a model to study cardiovascular disease: a review. Appl Sci. (2020) 10:938. doi: 10.3390/app10030938
176. Gaskill C, Majka SM. A high-yield isolation and enrichment strategy for human lung microvascular endothelial cells. Pulm Circ. (2017) 7:108–16. doi: 10.1177/2045893217702346
177. Ricotta D, Alessandri G, Pollara C, Fiorentini S, Favilli F, Tosetti M, et al. Adult human heart microvascular endothelial cells are permissive for non-lytic infection by human cytomegalovirus. Cardiovasc Res. (2001) 49:440–8. doi: 10.1016/S0008-6363(00)00258-3
178. Strauss O, Phillips A, Ruggiero K, Bartlett A, Dunbar PR. Immunofluorescence identifies distinct subsets of endothelial cells in the human liver. Sci Rep. (2017) 7:44356. doi: 10.1038/srep44356
179. Soloyan H, Thornton M, Villani V, Khatchadourian P, Cravedi P, Angeletti A, et al. Glomerular endothelial cell heterogeneity in Alport syndrome. Sci Rep. (2020) 10:11414. doi: 10.1038/s41598-020-67588-0
180. Satchell SC, Tasman CH, Singh A, Ni L, Geelen J, von Ruhland CJ, et al. Conditionally immortalized human glomerular endothelial cells expressing fenestrations in response to VEGF. Kidney Int. (2006) 69:1633–40. doi: 10.1038/sj.ki.5000277
181. Huebert RC, Jagavelu K, Liebl AF, Huang BQ, Splinter PL, LaRusso NF, et al. Immortalized liver endothelial cells: a cell culture model for studies of motility and angiogenesis. Lab Invest. (2010) 90:1770–81. doi: 10.1038/labinvest.2010.132
182. Rahman NA, Rasil ANaHM, Meyding-Lamade U, Craemer EM, Diah S, Tuah AA, et al. Immortalized endothelial cell lines for in vitro blood–brain barrier models: a systematic review. Brain Res. (2016) 1642:532–45. doi: 10.1016/j.brainres.2016.04.024
183. Harding A, Cortez-Toledo E, Magner NL, Beegle JR, Coleal-Bergum DP, Hao D, et al. Highly efficient differentiation of endothelial cells from pluripotent stem cells requires the MAPK and the PI3K pathways. Stem Cells. (2017) 35:909–19. doi: 10.1002/stem.2577
Keywords: solid organ transplantation, allograft rejection, endothelial cells, organoids, 3D in vitro models, organ-on-a-chip
Citation: Peelen DM, Hoogduijn MJ, Hesselink DA and Baan CC (2021) Advanced in vitro Research Models to Study the Role of Endothelial Cells in Solid Organ Transplantation. Front. Immunol. 12:607953. doi: 10.3389/fimmu.2021.607953
Received: 18 September 2020; Accepted: 21 January 2021;
Published: 10 February 2021.
Edited by:
Niels Olsen Saraiva Camara, University of São Paulo, BrazilReviewed by:
Nuala Mooney, Institut National de la Santé et de la Recherche Médicale (INSERM), FranceConstanca Figueiredo, Hannover Medical School, Germany
Copyright © 2021 Peelen, Hoogduijn, Hesselink and Baan. This is an open-access article distributed under the terms of the Creative Commons Attribution License (CC BY). The use, distribution or reproduction in other forums is permitted, provided the original author(s) and the copyright owner(s) are credited and that the original publication in this journal is cited, in accordance with accepted academic practice. No use, distribution or reproduction is permitted which does not comply with these terms.
*Correspondence: Daphne M. Peelen, ZC5wZWVsZW4mI3gwMDA0MDtlcmFzbXVzbWMubmw=