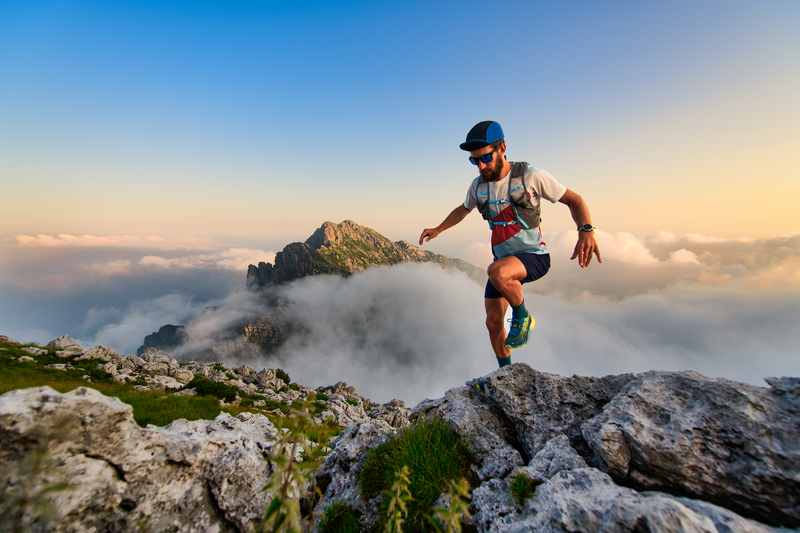
94% of researchers rate our articles as excellent or good
Learn more about the work of our research integrity team to safeguard the quality of each article we publish.
Find out more
ORIGINAL RESEARCH article
Front. Immunol. , 16 February 2021
Sec. Alloimmunity and Transplantation
Volume 11 - 2020 | https://doi.org/10.3389/fimmu.2020.623693
This article is part of the Research Topic Innate Immunity in Kidney Injury, Repair and Fibrosis View all 28 articles
Mesenchymal stem cells (MSCs) have regenerative properties in acute kidney injury (AKI). However, the potential function of MSCs in chronic kidney disease remains elusive. Renal fibrosis is the common endpoint of chronic progressive kidney diseases and causes a considerable health burden worldwide. In this study, the protective effects of bone marrow mesenchymal stem cells (BM-MSCs) were assessed in repeated administration of low-dose cisplatin-induced renal fibrosis mouse model in vivo as well as a TGF-β1-induced fibrotic model in vitro. Differentially expressed miRNAs in mouse renal tubular epithelial cells (mRTECs) regulated by BM-MSCs were screened by high-throughput sequencing. We found microRNA (miR)-146a-5p was the most significant up-regulated miRNA in mRTECs. In addition, the gene Tfdp2 was identified as one target gene of miR-146a-5p by bioinformatics analysis. The expression of Tfdp2 in the treatment of BM-MSCs on cisplatin-induced renal injury was evaluated by immunohistochemistry analysis. Our results indicate that BM-MSC attenuates cisplatin-induced renal fibrosis by regulating the miR-146a-5p/Tfdp2 axis in mRTECs.
Renal fibrosis is characterized by the activation of massive fibroblast and deposition of the fibrotic matrix in kidney tissue, which is considered as the common endpoint of chronic kidney disease (CKD) (1, 2). Renal fibrosis mainly affects over 70-years old adults who account for over 10% of the world population (3). In 2017, the global population of CKDs was about 6.97 million, and patients with CKD in China were approximately 1.32 million. Between 1990 and 2017, the global prevalence of CKD increased by 29.3%, and the global mortality rate increased by 41.5% (4). However, the therapeutic strategies for CKD, such as kidney transplantation or dialysis, are still limited. A shortage of donor organs impedes kidney transplantation, and the cost of dialysis becomes unsustainable, especially in developing countries (5). Therefore, exploring novel and better therapeutic approaches are urgently needed.
Mesenchymal stem cells (MSCs) are multipotent cells with robust capacities of self-renewal, regeneration, proliferation, and differentiating into distinct functional cells (6, 7). MSCs can differentiate into various types of cells, such as osteoblasts, chondrocytes, or adipocytes under appropriate conditions in vivo or in vitro. Therefore, MSCs are widely used in tissue engineering, gene therapy, and immunotherapy (8, 9). The immunomodulatory and paracrine capabilities of MSCs may present the potential for therapeutic capacities in response to local environmental cues. Recent studies have reported that bone marrow MSCs (BM-MSCs) possess regenerative properties and immense plasticity to contribute to their therapeutic effects in organ injury, including acute kidney injury (AKI) and CKD (10–13). However, the mechanisms by which BM-MSCs protect renal tissues are complex, such as preventing renal tubular epithelial cell apoptosis, promoting renal tubular epithelial cell proliferation, and modulating the immune system by various cytokines and growth factors (14). MicroRNAs (miRNAs) are endogenous non-coding RNAs with a length of about 21–24 nucleotides, which regulate protein translation by combining with complementary sequences in the 3’non-coding region (3’UTR) various mRNAs (15). Recently, it has been widely accepted that miRNAs play vital roles in the progression of kidney disease (16). Specific miRNAs are associated with the initiation and development of renal fibrosis by anti- or pro-fibrogenic effects (17).
In the present study, we established a mouse model with renal fibrosis by repeated administration of low-dose cisplatin. The protective effects of BM-MSCs have been examined in vivo as well as in TGF-β1-treated mouse renal tubular epithelial cells (mRTECs) with an indirect co-culture environment. Next, we performed a high-throughput sequencing to identify significantly regulated miRNAs by mRTECs and probe further the underlying indirect molecular mechanism of miRNAs in the therapeutic process of BM-MSCs.
Experimental protocols were approved by the Animal Ethics Committee of Soochow University and were performed following the guidelines for use and care of laboratory animals of the Experimental Animal Committee of Soochow University (Approval No : ECSU-201800099).
Eight-week-old male C57BL/6 mice were purchased from Zhao Yan Animals Co., Ltd. (Suzhou, China). On arrival, the mice were housed under antiviral and antibody-free micro-isolator conditions and fed a standard diet. Previous studies reported that multiple administrations of cisplatin cause renal fibrosis (18). We established and developed kidney injury and fibrosis C57BL/6 mice models using a low dose treatment of cisplatin once a week for 6 weeks. The mice were randomly divided into three groups as normal control (NC) group, cisplatin group, and cisplatin + BM-MSC group (n=6 for each group): 1) NC group: mice underwent intraperitoneal injection of 0.9% NaCl solution 10μl/g, once a week; from the 4th week underwent injection of 0.9% NaCl solution 0.4 ml through the tail vein, once per week. 2) Cisplatin group: intraperitoneal injection of 3.5mg/kg cisplatin solution according to the bodyweight of the mouse, once per week; from the 4th week, 0.9% NaCl solution 0.4 ml was injected through the tail vein once per week. 3) Cisplatin + BM-MSC group: intraperitoneal injection of 3.5mg/kg cisplatin solution according to the bodyweight of the mouse, once per week; from the 4th week, injection with normal saline containing 1.5×106/ml BM-MSCs, 0.4 ml per mouse, once a week. At 6th weeks post-injection, the mice were sacrificed, the kidneys and serum from all mice were collected for further investigation.
mRTECs were obtained from ScienCell Research Laboratories. BM-MSCs were gifted by Prof. Dr. Yufang Shi’s Laboratory, Soochow University. mRTECs and BM-MSCs were cultured in DMEM, low glucose medium (HyClone) with 10% FBS (Gibco), 100 units/ml penicillin, and 100 μg/ml streptomycin. All cells were maintained at 37°C in a humidified atmosphere with 5% CO2. mRTECs were seeded in 6-well plates at 1×105/well, and TGF-β1 (10 ng/ml) was added into the medium. After 48 h of stimulation, the medium of each group was changed to a complete medium without exogenous cytokine TGF-β1. A Transwell system was selected to establish the indirect co−culture environment. BM-MSCs were added to the transwell chamber at 1×105/well and indirectly co-cultured with mRTECs in a 37°C incubator for 24 h. A complete medium without BM-MSCs was added to the upper chamber for the control group.
C57BL/6 mouse kidney tissues were sectioned and fixed in formalin. Paraffin-embedded tissues were stained with Hematoxylin and eosin (H&E), Masson trichrome, and Sirius red. Sirius red staining was performed following the Sirius Red Stain Kit (Yuanye Bio-Technology, Shanghai, China). Interstitial fibrosis areas were assessed using microscopy (Olympus) by examining five randomly selected fields (×100) of the cortex. The staining figures were evaluated independently by two pathologists and quantified by histograms.
High-throughput sequencing of miRNA was performed by Cloud-seq Biotechnology Co., Ltd (Shanghai, China). Isolation of miRNA from cultured cells and tissues was performed by using the Total RNA Miniprep Purification Kit (GeneMark). Grind fresh mouse kidney tissues with an electric grinder, total RNA was extracted from mRTECs, and grated kidney tissues by 1ml TRIzol and miRNA were isolated according to the manufacture’s instructions. Reverse transcription for specific miRNAs was performed using 2 μg miRNA and respective primers for reverse transcription (listed in Supplementary Table 1) according to the Stem-loop method. Quantitative real-time PCR was carried out using the ABsolute qPCR SYBR Green ROX Mix (Thermo Fisher Scientific) and the following cycling condition: 95°C for 10 min, 40 cycles of 95°C for 15 s, and 60°C for 60 s. StepOne Plus device (Thermo Fisher Scientific) was used for detection. The snRNA U6 was used for normalization.
The protein was extracted from fresh tissues and mRTECs by using RIPA lysate. The total protein concentration was measured using a BCA protein quantitative kit and denatured by heating. The protein samples were assessed by 10% sodium dodecyl sulfate polyacrylamide gel electrophoresis (SDS-PAGE), semi-dry transferred to polyvinylidene fluoride (PVDF), and incubated with primary antibody against α-SMA (1:350, Affinity, USA) and Col1α1 (1:500, Abcam, USA) at 4°C overnight. Next, after being washed by TBST three times and incubated with a specific secondary antibody (1:3,000; Boster, China) for 2 h at room temperature. Immunoreactivity was visualized using an ECL detection system.
Cells were transfected with 50nM miR-146a-5p mimics/inhibitors or Tfdp2 siRNA (RiboBio Co, Guangzhou, China) when the cell density reached 80–90% prior. Lipofectamine 2000 reagent (Invitrogen, No. 1070962) was used for siRNA transfection. The cells were transferred to low glucose-DMEM with 10% FBS complete medium 6 h after transfection. Cells were harvested after 24 h.
C57BL/6 mouse kidney tissues were sectioned and fixed in formalin. After the sections were dewaxed, antigen repaired, and serum sealed, Incubate membrane and Tfdp2 primary antibody (1:200, Abcam, ab235830) overnight at 4°C. PBS was used to wash the sections, and then the samples were incubated in biotinylated anti-rabbit secondary antibody (1:200, Beyotime, China).
All data are expressed as the mean + standard error of the mean. Multiple comparisons were performed using Student’s t-test and one-way ANOVA. Statistical analysis was processed by Prism 8 software (GraphPad Software, CA). Differences between mean values were considered statistically significant at P < 0.05.
We established and developed kidney injury and fibrosis C57BL/6 mice models using a low dose treatment of cisplatin once a week for 6 weeks (cisplatin group). To explore the effect of BM-MSCs on renal injury and fibrosis, mice were injected with the suspension of BM-MSCs via the tail vein (cisplatin +BM-MSCs group) (Figure 1A). All mice were euthanized at 6 weeks after the first cisplatin administration. Compared to the normal control group (NC), the volume and weight of kidneys in the cisplatin group and BM-MSCs-treated group were obviously reduced, which displayed granular or nodular surface. There was no significant difference in the appearance of the kidney between cisplatin group and cisplatin +BM-MSCs group (Figure 1B). To characterize this phenotype in more details, histologic and molecular tests were performed. H&E staining, Masson staining (collagen fibers marked blue), and Sirius red staining (collagen fibers marked red) showed that the fibrotic areas of the cisplatin group were significantly induced as compared to NC group kidneys. After injected the suspension of BM-MSCs via the tail vein, the fibrotic areas were reduced as compared with the cisplatin group (Figure 1C, Supplementary Figures 1A, B). Next, the biomarkers of kidney function and injury in serum were measured. The apparent elevation of serum creatinine (SCr) and blood urea nitrogen (BUN) levels was observed in the cisplatin group. Only the BUN level was significantly decreased by the treatment of BM-MSCs (Figures 1D, E). Analysis of fibrotic gene markers by RT-qPCR showed that the transcript levels of α-SMA and Col1α1 were significantly elevated in the cisplatin group compared to NC kidney tissues, while injection of BM-MSCs reduced the levels of α-SMA and Col1α1 (Figures 1F, G). The findings were confirmed by western blot analysis in protein level (Figure 1H). These results demonstrated that multiple administrations of cisplatin induced renal injury and fibrosis in mice, which could be attenuated by treatment with BM-MSCs through decreasing the expression of α-SMA and Col1α1.
Figure 1 The effect of bone marrow mesenchymal stem cells (BM-MSCs) on cisplatin-induced renal injury and fibrosis in vivo. (A) The schematic diagram of mice infected with cisplatin solution intraperitoneally and suspension of BM-MSCs via the tail vein. (B) Image of the morphology of kidney in mice infected cisplatin solution intraperitoneally and suspension of BM-MSCs via the tail vein. (C) H&E, Masson, and Sirius red staining. (D, E) serum creatinine (SCr) and blood urea nitrogen (BUN) levels of serum in mice were determined. The relative mRNA expression levels (F, G) and the protein levels (H) of α-SMA and Col1α1 in mice kidney tissues were evaluated by real-time PCR and Western blot, respectively. Data represent the mean ± SE from three independent experiments. *P < 0.05; **P < 0.01; ***P < 0.001; NS, not significant.
To verify whether BM-MSCs had a therapeutic effect on renal fibrosis in vitro, we used exogenous cytokine TGF-β1 to stimulate mRTECs for 48h and to establish a fibrotic model in vitro. Subsequently, TGF-β1-treated mRTECs were divided into two groups: with or without BM-MSCs co-cultured treatment for 24 h. The expression of α-SMA and Col1α1 in mRTECs were detected by RT-qPCR analysis in transcript level (Figures 2A, B) and western blot analysis in protein level (Figure 2C). Our results revealed that both transcriptional and translational levels of α-SMA and Col1α1 in mRTECs were significantly reduced while co-cultured with BM-MSCs, which suggested that BM-MSCs could alleviate fibrosis in mRTECs.
Figure 2 The effect of bone marrow mesenchymal stem cells (BM-MSCs) on TGF-β1-induced a fibrotic model in vitro. The relative mRNA expression levels (A, B) and the protein levels (C) of α-SMA and Col1α1 in mRTECs were evaluated by real-time PCR and Western blot, respectively. Data represent the mean ± SE from three independent experiments. ***P < 0.001; NS, not significant.
To screen the differentially expressed miRNAs in mRTECs regulated by BM-MSCs, we performed a miRNA high-throughput sequencing (series GSE148144) for TGF-β1-treated mRTECs with or without BM-MSCs co-culture. The heatmap of all the different expressed miRNAs was shown in Figure 3A. We discovered a panel of miRNAs (miR-146a-5p, miR-12194-3p, miR-210-3p, miR-210-5p, let-7i-3p) with the top-five most significant changes in two groups, which were verified by RT-qPCR analysis and revealed that miR-146a-5p was the most significant up-regulated miRNA (Figure 3B). According to these results, we confirmed that BM-MSCs exert the anti-fibrosis function by regulating miR-146a-5p expression in mRTECs.
Figure 3 miR-146a-5p was significantly up-regulated by bone marrow mesenchymal stem cells (BM-MSCs) in mouse renal tubular epithelial cells (mRTECs). (A) Heatmap of differential microRNA (miRNA) expression in TGF-β1-treated mRTECs with or without BM-MSCs. Gene expression data were obtained with miRNA sequence and the most obvious values are shown. Red indicates up-regulation, while green means down-regulation. (B) Top-five most significant changed miRNAs were verified by real-time PCR. (C) TGF-β1-treated mRTECs were transfected with miR-146a-5p mimics. The levels of miR-146a-5p were determined by real-time PCR. The relative mRNA expression levels (D, E) and the protein levels (F) of α-SMA and Col1α1 in mRTECs transfected with miR-146a-5p mimics were evaluated by real-time PCR and Western blot, respectively. (G) TGF-β1-treated mRTECs were transfected with miR-146a-5p inhibitor. The levels of miR-146a-5p were determined by real-time PCR. The relative mRNA expression levels (H, I) and the protein levels (J) of α-SMA and Col1α1 in mRTECs transfected with miR-146a-5p inhibitor were evaluated by real-time PCR and Western blot, respectively. Data represent the mean ± SE from three independent experiments. *P < 0.05; **P < 0.01; ***P < 0.001; NS, not significant.
To investigate the function of miR-146a-5p regulated by BM-MSCs in the progression of renal fibrosis in vitro, we transiently transfected miR-146a-5p mimics into TGF-β1-treated mRTECs to significantly up-regulate the expression of miR-146a-5p (Figure 3C). In line with the previous results, both transcriptional and translational levels of α-SMA and Col1α1 in mRTECs were significantly reduced by the miR-146a-5p mimics treatment (Figures 3D–F). Next, we transiently transfected miR-146a-5p inhibitors into TGF-β1-treated mRTECs to down-regulate miR-146a-5p expression (Figure 3G). The RT-qPCR analysis and western blot analysis showed that loss of miR-146a-5p significantly increased the expression of α-SMA and Col1α1 in mRTECs (Figures 3H–J). In summary, gain- or loss-function of miR-146a-5p studies by mimic and inhibitor revealed that BM-MSCs might exert its anti-fibrosis effect by increasing the expression of miR-146a-5p in mRTECs.
We predicted the potential target genes of miR-146a-5p using multiple software programs (TargetScan, miRanda, miRDB, and DIANA-microT). Totally, 59 candidate genes were identified (Figure 4A). Next, gene ontology (GO) and Kyoto Encyclopedia of Genes and Genomes (KEGG) analysis were performed to evaluate mRNA enrichments in terms of biological process, cellular component, and molecular function. The top 10 enriched GO terms were involved in the regulation of cellular and metabolic processes (Figure 4B). KEGG analysis revealed two pathways, including the cell cycle and the pathways in cancer (Figure 4C), which are associated with cell activation and cell proliferation. Our results suggested that the differentially expressed miRNAs might be involved in the progression of renal fibrosis.
Figure 4 Bioinformatic prediction of miR-146a-5p target genes in mRTECs. (A) Venn diagram of identified miR-146a-5p target genes using multiple software programs (TargetScan, miRanda, miRDB, and DIANA-microT). Gene ontology (GO) analysis (B) and Kyoto Encyclopedia of Genes and Genomes (KEGG) analysis (C) of candidate genes in terms of biological process, cellular component, and molecular function.
Next, six candidate target genes of miR-146a-5p were selected from the KEGG pathway analysis. Expression of one candidate gene Tfdp2 was increased by exogenous cytokine TGF-β1 stimulation and decreased after co-cultured with BM-MSCs in mRTECs (Figure 5A). Whereas the expression of the other five candidate target genes (Smad4, Traf6, Rbl1, Ar, Rarb) revealed no statistical significance (Supplementary Figures 1A–E). The experimental data confirmed Tfdp2 gene as a candidate target gene of miR-146a-5p. To explore the direct relationship between Tfdp2 and miR-146a-5p, the expression of Tfdp2 mRNA and protein in mRTECs transfected by miR-146a-5p mimics was detected by RT-qPCR and western blot analysis. Our data showed that both mRNA and protein levels of Tfdp2 were decreased significantly compared with control (Figures 5B, C). Moreover, the mRNA level of Tfdp2 was gradually decreased depending on the miR-146a-5p mimic transfection concentration (Figure 5D). In summary, our experimental data indicate that Tfdp2 is the target gene of miR-146a-5p.
Figure 5 Verification of one candidate gene Tfdp2 in mouse renal tubular epithelial cells (mRTECs). (A) The levels of Tfdp2 were determined by real-time PCR in TGF-β1-treated mRTECs with or without bone marrow mesenchymal stem cells (BM-MSCs). The relative mRNA expression levels (B) and the protein levels (C) of Tfdp2 in mRTECs transfected by miR-146a-5p mimics were evaluated by real-time PCR and Western blot, respectively. (D) The transcript levels of Tfdp2 in mRTECs transfected by miR-146a-5p mimics with different concentrations. Data represent the mean ± SE from three independent experiments. *P < 0.05; **P < 0.01; ***P < 0.001.
To evaluate the expression and function of Tfdp2 in renal fibrosis, we first transiently transfected Tfdp2 siRNA into mRTECs to down-regulate the expression of Tfdp2 in mRTECs (Figure 6A). Both transcriptional and translational levels of α-SMA and Col1α1 in mRTECs were significantly reduced by the knockdown of Tfdp2 (Figures 6B–D). These results suggested that inhibition of Tfdp2 expression can reduce the expression of fibrosis-related proteins. IHC analysis showed that a weak staining of Tfdp2 was predominantly localized to the cytoplasm in the normal tubulointerstitial compartment which was utilized as a reference. Stronger staining of Tfdp2 in renal tubular epithelial cells was observed in the renal tissues of the cisplatin group as compared with the control group, whereas the expression of Tfdp2 was obviously reduced in cisplatin + BM-MSCs group as compared to the cisplatin group. Taken together, these experimental data indicated that Tfdp2 exerts a significant function in the treatment of BM-MSCs on cisplatin -induced renal injury and fibrosis.
Figure 6 Overexpression of Tfdp2 in cisplatin-induced renal fibrosis. (A) The transcript levels of Tfdp2 in mouse renal tubular epithelial cells (mRTECs) was down-regulated by siRNA. The relative mRNA expression levels (B, C) and the protein levels (D) of α-SMA and Col1α1 after knockdown of Tfdp2 were by real-time PCR and Western blot, respectively. (E) Representative images of Tfdp2 immunohistochemical staining in cisplatin (CP)-induced mice kidney with or without bone marrow mesenchymal stem cells (BM-MSCs) treatment. Data represent the mean ± SE from three independent experiments. **P < 0.01; ***P < 0.001.
MSCs-based therapeutic approaches have been successfully applied to attenuate organ injury, including the kidney. Kidney fibrosis is a common endpoint outcome of CKD, which has a poor clinical outcome (19, 20). BM-MSCs are extracted from bone marrow and are defined as multipotent cells due to the robust capacities of differentiating into distinct functional cells. Most data reveal that BM-MSCs repair renal injury processes by the means of indirect secreted biomaterial, including the extracellular vesicles and multiple miRNA particles (21). In the present study, we established a model of cisplatin-induced renal fibrosis in C57BL/6 mice and found that BM-MSCs have an anti-renal fibrosis effect and renoprotection in vivo. Moreover, we showed that BM-MSCs significantly reduced the levels of α-SMA and Col1α1 in mRTECs treated by TGF-β1 in an indirect co-culture environment. Mechanically, we have identified miR-146a-5p as the most significant up-regulated miRNA in mRTECs regulated by BM-MSCs and plays a significant role in renal fibrosis in a co-culture experimental model. The results also found the gene Tfdp2 is one target gene of miR-146a-5p and exerts a vital role in the treatment of BM-MSCs on cisplatin-induced renal injury and fibrosis. Our study indicates that BM-MSC attenuates cisplatin-induced renal fibrosis by regulating miR-146a-5p/Tfdp2 axis in mRTECs.
Renal tubulointerstitial fibrosis is generally characterized by an excessive accumulation of extracellular matrix (ECM) factors (predominantly collagen type I) that prevent the regeneration of kidney tissue (22, 23). The severity of fibrosis notably correlates with the degree of kidney dysfunction and the renal failure outcome (24). TGF-β is well accepted as a critical regulator in renal fibrosis. Growing evidence indicates that exogenous cytokine, such as TGF-β1, can induce renal tubular epithelial cells to undergo phenotypic transformation into matrix-producing myofibroblasts (25, 26). Our study successfully established a mouse model to investigate the multiple low-dose cisplatin-induced renal fibrosis, which could be determined by increased levels of α-SMA and Col1α1 in mRTECs. In line with previous studies (11), we found BM-MSCs alleviate the severity of kidney dysfunction and fibrosis. Asanuma et al. (27). found arterially delivered MSCs protect against obstruction induced epithelial-mesenchymal transition (EMT) and chronic renal fibrosis involving alterations in TNF-α production. MSCs-based therapy was also reported to attenuate renal fibrosis through immune response modulation (28). However, the specific mechanisms of MSCs renoprotection remain poorly elucidative.
As a post-transcriptional regulator, miRNAs play an important role in the development, physiology, and maintenance of kidney microstructure. Dysregulation of miRNAs disrupts early kidney development, differentiation of kidney progenitor cells, and mature nephrons’ maintenance (29). Recent studies indicate that dysregulation of miRNAs is associated with the initiation and development of renal fibrosis in patients with diabetic nephropathy (DN) as well as in mouse models of DN (30, 31). Based on the function of BM-MSCs and miRNAs in renal fibrosis, we performed a high-throughput sequencing to identify the most significant regulated miRNAs by BM-MSCs in a co-culture experimental model. MiR-146a-5p was found as the most significant up-regulated miRNA, which was also verified by RT-PCR. Subsequently, we explored the function of miR-146a-5p in renal fibrosis in vitro. The gain- and loss-function of miR-146a-5p studies by mimic and inhibitor showed that the expression of α-SMA, Col1α1 mRNA, and protein were significantly altered in mRTECs. Therefore, our results indicate that BM-MSCs could inhibit the progression of renal fibrosis by regulating the expression of miR-146a-5p in mRTECs. Interestingly, a previous study has reported that miR-146a-5p acts as a negative regulator of TGF-β signaling in skeletal muscle after acute contusion, which indicates that miR-146 might have a therapeutic potential to alleviate skeletal muscle fibrosis (32). In addition, miR-146a-5p was found to inhibit the activation and proliferation of hepatic stellate cells (HSCs) in the progress of non-alcoholic fibrosing steatohepatitis (33). Therefore, miR-146a-5p exerts a critical function in the renoprotection of BM-MSCs.
Six candidate genes from the KEGG pathway analyses were confirmed by RT-qPCR. We found the transcription factor DP2 (Tfdp2) gene was increased in mRTECs stimulated by exogenous cytokine TGF-β1 and reduced after co-cultured with BM-MSCs. Consistent with miR-146a-5p targeting mRNA of Tfdp2, expression of Tfdp2 in mRTECs was significantly altered by the mimic and inhibitor of miR-146a-5p. To further investigate the function of Tfdp2 in renal fibrosis, Tfdp2 expression was knockdown by siRNA transfection. Both transcriptional and translational levels of α-SMA and Col1α1 in mRTECs were significantly reduced by the knockdown of Tfdp2. In addition, the relevance of Tfdp2 in renal fibrosis was confirmed in cisplatin-treated mouse kidney tissues by the IHC staining. Tfdp2 is a significant cofactor for the cell cycle control and gene expression, which combines with E2F family members to drive the function of c-Myc gene (34). The whole-genome analysis report for chronic kidney disease shows that Tfdp2 is related to renal function and CKD (35, 36). In the present study, we provided the first experimental evidence that BM-MSCs attenuate cisplatin-induced renal injury and fibrosis by the regulation of miR-146a-5p and the targeting gene Tfdp2, indicating that BM-MSCs may be a promising approach for developing novel therapeutics to treat renal fibrosis.
Nevertheless, our findings are only confirmed in the mouse model. The value and significance of BM-MSCs for the clinical treatment of renal fibrosis require more preclinical data support. In addition, the contribution of miR-146a-5p/Tfdp2 axis in renal fibrosis is worth further investigating and verifying in human kidney tissues.
In conclusion, our findings demonstrate that BM-MSCs ameliorate renal injury and fibrosis in vivo and in vitro. Our study provides the first evidence that the protection of BM-MSCs on renal fibrosis via miR-146a-5p/Tfdp2 axis in renal tubular epithelial cells. These results offer novel mechanistic insights into the renoprotection of BM-MSCs, which highlights the promising clinical studies on MSCs-based therapy for kidney diseases in the near future. Additionally, the function of miR-146a-5p/Tfdp2 axis in renal fibrosis should be further investigated in preclinical and clinical studies, which might be a potential therapeutic target for renal fibrosis.
The datasets presented in this study can be found in online repositories. The names of the repository/repositories and accession number(s) can be found below: https://www.ncbi.nlm.nih.gov/geo/, GSE148144.
The animal study was reviewed and approved by Experimental Animal Committee of Soochow University (Approval No: ECSU-201800099).
S-LW and M-MS conceived and guided the study. LW, QZ, XZ, and X-MZ performed the experiments. LW, CR, and SW analyzed and interpreted the data. LW and CR contributed to the writing and data presentation of the manuscript. All authors contributed to the article and approved the submitted version.
This study was supported by the grants from the National Key R&D Program of China (No. 2018YFA0107500), Natural Science Foundation of Jiangsu Province (No. BK20200878), and the Priority Academic Program Development of Jiangsu Higher Education Institutions (PAPD).
The authors declare that the research was conducted in the absence of any commercial or financial relationships that could be construed as a potential conflict of interest.
We thank Prof. Dr. Yufang Shi for the establishment of BM-MSCs and technical assistance from his laboratory.
The Supplementary Material for this article can be found online at: https://www.frontiersin.org/articles/10.3389/fimmu.2020.623693/full#supplementary-material
1. Boor P, Ostendorf T, Floege J. Renal fibrosis: novel insights into mechanisms and therapeutic targets. Nat Rev Nephrol (2010) 6(11):643–56. doi: 10.1038/nrneph.2010.120
2. O’Sullivan ED, Hughes J, Ferenbach DA. Renal Aging: Causes and Consequences. J Am Soc Nephrol (2017) 28(2):407–20. doi: 10.1681/ASN.2015121308
3. Webster AC, Nagler EV, Morton RL, Masson P. Chronic Kidney Disease. Lancet (2017) 389(10075):1238–52. doi: 10.1016/S0140-6736(16)32064-5
4. Collaboration GBDCKD. Global, regional, and national burden of chronic kidney disease, 1990-2017: a systematic analysis for the Global Burden of Disease Study 2017. Lancet (2020) 395(10225):709–33. doi: 10.1016/S0140-6736(20)30045-3
5. Alicic RZ, Rooney MT, Tuttle KR. Diabetic Kidney Disease: Challenges, Progress, and Possibilities. Clin J Am Soc Nephrol (2017) 12(12):2032–45. doi: 10.2215/CJN.11491116
6. Wei X, Yang X, Han ZP, Qu FF, Shao L, Shi YF. Mesenchymal stem cells: a new trend for cell therapy. Acta Pharmacol Sin (2013) 34(6):747–54. doi: 10.1038/aps.2013.50
7. Weiss ARR, Dahlke MH. Immunomodulation by Mesenchymal Stem Cells (MSCs): Mechanisms of Action of Living, Apoptotic, and Dead MSCs. Front Immunol (2019) 10:1191. doi: 10.3389/fimmu.2019.01191
8. Brown C, McKee C, Bakshi S, Walker K, Hakman E, Halassy S, et al. Mesenchymal stem cells: Cell therapy and regeneration potential. J Tissue Eng Regener Med (2019) 13(9):1738–55. doi: 10.1002/term.2914
9. Ridge SM, Sullivan FJ, Glynn SA. Mesenchymal stem cells: key players in cancer progression. Mol Cancer (2017) 16(1):31. doi: 10.1186/s12943-017-0597-8
10. Uccelli A, Moretta L, Pistoia V. Mesenchymal stem cells in health and disease. Nat Rev Immunol (2008) 8(9):726–36. doi: 10.1038/nri2395
11. El Agha E, Kramann R, Schneider RK, Li X, Seeger W, Humphreys BD, et al. Mesenchymal Stem Cells in Fibrotic Disease. Cell Stem Cell (2017) 21(2):166–77. doi: 10.1016/j.stem.2017.07.011
12. Herzog EL, Chai L, Krause DS. Plasticity of marrow-derived stem cells. Blood (2003) 102(10):3483–93. doi: 10.1182/blood-2003-05-1664
13. Zhang F, Wang C, Wen X, Chen Y, Mao R, Cui D, et al. Mesenchymal stem cells alleviate rat diabetic nephropathy by suppressing CD103(+) DCs-mediated CD8(+) T cell responses. J Cell Mol Med (2020) 24(10):5817–31. doi: 10.1111/jcmm.15250
14. Rabelink TJ, Little MH. Stromal cells in tissue homeostasis: balancing regeneration and fibrosis. Nat Rev Nephrol (2013) 9(12):747–53. doi: 10.1038/nrneph.2013.152
15. O’Reilly S. MicroRNAs in fibrosis: opportunities and challenges. Arthritis Res Ther (2016) 18:11. doi: 10.1186/s13075-016-0929-x
16. Chandrasekaran K, Karolina DS, Sepramaniam S, Armugam A, Wintour EM, Bertram JF, et al. Role of microRNAs in kidney homeostasis and disease. Kidney Int (2012) 81(7):617–27. doi: 10.1038/ki.2011.448
17. Chung AC, Lan HY. MicroRNAs in renal fibrosis. Front Physiol (2015) 6:50. doi: 10.3389/fphys.2015.00050
18. Sharp CN, Doll MA, Dupre TV, Shah PP, Subathra M, Siow D, et al. Repeated administration of low-dose cisplatin in mice induces fibrosis. Am J Physiol Renal Physiol (2016) 310(6):F560–8. doi: 10.1152/ajprenal.00512.2015
19. Zhao L, Han F, Wang J, Chen J. Current understanding of the administration of mesenchymal stem cells in acute kidney injury to chronic kidney disease transition: a review with a focus on preclinical models. Stem Cell Res Ther (2019) 10(1):385. doi: 10.1186/s13287-019-1507-3
20. Ruiz-Ortega M, Rayego-Mateos S, Lamas S, Ortiz A, Rodrigues-Diez RR. Targeting the progression of chronic kidney disease. Nat Rev Nephrol (2020) 16(5):269–88. doi: 10.1038/s41581-019-0248-y
21. Bochon B, Kozubska M, Surygala G, Witkowska A, Kuzniewicz R, Grzeszczak W, et al. Mesenchymal Stem Cells-Potential Applications in Kidney Diseases. Int J Mol Sci (2019) 20(10):2462. doi: 10.3390/ijms20102462
22. Liu Y. Cellular and molecular mechanisms of renal fibrosis. Nat Rev Nephrol (2011) 7(12):684–96. doi: 10.1038/nrneph.2011.149
23. Hong S, Healy H, Kassianos AJ. The Emerging Role of Renal Tubular Epithelial Cells in the Immunological Pathophysiology of Lupus Nephritis. Front Immunol (2020) 11:578952. doi: 10.3389/fimmu.2020.578952
24. Law BMP, Wilkinson R, Wang X, Kildey K, Giuliani K, Beagley KW, et al. Human Tissue-Resident Mucosal-Associated Invariant T (MAIT) Cells in Renal Fibrosis and CKD. J Am Soc Nephrol (2019) 30(7):1322–35. doi: 10.1681/ASN.2018101064
25. Meng XM, Nikolic-Paterson DJ, Lan HY. TGF-beta: the master regulator of fibrosis. Nat Rev Nephrol (2016) 12(6):325–38. doi: 10.1038/nrneph.2016.48
26. Tang PM, Zhang YY, Mak TS, Tang PC, Huang XR, Lan HY. Transforming growth factor-beta signalling in renal fibrosis: from Smads to non-coding RNAs. J Physiol (2018) 596(16):3493–503. doi: 10.1113/JP274492
27. Asanuma H, Vanderbrink BA, Campbell MT, Hile KL, Zhang H, Meldrum DR, et al. Arterially delivered mesenchymal stem cells prevent obstruction-induced renal fibrosis. J Surg Res (2011) 168(1):e51–9. doi: 10.1016/j.jss.2010.06.022
28. Semedo P, Correa-Costa M, Antonio Cenedeze M, Maria Avancini Costa Malheiros D, Antonia dos Reis M, Shimizu MH, et al. Mesenchymal stem cells attenuate renal fibrosis through immune modulation and remodeling properties in a rat remnant kidney model. Stem Cells (2009) 27(12):3063–73. doi: 10.1002/stem.214
29. Fan Y, Chen H, Huang Z, Zheng H, Zhou J. Emerging role of miRNAs in renal fibrosis. RNA Biol (2020) 17(1):1–12. doi: 10.1080/15476286.2019.1667215
30. Kato M, Zhang J, Wang M, Lanting L, Yuan H, Rossi JJ, et al. MicroRNA-192 in diabetic kidney glomeruli and its function in TGF-beta-induced collagen expression via inhibition of E-box repressors. Proc Natl Acad Sci USA (2007) 104(9):3432–7. doi: 10.1073/pnas.0611192104
31. Wang Q, Wang Y, Minto AW, Wang J, Shi Q, Li X, et al. MicroRNA-377 is up-regulated and can lead to increased fibronectin production in diabetic nephropathy. FASEB J (2008) 22(12):4126–35. doi: 10.1096/fj.08-112326
32. Sun Y, Li Y, Wang H, Li H, Liu S, Chen J, et al. miR-146a-5p acts as a negative regulator of TGF-beta signaling in skeletal muscle after acute contusion. Acta Biochim Biophys Sin (Shanghai) (2017) 49(7):628–34. doi: 10.1093/abbs/gmx052
33. Du J, Niu X, Wang Y, Kong L, Wang R, Zhang Y, et al. MiR-146a-5p suppresses activation and proliferation of hepatic stellate cells in nonalcoholic fibrosing steatohepatitis through directly targeting Wnt1 and Wnt5a. Sci Rep (2015) 5:16163. doi: 10.1038/srep16163
34. Chen C, Lodish HF. Global analysis of induced transcription factors and cofactors identifies Tfdp2 as an essential coregulator during terminal erythropoiesis. Exp Hematol (2014) 42(6):464–76.e5. doi: 10.1016/j.exphem.2014.03.001
35. Boger CA, Heid IM. Chronic kidney disease: novel insights from genome-wide association studies. Kidney Blood Press Res (2011) 34(4):225–34. doi: 10.1159/000326901
Keywords: bone marrow mesenchymal stem cells, renal fibrosis, microRNA, TGF-β1, renal tubular epithelial cells
Citation: Wu L, Rong C, Zhou Q, Zhao X, Zhuansun X-M, Wan S, Sun M-M and Wang S-L (2021) Bone Marrow Mesenchymal Stem Cells Ameliorate Cisplatin-Induced Renal Fibrosis via miR-146a-5p/Tfdp2 Axis in Renal Tubular Epithelial Cells. Front. Immunol. 11:623693. doi: 10.3389/fimmu.2020.623693
Received: 30 October 2020; Accepted: 24 December 2020;
Published: 16 February 2021.
Edited by:
Cheng Yang, Fudan University, ChinaCopyright © 2021 Wu, Rong, Zhou, Zhao, Zhuansun, Wan, Sun and Wang. This is an open-access article distributed under the terms of the Creative Commons Attribution License (CC BY). The use, distribution or reproduction in other forums is permitted, provided the original author(s) and the copyright owner(s) are credited and that the original publication in this journal is cited, in accordance with accepted academic practice. No use, distribution or reproduction is permitted which does not comply with these terms.
*Correspondence: Mao-Min Sun, c3VubWFvbWluQHN1ZGEuZWR1LmNu; Shou-Li Wang, d2FuZ3Nob3VsaUBzdWRhLmVkdS5jbg==
†These authors have contributed equally to this work
Disclaimer: All claims expressed in this article are solely those of the authors and do not necessarily represent those of their affiliated organizations, or those of the publisher, the editors and the reviewers. Any product that may be evaluated in this article or claim that may be made by its manufacturer is not guaranteed or endorsed by the publisher.
Research integrity at Frontiers
Learn more about the work of our research integrity team to safeguard the quality of each article we publish.