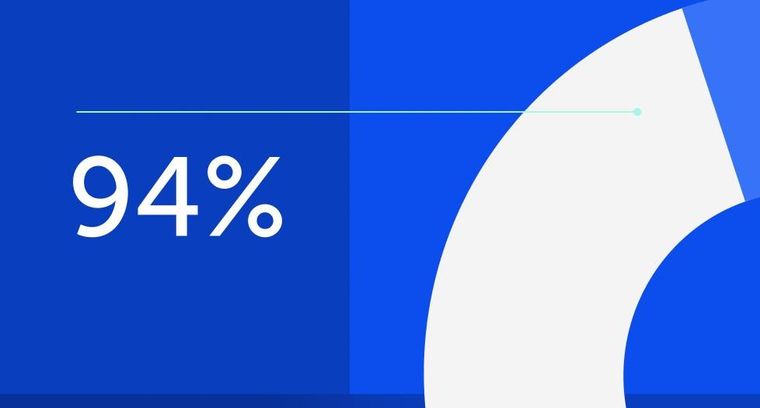
94% of researchers rate our articles as excellent or good
Learn more about the work of our research integrity team to safeguard the quality of each article we publish.
Find out more
ORIGINAL RESEARCH article
Front. Immunol., 28 June 2021
Sec. Viral Immunology
Volume 12 - 2021 | https://doi.org/10.3389/fimmu.2021.595150
This article is part of the Research TopicImmune Evasion Mechanisms by RNA VirusesView all 32 articles
As one of the current global health conundrums, COVID-19 pandemic caused a dramatic increase of cases exceeding 79 million and 1.7 million deaths worldwide. Severe presentation of COVID-19 is characterized by cytokine storm and chronic inflammation resulting in multi-organ dysfunction. Currently, it is unclear whether extrapulmonary tissues contribute to the cytokine storm mediated-disease exacerbation. In this study, we applied systems immunology analysis to investigate the immunomodulatory effects of SARS-CoV-2 infection in lung, liver, kidney, and heart tissues and the potential contribution of these tissues to cytokines production. Notably, genes associated with neutrophil-mediated immune response (e.g. CXCL1) were particularly upregulated in lung, whereas genes associated with eosinophil-mediated immune response (e.g. CCL11) were particularly upregulated in heart tissue. In contrast, immune responses mediated by monocytes, dendritic cells, T-cells and B-cells were almost similarly dysregulated in all tissue types. Focused analysis of 14 cytokines classically upregulated in COVID-19 patients revealed that only some of these cytokines are dysregulated in lung tissue, whereas the other cytokines are upregulated in extrapulmonary tissues (e.g. IL6 and IL2RA). Investigations of potential mechanisms by which SARS-CoV-2 modulates the immune response and cytokine production revealed a marked dysregulation of NF-κB signaling particularly CBM complex and the NF-κB inhibitor BCL3. Moreover, overexpression of mucin family genes (e.g. MUC3A, MUC4, MUC5B, MUC16, and MUC17) and HSP90AB1 suggest that the exacerbated inflammation activated pulmonary and extrapulmonary tissues remodeling. In addition, we identified multiple sets of immune response associated genes upregulated in a tissue-specific manner (DCLRE1C, CHI3L1, and PARP14 in lung; APOA4, NFASC, WIPF3, and CD34 in liver; LILRA5, ISG20, S100A12, and HLX in kidney; and ASS1 and PTPN1 in heart). Altogether, these findings suggest that the cytokines storm triggered by SARS-CoV-2 infection is potentially the result of dysregulated cytokine production by inflamed pulmonary and extrapulmonary (e.g. liver, kidney, and heart) tissues.
The outbreak of Coronavirus disease 2019 (COVID-19) pandemic caused by the severe acute respiratory syndrome coronavirus 2 (SARS-CoV-2), led to more than 79 million cases and 1.7 million deaths worldwide to date, as reported by the World Health Organization. The clinical presentation of COVID-19 is characterized by a wide variation in severity and symptoms reflecting both pulmonary and extra-pulmonary manifestations including hepatic and renal dysfunction and heart failure (1, 2). Importantly, expression of angiotensin converting enzyme II (ACE2), which is implicated in the cellular entry of SARS-CoV-2, in various extrapulmonary human tissues including proximal tubular cells (kidney), cholangiocytes (liver), and myocardial cells (heart) suggested the vulnerability of these tissues to SARS-CoV-2 infection (3). Moreover, SARS-CoV-2 infectivity of extrapulmonary tissues including liver, kidney, and heart tissues has been proven using in vitro cultures, organoid models, and tissue autopsies (4–6).
One of the critical mechanisms contributing to the progression of COVID-19 is the viral induction of cytokine storm, a phenomenon characterized by the unrestrained production and release of cytokines into the circulation resulting in a systemic inflammation (7). This uncontrolled release of cytokines dysregulates innate and adaptive immune responses, resulting in the infiltration of various immune effectors into different tissues. In this regards, analysis of pulmonary and extrapulmonary tissue biopsy/autopsy samples revealed interstitial infiltration of lymphocytes, macrophages, neutrophils, NK cells and dendritic cells into lung tissue (8, 9); lymphocytes into liver tissue (10); lymphocytes and macrophages into kidney tissue (11); and mononuclear inflammatory cells into heart tissue (8). Although these immune cells are initially attracted to counteract SARS-CoV-2 infection, the exacerbation of the immune response elicited by the overexpressed cytokines and transcriptional shifts can potentially promote tissue remodeling, cellular apoptosis and tissue dysfunction and cytotoxicity (11). Moreover, the progression of systemic inflammation and cytokine storm (e.g. significantly increased IL-2, IL-7, IL-10, GSCF, IP10, MCP-1, MIP1A and TNF-α levels) can potentially lead to multi-organ dysfunction (observed in approximately 5% of COVID-19 patients) and viral sepsis increasing the risk of mortality (12, 13).
This study aimed at comparing changes in immune response activation patterns across pulmonary and extrapulmonary tissues (i.e. liver, kidney, and heart) in response to SARS-CoV-2 infection. Therefore, The differential immune transcriptomic profile of lung, liver, kidney, and heart autopsy samples from COVID-19 patients was compared.
Datasets were retrieved from publicly available sets deposited in Gene Expression Omnibus (GEO) for Covid-19 infected lung, liver, kidney, and heart samples from 12 patient autopsies (GSE150316) (a total number of 58 COVID-19 samples from all tissues), and healthy lung, liver, kidney, and heart autopsy samples (GSE112356). 6 liver samples (cases 3, 4, 5, 8, 10, 12), 3 kidney samples (cases 4, 5, and 11), 7 heart samples (cases 1, 2, 3, 4, 5, and 8), and 42 lung samples (cases 1, 2, 3, 4, 5, 6, 7, 8, 9, 10, and 11) were selected from the COVID-19 patient autopsy dataset (GSE150316) for further analysis (Table 1). As elaborated in Table 1, multiple samples were taken from different locations in lung (case1: 4 samples, case 2: 3 samples, case 3: 2 samples, case 4: 2 samples, case 5: 5 samples, case 6: 5 samples, case 7: 5 samples, case 8: 5 samples, case 9: 5 samples, 3 from case 10, and 3 from case 11) and heart tissues; however, all samples were taken into consideration to avoid biased selection.
Table 1 List of analyzed healthy and COVID-19 autopsy samples retrieved from the datasets GSE112356 and GSE150316 deposited in GEO.
As reported by the authors depositing the dataset (14), the donors of the COVID-19 samples were severe COVID-19 patients; the majority requiring mechanical ventilation during their hospitalization (9 out of 12) and were deceased after 6-23 days of hospitalization, with a 100% mortality rate. Some of the donors were characterized to be immunocompromised (cases 1, 3, 6, 9, 11) while others were diabetic (cases 1, 3, 4, 5, 6, 12) and were under anti-diabetic and immunosuppressive treatments prior to contacting SARS-CoV-2 infection. The majority of the patients were reported to have elevated levels of D-Dimer (Cases 1-10 and 12), CRP (Cases 1-10 and 12), and AST (Cases 2-10 and 12), which were proposed to correlate with COVID-19 severity, inflammation and extrapulmonary tissue dysfunction (15, 16).
15 healthy autopsy samples were selected from the dataset GSE112356 including 4 healthy lung samples, 4 healthy liver samples, 4 healthy kidney samples and 3 healthy heart samples (excluding the sample H1H as it was reported to be misclassified) collected from Caucasian male donors.
Whole blood bulk RNA sequencing data (Normalized counts) deposited by Bernardes et al. (17) were retrieved for the cross validation of the autopsy tissue samples analysis (https://github.com/Systems-Immunology-IKMB/COVIDOMICs/tree/main/TF_enrichment/TF_enrichment_analysis-main/data). The dataset included samples from 14 healthy donors, 12 asymptomatic COVID-19 patients, 11 mild, 19 severe (subdivided into 6 complicated, 4 complicated incremental, 6 complicated hyperinflammatory, and 3 critical) COVID-19 patients. Complicated-incremental refers to severe COVID-19 patients with increasing clinical symptoms and inflammatory markers; Complicated-hyperinflammatory refers to patients presenting with severe signs of systemic inflammatory response; and critical refers to patients requiring ICU administration and mechanical ventilation with signs of acute respiratory distress.
To determine if the cohort used in this analysis has sufficient power to allow for the identification of predictive biomarkers, power calculation based on Wei et al. (18) was carried out. Since there are limited phenotypic data on severe COVID19 cases with affected extrapulmonary tissues in response to SARS-CoV-2 infection, well characterized severe COVID19 cases mainly from autopsy samples were included for biomarker discovery. Thus, based on whole transcriptome data of severe COVID19 studies, the standard deviation for detection of differentially expressed genes for severe cases across different tissue was determined to be around 1.5 (σ = 1.5) and effect size around 5 in well characterized cases. Carrying out the power calculation at p = 0.05 (5% significance testing) and power of 90% using R (version 3.6.2) showed that the minimum required number of samples per group to be 3 patients per group for each tissue (healthy tissue autopsy vs. lung, liver, kidney and heart tissue from severe COVID-19 patient autopsies) or disease severity group (healthy, mild, moderate and severe). Having said that, the biomarkers discovered were validated on a larger cohort of whole blood samples from different groups of COVID-19 patients.
Raw gene counts data were retrieved for all the samples and the successfully mapped genes overlapping between the samples from both datasets were filtered for further analysis (21831 overlapping genes). The row gene counts were then normalized (quantile normalization) using AltAnalyze software (19). Differentially expressed genes (DEGs) were identified by comparing each SARS-CoV-2 infected tissue against a healthy control tissue. Cutoff values for DEGs included fold change value >2 or <-2 and adjusted p-value (q-value) of 0.25 based on the methodologies described by Li et al. (20) and Subramanian et al. (21), where they chose < 0.25 cutoff for adjusted p-value to select for DEG and pathways. DEGs were intersected to identify the commonly upregulated and downregulated genes between the infected lung, liver and kidney tissues using InteractiVenn (22).
Immune response genes were identified from the DEGs using immune response gene ontology set (GO:0006955) as a reference, which were then further analyzed for functional clustering and pathway enrichment using Metascape (23). To identify the specific effect of SARS-CoV-2 infection on the expression of immune response, we cross matched the differential transcriptome from the infected lung, liver, and kidney samples with gene ontology sets retrieved using AmiGO 2 database (Table S1). Heatmap and dotplot representations were generated using R (version 3.6.0); histogram representations were generated using GraphPad Prism (version 5.01).
Blood Serum was isolated from fresh blood samples collected from 3 healthy donors, 6 asymptomatic COVID-19 patients, 2 severe COVID-19 patients with pulmonary findings only, and 4 severe COVID-19 patients with pulmonary and extrapulmonary findings (i.e. elevated levels of creatinine and liver enzymes) following the approval of the ethical committee at by the Abu Dhabi Health COVID-19 Research Ethics Committee (DOH/DQD/2020/538), SEHA Research Ethics committee (SEHA-IRB-005) and Dubai Scientific Research Ethics Committee (DSREC-04/2020_09). The blood plasma was isolated using histopaque gradient separation (Sigma). Total RNA was extracted from 300µl of Plasma using QIAamp Viral RNA Mini Kit (Qiagen). cDNA was synthesized using the High-Capacity cDNA Reverse Transcription Kit for RT PCR (Applied Biosystems). qRT-PCR was performed in triplicates with the Maxima SYBR Green/ROX qPCR Master Mix (Thermoscientific) using QuantStudio3 Real-Time PCR instrument (Applied biosystems). qRT-PCR were performed using primers for 18SrRNA, SOCS3, and TRIM56 as per the sequences in Table S2.
Unpaired, two-tailed t-test statistical analysis was performed using GraphPad Prism (version 5.01) to analyze the statistical significance of the gene expression. The significance was taken to be p < 0.05.
Analysis of the general effect of SARS-CoV-2 infection of the transcriptomic profiles of pulmonary and extrapulmonary tissues (i.e. liver, kidney, and heart tissue) showed the upregulation of 3421 genes in lung tissue, 4805 genes in liver tissue, 5685 genes in kidney tissue, and 4176 genes in heart tissue (Figure S1A). Out of these differentially upregulated genes, 1537 genes were commonly upregulated across the four types of tissues (Figure S1B). Functional clustering analysis revealed the implication of these transcripts in viral infection, intracellular and across membrane transport, GPCR signaling, WNT and mTOR signaling, tissue development and morphogenesis, response to transforming growth factor beta signaling, regulation of protein modification and degradation, response to granulocyte macrophage colony-stimulating factor, and response to hormonal stimulus and growth factors (Figure S1C).
Moreover, the analysis revealed the downregulation of 3928 genes in lung tissue, 3881 genes in liver tissue, 4848 genes in kidney tissue, and 3312 genes in heart tissue. 1375 genes out of these genes were commonly downregulated across the four types of tissues (Figure S1D). Most of the commonly downregulated transcriptome were shown to be involved in the regulation of metabolic pathways, protein translation, mitochondrial processes, and autophagy through functional clustering analysis (Figure S1E).
Examination of the enrichment of differentially expressed immune response genes in SARS-CoV-2 infected lung, liver, kidney, and heart tissues was carried out using the immune response gene ontology set (GO:0006955) from AmiGO 2 database as a reference. The count of the differentially expressed genes across the four types of infected tissues were then compared (Figure 1A). Intriguingly, the count of the immune response genes differentially expressed in severe COVID-19 patients’ liver, kidney, and heart tissue was relatively close to that of the genes differentially expressed in lung tissue. 129 immune response genes were commonly upregulated amongst the four types of tissue in response to severe SARS-CoV-2 infection (Figure 1B) while 72 genes were commonly downregulated (Figure 1C).
Figure 1 (A) Count of differentially expressed immune response genes from the immune response gene ontology set (GO:0006955) in SARS-CoV-2 infected lung, liver, kidney, and heart tissues. Venn diagram representation of the overlap of significantly (B) upregulated and (C) downregulated immune response genes amongst the four types of infected tissues. Functional clustering and pathway analysis of the commonly (D) upregulated and (E) downregulated genes across lung, liver, kidney, and heart tissues in response to SARS-CoV-2 infection.
Functional clustering and pathways analysis of the differentially expressed genes common to the four different types of infected tissues revealed a significant enrichment of transcripts implicated in many arms of the innate and adaptive immune response including the activation, chemotaxis and differentiation of leukocytes; cytokines production and signaling; response to interferon-gamma; cell-cell adhesion; NF-κB signaling; and acute inflammatory response (Figures 1D, E).
Previous studies reported that COVID-19 is frequently associated with a massive production of 14 cytokines including IFN-γ, IL-1RA (IL1RN), IL-2RA, IL-6, IL-10, IL-18, HGF, MCP-3 (CCL7), MIG (CXCL9), M-CSF (CSF1), G-CSF (CSF3), MIG-1a (CCL3), CTACK (CCL27), and IP-10 (CXCL10) (24). Therefore, the next aim of the study was to investigate the expression levels of these cytokines in pulmonary and extrapulmonary tissues such as heart, Kidney, and liver. As shown in Figure 2A, out of the 14 cytokines analyzed, 4 cytokines were differentially expressed (downregulated) in lung tissue including IL6, CSF3, IFNG, and CSF1. 10 of the cytokines were differentially expressed in liver tissue: IL6 and IL2RA were upregulated and IL10, IL18, IL1RN, CSF1, IFNG, CXCL10, HGF, and CCL27 were downregulated. A total of 7 of the cytokines were differentially expressed in kidney tissue, out of which 1 cytokine was upregulated (IL6) and 6 cytokines were downregulated (CXCL10, IL18, CCL27, IL10, IL2RA, and CXCL9). Eight cytokines were differentially expressed (downregulated) in heart tissue including IL6, IL1RN, CSF3, HGF, CCL27, CXCL9, CXCL10, and CSF1.
Figure 2 (A) dotplot representation of the expression fold change of cytokines contributing to the cytokine storm in response to SARS-CoV-2 infection in lung, liver, kidney, and heart tissues. Dotplot representation of the differentially expressed (B) chemokines, (C) interleukins, (D) interferons and tumor necrosis factor family members in lung, liver, kidney, and heart tissues infected with SARS-CoV-2.
Although some of the cytokines were commonly, differentially expressed in pulmonary and some extrapulmonary tissues (e.g. IL6, IFNG, CSF3, and CSF1), the differential expression of some of the cytokines is limited to extrapulmonary tissues such including IL2RA, IL1RN, IL18, IL10, and HGF, and CXCL9 (Figure 2A). Altogether, these results suggest that the uncontrolled production of cytokines induced by SARS-CoV-2 infection is potentially contributed by pulmonary and extrapulmonary tissues.
Further evaluation of changes in the expression of extended list of cytokines across lung, liver, kidney, and heart tissue was carried out using the chemokine gene set within the immunome gene ontology set as a reference. Analysis of the differentially expressed chemokines (Figure 2B) revealed the unique upregulation of the neutrophil specific chemokine CXCL1 in lung (25), and the eosinophil specific chemoattractant CCL11 in heart tissue (26). Monocytes chemoattractants were enriched in lung (CXCL4, CCL8, and CX3CL1), liver, (CX3CL1), kidney (CXCL4), and heart (CXCL4, and CX3CL1) (27, 28). Dendritic cells chemoattractants were enriched in lung (CCL8 and CX3CL1), liver (XCL1 and CX3CL1), kidney (XCL1), and heart (XCL1 and CX3CL1) (29–33). T-cells and B-cells chemotactic chemokines were upregulated in lung (CCL8, CXCL4 and CX3CL1), liver (CXCL16), kidney (CXCL4 and CXCL16) and heart (CXCL4 and CX3CL1) (29, 30, 32, 34).
Analysis of the differential expression of interleukins (Figure 2C) revealed the upregulation of eosinophil activator IL5 and activator of T helper cell immune response IL17B in liver and the activators of T helper cell immune response IL1B and IL17B as well as IL25 (activator of NKT cells, T helper cells, neutrophils, eosinophils, and mast cells) in kidney (35–38). On the other hand, none of the interleukins was significantly upregulated in lung or heart tissues.
Interferon gamma (IFNG) was significantly downregulated in infected lung and liver tissues (Figure 2D) while changes in the interferon alpha and beta production were negligible (FC <2 or >-2 or q-value>0.25). The absence of dysregulated interferon alpha and beta production has been previously reported in analysis of samples of COVID-19 patients which was proposed to contribute to the dysregulated early innate immune response (39, 40). interferon epsilon (IFNE) was differentially expressed (downregulated) in kidney.
Analysis of the differential expression of tumor necrosis factor family members revealed the upregulation of TNFSF13B, TNFSF14, and TNFSF15 expression in lung; TNF, TNFSF8, TNFSF14 and TNFSF15 in liver; TNFSF8, TNFSF13B, TNFSF45 and TNFSF15 expression in kidney; and TNFSF8, TNFSF14, and TNFSF15 in heart (Figure 2D). Tumor necrosis factor alpha (TNF) was only significantly upregulated in liver tissue in response to SARS-CoV-2 infection, indicating the potentially critical role of contributed by infected liver tissue in the exacerbation of systemic inflammation and cytokines storm (41).
As the degradation of the mRNA can distort the expression results of the cytokines, the next aim was to cross-validated the cytokines response pathways using functional clustering and pathways analysis of differentially expressed immune response genes in SARS-CoV-2 infected tissues. Transcripts implicated in IL-6 production pathway (GO:0032635) was significantly enriched in the upregulated transcriptome of liver and kidney tissues (log10 q-value -9.11 and -8.93, respectively). On the other, analysis of the downregulated transcriptome revealed the enrichment of transcripts implicated in cellular response to IL-1 (GO:0071347) in lung, liver, kidney and heart tissues (log10 q-value -22.56, -19.22, -29.48, and -25.59, respectively).
Upregulated transcripts implicated in the response to interferon alpha and beta (Reactome gene set: R-HSA-909733) were enriched in lung and heart tissues (log10 q-value -10.69 and -6.94, respectively), whereas downregulated transcripts were more enriched in infected heart tissue (log10 q-value -10.03). Transcripts contributing to interferon gamma response (GO:0034341) were dysregulated in the upregulated transcriptome lung, liver, kidney, and heart tissues (log10 q-value -19.24, -13.90, -20.27, and -17.70, respectively), as well as in the downregulated transcriptome of kidney tissue (log10 q-value -20.86). Functional clustering and pathway analysis of the differentially expressed immune response genes revealed the upregulation of tumor necrosis factor superfamily cytokine production (GO:0071706) in liver and kidney tissues (log10 q-value -8.65 and -13.00, respectively). On the other hand, transcripts implicated in the response to tumor necrosis factor (GO:0071356) were significantly downregulated in lung, liver, kidney, and heart tissues (log10 q-value -23.47, -27.36, -26.20, and -30.08, respectively).
Altogether, these results suggest that both pulmonary and extrapulmonary tissues may play important role in the dysregulated levels of interleukins (e.g. IL-6 and IL-1), interferon-gamma, and tumor necrosis factor superfamily cytokines in COVID-19 patients.
Contextualizing the differential expression of the identified cytokines as a part of the innate and adaptive immune responses was carried out by focusing the functional clustering and pathway analysis to assess the enrichment of the innate (GO:0002218) and adaptive (GO:0002250) immune response clusters in the differential transcriptome of each of the tissues.
In infected lung tissue, the innate and adaptive immune response functional clusters were significantly enriched in the upregulated transcriptome (log 10 q-value: -38.37 and -72.10) as well as in the downregulated transcriptome (-28.30 and -55.36), respectively. A similar pattern was observed in liver, kidney and heart tissue, revealing a significant enrichment of the innate immune response in the upregulated (-29.14, -34.20, and -31.84) and downregulated (-33.71, -49.14, and -32.08) transcriptomes; as well as a significant enrichment of the adaptive immune response in the upregulated (-80.25, -94.66, -81.95) and downregulated (-50.04, -46.11, and -37.06) transcriptomes, respectively. Remarkably, the pulmonary tissue presented a higher number of upregulated transcripts contributing to the innate immune response, whereas the extrapulmonary tissues revealed a higher number of upregulated transcripts contributing to adaptive immune response.
Taken together, these findings suggest that innate and adaptive immune response elements are significantly dysregulated in pulmonary and extrapulmonary tissues. However, infected lung tissue might display a relatively more inflammatory phenotype in response to the higher upregulation of the innate immune response genes in comparison to other tissues.
With a general idea about the immune response polarization for each of the tissues, the next aim was to further analyze the enrichment of transcripts implicated in the response of each immune effector. Therefore, the overlap of the differential transcriptome of SARS-CoV-2 infected lung, liver, kidney and heart tissues with immune cells chemotaxis gene ontology set (Figure 3A), immune cells activation gene ontology set (Figure 3B), and immunome gene ontology set (Figure 3C) was examined.
Figure 3 The count of differentially expressed genes in SARS-CoV-2 infected lung, liver, kidney, and heart tissues overlapping with the (A) immune cells chemotaxis gene ontology set, (B) immune cells activation gene ontology sets, and (C) Immunome gene ontology set ontology sets.
The analysis revealed a similar immunomodulatory effect of SARS-CoV-2 on extrapulmonary tissues (especially kidney tissue) to that exerted on the pulmonary tissue, which correlates with the close number of immune response genes differentially expressed across all examined tissues. However, a relatively higher immunomodulatory effect was observed on the extrapulmonary tissue in the chemotaxis of T-cells, B-cells, NK cells, and dendritic cells (Figure 3A) as well as the activation of NK cells, macrophages, and monocytes (Figure 3B). Moreover, kidney tissue was more enriched in transcripts implicated in inflammation, monocytes-mediated immune response, innate immunity, cytokines expression, and cellular immunity (Figure 3C).
These results support the speculation on the potential contribution of infected extrapulmonary tissues to the exacerbation of the dysregulation of the immune response and systemic inflammation, alongside infected pulmonary tissue in response to SARS-CoV-2 infection.
Since many of the discussed immune response genes are inducible by NF-κB signaling (Figure 4A), the next aim of the study was to investigate the dysregulation of NF-κB signaling as a potential underlying cause for the observed immune transcriptomic shifts in pulmonary and extrapulmonary tissues. Analysis of the differential expression revealed a predominant pattern towards the dysregulation of the NF-κB pathway elements (e.g. RELB, NFKB1, NFKB2, and BCL3) (Figure 4A). Moreover, the expression of the CBM signalosome complex genes, BCL10, MALT1 and CARD family members (CARD6, CARD8, CARD9, CARD10, CARD11, CARD14), which regulate and activate NF-κB signaling, was dysregulated across the four types of tissues (Figure 4B) (42–44).
Figure 4 dotplot representation of the expression fold change of (A) inducible NF-κB genes and (B) CBM complex and CARD family proteins in lung, liver, kidney, and heart tissues infected with SARS-CoV-2. Red arrows representing core elements of the NF-κB signaling pathways.
Functional clustering and pathway analysis of the differentially expressed immune response genes revealed a significant enrichment of NK-κB signaling pathway genes (GO:0038061) in the differential transcriptome of the four types of tissue. The enrichment of NF-kB signaling elements was most significant in kidney, then heart, liver, and the least in lung tissue (log10 q-value -34.35, -29.38, -27.37, and -21.10, respectively).
These results suggest that SARS-CoV-2 infection induces a shift in the activation state of NF-κB signaling, resulting in the transcriptional shift of NF-κB inducible genes and aberrant production of cytokines.
Analyzing commonly and uniquely differentially expressed genes across lung, liver, kidney, and heart tissue to identify potential diagnostic biomarkers for tissue-specific inflammation was carried out by examining the overlap amongst the top 20 immune response genes upregulated in each of the tissues in response to SARS-CoV-2 infection revealed 8 common genes including HSP90AB1, MUC3A, PAK2, SPN, SOCS3, TRIM56, DBNL, and BMPR1A (Figures 5A, B).
Figure 5 (A) Top 30 upregulated immune response genes in lung, liver, kidney, and heart tissues in response to SARS-CoV-2 infection; F.C.: fold change; light red represents commonly upregulated genes within the top 20 genes across the 4 types of tissues. (B) Venn diagram representation of the overlap between the top 20 upregulated immune response genes in each tissue, enlisted in (A). (C, D) Top Immune response genes uniquely upregulated in lung, liver, kidney, and heart tissues in response to SARS-CoV-2 infection. * represents p-value < 0.05; ** represents p-value < 0.01 analyzed using T-test statistical analysis.
Tripartite motif 56 (TRIM56) is an important regulator of innate immunity and TLR3-mediated anti-viral defense against various infections including hepatitis C virus (45). Moreover, two of the genes commonly upregulated are the suppressor of cytokine signaling-3 (SOCS3) and the heat shock protein HSP90AB1, which contribute to the regulation of cytokines production and signaling and subsequently, immune response and inflammation (46, 47). Furthermore, the mucin family genes, including MUC3A, MUC4, MUC5B, MUC16, and MUC17 were consistently upregulated across the four types of tissues (Figure 5A). LPCAT1, is another commonly upregulated gene between the four types of tissues, and it a regulator of inflammatory lipids synthesis (e.g. platelet-activating factor and lysophosphatidylcholine) (48).
Analysis of significantly and uniquely upregulated genes in each of the tissues in response to SARS-CoV-2 infection (Figure 5) showed that 55 genes were uniquely upregulated in lung tissue which included multiple important immune response regulators (e.g. DCLRE1C, CHI3L1, and PARP14) as well as cytokine receptors (e.g. CCR1, CCR2, and IL1RL1) (Figures 5C, D). Chitinase 3-like-1 (CHI3L1), was shown to play an important role in antipathogen innate and adaptive immune response in addition to apoptosis and tissue remodeling (49). poly-ADP-ribose polymerase (PARP14) similarly plays a central role in the activation and regulation of anti-viral interferon-based immune response (50).
23 immune response genes were significantly and uniquely upregulated in liver tissue which included some cytokines (e.g. TNFRSF4 and CXCL16) and anti-inflammatory proteins and immune checkpoints (e.g. APOA4) (Figures 5C, D); which could potentially indicate the activation of a negative feedback loop to regulate the aberrant expression of cytokines and immune mediators (51).
101 genes were significantly uniquely upregulated in kidney tissue which included some cytokines and cytokine receptors (e.g. TGFB3, CXCR2, IL5RA, and IL36NA) as well as multiple immune response regulators including the monocyte activator S100A12 (52), the interferon production regulator H2.0-like homeobox 1 (HLX) (53), LILRA5, and ISG20 (52, 53) (Figures 5C, D). Moreover, interferon-induced protein 20 (ISG20), an antiviral protein that directly degrades viral RNA (54), was uniquely upregulated in kidney tissue. Moreover, The upregulation of adhesion regulatory molecules including the intercellular adhesion molecule 5 (ICAM5) (55) and Glia maturation factor gamma (GMFG; regulates T-cell chemotaxis by regulating adhesion molecular and cellular detachment) (56) in kidney supports the observed enrichment of leukocytes (especially T-cells) recruitment pathways and immune cells infiltration into tissue biopsies in response to SARS-CoV-2 infection (11).
30 genes were significantly uniquely upregulated in heart tissue which included antiviral and immune response regulators (e.g. ASS1 and PTPN1) (Figures 5C, D). ASS1 regulates cellular survival, autophagy, immune cells differentiation (57, 58). Protein tyrosine phosphatase 1B (PTPN1) is a regulator of anti-viral interferon-based innate immune response by mediating the dephosphorylation of the mediator of IRF3 activation (MITA/STING) and a facilitator of macrophage-mediated inflammation (59, 60).
Validation of some of the putative biomarkers from the discovery analysis shown to be commonly or uniquely expressed by pulmonary and extrapulmonary tissues was carried out using the RNA sequencing data from COVID-19 patients’ whole blood samples. The expression of these putative biomarkers was analyzed across different stages of disease severity, including healthy, asymptomatic, mild, and severe (subclassified into complicated, incremental complicated, hyperinflammatory complicated and critical) (17). A significant elevation in the expression of some of the proposed putative biomarkers was revealed in the blood of COVID-19 patients with severe hyperinflammatory disease presentation (Figure 6A). Some of these biomarkers include genes identified to be uniquely dysregulated in lung tissue (ABCE1, MR1, PARP14, and IFI16), liver tissue (NFASC), kidney tissue (LILRA5, S100A12, and LILRB5), and heart tissue (ASS1), in addition to genes commonly dysregulated across the pulmonary and extrapulmonary tissues (SOCS3 and TRIM56).
Figure 6 (A) Gene expression cross-validation of identified uniquely and commonly expressed immune putative biomarkers across pulmonary and extrapulmonary tissues using RNA sequencing dataset of whole blood samples from healthy donors and asymptomatic, mild, and severe COVID-19 patients. (B) Gene expression cross-validation of commonly expressed immune putative biomarkers across pulmonary and extrapulmonary tissues using qRT-PCR analysis of blood plasma samples from 3 healthy donors as well as 6 asymptomatic COVID-19 patients, 2 severe COVID-19 patients with pulmonary findings (pulmonary findings) only, and 4 severe COVID-19 with pulmonary and extrapulmonary finding (extrapulmonary findings). * represents p-value < 0.05; ** represents p-value < 0.01; *** represents p-value < 0.001; analyzed using unpaired t-test statistical analysis.
Moreover, qRT-PCR analysis of the whole RNA extracted from the blood serum of severe COVID-19 patients with pulmonary and extrapulmonary findings, confirmed the significant elevation in the gene expression of TRIM56 and SOCS3 in response to extrapulmonary dysfunction in severe COVID-19 patients (Figure 6B).
SARS-CoV-2 infection elicits aberrant transcriptional shifts in the expression of cytokines and immune mediators, resulting in an exacerbated systemic inflammation with detrimental consequences including multi-organ dysfunction (24). However, despite the existence of experimental evidence on SARS-CoV-2 infectivity of extrapulmonary tissues including liver, kidney, and heart tissues (4–6), the effect of the SARS-CoV-2 infection on their immune transcriptome is yet to be explored. Moreover, the contribution of these extrapulmonary tissues to the exacerbation of the cytokine storm in response to SARS-CoV-2 infection remains unclear. Therefore, in this study we aimed at investigating immune response shifts in pulmonary and extrapulmonary tissues (liver, kidney, and heart) in patients with COVID-19 using systems immunology analysis.
The results showed significant dysregulation of immune response genes in both pulmonary and extrapulmonary tissues; remarkably, the dysregulation in some extrapulmonary tissues (kidney) supersedes the dysregulation in pulmonary tissue. Moreover, this dysregulation of the immune response comprises the significant dysregulation of the innate and adaptive arms of the immune response in the upregulated and downregulated transcriptome of the four types of tissues. Although immune cells infiltration into some SARS-CoV-2 infected extrapulmonary tissues has been detected histologically (8, 10, 11), tissue-specific transcriptional shifts in immune response across different extrapulmonary tissues has not been explored before. For the first time we report that SARS-CoV-2 infection elicits immunomodulatory effects in extrapulmonary tissues in addition to pulmonary tissues (Figure 7).
Figure 7 SARS-CoV-2 may target extrapulmonary tissues along pulmonary tissues thereby inducing transcriptional shifts in the expression of central immune regulators (examples in the blue boxes; putative biomarkers unique for each tissue). Consequently, pulmonary as well as extrapulmonary tissues may secrete cytokines and immune mediators (in blue font) resulting in the chemoattraction and activation of innate and adaptive immune cells (e.g. Neutrophils, eosinophils, monocytes, dendritic cells, T-cells and B-cells). Upon activation, the infiltrating immune cells begin to uncontrollably secrete additional cytokines and immune mediators which elicits the cytokine storm and system inflammation phenomena.
Investigations on the pathogenesis of COVID-19 revealed a massive elevation of 14 cytokines levels in patient sera reflecting the cytokine storm and systemic inflammation (24). The analysis showed that out of these 14 cytokines, IL6 was significantly upregulated in liver and kidney tissues and IL2RA was significantly upregulated in liver tissue. More extended analysis of cytokines expression revealed the upregulation of neutrophil specific chemokine, CXCL1, in lung (25); the eosinophil chemoattractant, CCL11, in heart tissue (26); Monocytes chemoattractants in lung (CXCL4, CCL8, and CX3CL1), liver, (CX3CL1), kidney (CXCL4), and heart (CXCL4, and CX3CL1) (27, 28); dendritic cell chemoattractants in lung (CCL8 and CX3CL1), liver (XCL1 and CX3CL1), kidney (XCL1), and heart (XCL1 and CX3CL1) (29–33); and T-cells and B-cells chemotactic chemokines in lung (CCL8, CXCL4 and CX3CL1), liver (CXCL16), kidney (CXCL4 and CXCL16) and heart (CXCL4 and CX3CL1) (29, 30, 32, 34). These findings suggest that the systemic cytokine storm is a product of the combined aberrant production of cytokines by pulmonary and extrapulmonary tissues.
The unique upregulation of CXCL1 in lung tissue in combination with gene set ontology analysis suggest that neutrophil-mediated inflammatory immune response is predominant in pulmonary tissue. This speculation is concordant with the clinical findings reporting the neutrophilic infiltration into patients’ pulmonary tissue in response to SARS-CoV-2 infection (61). Increased neutrophilic infiltration was proposed to contribute to the initiation of the cytokine storm, aggravation of immune response dysregulation, and potentially development of vascular thrombosis (61). Although CXCL1 was not reported to be upregulated in COVID-19 patient sera, it was highlighted as a significant element of upregulated transcriptome of SARS-CoV-2 infected normal human bronchial epithelial cells (62).
The unique upregulation of the eosinophil-specific chemoattractant, CCL11, suggests the potential activation of an eosinophil-mediated inflammatory response, which can be one of the mechanisms contributing to myocardial injury and heart failure in COVID-19 patients (63). However, as the upregulation of the eosinophilic response elements is unique to heart tissue, the lack of eosinophilic activation in lung tissues can potentially explain the lack of correlation between eosinophilic disorders (e.g. asthma) and SARS-CoV-2 as risk factors, on the contrary to other influenza virus infections (64).
Another important finding in this study is the significant enrichment of T-cell and B-cell chemoattractants in all analyzed tissues, which may explain the lymphocytic infiltrates into pulmonary and extrapulmonary tissues detected in COVID-19 patient autopsy samples (8, 10, 11). In this regards, it is plausible that the lymphopenia observed in COVID-19 patients (65) may result from increased lymphocytic recruitment to pulmonary and extrapulmonary tissues. Intriguingly, CKLF Like MARVEL Transmembrane Domain Containing 6 (CMTM6), a regulator and stabilizer of PD-L1 (66), was commonly upregulated across the four types of tissues. Through stabilizing and enhancing PD-L1 function, CMTM6 can contribute to excessive T-cell exhaustion, as observed in COVID-19 patients (67).
Histologic analysis revealed as well the infiltration of monocytes into lung, liver, kidney, and heart tissue in response to SARS-CoV-2 infection (68), which is consistent with the upregulation of monocyte chemoattractants in the four types of tissues in the transcriptome analysis. Remarkably, the consistent enrichment of transcripts implicated in immune response mediated by dendritic cells, monocytes, T-cell and B-cells suggests the activation of late stage innate immunity and adaptive immunity in both pulmonary and extrapulmonary tissues. However, the absence of significant Interferon-alpha/beta/gamma upregulation in the investigated pulmonary and extrapulmonary tissues confirms previous speculations on the shift of immune response polarization from a classic anti-viral response to a pathogenic inflammation that fails to safeguard against SARS-CoV-2 (69).
Functional clustering and pathway analysis of potential upstream regulators of the observed immune response dysregulation revealed NF-κB signaling as a potential upstream target of SARS-CoV-2 infection. NF-κB signaling was dysregulated in pulmonary and extrapulmonary tissues (liver, kidney, and heart) resulting a shift in the activation pattern of NF-κB signaling and consequent transcriptional shifts of NF-κB inducible genes including cytokines. Amongst the different elements dysregulated in the NF-κB signaling pathway across all the investigated tissues was the CBM complex (CARD-BCL10-MALT1), a molecular bridge that propagates extracellular immunomodulatory signals by regulating NF-κB signaling (70). Previous investigations on the molecular mechanisms underlying the pathogenesis of COVID-19 proposed NF-κB signaling as a potential mechanism underlying the initiation and polarization of the inflammatory response towards a pathogenic phenotype in pulmonary tissue (69). This study extended this theory to include extrapulmonary tissue as a target of SARS-CoV-2 immunomodulatory effect potentially through the dysregulation of NF-κB signaling.
Mucin family members including MUC4, MUC16, MUC20, MUC5AC, and MUC5B have been reported to be upregulated in bronchial club cells in response to SARS-CoV-2 infection (71). However, for the first time we report upregulation of multiple Mucin family members (e.g. MUC3A, MUC4, MUC5B, MUC16, and MUC17) in pulmonary and extrapulmonary tissues in response SARS-CoV-2 infection. Mucin family members, including MUC4, play important regulatory roles in proliferation, epithelial to mesenchymal transition (EMT), fibroblast to myofibroblast transition (FMT), tissue remodeling and fibrosis as observed in diseases such as idiopathic pulmonary fibrosis (IPF) and cancer (72–74).
Similarly, we report the novel finding on the significant upregulation of Heat Shock Protein 90 Alpha Family Class B Member 1 (HSP90AB1) was in the four types of pulmonary and extrapulmonary tissues. Beyond the role of HSP90AB1 in eliciting immune response and regulating viral infectivity (75), HSP90AB1 has been shown to promote tissue remodeling by stabilizing and enhancing TGF-β signaling (76). Moreover, HSP90AB1 promotes epithelial to mesenchymal transition by activating AKT and WNT signaling pathways through the WNT signaling receptor, LRP5 (77). Additionally, the infiltration of the immune effectors, including macrophages, into tissues in response to SARS-CoV-2 infection has been associated with tissue remodeling and fibrosis (68). Taken together, these results suggest that the exacerbated inflammation activates tissue remodeling genes which contributed to the observed pulmonary fibrosis and multi-organ dysfunction in COVID-19 patients.
Moreover, Lysophosphatidylcholine Acyltransferase 1 (LPCAT1), a commonly upregulated gene in SARS-CoV-2 infected pulmonary and extrapulmonary tissues, is an important regulator of the synthesis of potent inflammatory lipids such as platelet-activating factor and lysophosphatidylcholine (48). Besides its potential contribution to the modulation of the immune response and inflammation, the upregulation LPCAT1 could potentially link to the high venous and arterial thromboembolism incidence observed in severe cases of COVID-19 patients (~30.7%) (78).
The analysis further revealed potential tissue-specific immune signatures with uniquely upregulated immune response genes in each of the pulmonary and extrapulmonary tissues including DCLRE1C, CHI3L1, and PARP14 in lung; APOA4, NFASC, WIPF3, and CD34 in liver; LILRA5, ISG20, S100A12, and HLX in kidney; and ASS1 and PTPN1 in heart. The overexpression of some of these biomarkers was similarly observed in the blood serum of severe COVID-19 patients with extrapulmonary dysfunction (e.g. SOCS3 and TRIM56). The unique upregulation of these putative biomarkers offers a potential diagnostic approach to assess the inflammation of each of these extrapulmonary tissues. Since the exacerbation of the inflammation in liver, kidney and heart can potentially result in tissue dysfunction and potential organ failure, these biomarkers can be assessed for their potential to predict tissue dysfunction at early stages and therefore guide medical intervention. Therefore, further investigation on these biomarkers is required to assess their accessibility through liquid biopsies and their diagnostic and predictive potential in COVID-19 pathogenesis.
Whilst the main limitation is the lack of validation of the genes on patients’ tissue samples, nevertheless we feel that the systems immunology analysis carried out in this study have resulted in identifying unique set of genes that are linked to different tissues from severe COVID-19 patients warranting further studies to carry out the validation on different cohort of patients’ autopsies once they become available. In addition to address the validity of the results we carried out cross sample validation using a smaller number of samples (15 lung samples, 2 liver samples, 2 kidney samples and 3 heart samples) and the results were similar. For instance, the analysis revealed the unique upregulation of CXCL1 in lung, CCL11 in heart, and IL6 in liver and kidney as well as the common upregulation of mucin family genes and HSP90AB1 across the four types of tissues. Moreover, the identified unique set of genes were cross-validated using patients’ blood serum samples as well as publicly deposited RNA-seq data from COVID-19 patients whole blood samples. The cross-validation confirmed the upregulation of some of these genes in correlation with disease severity and extrapulmonary dysfunction.
Publicly available datasets were analyzed in this study. This data can be found here: COVID19 autopsy samples were retrieved from https://www.ncbi.nlm.nih.gov/geo/query/acc.cgi?acc=GSE150316, Gene Expression Omnibus (GEO), accession number GSE150316. Healthy autopsy samples were retrieved from https://www.ncbi.nlm.nih.gov/geo/query/acc.cgi?acc=GSE112356, Gene Expression Omnibus (GEO), accession number GSE112356. COVID-19 Whole blood RNA-seq dataset was retrieved from https://github.com/Systems-Immunology-IKMB/COVIDOMICs/tree/main/TF_enrichment/TF_enrichment_analysis-main/data.
The studies involving human participants were reviewed and approved by Abu Dhabi Health COVID-19 Research Ethics Committee (DOH/DQD/2020/538), SEHA Research Ethics committee (SEHA-IRB-005) and Dubai Scientific Research Ethics Committee (DSREC-04/2020_09). The patients/participants provided their written informed consent to participate in this study.
SH, AH, MR, and RH were responsible for the conception, design, and development of the methodology. SH, AH, PB, AK, MR, and RH were responsible for the application of the methodology, the bioinformatics analysis and data interpretation. SH, AH, PB, HA, BM, AK, HB, RabH, QH, MR and RH were responsible for writing and reviewing the manuscript. RH, MR, QH, HB were responsible for the supervision of the study. All authors contributed to the article and approved the submitted version.
This research has been financially supported by University of Sharjah COVID-19 grant (CoV19-0308) and Sharjah Research Academy (Grant code: MED001) and University of Sharjah (Grant code: 1901090254). HB and AK acknowledge funding by the Deutsche Forschungsgemeinschaft (DFG, German Research Foundation) under Germany’s Excellence Strategy – EXC 22167-390884018.
The authors declare that the research was conducted in the absence of any commercial or financial relationships that could be construed as a potential conflict of interest.
The Supplementary Material for this article can be found online at: https://www.frontiersin.org/articles/10.3389/fimmu.2021.595150/full#supplementary-material
1. Lin L, Jiang X, Zhang Z, Huang S, Zhang Z, Fang Z, et al. Gastrointestinal Symptoms of 95 Cases With SARS-CoV-2 Infection. Gut (2020) 69(6):997–1001. doi: 10.1136/gutjnl-2020-321013
2. Behzad S, Aghaghazvini L, Radmard AR, Gholamrezanezhad A. Extrapulmonary Manifestations of COVID-19: Radiologic and Clinical Overview. Clin Imaging (2020) 66:35–41. doi: 10.1016/j.clinimag.2020.05.013
3. Zou X, Chen K, Zou J, Han P, Hao J, Han Z. Single-Cell RNA-seq Data Analysis on the Receptor ACE2 Expression Reveals the Potential Risk of Different Human Organs Vulnerable to 2019-nCoV Infection. Front Med (2020) 14(2):185–92. doi: 10.1007/s11684-020-0754-0
4. Monteil V, Kwon H, Prado P, Hagelkrüys A, Wimmer RA, Stahl M, et al. Inhibition of SARS-CoV-2 Infections in Engineered Human Tissues Using Clinical-Grade Soluble Human Ace2. Cell (2020) 181(4):905–13. doi: 10.1016/j.cell.2020.04.004
5. Zhao B, Ni C, Gao R, Wang Y, Yang L, Wei J, et al. Recapitulation of SARS-CoV-2 Infection and Cholangiocyte Damage With Human Liver Ductal Organoids. Protein Cell (2020) 11:771–5. doi: 10.1007/s13238-020-00718-6
6. Kalkeri R, Goebel S, Sharma GD. SARS-Cov-2 Shedding From Asymptomatic Patients: Contribution of Potential Extrapulmonary Tissue Reservoirs. Am J Trop Med Hyg (2020) 103(1):18–21. doi: 10.4269/ajtmh.20-0279
7. Coperchini F, Chiovato L, Croce L, Magri F, Rotondi M. The Cytokine Storm in COVID-19: An Overview of the Involvement of the Chemokine/Chemokine-Receptor System. Cytokine Growth Factor Rev (2020) 53:25–32. doi: 10.1016/j.cytogfr.2020.05.003
8. Xu Z, Shi L, Wang Y, Zhang J, Huang L, Zhang C, et al. Pathological Findings of COVID-19 Associated With Acute Respiratory Distress Syndrome. Lancet Respir Med (2020) 8(4):420–2. doi: 10.1016/s2213-2600(20)30076-x
9. Zhang Y, Gao Y, Qiao L, Wang W, Chen D. Inflammatory Response Cells During Acute Respiratory Distress Syndrome in Patients With Coronavirus Disease 2019 (Covid-19). Ann Internal Med (2020) 173(5):402–4. doi: 10.7326/l20-0227%m32282871.
10. Wang Y, Liu S, Liu H, Li W, Lin F, Jiang L, et al. Sars-CoV-2 Infection of the Liver Directly Contributes to Hepatic Impairment in Patients With COVID-19. J Hepatol (2020) 73(4):807–16. doi: 10.1016/j.jhep.2020.05.002
11. Diao B, Wang C, Wang R, Feng Z, Tan Y, Wang H, et al. Human Kidney Is A Target For Novel Severe Acute Respiratory Syndrome Coronavirus 2 Infection. Nat Commun (2021) 12:2506. doi: 10.1101/2020.03.04.20031120. 2020.03.04.20031120.
12. Song P, Li W, Xie J, Hou Y, You C. Cytokine Storm Induced by SARS-Cov-2. Clin Chim Acta (2020) 509:280–7. doi: 10.1016/j.cca.2020.06.017
13. Li H, Liu L, Zhang D, Xu J, Dai H, Tang N, et al. Sars-CoV-2 and Viral Sepsis: Observations and Hypotheses. Lancet (2020) 395(10235):1517–20. doi: 10.1016/s0140-6736(20)30920-x
14. Desai N, Neyaz A, Szabolcs A, Shih AR, Chen JH, Thapar V, et al. Temporal And Spatial Heterogeneity Of Host Response To SARS-Cov-2 Pulmonary Infection. Nat Commun (2020) 11:6319. doi: 10.1101/2020.07.30.20165241. 2020.07.30.20165241.
15. Liao D, Zhou F, Luo L, Xu M, Wang H, Xia J, et al. Haematological Characteristics and Risk Factors in the Classification and Prognosis Evaluation of COVID-19: A Retrospective Cohort Study. Lancet Haematology (2020) 7(9):e671–e8. doi: 10.1016/s2352-3026(20)30217-9
16. Oussalah A, Gleye S, Urmes IC, Laugel E, Barbé F, Orlowski S, et al. The Spectrum of Biochemical Alterations Associated With Organ Dysfunction and Inflammatory Status and Their Association With Disease Outcomes in Severe COVID-19: A Longitudinal Cohort and Time-Series Design Study. EClinicalMedicine (2020) 27:100554. doi: 10.1016/j.eclinm.2020.100554
17. Bernardes JP, Mishra N, Tran F, Bahmer T, Best L, Blase JI, et al. Longitudinal Multi-omics Analyses Identify Responses of Megakaryocytes, Erythroid Cells, and Plasmablasts as Hallmarks of Severe Covid-19. Immunity (2020) 53(6):1296–314.e9. doi: 10.1016/j.immuni.2020.11.017
18. Wei C, Li J, Bumgarner RE. Sample Size for Detecting Differentially Expressed Genes in Microarray Experiments. BMC Genomics (2004) 5(87):1471–2164. doi: 10.1186/1471-2164-5-87
19. Emig D, Salomonis N, Baumbach J, Lengauer T, Conklin BR, Albrecht M. AltAnalyze and DomainGraph: Analyzing and Visualizing Exon Expression Data. Nucleic Acids Res (2010) 38(2):W755–62. doi: 10.1093/nar/gkq405
20. Li J, Witten DM, Johnstone IM, Tibshirani R. Normalization, Testing, and False Discovery Rate Estimation for RNA-Sequencing Data. Biostatistics (2012) 13(3):523–38. doi: 10.1093/biostatistics/kxr031
21. Subramanian A, Tamayo P, Mootha VK, Mukherjee S, Ebert BL, Gillette MA, et al. Gene Set Enrichment Analysis: A Knowledge-Based Approach for Interpreting Genome-Wide Expression Profiles. Proc Natl Acad Sci (2005) 102(43):15545–50. doi: 10.1073/pnas.0506580102
22. Heberle H, Meirelles GV, da Silva FR, Telles GP, Minghim R. InteractiVenn: A Web-Based Tool for the Analysis of Sets Through Venn Diagrams. BMC Bioinf (2015) 16(1):169–. doi: 10.1186/s12859-015-0611-3
23. Zhou Y, Zhou B, Pache L, Chang M, Khodabakhshi AH, Tanaseichuk O, et al. Metascape Provides a Biologist-Oriented Resource for the Analysis of Systems-Level Datasets. Nat Commun (2019) 10(1):019–09234. doi: 10.1038/s41467-019-09234-6
24. Yang Y, Shen C, Li J, Yuan J, Yang M, Wang F, et al. Plasma IP-10 And MCP-3 Levels Are Highly Associated With Disease Severity And Predict The Progression Of COVID-19. J Allergy Clin Immunol (2020) 146(1):119–27.e4. doi: 10.1016/j.jaci.2020.04.027
25. Sawant KV, Poluri KM, Dutta AK, Sepuru KM, Troshkina A, Garofalo RP, et al. Chemokine CXCL1 Mediated Neutrophil Recruitment: Role of Glycosaminoglycan Interactions. Sci Rep (2016) 6(1):33123. doi: 10.1038/srep33123
26. Kitaura M, Nakajima T, Imai T, Harada S, Combadiere C, Tiffany HL, et al. Molecular Cloning of Human Eotaxin, An Eosinophil-Selective CC Chemokine, and Identification of a Specific Eosinophil Eotaxin Receptor, CC Chemokine Receptor 3. J Biol Chem (1996) 271(13):7725–30. doi: 10.1074/jbc.271.13.7725
27. Fox JM, Kausar F, Day A, Osborne M, Hussain K, Mueller A, et al. Cxcl4/Platelet Factor 4 is An Agonist of CCR1 and Drives Human Monocyte Migration. Sci Rep (2018) 8(1):9466. doi: 10.1038/s41598-018-27710-9
28. Struyf S, Proost P, Vandercappellen J, Dempe S, Noyens B, Nelissen S, et al. Synergistic Up-Regulation of MCP-2/CCL8 Activity is Counteracted by Chemokine Cleavage, Limiting its Inflammatory and Anti-Tumoral Effects. Eur J Immunol (2009) 39(3):843–57. doi: 10.1002/eji.200838660
29. Takamura K, Fukuyama S, Nagatake T, Kim D-Y, Kawamura A, Kawauchi H, et al. Regulatory Role of Lymphoid Chemokine CCL19 and CCL21 in the Control of Allergic Rhinitis. J Immunol (2007) 179(9):5897–906. doi: 10.4049/jimmunol.179.9.5897
30. Ferretti E, Pistoia V, Corcione A. Role of Fractalkine/CX3CL1 and Its Receptor in the Pathogenesis of Inflammatory and Malignant Diseases With Emphasis on B Cell Malignancies. Mediators Inflammation (2014) 2014:480941. doi: 10.1155/2014/480941
31. Matsuo K, Kitahata K, Kawabata F, Kamei M, Hara Y, Takamura S, et al. A Highly Active Form of XCL1/Lymphotactin Functions as an Effective Adjuvant to Recruit Cross-Presenting Dendritic Cells for Induction of Effector and Memory Cd8+ T Cells. Front Immunol (2018) 9:2775. doi: 10.3389/fimmu.2018.02775
32. Lee A, Eri R, Lyons A, Grimm M, Korner H. Cc Chemokine Ligand 20 and Its Cognate Receptor CCR6 in Mucosal T Cell Immunology and Inflammatory Bowel Disease: Odd Couple or Axis of Evil? Front Immunol (2013) 4:194. doi: 10.3389/fimmu.2013.00194
33. Sokol CL, Camire RB, Jones MC, Luster AD. The Chemokine Receptor CCR8 Promotes the Migration of Dendritic Cells Into the Lymph Node Parenchyma to Initiate the Allergic Immune Response. Immunity (2018) 49(3):449–63.e6. doi: 10.1016/j.immuni.2018.07.012
34. Mueller A, Meiser A, McDonagh EM, Fox JM, Petit SJ, Xanthou G, et al. CXCL4-Induced Migration of Activated T Lymphocytes is Mediated by the Chemokine Receptor CXCR3. J Leukoc Biol (2008) 83(4):875–82. doi: 10.1189/jlb.1006645
35. Roufosse F. Targeting the Interleukin-5 Pathway for Treatment of Eosinophilic Conditions Other Than Asthma. Front Med (2018) 5:49. doi: 10.3389/fmed.2018.00049
36. Santarlasci V, Cosmi L, Maggi L, Liotta F, Annunziato F. IL-1 and T Helper Immune Responses. Front Immunol (2013) 4:182. doi: 10.3389/fimmu.2013.00182
37. Ramirez-Carrozzi V, Ota N, Sambandam A, Wong K, Hackney J, Martinez-Martin N, et al. Cutting Edge: Il-17b Uses Il-17RA and IL-17RB to Induce Type 2 Inflammation From Human Lymphocytes. J Immunol (2019) 202(7):1935–41. doi: 10.4049/jimmunol.1800696. ji1800696.
38. Liu Y, Shao Z, Shangguan G, Bie Q, Zhang B. Biological Properties and the Role of IL-25 in Disease Pathogenesis. J Immunol Res (2018) 2018:6519465. doi: 10.1155/2018/6519465
39. Chu H, Chan JF-W, Wang Y, Yuen TT-T, Chai Y, Hou Y, et al. Comparative Replication and Immune Activation Profiles of SARS-CoV-2 and SARS-CoV in Human Lungs: An Ex Vivo Study With Implications for the Pathogenesis of COVID-19. Clin Infect Dis (2020) 71(6):1400–9. doi: 10.1093/cid/ciaa410
40. O’Brien TR, Thomas DL, Jackson SS, Prokunina-Olsson L, Donnelly RP, Hartmann R. Weak Induction of Interferon Expression by Severe Acute Respiratory Syndrome Coronavirus 2 Supports Clinical Trials of Interferon-λ to Treat Early Coronavirus Disease 2019. Clin Infect Dis (2020) 71(6):1410–2. doi: 10.1093/cid/ciaa453
41. Girija ASS, Shankar EM, Larsson M. Could SARS-CoV-2-Induced Hyperinflammation Magnify the Severity of Coronavirus Disease (Covid-19) Leading to Acute Respiratory Distress Syndrome? Front Immunol (2020) 11:1206. doi: 10.3389/fimmu.2020.01206
42. Ruland J, Hartjes L. Card–Bcl-10–MALT1 Signalling in Protective and Pathological Immunity. Nat Rev Immunol (2019) 19(2):118–34. doi: 10.1038/s41577-018-0087-2
43. Bouchier-Hayes L, Conroy H, Egan H, Adrain C, Creagh EM, MacFarlane M, et al. CARDINAL, a Novel Caspase Recruitment Domain Protein, Is an Inhibitor of Multiple Nf-κb Activation Pathways. J Biol Chem (2001) 276(47):44069–77. doi: 10.1074/jbc.M107373200
44. Stehlik C, Hayashi H, Pio F, Godzik A, Reed JC. Card6 Is a Modulator of NF-κb Activation by Nod1- and Cardiak-Mediated Pathways. J Biol Chem (2003) 278(34):31941–9. doi: 10.1074/jbc.M300009200
45. Shen Y, Li NL, Wang J, Liu B, Lester S, Li K. TRIM56 is an Essential Component of the TLR3 Antiviral Signaling Pathway. J Biol Chem (2012) 287(43):36404–13. doi: 10.1074/jbc.M112.397075
46. Zininga T, Ramatsui L, Shonhai A. Heat Shock Proteins as Immunomodulants. Molecules (2018) 23(11):2846. doi: 10.3390/molecules23112846
47. Rottenberg M, Carow B. SOCS3, a Major Regulator of Infection and Inflammation. Front Immunol (2014) 5:58. doi: 10.3389/fimmu.2014.00058
48. Cheng L, Han X, Shi Y. A Regulatory Role of LPCAT1 in the Synthesis of Inflammatory Lipids, PAF and LPC, in the Retina of Diabetic Mice. Am J Physiol Endocrinol Metab (2009) 297(6):E1276–E82. doi: 10.1152/ajpendo.00475.2009
49. He CH, Lee CG, Dela Cruz CS, Lee C-M, Zhou Y, Ahangari F, et al. Chitinase 3-Like 1 Regulates Cellular and Tissue Responses Via IL-13 Receptor α2. Cell Rep (2013) 4(4):830–41. doi: 10.1016/j.celrep.2013.07.032
50. Caprara G, Prosperini E, Piccolo V, Sigismondo G, Melacarne A, Cuomo A, et al. Parp14 Controls the Nuclear Accumulation of a Subset of Type I IFN–Inducible Proteins. J Immunol (2018) 200(7):2439–54. doi: 10.4049/jimmunol.1701117
51. Zhang Y, He J, Zhao J, Xu M, Lou D, Tso P, et al. Effect of ApoA4 on SERPINA3 Mediated by Nuclear Receptors NR4A1 and NR1D1 in Hepatocytes. Biochem Biophys Res Commun (2017) 487(2):327–32. doi: 10.1016/j.bbrc.2017.04.058
52. Becknell B, Hughes TL, Freud AG, Blaser BW, Yu J, Trotta R, et al. Hlx Homeobox Transcription Factor Negatively Regulates Interferon-Gamma Production in Monokine-Activated Natural Killer Cells. Blood (2007) 109(6):2481–7. doi: 10.1182/blood-2006-10-050096
53. Foell D, Wittkowski H, Kessel C, Lüken A, Weinhage T, Varga G, et al. Proinflammatory S100A12 Can Activate Human Monocytes Via Toll-Like Receptor 4. Am J Respir Crit Care Med (2013) 187(12):1324–34. doi: 10.1164/rccm.201209-1602OC
54. Wu N, Nguyen X-N, Wang L, Appourchaux R, Zhang C, Panthu B, et al. The Interferon Stimulated Gene 20 Protein (ISG20) Is an Innate Defense Antiviral Factor That Discriminates Self Versus Non-Self Translation. PloS Pathog (2019) 15(10):e1008093. doi: 10.1371/journal.ppat.1008093
55. Yang H. Structure, Expression, and Function of ICAM-5. Comp Funct Genomics (2012) 2012:368938–. doi: 10.1155/2012/368938
56. Lippert DN, Wilkins JA. Glia Maturation Factor Gamma Regulates the Migration and Adherence of Human T Lymphocytes. BMC Immunol (2012) 13(21):1471–2172. doi: 10.1186/1471-2172-13-21
57. Delage B, Luong P, Maharaj L, O’Riain C, Syed N, Crook T, et al. Promoter Methylation of Argininosuccinate Synthetase-1 Sensitises Lymphomas to Arginine Deiminase Treatment, Autophagy and Caspase-Dependent Apoptosis. Cell Death Dis (2012) 3(7):e342–e. doi: 10.1038/cddis.2012.83
58. Tarasenko TN, Gomez-Rodriguez J, McGuire PJ. Impaired T Cell Function in Argininosuccinate Synthetase Deficiency. J Leukocyte Biol (2015) 97(2):273–8. doi: 10.1189/jlb.1AB0714-365R
59. Xia T, Yi X-M, Wu X, Shang J, Shu H-B. PTPN1/2-Mediated Dephosphorylation of MITA/STING Promotes Its 20S Proteasomal Degradation and Attenuates Innate Antiviral Response. Proc Natl Acad Sci (2019) 116(40):20063–9. doi: 10.1073/pnas.1906431116
60. Través PG, Pardo V, Pimentel-Santillana M, González-Rodríguez Á, Mojena M, Rico D, et al. Pivotal Role of Protein Tyrosine Phosphatase 1B (PTP1B) in the Macrophage Response to Pro-Inflammatory and Anti-Inflammatory Challenge. Cell Death Dis (2014) 5(3):e1125–e. doi: 10.1038/cddis.2014.90
61. Tomar B, Anders HJ, Desai J, Mulay SR. Neutrophils and Neutrophil Extracellular Traps Drive Necroinflammation in COVID-19. Cells (2020) 9(6):1383. doi: 10.3390/cells9061383
62. Blanco-Melo D, Nilsson-Payant BE, Liu W-C, Uhl S, Hoagland D, Møller R, et al. Imbalanced Host Response to SARS-CoV-2 Drives Development of COVID-19. Cell (2020) 181(5):1036–45.e9. doi: 10.1016/j.cell.2020.04.026
63. Zheng Y-Y, Ma Y-T, Zhang J-Y, Xie X. COVID-19 and the Cardiovascular System. Nat Rev Cardiol (2020) 17(5):259–60. doi: 10.1038/s41569-020-0360-5
64. Lindsley AW, Schwartz JT, Rothenberg ME. Eosinophil Responses During COVID-19 Infections and Coronavirus Vaccination. J Allergy Clin Immunol (2020) 146(1):1–7. doi: 10.1016/j.jaci.2020.04.021
65. Alqahtani SA, Schattenberg JM. Liver Injury in COVID-19: The Current Evidence. United Eur Gastroenterol J (2020) 8(5):509–19. doi: 10.1177/2050640620924157
66. Mezzadra R, Sun C, Jae LT, Gomez-Eerland R, de Vries E, Wu W, et al. Identification of CMTM6 and CMTM4 as PD-L1 Protein Regulators. Nature (2017) 549(7670):106–10. doi: 10.1038/nature23669
67. Zheng H-Y, Zhang M, Yang C-X, Zhang N, Wang X-C, Yang X-P, et al. Elevated Exhaustion Levels and Reduced Functional Diversity of T Cells in Peripheral Blood May Predict Severe Progression in COVID-19 Patients. Cell Mol Immunol (2020) 17(5):541–3. doi: 10.1038/s41423-020-0401-3
68. Merad M, Martin JC. Pathological Inflammation in Patients With COVID-19: A Key Role for Monocytes and Macrophages. Nat Rev Immunol (2020) 20(6):355–62. doi: 10.1038/s41577-020-0331-4
69. Neufeldt CJ, Cerikan B, Cortese M, Frankish J, Lee J-Y, Plociennikowska A, et al. Sars-CoV-2 Infection Induces a Pro-Inflammatory Cytokine Response Through cGAS-STING and NF-κb. bioRxiv (2020). doi: 10.1101/2020.07.21.212639
70. Lu HY, Bauman BM, Arjunaraja S, Dorjbal B, Milner JD, Snow AL, et al. The CBM-opathies-A Rapidly Expanding Spectrum of Human Inborn Errors of Immunity Caused by Mutations in the CARD11-BCL10-MALT1 Complex. Front Immunol (2018) 9:2078. doi: 10.3389/fimmu.2018.02078
71. He J, Cai S, Feng H, Cai B, Lin L, Mai Y, et al. Single-Cell Analysis Reveals Bronchoalveolar Epithelial Dysfunction in COVID-19 Patients. Protein Cell (2020) 11(9):680–7. doi: 10.1007/s13238-020-00752-4. 1-8.
72. Ballester B, Milara J, Guijarro R, Morcillo E, Cortijo J. Role of MUC4 in Idiopathic Pulmonary Fibrosis. Eur Respir J (2018) 52(suppl 62):PA4798. doi: 10.1183/13993003.congress-2018.PA4798
73. Ballester B, Milara J, Cortijo J. Mucins as a New Frontier in Pulmonary Fibrosis. J Clin Med (2019) 8(9):1447. doi: 10.3390/jcm8091447
74. Mukhopadhyay P, Lakshmanan I, Ponnusamy MP, Chakraborty S, Jain M, Pai P, et al. MUC4 Overexpression Augments Cell Migration and Metastasis Through EGFR Family Proteins in Triple Negative Breast Cancer Cells. PLoS One (2013) 8(2):e54455–e. doi: 10.1371/journal.pone.0054455
75. Haase M, Fitze G. Hsp90ab1: Helping the Good and the Bad. Gene (2016) 575(2 Pt 1):171–86. doi: 10.1016/j.gene.2015.08.063
76. Noh H, Kim HJ, Yu MR, Kim WY, Kim J, Ryu JH, et al. Heat Shock Protein 90 Inhibitor Attenuates Renal Fibrosis Through Degradation of Transforming Growth Factor-β Type II Receptor. Lab Invest (2012) 92(11):1583–96. doi: 10.1038/labinvest.2012.127
77. Wang H, Deng G, Ai M, Xu Z, Mou T, Yu J, et al. Hsp90ab1 Stabilizes LRP5 to Promote Epithelial–Mesenchymal Transition Via Activating of AKT and Wnt/β-Catenin Signaling Pathways in Gastric Cancer Progression. Oncogene (2019) 38(9):1489–507. doi: 10.1038/s41388-018-0532-5
Keywords: COVID19, SARS-CoV-2, cytokine storm, immunopathogenesis, extrapulmonary tissues, liver, kidney, heart
Citation: Hammoudeh SM, Hammoudeh AM, Bhamidimarri PM, Al Safar H, Mahboub B, Künstner A, Busch H, Halwani R, Hamid Q, Rahmani M and Hamoudi R (2021) Systems Immunology Analysis Reveals the Contribution of Pulmonary and Extrapulmonary Tissues to the Immunopathogenesis of Severe COVID-19 Patients. Front. Immunol. 12:595150. doi: 10.3389/fimmu.2021.595150
Received: 15 August 2020; Accepted: 01 June 2021;
Published: 28 June 2021.
Edited by:
Christiane Moog, Institut National de la Santé et de la Recherche Médicale (INSERM), FranceReviewed by:
Talia H. Swartz, Icahn School of Medicine at Mount Sinai, United StatesCopyright © 2021 Hammoudeh, Hammoudeh, Bhamidimarri, Al Safar, Mahboub, Künstner, Busch, Halwani, Hamid, Rahmani and Hamoudi. This is an open-access article distributed under the terms of the Creative Commons Attribution License (CC BY). The use, distribution or reproduction in other forums is permitted, provided the original author(s) and the copyright owner(s) are credited and that the original publication in this journal is cited, in accordance with accepted academic practice. No use, distribution or reproduction is permitted which does not comply with these terms.
*Correspondence: Rifat Hamoudi, cmhhbW91ZGlAc2hhcmphaC5hYy5hZQ==
Disclaimer: All claims expressed in this article are solely those of the authors and do not necessarily represent those of their affiliated organizations, or those of the publisher, the editors and the reviewers. Any product that may be evaluated in this article or claim that may be made by its manufacturer is not guaranteed or endorsed by the publisher.
Research integrity at Frontiers
Learn more about the work of our research integrity team to safeguard the quality of each article we publish.