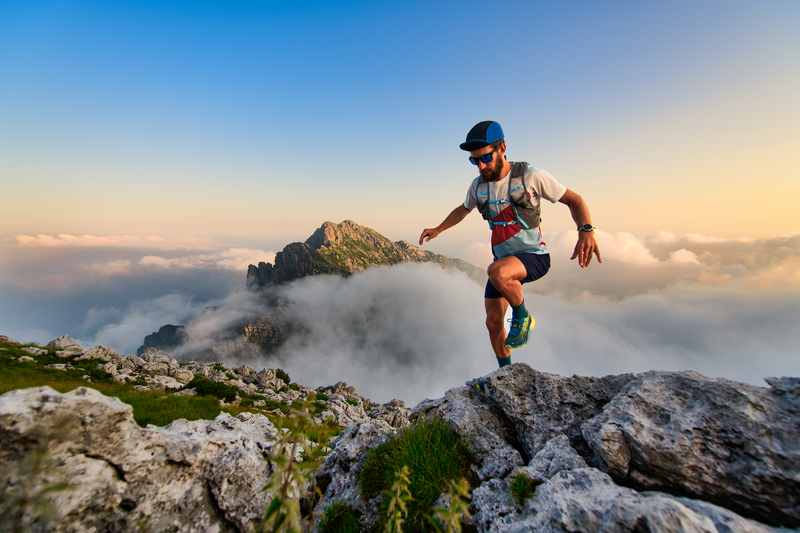
94% of researchers rate our articles as excellent or good
Learn more about the work of our research integrity team to safeguard the quality of each article we publish.
Find out more
REVIEW article
Front. Immunol. , 19 January 2021
Sec. Molecular Innate Immunity
Volume 11 - 2020 | https://doi.org/10.3389/fimmu.2020.622598
Surfactant proteins A (SP-A) and D (SP-D) are soluble innate immune molecules which maintain lung homeostasis through their dual roles as anti-infectious and immunomodulatory agents. SP-A and SP-D bind numerous viruses including influenza A virus, respiratory syncytial virus (RSV) and human immunodeficiency virus (HIV), enhancing their clearance from mucosal points of entry and modulating the inflammatory response. They also have diverse roles in mediating innate and adaptive cell functions and in clearing apoptotic cells, allergens and other noxious particles. Here, we review how the properties of these first line defense molecules modulate inflammatory responses, as well as host-mediated immunopathology in response to viral infections. Since SP-A and SP-D are known to offer protection from viral and other infections, if their levels are decreased in some disease states as they are in severe asthma and chronic obstructive pulmonary disease (COPD), this may confer an increased risk of viral infection and exacerbations of disease. Recombinant molecules of SP-A and SP-D could be useful in both blocking respiratory viral infection while also modulating the immune system to prevent excessive inflammatory responses seen in, for example, RSV or coronavirus disease 2019 (COVID-19). Recombinant SP-A and SP-D could have therapeutic potential in neutralizing both current and future strains of severe acute respiratory syndrome coronavirus 2 (SARS-CoV-2) virus as well as modulating the inflammation-mediated pathology associated with COVID-19. A recombinant fragment of human (rfh)SP-D has recently been shown to neutralize SARS-CoV-2. Further work investigating the potential therapeutic role of SP-A and SP-D in COVID-19 and other infectious and inflammatory diseases is indicated.
Surfactant proteins A (SP-A) and D (SP-D) are essential innate immune molecules with important roles in lung health (1–3). These work to both neutralize and enhance the clearance of pathogens while modulating the inflammatory response (4, 5). SP-A and SP-D play key roles in keeping the lungs in a non-inflamed and infection-free homeostatic state to ensure efficient gaseous exchange. In this review we focus on the dual roles of SP-A and SP-D in immunoregulation and anti-viral defense and in particular their role in protecting against immune-mediated pathophysiological processes following viral infection. Furthermore, we discuss the potential of recombinant versions of these proteins as prophylactic treatments for infectious and inflammatory diseases, ranging from neonatal chronic lung disease to coronavirus disease 2019 (COVID-19).
Pulmonary surfactant is an important lipoprotein complex of the lung lining made of 90% lipids and 10% proteins. Surfactant is produced predominantly by alveolar type 2 cells and forms a mobile-liquid phase which covers the alveolar epithelium to facilitate breathing by reducing surface tension at end-expiration and preventing alveolar collapse (6, 7). Surfactant proteins B (SP-B) and C (SP-C) are small hydrophobic peptides of 14 kDa and 6 kDa, respectively. These are involved in the packaging and recycling of surfactant as well as contributing to its biophysical properties. Contrastingly, surfactant protein A (SP-A) and surfactant protein D (SP-D) are large, soluble, hydrophilic proteins which are expressed on most mucosal surfaces and have key overlapping and distinct roles in innate immunity and immunological homeostasis of the lung.
SP-A and SP-D form functional trimeric units, consisting of four domains, a C-terminal carbohydrate binding domain (CRD), an α-helical coiled-coil neck, a collagenous domain and an N-terminal domain (Figure 1). SP-A and SP-D are termed collectins as they contain collagen and are functional (group III) lectins, which bind carbohydrates in a calcium-dependent manner using their CRD. While the SP-D trimer is a homotrimeric unit, SP-A is formed of two gene products, SP-A1 and SP-A2, and some functional differences have been described between these two molecules (9). Through interaction of their N-terminal domains, these trimeric units oligomerize into an octadecameric-like structure for SP-A, which is similar to a bunch of flowers, and a dodecameric cruciform-like structure which can further assemble into ‘stellate multimers’ for SP-D (Figure 1) (10). This multimerization enhances the overall avidity of binding to carbohydrate targets and enhances their capacity for pathogen agglutination.
Figure 1 Structure of surfactant proteins A (SP-A) and SP-D. SP-A and SP-D contain four domains: the N-terminal domain (black), collagen-like domain (green), neck region (blue) and carbohydrate recognition domain (CRD) (red). SP-A and SP-D form functional trimers and can then further oligomerize into an octadecameric-like structure for SP-A and a dodecameric cruciform-like structure which can further assemble into ‘stellate multimers’ for SP-D. Also shown is the crystal structure of the recombinant fragment of human SP-D (rfhSP-D) (8). rfhSP-D is formed of the CRD, neck and 8x Gly Xaa Yaa repeats of the collagen-like region.
Recombinant trimeric fragments of human SP-A (rfhSP-A) and D (rfhSP-D) have been produced and consist of the CRD and trimerizing neck regions and a collagenous stalk consisting of 8 x Gly-Xaa-Yaa repeats. These lack the capacity to agglutinate pathogens but maintain many of the anti-pathogenic and immunomodulatory functions of the native proteins. Furthermore, they have potential for development into therapeutics for a variety of inflammatory and infectious lung diseases (11–15).
SP-A and SP-D bind to and neutralize a number of different viruses (14). Their importance in protecting the lung against viral infections has been demonstrated by the increased susceptibility of SP-A and SP-D knockout mice to influenza A virus and respiratory syncytial virus (RSV) infection and viral-mediated inflammation (16–21).
As compared with wildtype mice, both SP-A and SP-D knockout mice have increased susceptibility to influenza infection with an increase in viral load, infiltration of inflammatory cells, production of inflammatory cytokines and immunopathology (17, 18, 22, 23). SP-D neutralizes influenza virus through interaction with high mannose oligosaccharides in close proximity to the hemagglutinin (HA) binding site, preventing binding to the sialic acids on the host cell (24). Administration of exogenous SP-D into the lung of SP-D knockout mice decreases viral load and reduces neutrophil infiltration, as well as levels of inflammatory cytokines within the lung, including tumor necrosis factor alpha (TNF-α) and interleukin (IL)-6. Similarly, rfhSP-D has been shown to neutralize and downregulate pro-inflammatory cytokines in vitro including TNF-α, interferon (IFN)-α, IFN-β, interleukin (IL)-6, and regulated on activation normal T-cell expressed and secreted (RANTES), upon influenza infection of a basal epithelial cell line (25, 26). Comparatively, SP-A occupies the HA binding site through its own salicylic acid, found naturally on the asparagine 187 residue of the CRD. This prevents binding of influenza to salicylic acids on the host cell (27). Alongside increased viral loads, SP-A knockout mice infected with influenza develop epithelial injury and higher levels of IL-6, macrophage inflammatory protein 2 (MIP-2) and macrophage and neutrophil infiltration (20, 23). Treatment with exogenous SP-A decreases influenza infection and the production of inflammatory cytokines including TNF-α, IL-6 and IFN-γ (28).
Although both SP-A and SP-D have overlapping roles in neutralizing influenza virus, they also likely have distinct roles in vivo. For example, SP-A but not SP-D has been shown to opsonize influenza and enhance phagocytosis by rat macrophages (29). A recent study further demonstrated that native human SP-A reduced infection of an epithelial cell line by pH1N1 and H3N2 strains of influenza in vitro (26). In this paper a rfhSP-A, which was composed of the CRD and neck without a collagen stalk, interacted with neuraminidase and matrix protein 1 in a calcium-dependent manner. However, it was shown that this fragment enhanced influenza infection as well as expression of inflammatory cytokines TNF-α, IFN-α, IFN-β, IL-12, IL-6, and RANTES, contrasting to the native molecule. This opposing effect of the SP-A fragment is interesting and could be explained by its expression in an Escherichia coli strain, which lacks the capacity to add N-linked glycosylations to the expressed protein. This fragment, therefore, lacks the asparagine 187 residue which is known to be important for influenza A neutralization, which may mean that the SP-A fragment interacts with influenza through a different mechanism. Alternatively, the trimeric structure of this molecule as opposed to the octadecameric structure of the native protein could impact the ability of this molecule to neutralize or aggregate influenza and allow enhancement of epithelial cell infection. Further work elucidating this difference between native SP-A and SP-D and their recombinant fragments in ex vivo and epithelial-macrophage co-culture models will be important to understand their role in the influenza infected lung and the potential for therapeutic use (30).
RSV is the leading cause of lower respiratory tract infection in infants worldwide and is characterized by an excessive immune response with a T helper (Th)2 bias (31, 32). SP-A knockout mice have an enhanced susceptibility to RSV, with increased viral loads, infiltration of immune cells and production of inflammatory cytokines including TNF-α, IL-6, and IL-1β (18). In vitro work has demonstrated the capacity of SP-A to neutralize RSV through binding the fusion (F) protein (33). Furthermore, administration of native SP-A to SP-A knockout mice both prevented RSV infection and decreased total bronchoalveolar lavage (BAL) inflammatory cell numbers (33). However, another in vitro study found native human SP-A to bind to the attachment protein (G) of RSV and enhance the update of RSV by Hep-2C cells, potentially via its N-terminal domain (33). More recent research confirmed the role of native SP-A in neutralizing RSV, but found trimeric rfhSP-A lacking the N-terminal domain to be more efficacious (11). Further work is, therefore, needed to investigate the importance of the N-terminal domain in mediating RSV infection.
SP-D interacts with RSV through both the fusion (F) and attachment (G) proteins (19). SP-D knockout mice also have increased levels of inflammatory cytokines following RSV infection including TNF-α, IL-1β, IL-6, and MIP-2. Administration of either exogenous native human SP-D or rfhSP-D into the lung neutralizes RSV in vivo (19, 35). However, despite these early studies, there has been no recent work demonstrating the importance of SP-D treatment in preventing RSV-induced inflammation and immunopathology. Further work will be key in assessing the potential of recombinant SP-D as a therapeutic in RSV infections.
Parainfluenza is a virus related to RSV which commonly infects the elderly and immunocompromised (36). However, the role of SP-A and SP-D in modulating parainfluenza infection and parainfluenza-mediated immunopathology is yet to be described. SP-D has, however, been reported to inhibit hemagglutination activity of Sendai virus, the related murine parainfluenza virus (37). Further studies on the direct interaction of SP-A and SP-D with parainfluenza are needed.
SP-A and SP-D play roles in modulating coronavirus infection and early work demonstrated their ability to bind human coronavirus 229E (HCoV-229E) virions and prevent infection of human bronchial epithelial cells (38). Notably SP-D was more efficient than SP-A at neutralizing HCoV-229E virions to prevent human bronchial epithelial cell line infection. However, SP-A, but not SP-D was demonstrated to reduce infection of human alveolar macrophages (39).
SP-D has been shown to bind the heavily glycosylated SARS-coronavirus (CoV) spike (S) protein (39). Furthermore, pre-incubation of SP-D with SARS S-protein increases binding of S-protein to DCs, but not macrophages or a kidney epithelial cell line (40). Plasma levels of SP-D have been found to be elevated in severe acute respiratory syndrome (SARS)-related pneumonia, potentially through leakage from the damaged lung into the blood (40). Furthermore, recent studies have shown that COVID-19 patients who went on to develop macrophage activation syndrome had significantly higher serum levels of SP-D on admission and that SP-A and SP-D serum levels correlated with more severe COVID-19 disease (41, 42). Thus, early work suggests a role for SP-D in SARS-CoV-2 infection, which may modulate infection and the pathologic host inflammatory response. The level of SP-D in the lung and potential role in SARS infection immunopathology, therefore, merit consideration. Equally, the potential role of serum SP-D levels as a potential biomarker in SARS-related pneumonia warrants further investigation.
The severity of COVID-19 disease resulting from the current SARS-CoV-2 pandemic is in part related to aberrant host-inflammatory responses (43, 44). SP-A and SP-D could play roles in modulating inflammation and binding to and neutralizing SARS-CoV-2 through interaction with the spike protein which is also heavily glycosylated (43) rfhSP-D has recently been shown to compete with ACE-2 for binding of the S1 spike protein subunit of SARS-CoV-2. Furthermore, rfhSP-D reduced infection of a cell line by SARS-CoV-2 from clinical samples (45, 46). The impact of rfhSP-D on the inflammatory response is still to be demonstrated. However, rfhSP-D may have therapeutic potential in treatment of COVID-19. The role of SP-A and SP-D in COVID-19 disease now needs to be fully determined in both the serum and lung in in vivo and ex vivo models. Furthermore, the potential for recombinant versions of SP-A and SP-D to modulate SARS-CoV-2 infection and immunopathology warrants investigation.
Outside the lungs, SP-A and SP-D are expressed within the urogenital tract and most other extra-pulmonary mucosal surfaces (47–49). SP-A and SP-D play dual roles in HIV infection and pre-incubation with HIV both neutralizes the virus to prevent infection of a cluster of differentiation (CD)4+ T cell line (PM1 cells), as well as enhance infection of immature monocyte-derived dendritic cells (IMDDCs) and subsequent transfer to T cells (50, 51). SP-A binds to glycoprotein (gp)120 and blocks its interaction with both CD4 and Dendritic Cell-Specific Intercellular adhesion molecule-3-Grabbing non-integrin (DC-SIGN). Similarly, SP-D also binds gp120 and gp41 and blocks the interaction of gp120 with DC-SIGN. However, there have been conflicting reports around the capacity of SP-D to disrupt the binding of gp120 to CD4 (50, 52). The mechanism by which SP-A and SP-D enhances uptake into DCs is still not fully characterized. This could be through interaction of the HIV-bound collectin with a host cell receptor and agglutination of the virus to enhance uptake. Upon occupation of the collectin CRD, SP-A and SP-D bind numerous host cell receptors, principally through their N-terminus (Table 1). A rfhSP-D molecule lacking the N-terminal domain could therefore be advantageous in neutralizing HIV without agglutination or interacting with dendritic cell (DC) receptors. Pandit et al. demonstrated the function of rfhSP-D in neutralizing HIV to prevent T cell infection. However, the effects of HIV pre-incubation with rfhSP-D on DC uptake and subsequent transfer to T cells was not determined (52). Dodagatta-Marri et al. demonstrated that rfhSP-D inhibited HIV-1 transfer to activated peripheral blood mononuclear cells when pre-incubated with a human embryonic kidney cell line (60). They further demonstrated that rfhSP-D was able to interact with DC-SIGN as well as compete with DC-SIGN to interact with gp120. Further work directly comparing the interaction with HIV of both native SP-A and SP-D and their recombinant fragments would be useful to determine the structure-function relationships affecting their capacity to neutralize HIV and modulate viral transfer to T cells.
Alongside neutralization of HIV, SP-D has been shown to play important roles in modulating HIV-mediated inflammation. In vitro treatment of Jurkat T cells with SP-D upon HIV infection decreased expression levels of IL-2, IFN-γ, vascular endothelial growth factor (VEGF), IL-1α, and TNF-α. Similarly, treatment of peripheral blood mononuclear cells (PBMCs) with SP-D during HIV infection decreased IL-2, IFN-γ, VEGF, IL-6, monocyte chemoattractant protein-1 (MCP-1) and IL-1β (52). However, despite the promising potential of SP-A and SP-D for modulating HIV infection and HIV mediated inflammation, the full impact of SP-A and SP-D on HIV infection and immunopathology in vivo and in the human disease is yet to be determined.
SP-A and SP-D are broadly selective innate immune proteins and thus are likely to play key roles in modulating infection and inflammation mediated by other viruses. SP-A, but not SP-D, binds to human papillomavirus 16 (HPV16) pseudovirions and enhances their uptake by RAW267.4 macrophages and clearance in vivo (91). SP-A also binds herpes simplex virus (HSV) infected cells, as well as HSV virions through its Asn187 carbohydrate moiety to enhance phagocytosis by rat macrophages (92, 93). Further work is needed to characterize the mechanism of SP-A interactions with HPV and HSV and its role in modulating inflammatory responses to these viruses (94).
SP-D but not SP-A has been demonstrated to bind to the Ebola virus in a calcium-dependent manner through its CRD (95). However, pre-incubation of human SP-D (but not rfhSP-D) with the Ebola virus enhanced infection of Vero cells; this could be mediated through membrane receptor interactions with the collectin N-terminal domain. The potential of trimeric rfhSP-D in therapeutic modulation of the aberrant pro-inflammatory cytokine release by monocytes and macrophages in Ebola virus infection is as yet untested (96), as it is for other currently emerging viruses.
SP-A and SP-D are key defense molecules which neutralize a range of viruses. However, a plethora of in vitro and in vivo studies have demonstrated SP-A and SP-D as key players in directly modulating the innate and adaptive immune system independent of infection (Figure 2). Through these mechanisms, SP-A and SP-D could be important in balancing the inflammatory response to prevent immune-mediated pulmonary pathology, an important feature of influenza and SARS-related viral pneumonia, as well as RSV induced bronchiolitis (97, 98).
Figure 2 Maintenance of homeostasis in the lung by surfactant protein D (SP-D). Shown is an overview of the roles of SP-D in the lung. Indicated is the role SP-D plays in neutralizing, agglutinating and clearing viruses as well as reducing the inflammatory response upon infection with influenza A virus, respiratory syncytial virus (RSV), and human immunodeficiency virus (HIV). The role of SP-D in enhancing phagocytosis by dendritic cells (DCs) while simultaneously reducing antigen presentation and activation of co-stimulatory markers is indicated. Also shown is the role of SP-D in keeping T cells in a hyporesponsive state to increase CTLA4 expression, reduce T cell proliferation, reduce allergen induced Th2 cytokine production and modulate apoptosis. The role of SP-D in clearing and agglutinating noxious particles, pollen and pathogens is indicated. Similarly, the role of SP-D in enhancing macrophage-mediated pathogen killing, modulating inflammatory cytokine production by macrophages and macrophage chemotaxis and reducing antigen presentation is displayed. Also shown is the role of SP-D in clearing apoptotic and necrotic cells in the lung as well as its interaction with neutrophils in binding to neutrophil NETS, and eosinophil extracellular traps, preventing degranulation and modulating cytokine production. Finally, the role of SP-D and rfhSP-D in correcting the phenotype of the SP-D knockout mouse is indicated, specifically their role in decreasing emphysema, excessive phospholipid production, decreasing inflammatory cell and apoptotic and necrotic cell numbers, decreasing the level of reactive oxygen species (ROS), decreasing inflammatory cytokines including IL-6 and IL-12 and decreasing the susceptibility of SP-D knockout mice to pathology as a result of challenge with pathogens, allergens and noxious particles. Adapted from “Alveolar Epithelium (Comparison)”, by BioRender.com (2020). Retrieved from https://app.biorender.com/biorender-templates.
The importance of SP-A and SP-D in maintaining lung homeostasis is highlighted by the inflammatory phenotype of the knockout mice. SP-D deficient mice have increased infiltration of macrophages and activated T cells, the appearance of foamy macrophages with an excessive level of apoptotic and necrotic macrophages and alveolar type II cell hyperplasia in the airways. They also have excessive levels of phospholipids, overproduction of reactive oxygen species (ROS) and increased levels of lung IL-6, IL-12 and metalloproteinases (MMPs). By the age of three weeks, these mice already show signs of a progressive emphysema-like phenotype with loss of alveolar septation and the appearance of foamy macrophages (99–101). This phenotype can be resolved upon therapeutic treatment with rfhSP-D, which corrects the emphysema and decreases the number of apoptotic and necrotic alveolar macrophages, excess phospholipid production and alveolar type II cell hyperplasia (100, 102).
Allergy mouse models have also demonstrated the role of SP-D in immunoregulation with increased IL-13 levels and BAL eosinophils upon ovalbumin sensitization in SP-D knockout mice (103). Similarly, as compared with wildtype mice, SP-D deficient mice exposed to Aspergillus fumigatus allergen have enhanced CD4 T cells numbers, IgG1 and IgE immunoglobulins and Th2 cytokines, with a decrease in IFN-γ (104–106). This highlights the potential role of SP-D in preventing Th2 inflammatory skewing, which has been shown to be important in immune evasion by viruses such as RSV (107). SP-D knockout mice also have an enhanced susceptibility to cigarette smoke-induced airway inflammation with influx of alveolar macrophages, secretion of chemokine (C-C motif) ligand 3 (CCL3) and IL-6 and upregulation of ceramide genes; rfhSP-D alleviates this in vivo phenotype and attenuates cigarette smoke induced human epithelial cell apoptosis (108).
By contrast, SP-A knockout mice kept in sterile vivarium conditions have relatively unaltered lungs and normal lung function. However, they also exhibit increased susceptibility to a range of pathogens and enhanced inflammatory responses to pathogen challenge, showing, for example higher levels of TNF-α and nitric oxide metabolites upon intranasal delivery of lipopolysaccharide (LPS); this is corrected upon therapeutic treatment with exogenous SP-A (18, 22, 109–114). SP-A deficient mice also show excessive inflammation following allergen challenge with marked hyper-eosinophilia and increased IL-5 and IL-13 upon challenge with Aspergillus fumigatus allergens (106). This excessive inflammatory response in SP-A and SP-D knockout mice to pathogen-associated molecular patterns (PAMPs) on allergens and in infection, highlights their critical role in maintaining the lung in a non-inflamed condition, preserving homeostasis and facilitating gas exchange. This may be crucial to both prevent excessive inflammation and reduce viral-mediated lung pathology in chronic lung diseases.
SP-A and SP-D help preserve lung homeostasis by acting as innate immune scavenger receptors (115, 116). Their importance in binding to and clearing an array of different gram negative and positive bacteria and fungi, as well as their components, has been widely reported (116–118). This can occur through interacting with LPS through binding to terminal monosaccharides and lipid A. Binding pathogens by SP-A and D leads to their agglutination, while also directly enhancing uptake by macrophages and neutrophils through interactions mediated by various receptors (Table 1) (5, 116, 117, 119, 120). Comparative to the native oligomeric proteins, fragments of SP-A and SP-D are trimeric and lack the capacity to agglutinate bacteria. SP-A and SP-D can also modulate receptor expression on macrophages including mannose receptor, an important receptor for mediating phagocytosis (121, 122). Alongside enhancing clearance of pathogens, SP-A and SP-D also enhance macrophage-mediated killing of bacteria through increasing the production of nitric oxide as well as directly increasing membrane permeability of gram negative bacteria to inhibit their growth (123–126). However, Bordetella pertussis lipopolysaccharide resists the bactericidal effects of pulmonary surfactant protein A and the ability of SP-A to bind and aggregate the bacteria; this protective effect was lost in LPS mutants which lacked the terminal trisaccharides, suggesting that B. pertussis has evolved a mechanism which shields against the anti-bacterial function of SP-A (124). SP-A has been shown to enhance TNF-α and nitric oxide mediated killing of Bacillus Calmette-Guerin by rat macrophages (127). These roles could be particularly important for the prevention of secondary bacterial infection and resolution of inflammation following viral infection (128).
Table 1 The interaction of surfactant proteins A (SP-A) and SP-D with host cell surface proteins, soluble proteins, and receptors.
Promoting the clearance of apoptotic cells before the later stages of apoptosis and necrosis is important in preventing cell membrane breakdown and leakage of toxic intracellular enzymes, which can lead to inflammation and damage to the delicate lung tissue (129, 130). Administration of rfhSP-D is effective in clearing apoptotic and necrotic cells from the lungs of SP-D knockout mice (100). Both SP-A and SP-D bind and enhance the clearance of apoptotic cells, including polymorphonuclear leukocytes (PMNs) and T cells, through distinct mechanisms (131–135). SP-A and SP-D suppress alveolar macrophage phagocytosis through binding of the CRD to signal-regulatory protein (SIRP)α in the resting lung. However, upon initiation of inflammation, SP-A and SP-D activate phagocytosis through binding to the CD91 receptor. Thus, SP-A and SP-D play flexible roles in modulating the inflammatory response depending on the lung environment (61, 66, 136).
During cell apoptosis, nuclear fragments migrate toward the plasma membrane which form “bleb” like protrusions to display deoxyribonucleic acid (DNA) and ribonucleoproteins at the cell surface (137). One mechanism by which rfhSP-D has been shown to bind to and enhance the clearance of apoptotic cells in vivo is through binding genomic DNA (138). SP-D interacts with neutrophil extracellular traps (NET) while simultaneously binding to carbohydrate ligands in vivo. Through this mechanism, SP-D agglutinates Pseudomonas aeruginosa and alters the mode of NET-mediated bacterial trapping (139). SP-D has also been shown to inhibit eosinophil extracellular DNA trap formation. This effect was lost upon nitrosylation of SP-D, highlighting the potential differing role in modulating eosinophil DNA trap formation depending on the inflammatory status of the lung (140). Alongside binding to apoptotic cells, cell debris and extracellular traps, SP-D binds free bacterial and host DNA. Palaniyar et al. demonstrated the decreased clearance and accumulation of both free DNA and auto-antibodies in SP-A and SP-D knockout mice (141, 142).
Clearance of allergens is also an important function of SP-A and SP-D as allergens may have a synergistic role with viruses in inducing exacerbations of inflammatory lung diseases including asthma (143). SP-A and SP-D are widely reported to bind to and enhance the uptake and clearance of allergens from A. fumigatus (144), house dust mite (145) and various types of pollen (146, 147). Furthermore, they modulate the allergen-induced inflammatory response by reducing basophil, eosinophil and mast cell degranulation to prevent the release of pro-inflammatory mediators including histamine and beta-hexosaminidase (106, 158–160, 162). rfhSP-D modulates allergic inflammatory responses and reduces mast cell and basophil degranulation in allergic inflammation in vivo. rfhSP-D also both prevents eosinophil recruitment in allergen-challenged mice and enhances the apoptosis and clearance of primed eosinophils by macrophages and PBMCs (148–151).
Inhalation of noxious particles is an important risk factor for the development of inflammatory lung diseases such as chronic obstructive pulmonary disease (COPD), and patients with COPD have an increased risk of viral lower respiratory tract infections (LRTI) (152–154). SP-A and SP-D agglutinate and clear a range of different hydrophobic and hydrophilic nanoparticles and rfhSP-D enhances the co-localization of nanoparticles to epithelial cells in vitro (155, 156). Nanoparticles may inhibit the capacity of SP-A and SP-D to neutralize influenza virus (2, 155, 157). Diesel exhaust pollutant exposed mice have an increased susceptibility to influenza and RSV infection, associated with a decrease in surfactant protein expression (157, 158). Thus, both decreased expression and modulation of SP-A’s and SP-D’s anti-viral activity could play roles in the increased susceptibility of smokers and COPD patients to viral LRTI. Moreover, SP-A and SP-D could play additional indirect roles in preventing viral infection and inflammation through the clearance of noxious particles.
SP-A and SP-D interact with various receptors on innate immune cells to modulate inflammation (Table 1) (119). An elegant model by Gardai et al. demonstrated the dual manner by which SP-A and SP-D mediate or suppress inflammation, dependent on the orientation of the collectin and, therefore, receptor with which it interacts; a similar mechanism to their role in modulating apoptosis (61). Gardai et al. described the interaction of SP-A and SP-D through the CRD with SIRPα on myeloid lineage cells in the resting lung. This was shown to prevent pro-inflammatory cytokine production to maintain homeostasis. However, upon occupation of the CRD through pathogen binding, SP-A and SP-D instead interact with the calrecticulin/CD91 receptor complex through their N-terminal tails. This mediates the production of pro-inflammatory cytokine production for anti-pathogen immune responses. An exemplar of this dual role in viral infection has been demonstrated by the ability of SP-D to decrease neutrophil burst in vitro, but increase neutrophil burst in the presence of influenza (159).
SP-A and SP-D bind various other receptors on alveolar macrophages and have been shown to reduce TNF-α production through competing with LPS for CD14 binding (Table 1) (160). Furthermore, SP-A and SP-D modulate inflammatory cytokine production after stimulation of macrophages by cytokines or PAMPs. For example, SP-A inhibits peptidoglycan-induced TNF-α secretion upon binding to toll-like receptor (TLR)-2. SP-A also inhibits TNF-α production in IFN-γ stimulated macrophages to reduce nitric oxide production (161, 162). Minutti et al. demonstrated the role of SP-A in directly binding to IFN-γ and inhibiting IFN-γ and LPS–induced TNF-α, inducible nitric oxide synthase (iNOS), and C-X-C motif chemokine ligand 10 (CXCL10) production (163). SP-A and SP-D also mediate alveolar macrophage and neutrophil chemotaxis and stimulate alveolar macrophage directional actin polymerization (164–167).
Two new receptors have recently been discovered for SP-D on monocytes which demonstrate the dual role SP-D plays in modulating their functions through the collagen domain. SP-D binds to Leukocyte-associated Ig-like receptor-1 (LAIR1), a receptor expressed on neutrophils and monocytes, and prevents the production of FcR-mediated ROS, in a human myeloid leukemia cell (70). However, SP-D also binds to osteoclast-associated receptor (OSCAR) on human C-C chemokine receptor 2 positive (CCR2+) inflammatory monocytes to activate TNF-α release through its collagen domain (68). Further work to investigate the impact of these interactions in anti-viral and inflammatory responses is needed.
Although there has been limited research investigating the interaction of SP-A and SP-D with innate lymphoid cells (ILC), one study demonstrated the importance of SP-D in mediating effective ILC2-mediated immune responses to the parasite Nippostrongylus brasiliensis (168). SP-D knockout mice had an impaired ability to resolve N. brasiliensis infection. However, intra-nasal treatment with rfhSP-D was shown to increase numbers of IL-13 producing ILC2s and numbers of alternatively activated macrophages in the lung. Moreover, rfhSP-D administration enhanced parasitic killing during the larval L4 lung stage of its natural life cycle (168).
Natural killer (NK) cells are an essential component of the anti-viral immune response. However, little is known about their interaction with SP-A and SP-D. A study by Ge et al. demonstrated a decreased IFN-γ expression in SP-D knockout mice upon ozone exposure (69). The authors hypothesized that this decrease was as a result of the absence of SP-D interacting with the glycosylated NKp46 receptor on NK cells (69). They further postulated that this could play a role in the impaired dendritic cell homing to lymphoid tissue seen in SP-D knockout mice. SP-A has also been suggested to interact with NK cells through the SPR-210 receptor, now identified as Myosin 18A (or CD245) (53). A study looking at the impact of SP-A on NK cell function found an increase in IL-2 activated NK cell-mediated lymphokine-activated killer (LAK) activity toward Epstein-Barr Virus-infected B cells (169). These interactions could have important potential consequences for modulating NK cell function in anti-viral and inflammatory responses. Further work characterizing the role of SP-A and SP-D in NK-cell mediated anti-viral responses may be important in understanding the pathogenicity of emerging viral threats.
SP-A and SP-D bridge the innate and adaptive immune system through their functions in modulating DC function. These roles could be key in directing the inflammatory response after respiratory viral infection. SP-D knockout mice have increased activation of DCs as demonstrated by CD11b and CD86 co-stimulatory molecule upregulation and increased TNF-α expression; this is corrected upon treatment with recombinant murine SP-D (170). Furthermore, SP-A and SP-D modulate lung DC function through inhibiting antigen presentation and SP-A has been shown to inhibit E. coli antigen presentation, while simultaneously increasing its phagocytosis (171, 172).
The resultant impact of collectin-modulated DC function on T cells has been demonstrated by a decrease in LPS-mediated major histocompatibility complex II (MHCII) and CD86 expression by DCs and a reduction in allo-stimulation of CD4 T cells upon treatment with SP-A. DCs from SP-D knockout mice also express thymus and activation-regulated chemokine (TARC), which is chemotactic for activated T cells (104).
SP-A and SP-D directly modulate T cells and inhibit antigenic and mitogenic induced T cell proliferation through both IL-2-dependent and IL-2-independent mechanisms (173–178). They also alter T cell function and activation. For example, SP-D knockout mice have increased numbers of activated CD4 and CD8 T cells which express CD69 and CD25, while SP-A has been shown to reduce IFN-γ production and T cell mediated inflammation (101, 104, 178). Treatment with rfhSP-D both decreases T cell activation and lymphoproliferation through the upregulation of cytotoxic T-lymphocyte-associated protein 4 (CTLA4), as well as decreasing allergen induced IgE production by B cells (148, 179).
SP-D impacts the adaptive immune system by modulating T cell apoptosis. This is seen through the prevention of caspase-8 and caspase-3 activation by SP-D to inhibit Fas (CD95)-Fas ligand and tumor necrosis factor-related apoptosis-inducing ligand (TRAIL)-TRAIL receptor induced apoptosis (180, 181). T cells are essential in anti-viral immunity and collectins could play roles in coordinating this response (182). Further investigation of the roles of SP-A and SP-D in modulating T cell-mediated responses and viral-mediated immunopathology is now indicated, through the use of appropriate ex vivo viral and inflammatory models (183).
Consistent with their array of roles in viral immunity and lung homeostasis, SP-A and SP-D deficiency may contribute to pathological mechanisms in a range of respiratory diseases. Winkler et al. demonstrated a decreased level of SP-D in the airways of smokers, with a further reduction in patients with COPD (2). This was inversely related to serum SP-D levels due to leakage of alveolar SP-D across the inflamed or damaged alveolar capillary membrane. Patients experiencing COPD exacerbations have higher serum SP-D levels which are reduced by treatment with anti-inflammatory glucocorticoids, highlighting its potential role as a biomarker for COPD acute inflammation (184, 185). SP-A and SP-D have also been shown to be reduced in allergen-challenged asthma patients and lower airway levels have been shown to correlate with asthma severity (1, 186).
Along with lipid surfactant, SP-A and SP-D levels are deficient in premature neonates. However, these key proteins are not replaced with current surfactant therapy, as they are not present in current formulations. Low collectin levels are correlated with risk of infection and development of neonatal chronic lung disease, both in animal models of preterm lung disease and in clinical studies. In particular, low SP-D levels in human preterm infants soon after birth are linked with an increased risk of neonatal chronic lung disease development (3, 187–189). SP-D levels increase in response to infection in the preterm infant, but this acute phase response may be inadequate to counter ongoing inflammation, due to degradation in the inflamed preterm lung. Exogenous therapeutic recombinant SP-D administration has been shown to reduce ventilation-induced inflammation in preterm lambs, highlighting its potential to reduce inflammation caused by barotrauma in ventilated preterm infants developing neonatal chronic lung disease (190, 191).
Deficiency of collectins in inflammatory lung diseases could be related to multiple factors (Figure 3). Firstly, SP-A and D have key roles as scavenger molecules in maintaining lung homeostasis. Thus, in the chronic inflammatory environment of the diseased lung, a constant turnover and degradation of SP-A and SP-D through binding to and enhancing clearance of pathogens, noxious particles, apoptotic cells and cell debris could lead to decreased levels. Furthermore, inflammatory mediators which damage the delicate epithelium could compromise the air-blood barrier with resultant leakage of SP-A and SP-D from the lung into the blood. Alveolar type 2 cell injury could similarly lead to a reduction in SP-A and SP-D lung levels due to decreased synthesis (192).
Figure 3 Mechanisms for reduction in surfactant proteins A (SP-A) and SP-D in the lung during infection and inflammation. Indicated is the degradation of SP-A and SP-D through their role as scavenger receptors to bind and enhance clearance of pathogens, noxious particles, apoptotic cells and cell debris (1); degradation of SP-A and SP-D through pathogen-derived proteases and elevated endogenous proteases secreted by recruited inflammatory cells or released from dying and damaged cells (2); damage to the alveolar epithelium leading to reduction of SP-A and SP-D production (3) and leakage into the blood (4). Adapted from “Cytokine Storm”, by BioRender.com (2020). Retrieved from https://app.biorender.com/biorender-templates.
Degradation of SP-A and SP-D through pathogen-derived proteases and elevated endogenous proteases, secreted by recruited inflammatory cells or released from dying and damaged cells, may also play a role in reducing SP-A and SP-D levels within the inflamed lung. SP-A and SP-D are degraded through various host and pathogen-derived enzymes including leucocyte elastase, proteinase 3, cathepsin G and Pseudomonas elastase (193–196). Children with cystic fibrosis have protease - antiprotease imbalance as well as coexisting low levels of SP-A and SP-D (197–199). Decreased SP-A and SP-D levels have also been found in BAL from children with RSV infection (21). Low SP-A and SP-D levels in such inflammatory lung diseases may both generate susceptibility to respiratory viral infection and lead to an exaggerated damaging host inflammatory response.
The potential for treatment of inflammatory diseases and respiratory viral infections by augmentation of the innate immune system is increasingly understood but as yet remains unexploited (200–202). SP-A and SP-D are anti-viral innate immune molecules and play key roles in orchestrating the innate and adaptive immune system to limit inflammation, making these mechanisms dually attractive as potential therapeutics.
Correction of SP-A and SP-D deficiency in inflammatory respiratory diseases could be achieved by supplementation with recombinant versions of SP-A and SP-D. However, development of full-length recombinant SP-A and SP-D molecules as therapeutics has been problematic due to low expression yields in eukaryotic systems. Furthermore, difficulties with handling of the proteins, with a tendency to oligomerize and/or agglomerate, generates difficulties with precise stable molecular characterization and solubility (203–208).
Smaller rfhSP-A and rfhSP-D trimeric fragment proteins have been developed and have the advantage of being more easily and cheaply produced in E. coli (209–211). These proteins maintain many of the anti-viral and immunomodulatory functions of the native proteins (11–13, 15, 45, 46, 212). Furthermore, they contain the functional CRD binding domain which mediates the anti-inflammatory collectin action through interacting with SIRPα on innate immune cells (61). However, they lack the majority of the collagen domain and the N-terminus of the native full-length protein. The N-terminal region has been shown to induce inflammation through binding calrecticulin/CD91 and may be exploited to facilitate a route of entry for viruses such as HIV, RSV, and the Ebola virus (14, 96, 61).
rfhSP-D functions to neutralize RSV, HIV and SARS-CoV-2 and modulates influenza-mediated inflammatory cytokine production (25, 45, 46, 53, 52). rfhSP-D also corrects the emphysematous phenotype seen in murine SP-D deficiency and reduces levels of apoptotic and necrotic macrophages and MMPs. rfhSP-D binds to and enhances the clearance of apoptotic cells, free DNA and neutrophil and eosinophil extracellular traps (139, 140) and modulates the adaptive immune system to suppress proliferation and activation of T cells through upregulation of CTLA4 (101, 179, 213). rfhSP-D, therefore, has properties which suggest it may be useful both as a prophylactic and treatment for infectious and inflammatory lung diseases. rfhSP-A has been shown to neutralize RSV, but requires further characterization (11).
Therapeutic rfhSP-D is currently under development for treatment of premature neonates with neonatal RDS as an adjunct to current surfactant therapies to help prevent the development of chronic inflammation leading to neonatal chronic lung disease. This may help prevent the inflammatory emphysematous phenotype seen in neonatal chronic lung disease and reduce susceptibility to severe respiratory viral infection. Alongside other inflammatory diseases such as asthma and COPD, rfhSP-D could have therapeutic potential in emerging respiratory infections such as SARS-CoV-2, by both neutralizing the virus and modulating the inflammation-mediated pathology associated with COVID-19.
AW: conceptualization, investigation, literature searching, analysis, project administration, writing original draft, reviewing and editing. JM: supervision, conceptualization, reviewing, and editing. HC: conceptualization, funding, supervision, writing, reviewing, and editing. All authors contributed to the article and approved the submitted version.
This work was supported by the UK Medical Research Council (MR/P026907/1) and by the UCLH/UCL NIHR Biomedical Research Centre.
AW, JM, and HC are named inventors on a patent jointly filed by University of Southampton and Spiber Technologies (WO2017109477A2·2017-06-29).
We would like to thank Dr Johanna Midelet for her help in generating the figures and Pam Watson for proof-reading the manuscript.
1. Mackay RM, Grainge CL, Lau LC, Barber C, Clark HW, Howarth PH. Airway Surfactant Protein D Deficiency in Adults With Severe Asthma. Chest (2016) 149(5):1165–72. doi: 10.1016/j.chest.2015.11.012
2. Winkler C, Atochina-Vasserman EN, Holz O, Beers MF, Erpenbeck VJ, Krug N, et al. Comprehensive characterisation of pulmonary and serum surfactant protein D in COPD. Respir Res (2011) 12:29. doi: 10.1186/1465-9921-12-29
3. Beresford MW, Shaw NJ. Bronchoalveolar Lavage Surfactant Protein A, B, and D Concentrations in Preterm Infants Ventilated for Respiratory Distress Syndrome Receiving Natural and Synthetic Surfactants. Pediatr Res (2003) 53(4):663–70. doi: 10.1203/01.PDR.0000054653.89527.F8
4. Wright JR. Immunoregulatory functions of surfactant proteins. Nat Rev Immunol (2005) 5(1):58–68. doi: 10.1038/nri1528
5. Littlejohn JR, da Silva RF, Neale WA, Smallcombe CC, Clark HW, Mackay R-MA, et al. Structural definition of hSP-D recognition of Salmonella enterica LPS inner core oligosaccharides reveals alternative binding modes for the same LPS. PLoS One (2018) 13(6):e0199175. doi: 10.1371/journal.pone.0199175
6. Pattle RE. Properties, function and origin of the alveolar lining layer. Nature (1955) 175(4469):1125–6. doi: 10.1038/1751125b0
7. Halliday HL. Surfactants: past, present and future. J Perinatol (2008) 28:S47–56. doi: 10.1038/jp.2008.50
8. Head JF, Mealy TR, McCormack FX, Seaton BA. Crystal structure of trimericcarbohydrate recognition and neck domains of surfactant protein A. J Biol Chem (2003) 278(44):43254–60.
9. Sanchez-Barbero F, Rivas G, Steinhilber W, Casals C. Structural and functional differences among human surfactant proteins SP-A1, SP-A2 and co-expressed SP-A1/SP-A2: role of supratrimeric oligomerization. Biochem J (2007) 406:479–89. doi: 10.1042/BJ20070275
10. Strang CJ, Slayter HS, Lachmann PJ, Davis AE. Ultrastructure and composition of bovine conglutinin. Biochem J (1986) 234(2):381–9. doi: 10.1042/bj2340381
11. Watson A, Kronqvist N, Spalluto CM, Griffiths M, Staples KJ, Wilkinson T, et al. Novel expression of a functional trimeric fragment of human SP-A with efficacy in neutralisation of RSV. Immunobiology (2017) 222(2):111–8. doi: 10.1016/j.imbio.2016.10.015
12. Watson A, Spalluto CM, Kronqvist N, Staples KJ, Rising A, Johansson J, et al. First Successful Expression Of A Trimeric Human Fragment Of SP-A And Demonstration Of Its Increased Efficacy In Neutralisation Of RSV Compared With Native Human SP-A. B106 cellular/molecular mechanisms and translational aspects of respiratory tract infections. Am Thoracic Soc (2016) 193:A7856–A. doi: 10.1164/ajrccm-conference.2016.193.1_MeetingAbstracts.A7856
13. Watson A. Recombinant expression of functional trimeric fragments of human SP-A and SP-D. University of Southampton (2016).
14. Watson A, Phipps MJS, Clark HW, Skylaris CK, Madsen J. Surfactant Proteins A and D: Trimerized Innate Immunity Proteins with an Affinity for Viral Fusion Proteins. J Innate Immun (2019) 11(1):13–28. doi: 10.1159/000492974
15. Clark H, Reid KBM. Structural Requirements for SP-D Function in vitro and in vivo: Therapeutic Potential of Recombinant SP-D. Immunobiology (2002) 205(4):619–31. doi: 10.1078/0171-2985-00159
16. LeVine AM, Bruno MD, Huelsman KM, Ross GF, Whitsett JA, Korfhagen TR. Surfactant protein A-deficient mice are susceptible to group B streptococcal infection. J Immunol (1997) 158(9):4336–40. doi: 10.1078/0171-2985-00159
17. LeVine AM, Whitsett JA, Hartshorn KL, Crouch EC, Korfhagen TR. Surfactant protein D enhances clearance of influenza A virus from the lung in vivo. J Immunol (Baltimore Md 1950) (2001) 167(10):5868–73. doi: 10.4049/jimmunol.167.10.5868
18. LeVine AM, Gwozdz J, Stark J, Bruno M, Whitsett J, Korfhagen T. Surfactant protein-A enhances respiratory syncytial virus clearance in vivo. J Clin Invest (1999) 103(7):1015–21. doi: 10.1172/JCI5849
19. LeVine AM, Elliott J, Whitsett JA, Srikiatkhachorn A, Crouch E, DeSilva N, et al. Surfactant protein-D enhances phagocytosis and pulmonary clearance of respiratory syncytial virus. Am J Respiratory Cell Mol Biol (2004) 31(2):193–9. doi: 10.1165/rcmb.2003-0107OC
20. Li G, Siddiqui J, Hendry M, Akiyama J, Edmondson J, Brown C, et al. Surfactant Protein-A–Deficient Mice Display an Exaggerated Early Inflammatory Response to a β -Resistant Strain of Influenza A Virus. Am J Respiratory Cell Mol Biol (2002) 26(3):277–82. doi: 10.1165/ajrcmb.26.3.4584
21. Kerr MH, Paton JY. Surfactant protein levels in severe respiratory syncytial virus infection. Am J Respir Crit Care Med (1999) 159(4):1115–8. doi: 10.1164/ajrccm.159.4.9709065
22. LeVine AM, Hartshorn K, Elliott J, Whitsett J, Korfhagen T. Absence of SP-A modulates innate and adaptive defense responses to pulmonary influenza infection. American journal of physiology Lung cellular and molecular physiology. Am J Physiol Lung Cell Mol Physiol (2002) 282(3):L563–72. doi: 10.1152/ajplung.00280.2001
23. Hawgood S, Brown C, Edmondson J, Stumbaugh A, Allen L, Goerke J, et al. Pulmonary Collectins Modulate Strain-Specific Influenza A Virus Infection and Host Responses. J Virol (2004) 78(16):8565–72. doi: 10.1128/JVI.78.16.8565-8572.2004
24. Hartshorn KL, Webby R, White MR, Tecle T, Pan C, Boucher S, et al. Role of viral hemagglutinin glycosylation in anti-influenza activities of recombinant surfactant protein D. Respiratory Res (2008) 9(65). doi: 10.1186/1465-9921-9-65
25. Al-Ahdal MN, Murugaiah V, Varghese PM, Abozaid SM, Saba I, Al-Qahtani AA, et al. Entry Inhibition and Modulation of Pro-Inflammatory Immune Response Against Influenza A Virus by a Recombinant Truncated Surfactant Protein D. Front Immunol (2018) 9(1586). doi: 10.3389/fimmu.2018.01586
26. Al-Qahtani AA, Murugaiah V, Bashir HA, Pathan AA, Abozaid SM, Makarov E, et al. Full-length human surfactant protein A inhibits influenza A virus infection of A549 lung epithelial cells: A recombinant form containing neck and lectin domains promotes infectivity. Immunobiology (2019) 224(3):408–18. doi: 10.1016/j.imbio.2019.02.006
27. Benne C, Kraaijeveld C, Van Strijp J, Brouwer E, Harmsen M, Verhoef J, et al. Interactions of surfactant protein A with influenza A viruses: binding and neutralization. J Infect Dis (1995) 171(2):335–41. doi: 10.1093/infdis/171.2.335
28. LeVine AM, Hartshorn K, Elliott J, Whitsett J, Korfhagen T. Absence of SP-A modulates innate and adaptive defense responses to pulmonary influenza infection. Am J Physiol-Lung Cell Mol Physiol (2002) 282(3):L563–L72. doi: 10.1152/ajplung.00280.2001
29. Benne CA, Benaissa-Trouw B, van Strijp JA, Kraaijeveld CA, van Iwaarden JF. Surfactant protein A, but not surfactant protein D, is an opsonin for influenza A virus phagocytosis by rat alveolar macrophages. Eur J Immunol (1997) 27(4):886–90. doi: 10.1002/eji.1830270413
30. Nicholas B, Staples KJ, Moese S, Meldrum E, Ward J, Dennison P, et al. A Novel Lung Explant Model for the Ex Vivo Study of Efficacy and Mechanisms of Anti-Influenza Drugs. J Immunol (2015) 194(12):6144–54. doi: 10.4049/jimmunol.1402283
31. Rosenberg HF, Domachowske JB. Inflammatory responses to respiratory syncytial virus (RSV) infection and the development of immunomodulatory pharmacotherapeutics. Curr Med Chem (2012) 19(10):1424–31. doi: 10.2174/092986712799828346
32. Bagga B, Cehelsky JE, Vaishnaw A, Wilkinson T, Meyers R, Harrison LM, et al. Effect of Preexisting Serum and Mucosal Antibody on Experimental Respiratory Syncytial Virus (RSV) Challenge and Infection of Adults. J Infect Dis (2015) 212(11):1719–25. doi: 10.1093/infdis/jiv281
33. Ghildyal R, Hartley C, Varrasso A, Meanger J, Voelker DR, Anders EM, et al. Surfactant protein a binds to the fusion glycoprotein of respiratory syncytial virus and neutralizes virion infectivity. J Infect Dis (1999) 180(6):2009–13. doi: 10.1086/315134
34. Hickling TP, Malhotra R, Bright H, McDowell W, Blair ED, Sim RB. Lung surfactant protein A provides a route of entry for respiratory syncytial virus into host cells. Viral Immunol (2000) 13(1):125–35. doi: 10.1089/vim.2000.13.125
35. Hickling TP, Bright H, Wing K, Gower D, Martin SL, Sim RB, et al. A recombinant trimeric surfactant protein D carbohydrate recognition domain inhibits respiratory syncytial virus infection in vitro and in vivo. Eur J Immunol (1999) 29(11):3478–84. doi: 10.1002/(SICI)1521-4141(199911)29:11<3478::AID-IMMU3478>3.0.CO;2-W
37. Hartshorn KL. Role of surfactant protein A and D (SP-A and SP-D) in human antiviral host defense. Front Biosci (Scholar Ed) (2010) 2:527–46. doi: 10.2741/s83
38. Funk CJ, Wang J, Ito Y, Travanty EA, Voelker DR, Holmes KV, et al. Infection of human alveolar macrophages by human coronavirus strain 229E. J Gen Virol (2012) 93(Pt 3):494–503. doi: 10.1099/vir.0.038414-0
39. Leth-Larsen R, Zhong F, Chow VT, Holmskov U, Lu J. The SARS coronavirus spike glycoprotein is selectively recognized by lung surfactant protein D and activates macrophages. Immunobiology (2007) 212(3):201–11. doi: 10.1016/j.imbio.2006.12.001
40. Wu YP, Liu ZH, Wei R, Pan SD, Mao NY, Chen B, et al. Elevated plasma surfactant protein D (SP-D) levels and a direct correlation with anti-severe acute respiratory syndrome coronavirus-specific IgG antibody in SARS patients. Scandinavian J Immunol (2009) 69(6):508–15. doi: 10.1111/j.1365-3083.2009.02245.x
41. Kerget B, Kerget F, Koçak AO, Kızıltunç A, Araz Ö, Uçar EY, et al. Are Serum Interleukin 6 and Surfactant Protein D Levels Associated with the Clinical Course of COVID-19? Lung (2020) 198(5):777–84. doi: 10.1007/s00408-020-00393-8
42. Saito A, Kuronuma K, Moniwa K, Kodama K, Takahashi S, Takahashi H, et al. Serum surfactant protein A and D may be novel biomarkers of COVID-19 pneumonia severity. Res Square (2020). doi: 10.21203/rs.3.rs-29567/v1
43. Walls AC, Park Y-J, Tortorici MA, Wall A, McGuire AT, Veesler D. Structure, Function, and Antigenicity of the SARS-CoV-2 Spike Glycoprotein. Cell (2020) 181(2):281–92.e6. doi: 10.1016/j.cell.2020.02.058
44. Tay MZ, Poh CM, Rénia L, MacAry PA, Ng LFP. The trinity of COVID-19: immunity, inflammation and intervention. Nat Rev Immunol (2020) 20:363–374. doi: 10.1038/s41577-020-0311-8
45. Hsieh M-H, Beirag N, Murugaiah V, Chou Y-C, Kuo W-S, Kao H-F, et al. Human Surfactant Protein D Binds S1 and Receptor Binding Domain of Spike protein and acts as an entry inhibitor of SARS-CoV-2 Pseudotyped viral particles in vitro. bioRxiv. (2020):2020.12.18.423418.
46. Madan T, Biswas B, Varghese P, Subedi R, Pandit H, Idicula-Thomas S, et al. A recombinant fragment of Human surfactant protein D binds Spike protein and inhibits infectivity and replication of SARS-CoV-2 in clinical samples. bioRxiv. (2020):2020.12.18.423415
47. Madsen J, Tornøe I, Nielsen O, Koch C, Steinhilber W, Holmskov U. Expression and Localization of Lung Surfactant Protein A in Human Tissues. Am J Respiratory Cell Mol Biol (2003) 29(5):591–7. doi: 10.1165/rcmb.2002-0274OC
48. Madsen J, Kliem A, Tornøe I, Skjødt K, Koch C, Holmskov U. Localization of Lung Surfactant Protein D on Mucosal Surfaces in Human Tissues. J Immunol (2000) 164(11):5866–70. doi: 10.4049/jimmunol.164.11.5866
49. MacNeill C, Umstead TM, Phelps DS, Lin Z, Floros J, Shearer DA, et al. Surfactant protein A, an innate immune factor, is expressed in the vaginal mucosa and is present in vaginal lavage fluid. Immunology (2004) 111(1):91–9. doi: 10.1111/j.1365-2567.2004.01782.x
50. Madsen J, Gaiha GD, Palaniyar N, Dong T, Mitchell DA, Clark HW. Surfactant Protein D Modulates HIV Infection of Both T-Cells and Dendritic Cells. PLoS One (2013) 8(3):e59047. doi: 10.1371/journal.pone.0059047
51. Gaiha GD, Dong T, Palaniyar N, Mitchell DA, Reid KBM, Clark HW. Surfactant protein a binds to HIV and inhibits direct infection of CD4(+) cells, but enhances dendritic cell-mediated viral transfer. J Immunol (2008) 181(1):601–9. doi: 10.4049/jimmunol.181.1.601
52. Pandit H, Gopal S, Sonawani A, Yadav AK, Qaseem AS, Warke H, et al. Surfactant protein D inhibits HIV-1 infection of target cells via interference with gp120-CD4 interaction and modulates pro-inflammatory cytokine production. PLoS One (2014) 9(7):e102395. doi: 10.1371/journal.pone.0102395
53. Yang CH, Szeliga J, Jordan J, Faske S, Sever-Chroneos Z, Dorsett B, et al. Identification of the surfactant protein A receptor 210 as the unconventional myosin 18A. J Biol Chem (2005) 280(41):34447–57. doi: 10.1074/jbc.M505229200
54. Chroneos ZC, Abdolrasulnia R, Whitsett JA, Rice WR, Shepherd VL. Purification of a cell-surface receptor for surfactant protein A. J Biol Chem (1996) 271(27):16375–83. doi: 10.1074/jbc.271.27.16375
55. Strayer D, Yang S, Jerng H. Surfactant protein A-binding proteins. Characterization and structures. J Biol Chem (1993) 268(25):18679–84.
56. Wissel H, Looman AC, Fritzsche I, Rustow B, Stevens PA. SP-A-binding protein BP55 is involved in surfactant endocytosis by type II pneumocytes. Am J Physiol-Lung Cell Mol Physiol (1996) 271(3):L432–L40. doi: 10.1152/ajplung.1996.271.3.L432
57. Kresch MJ, Christian C, Lu H. Isolation and partial characterization of a receptor to surfactant protein A expressed by rat type II pneumocytes. Am J Respiratory Cell Mol Biol (1998) 19(2):216–25. doi: 10.1165/ajrcmb.19.2.3061
58. Kielian TL, Blecha F. CD14 and other recognition molecules for lipopolysaccharide: a review. Immunopharmacology (1995) 29(3):187–205. doi: 10.1016/0162-3109(95)00003-C
59. Wright SD. CD14 and innate recognition of bacteria. J Immunol (Baltimore Md 1950) (1995) 155(1):6–8.
60. Dodagatta-Marri E, Mitchell DA, Pandit H, Sonawani A, Murugaiah V, Idicula-Thomas S, et al. Protein–Protein Interaction between Surfactant Protein D and DC-SIGN via C-Type Lectin Domain Can Suppress HIV-1 Transfer. Front Immunol (2017) 8(834). doi: 10.3389/fimmu.2017.00834
61. Gardai SJ, Xiao YQ, Dickinson M, Nick JA, Voelker DR, Greene KE, et al. By binding SIRP alpha or calreticulin/CD91, lung collectins act as dual function surveillance molecules to suppress or enhance inflammation. Cell (2003) 115(1):13–23. doi: 10.1016/S0092-8674(03)00758-X
62. Vandivier RW, Ogden CA, Fadok VA, Hoffmann PR, Brown KK, Botto M, et al. Role of surfactant proteins D, D, and C1q in the clearance of apoptotic cells in vivo and in vitro: Calreticulin and CD91 as a common collectin receptor complex. J Immunol (2002) 169(7):3978–86. doi: 10.4049/jimmunol.169.7.3978
63. Nepomuceno RR, Henschen-Edman AH, Burgess WH, Tenner AJ. cDNA cloning and primary structure analysis of C1qR(P), the human C1q/MBL/SPA receptor that mediates enhanced phagocytosis in vitro. Immunity (1997) 6(2):119–29. doi: 10.1016/S1074-7613(00)80419-7
64. McGreal EP, Ikewaki N, Akatsu H, Morgan BP, Gasque P. Human C1qRp is identical with CD93 and the mNI-11 antigen but does not bind C1q. J Immunol (Baltimore Md 1950) (2002) 168(10):5222–32. doi: 10.4049/jimmunol.168.10.5222
65. Tenner AJ, Robinson SL, Borchelt J, Wright JR. Human pulmonary surfactant protein (SP-A), a protein structurally homologous to C1q, can enhance FcR- and CR1-mediated phagocytosis. J Biol Chem (1989) 264(23):13923–8.
66. Janssen WJ, McPhillips KA, Dickinson MG, Linderman DJ, Morimoto K, Xiao YQ, et al. and D suppress alveolar macrophage phagocytosis via interaction with SIRP alpha. Am J Respir Crit Care Med (2008) 178(2):158–67. doi: 10.1164/rccm.200711-1661OC
67. Fournier B, Andargachew R, Robin AZ, Laur O, Voelker DR, Lee WY, et al. Surfactant protein D (Sp-D) binds to membrane-proximal domain (D3) of signal regulatory protein alpha (SIRPalpha), a site distant from binding domain of CD47, while also binding to analogous region on signal regulatory protein beta (SIRPbeta). J Biol Chem (2012) 287(23):19386–98. doi: 10.1074/jbc.M111.324533
68. Barrow AD, Palarasah Y, Bugatti M, Holehouse AS, Byers DE, Holtzman MJ, et al. OSCAR is a receptor for surfactant protein D that activates TNF-α release from human CCR2+ inflammatory monocytes. J Immunol (2015) 194(7):3317–26. doi: 10.4049/jimmunol.1402289
69. Ge MQ, Kokalari B, Flayer CH, Killingbeck SS, Redai IG, MacFarlane AW, et al. Correction: cutting edge: role of NK cells and surfactant protein D in dendritic cell lymph node homing: effects of ozone exposure. J Immunol (2016) 196(7):3212–. doi: 10.4049/jimmunol.1600095
70. Olde Nordkamp MJ, van Eijk M, Urbanus RT, Bont L, Haagsman HP, Meyaard L. Leukocyte-associated Ig-like receptor-1 is a novel inhibitory receptor for surfactant protein D. J Leukoc Biol (2014) 96(1):105–11. doi: 10.1189/jlb.3AB0213-092RR
71. von Bredow C, Hartl D, Schmid K, Schabaz F, Brack E, Reinhardt D, et al. Surfactant protein D regulates chemotaxis and degranulation of human eosinophils. Clin Exp Allergy J Br Soc Allergy Clin Immunol (2006) 36(12):1566–74. doi: 10.1111/j.1365-2222.2006.02598.x
72. Yamada C, Sano H, Shimizu T, Mitsuzawa H, Nishitani C, Himi T, et al. Surfactant protein A directly interacts with TLR4 and MD-2 and regulates inflammatory cellular response. Importance of supratrimeric oligomerization. J Biol Chem (2006) 281(31):21771–80. doi: 10.1074/jbc.M513041200
73. Yamazoe M, Nishitani C, Takahashi M, Katoh T, Ariki S, Shimizu T, et al. Pulmonary surfactant protein D inhibits lipopolysaccharide (LPS)-induced inflammatory cell responses by altering LPS binding to its receptors. J Biol Chem (2008) 283(51):35878–88. doi: 10.1074/jbc.M807268200
74. Ohya M, Nishitani C, Sano H, Yamada C, Mitsuzawa H, Shimizu T, et al. Human pulmonary surfactant protein D binds the extracellular domains of Toll-like receptors 2 and 4 through the carbohydrate recognition domain by a mechanism different from its binding to phosphatidylinositol and lipopolysaccharide. Biochemistry (2006) 45(28):8657–64. doi: 10.1021/bi060176z
75. Guillot L, Balloy V, McCormack FX, Golenbock DT, Chignard M, Si-Tahar M. Cutting edge: the immunostimulatory activity of the lung surfactant protein-A involves Toll-like receptor 4. J Immunol (2002) 168(12):5989–92. doi: 10.4049/jimmunol.168.12.5989
76. Gil M, McCormack FX, LeVine AM. Surfactant protein A modulates cell surface expression of CR3 on alveolar macrophages and enhances CR3-mediated phagocytosis. J Biol Chem (2009) 284(12):7495–504. doi: 10.1074/jbc.M808643200
77. Balagopal A, MacFarlane AS, Mohapatra N, Soni S, Gunn JS, Schlesinger LS. Characterization of the receptor-ligand pathways important for entry and survival of Francisella tularensis in human macrophages. Infect Immun (2006) 74(9):5114–25. doi: 10.1128/IAI.00795-06
78. Fukuzawa T, Ishida J, Kato A, Ichinose T, Ariestanti DM, Takahashi T, et al. Lung surfactant levels are regulated by Ig-Hepta/GPR116 by monitoring surfactant protein D. PLoS One (2013) 8(7):e69451. doi: 10.1371/journal.pone.0069451
79. Kurimura Y, Nishitani C, Ariki S, Saito A, Hasegawa Y, Takahashi M, et al. Surfactant protein D inhibits adherence of uropathogenic Escherichia coli to the bladder epithelial cells and the bacterium-induced cytotoxicity: a possible function in urinary tract. J Biol Chem (2012) 287(47):39578–88. doi: 10.1074/jbc.M112.380287
80. Umeda Y, Hasegawa Y, Otsuka M, Ariki S, Takamiya R, Saito A, et al. Surfactant protein D inhibits activation of non-small cell lung cancer-associated mutant EGFR and affects clinical outcomes of patients. Oncogene (2017) 36(46):6432–45. doi: 10.1038/onc.2017.253
81. Holmskov U, Lawson P, Teisner B, Tornoe I, Willis AC, Morgan C, et al. Isolation and characterization of a new member of the scavenger receptor superfamily, glycoprotein-340 (gp-340), as a lung surfactant protein-D binding molecule. J Biol Chem (1997) 272(21):13743–9. doi: 10.1074/jbc.272.21.13743
82. Tino MJ, Wright JR. Surfactant proteins A and D specifically stimulate directed actin-based responses in alveolar macrophages. Am J Physiol (1999) 276(1):L164–74. doi: 10.1152/ajplung.1999.276.1.L164
83. Jäkel A, Clark H, Reid KB, Sim RB. Surface-bound myeloperoxidase is a ligand for recognition of late apoptotic neutrophils by human lung surfactant proteins A and D. Protein Cell (2010) 1(6):563–72. doi: 10.1007/s13238-010-0076-0
84. Oosting RS, Wright J. Characterization of the surfactant protein A receptor: cell and ligand specificity. Am J Physiol-Lung Cell Mol Physiol (1994) 267(2):L165–L72. doi: 10.1152/ajplung.1994.267.2.L165
85. Watford WT, Smithers MB, Frank MM, Wright JR. Surfactant protein A enhances the phagocytosis of C1q-coated particles by alveolar macrophages. Am J Physiol-Lung Cell Mol Physiol (2002) 283(5):L1011–L22. doi: 10.1152/ajplung.00366.2001
86. Nadesalingam J, Reid KB, Palaniyar N. Collectin surfactant protein D binds antibodies and interlinks innate and adaptive immune systems. FEBS Lett (2005) 579(20):4449–53. doi: 10.1016/j.febslet.2005.07.012
87. Lin PM, Wright JR. Surfactant protein A binds to IgG and enhances phagocytosis of IgG-opsonized erythrocytes. Am J Physiol-Lung Cell Mol Physiol (2006) 291(6):L1199–L206. doi: 10.1152/ajplung.00188.2006
88. Hartshorn KL, White MR, Tecle T, Holmskov U, Crouch EC. Innate defense against influenza A virus: activity of human neutrophil defensins and interactions of defensins with surfactant protein D. J Immunol (2006) 176(11):6962–72. doi: 10.4049/jimmunol.176.11.6962
89. Doss M, White MR, Tecle T, Gantz D, Crouch EC, Jung G, et al. Interactions of alpha-, beta-, and theta-defensins with influenza A virus and surfactant protein D. J Immunol (Baltimore Md 1950) (2009) 182(12):7878–87. doi: 10.4049/jimmunol.0804049
90. Nadesalingam J, Bernal AL, Dodds AW, Willis AC, Mahoney DJ, Day AJ, et al. Identification and characterization of a novel interaction between pulmonary surfactant protein D and decorin. J Biol Chem (2003) 278(28):25678–87. doi: 10.1074/jbc.M210186200
91. Ujma S, Carse S, Chetty A, Horsnell W, Clark H, Madsen J, et al. Surfactant Protein A Impairs Genital HPV16 Pseudovirus Infection by Innate Immune Cell Activation in A Murine Model. Pathogens (2019) 8(4):288. doi: 10.3390/pathogens8040288
92. Iwaarden JFV, Strijp JAV, Ebskamp MJ, Welmers AC, Verhoef J, Golde LMV. Surfactant protein A is opsonin in phagocytosis of herpes simplex virus type 1 by rat alveolar macrophages. Am J Physiol-Lung Cell Mol Physiol (1991) 261(2):L204–L9. doi: 10.1152/ajplung.1991.261.2.L204
93. van Iwaarden JF, van Strijp JA, Visser H, Haagsman HP, Verhoef J, van Golde LM. Binding of surfactant protein A (SP-A) to herpes simplex virus type 1-infected cells is mediated by the carbohydrate moiety of SP-A. J Biol Chem (1992) 267(35):25039–43.
94. Georgescu SR, Mitran CI, Mitran MI, Caruntu C, Sarbu MI, Matei C, et al. New Insights in the Pathogenesis of HPV Infection and the Associated Carcinogenic Processes: The Role of Chronic Inflammation and Oxidative Stress. J Immunol Res (2018) 2018:5315816. doi: 10.1155/2018/5315816
95. Favier AL, Reynard O, Gout E, van Eijk M, Haagsman HP, Crouch E, et al. Involvement of Surfactant Protein D in Ebola Virus Infection Enhancement via Glycoprotein Interaction. Viruses (2018) 11(1):15. doi: 10.3390/v11010015
96. Rogers KJ, Brunton B, Mallinger L, Bohan D, Sevcik KM, Chen J, et al. IL-4/IL-13 polarization of macrophages enhances Ebola virus glycoprotein-dependent infection. PloS Neglected Trop Dis (2019) 13(12):e0007819. doi: 10.1371/journal.pntd.0007819
97. Duan S, Thomas PG. Balancing Immune Protection and Immune Pathology by CD8+ T-Cell Responses to Influenza Infection. Front Immunol (2016) 7(25). doi: 10.3389/fimmu.2016.00025
98. Cao X. COVID-19: immunopathology and its implications for therapy. Nat Rev Immunol (2020) 20(5):269–70. doi: 10.1038/s41577-020-0308-3
99. Wert SE, Yoshida M, LeVine AM, Ikegami M, Jones T, Ross GF, et al. Increased metalloproteinase activity, oxidant production, and emphysema in surfactant protein D gene-inactivated mice. Proc Natl Acad Sci (2000) 97(11):5972–7. doi: 10.1073/pnas.100448997
100. Clark H, Palaniyar N, Strong P, Edmondson J, Hawgood S, Reid KBM. Surfactant Protein D Reduces Alveolar Macrophage Apoptosis In Vivo. J Immunol (2002) 169(6):2892–9. doi: 10.4049/jimmunol.169.6.2892
101. Fisher JH, Larson J, Cool C, Dow SW. Lymphocyte activation in the lungs of SP-D null mice. Am J Respiratory Cell Mol Biol (2002) 27(1):24–33. doi: 10.1165/ajrcmb.27.1.4563
102. Knudsen L, Ochs M, MacKay R, Townsend P, Deb R, Muehlfeld C, et al. Truncated recombinant human SP-D attenuates emphysema and type II cell changes in SP-D deficient mice. Respiratory Res (2007) 8(70). doi: 10.1186/1465-9921-8-70
103. Schaub B, Westlake RM, He H, Arestides R, Haley KJ, Campo M, et al. Surfactant protein D deficiency influences allergic immune responses. Clin Exp Allergy J Br Soc Allergy Clin Immunol (2004) 34(12):1819–26. doi: 10.1111/j.1365-2222.2004.02068.x
104. Haczku A, Cao Y, Vass G, Kierstein S, Nath P, Atochina-Vasserman EN, et al. IL-4 and IL-13 form a negative feedback circuit with surfactant protein-D in the allergic airway response. J Immunol (Baltimore Md 1950) (2006) 176(6):3557–65. doi: 10.4049/jimmunol.176.6.3557
105. Brandt EB, Mingler MK, Stevenson MD, Wang N, Khurana Hershey GK, Whitsett JA, et al. Surfactant protein D alters allergic lung responses in mice and human subjects. J Allergy Clin Immunol (2008) 121(5):1140–7.e2. doi: 10.1016/j.jaci.2008.02.011
106. Madan T, Reid KB, Singh M, Sarma PU, Kishore U. Susceptibility of mice genetically deficient in the surfactant protein (SP)-A or SP-D gene to pulmonary hypersensitivity induced by antigens and allergens of Aspergillus fumigatus. J Immunol (Baltimore Md 1950) (2005) 174(11):6943–54. doi: 10.4049/jimmunol.174.11.6943
107. Becker Y. Respiratory syncytial virus (RSV) evades the human adaptive immune system by skewing the Th1/Th2 cytokine balance toward increased levels of Th2 cytokines and IgE, markers of allergy–a review. Virus Genes (2006) 33(2):235–52. doi: 10.1007/s11262-006-0064-x
108. Pilecki B, Wulf-Johansson H, Stottrup C, Jorgensen PT, Djiadeu P, Nexoe AB, et al. Surfactant Protein D Deficiency Aggravates Cigarette Smoke-Induced Lung Inflammation by Upregulation of Ceramide Synthesis. Front Immunol (2018) 9:3013. doi: 10.3389/fimmu.2018.03013
109. Linke MJ, Harris CE, Korfhagen TR, McCormack FX, Ashbaugh AD, Steele P, et al. Immunosuppressed surfactant protein A-deficient mice have increased susceptibility to Pneumocystis carinii infection. J Infect Dis (2001) 183(6):943–52. doi: 10.1086/319252
110. LeVine AM, Kurak KE, Wright JR, Watford WT, Bruno MD, Ross GF, et al. Surfactant protein-A binds group B Streptococcus enhancing phagocytosis and clearance from lungs of surfactant protein-A-deficient mice. Am J Respiratory Cell Mol Biol (1999) 20(2):279–86. doi: 10.1165/ajrcmb.20.2.3303
111. LeVine AM, Kurak KE, Bruno MD, Stark JM, Whitsett JA, Korfhagen TR. Surfactant protein-A-deficient mice are susceptible to Pseudomonas aeruginosa infection. Am J Respiratory Cell Mol Biol (1998) 19(4):700–8. doi: 10.1165/ajrcmb.19.4.3254
112. Hickman-Davis JM, Fang FC, Nathan C, Shepherd VL, Voelker DR, Wright JR. Lung surfactant and reactive oxygen-nitrogen species: antimicrobial activity and host-pathogen interactions. Am J Physiol Lung Cell Mol Physiol (2001) 281(3):L517–23. doi: 10.1152/ajplung.2001.281.3.L517
113. Atochina EN, Beck JM, Preston AM, Haczku A, Tomer Y, Scanlon ST, et al. Enhanced lung injury and delayed clearance of Pneumocystis carinii in surfactant protein A-deficient mice: attenuation of cytokine responses and reactive oxygen-nitrogen species. Infect Immun (2004) 72(10):6002–11. doi: 10.1128/IAI.72.10.6002-6011.2004
114. Borron P, McIntosh JC, Korfhagen TR, Whitsett JA, Taylor J, Wright JR. Surfactant-associated protein A inhibits LPS-induced cytokine and nitric oxide production in vivo. Am J Physiol-Lung Cell Mol Physiol (2000) 278(4):L840–L7. doi: 10.1152/ajplung.2000.278.4.L840
115. Hartshorn KL, Crouch E, White MR, Colamussi ML, Kakkanatt A, Tauber B, et al. Pulmonary surfactant proteins A and D enhance neutrophil uptake of bacteria. Am J Physiol-Lung Cell Mol Physiol (1998s) 274(6):L958–L69. doi: 10.1152/ajplung.1998.274.6.L958
116. Nayak A, Dodagatta-Marri E, Tsolaki A, Kishore U. An Insight into the Diverse Roles of Surfactant Proteins, SP-A and SP-D in Innate and Adaptive Immunity. Front Immunol (2012) 3(131). doi: 10.3389/fimmu.2012.00131
117. LeVine AM, Whitsett JA, Gwozdz JA, Richardson TR, Fisher JH, Burhans MS, et al. Distinct Effects of Surfactant Protein A or D Deficiency During Bacterial Infection on the Lung. J Immunol (2000) 165(7):3934–40. doi: 10.4049/jimmunol.165.7.3934
118. Carreto-Binaghi LE, Aliouat el M, Taylor ML. Surfactant proteins, SP-A and SP-D, in respiratory fungal infections: their role in the inflammatory response. Respir Res (2016) 17(1):66. doi: 10.1186/s12931-016-0385-9
119. Jakel A, Qaseem AS, Kishore U, Sim RB. Ligands and receptors of lung surfactant proteins SP-A and SP-D. Front Biosci-Landmark (2013) 18:1129–40. doi: 10.2741/4168
120. Heath CJ, del Mar Cendra M, Watson A, Auger J-P, Pandey A, Tighe P, et al. Co-transcriptomes of initial interactions in vitro between streptococcus pneumoniae and human pleural mesothelial cells. PloS One (2015) 10(11):e0142773. doi: 10.1371/journal.pone.0142773
121. Kabha K, Schmegner J, Keisari Y, Parolis H, SchlepperSchaefer J, Ofek I. SP-A enhances phagocytosis of Klebsiella by interaction with capsular polysaccharides and alveolar macrophages. Am J Physiol-Lung Cell Mol Physiol (1997) 272(2):L344–L52. doi: 10.1152/ajplung.1997.272.2.L344
122. Tsuruta T, Inoue R, Nagino T, Nishibayashi R, Makioka Y, Ushida K. Role of the mannose receptor in phagocytosis of Enterococcus faecalis strain EC-12 by antigen-presenting cells. MicrobiologyOpen (2013) 2(4):610–7. doi: 10.1002/mbo3.99
123. Wu HX, Kuzmenko A, Wan SJ, Schaffer L, Weiss A, Fisher JH, et al. Surfactant proteins A and D inhibit the growth of Gram-negative bacteria by increasing membrane permeability. J Clin Invest (2003) 111(10):1589–602. doi: 10.1172/JCI16889
124. Schaeffer LM, McCormack FX, Wu HX, Weiss AA. Bordetella pertussis lipopolysaccharide resists the bactericidal effects of pulmonary surfactant protein A. J Immunol (2004) 173(3):1959–65. doi: 10.4049/jimmunol.173.3.1959
125. Hickman-Davis JM, Lindsey JR, Zhu S, Matalon S. Surfactant protein A mediates mycoplasmacidal activity of alveolar macrophages. Am J Physiol-Lung Cell Mol Physiol (1998) 274(2):L270–L7. doi: 10.1152/ajplung.1998.274.2.L270
126. Hickman-Davis JM, Gibbs-Erwin J, Lindsey JR, Matalon S. Surfactant protein A mediates mycoplasmacidal activity of alveolar macrophages by production of peroxynitrite. Proc Natl Acad Sci USA (1999) 96(9):4953–8. doi: 10.1073/pnas.96.9.4953
127. Weikert LF, Lopez JP, Abdolrasulnia R, Chroneos ZC, Shepherd VL. Surfactant protein A enhances mycobacterial killing by rat macrophages through a nitric oxide-dependent pathway. Am J Physiol-Lung Cell Mol Physiol (2000) 279(2):L216–L23. doi: 10.1152/ajplung.2000.279.2.L216
128. Wilkinson TMA, Aris E, Bourne S, Clarke SC, Peeters M, Pascal TG, et al. A prospective, observational cohort study of the seasonal dynamics of airway pathogens in the aetiology of exacerbations in COPD. Thorax (2017) 72(10):919–27. doi: 10.1136/thoraxjnl-2016-209023
129. Rock KL, Kono H. The inflammatory response to cell death. Annu Rev Pathol (2008) 3:99–126. doi: 10.1146/annurev.pathmechdis.3.121806.151456
130. Elliott MR, Ravichandran KS. Clearance of apoptotic cells: implications in health and disease. J Cell Biol (2010) 189(7):1059–70. doi: 10.1083/jcb.201004096
131. Jäkel A, Clark H, Reid KBM, Sim RB. The human lung surfactant proteins A (SP-A) and D (SP-D) interact with apoptotic target cells by different binding mechanisms. Immunobiology (2010) 215(7):551–8. doi: 10.1016/j.imbio.2009.09.005
132. Jäkel A, Reid KB, Clark H. Surfactant protein A (SP-A) binds to phosphatidylserine and competes with annexin V binding on late apoptotic cells. Protein Cell (2010) 1(2):188–97. doi: 10.1007/s13238-010-0024-z
133. Schagat TL, Wofford JA, Wright JR. Surfactant Protein A Enhances Alveolar Macrophage Phagocytosis of Apoptotic Neutrophils. J Immunol (2001) 166(4):2727–33. doi: 10.4049/jimmunol.166.4.2727
134. Du J, Abdel-Razek O, Shi Q, Hu F, Ding G, Cooney RN, et al. Surfactant protein D attenuates acute lung and kidney injuries in pneumonia-induced sepsis through modulating apoptosis, inflammation and NF-κB signaling. Sci Rep (2018) 8(1):1–14. doi: 10.1038/s41598-018-33828-7
135. Djiadeu P, Kotra LP, Sweezey N, Palaniyar N. Surfactant protein D delays Fas-and TRAIL-mediated extrinsic pathway of apoptosis in T cells. Apoptosis Int J Programmed Cell Death (2017) 22(5):730–40. doi: 10.1007/s10495-017-1348-4
136. Vandivier RW, Ogden CA, Fadok VA, Hoffmann PR, Brown KK, Botto M, et al. Role of Surfactant Proteins A, D, and C1q in the Clearance of Apoptotic Cells In Vivo and In Vitro: Calreticulin and CD91 as a Common Collectin Receptor Complex. J Immunol (2002) 169(7):3978–86. doi: 10.4049/jimmunol.169.7.3978
137. Radic M, Marion T, Monestier M. Nucleosomes Are Exposed at the Cell Surface in Apoptosis. J Immunol (2004) 172(11):6692–700. doi: 10.4049/jimmunol.172.11.6692
138. Palaniyar N, Clark H, Nadesalingam J, Hawgood S, Reid KB. Surfactant protein D binds genomic DNA and apoptotic cells, and enhances their clearance, in vivo. Ann New Y Acad Sci (2003) 1010:471–5. doi: 10.1196/annals.1299.085
139. Douda DN, Jackson R, Grasemann H, Palaniyar N. Innate Immune Collectin Surfactant Protein D Simultaneously Binds Both Neutrophil Extracellular Traps and Carbohydrate Ligands and Promotes Bacterial Trapping. J Immunol (2011) 187(4):1856–65. doi: 10.4049/jimmunol.1004201
140. Yousefi S, Sharma SK, Stojkov D, Germic N, Aeschlimann S, Ge MQ, et al. Oxidative damage of SP-D abolishes control of eosinophil extracellular DNA trap formation. J Leukoc Biol (2018) 104(1):205–14. doi: 10.1002/JLB.3AB1117-455R
141. Palaniyar N, Clark H, Nadesalingam J, Shih MJ, Hawgood S, Reid KBM. Innate Immune Collectin Surfactant Protein D Enhances the Clearance of DNA by Macrophages and Minimizes Anti-DNA Antibody Generation. J Immunol (2005) 174(11):7352–8. doi: 10.4049/jimmunol.174.11.7352
142. Palaniyar N, Nadesalingam J, Clark H, Shih MJ, Dodds AW, Reid KBM. Nucleic Acid Is a Novel Ligand for Innate, Immune Pattern Recognition Collectins Surfactant Proteins A and D and Mannose-binding Lectin. J Biol Chem (2004) 279(31):32728–36. doi: 10.1074/jbc.M403763200
143. Magnan A, Botturi K, Pipet A, Cavaillès A, Reboulleau D, Langelot M, et al. 4 - Asthma exacerbations: a paradigm of synergy between allergens, pollutants and viruses. In: Williams MA, editor. Allergens and Respiratory Pollutants. Cambridge, United Kingdom: Woodhead Publishing (2011). p. 89–116. doi: 10.1533/9781908818065.89
144. Madan T, Kishore U, Shah A, Eggleton P, Strong P, Wang J-Y, et al. Lung surfactant proteins A and D can inhibit specific IgE binding to the allergens of Aspergillus fumigatus and block allergen-induced histamine release from human basophils. Clin Exp Immunol (1997) 110(2):241–9. doi: 10.1111/j.1365-2249.1997.tb08323.x
145. Deb R, Shakib F, Reid K, Clark H. Major house dust mite allergens Dermatophagoides pteronyssinus 1 and Dermatophagoides farinae 1 degrade and inactivate lung surfactant proteins A and D. J Biol Chem (2007) 282(51):36808–19. doi: 10.1074/jbc.M702336200
146. Malherbe DC, Erpenbeck VJ, Abraham SN, Crouch EC, Hohlfeld JM, Wright JR. Surfactant protein D decreases pollen-induced IgE-dependent mast cell degranulation. Am J Physiol Lung Cell Mol Physiol (2005) 289(5):L856–66. doi: 10.1152/ajplung.00009.2005
147. Malhotra R, Haurum J, Thiel S, Jensenius JC, Sim RB. Pollen grains bind to lung alveolar type II cells (A549) via lung surfactant protein A (SP-A). Biosci Rep (1993) 13(2):79–90. doi: 10.1007/BF01145960
148. Qaseem AS, Singh I, Pathan AA, Layhadi JA, Parkin R, Alexandra F, et al. A Recombinant Fragment of Human Surfactant Protein D Suppresses Basophil Activation and T-Helper Type 2 and B-Cell Responses in Grass Pollen-induced Allergic Inflammation. Am J Respir Crit Care Med (2017) 196(12):1526–34. doi: 10.1164/rccm.201701-0225OC
149. Mahajan L, Madan T, Kamal N, Singh VK, Sim RB, Telang SD, et al. Recombinant surfactant protein-D selectively increases apoptosis in eosinophils of allergic asthmatics and enhances uptake of apoptotic eosinophils by macrophages. Int Immunol (2008) 20(8):993–1007. doi: 10.1093/intimm/dxn058
150. Liu CF, Chen YL, Shieh CC, Yu CK, Reid KB, Wang JY. Therapeutic effect of surfactant protein D in allergic inflammation of mite-sensitized mice. Clin Exp Allergy J Br Soc Allergy Clin Immunol (2005) 35(4):515–21. doi: 10.1111/j.1365-2222.2005.02205.x
151. Fakih D, Pilecki B, Schlosser A, Jepsen CS, Thomsen LK, Ormhøj M, et al. Protective effects of surfactant protein D treatment in 1, 3-β-glucan-modulated allergic inflammation. Am J Physiol-Lung Cell Mol Physiol (2015) 309(11):L1333–L43. doi: 10.1152/ajplung.00090.2015
152. Marcy TW, Merrill WW. Cigarette smoking and respiratory tract infection. Clinics Chest Med (1987) 8(3):381–91.
153. Sethi S. Infection as a comorbidity of COPD. Eur Respiratory J (2010) 35(6):1209–15. doi: 10.1183/09031936.00081409
154. Day K, Ostridge K, Conway J, Cellura D, Watson A, Spalluto CM, et al. Interrelationships between small airways dysfunction, neutrophilic inflammation and exacerbation frequency in COPD. Chest (2020). doi: 10.1016/j.chest.2020.11.018
155. McKenzie Z, Kendall M, Mackay RM, Tetley TD, Morgan C, Griffiths M, et al. Nanoparticles modulate surfactant protein A and D mediated protection against influenza A infection in vitro. Philos Trans R Soc London Ser B Biol Sci (2015) 370(1661):20140049. doi: 10.1098/rstb.2014.0049
156. Kendall M, Ding P, Mackay RM, Deb R, McKenzie Z, Kendall K, et al. Surfactant protein D (SP-D) alters cellular uptake of particles and nanoparticles. Nanotoxicology (2013) 7(5):963–73. doi: 10.3109/17435390.2012.689880
157. Ciencewicki J, Gowdy K, Krantz QT, Linak WP, Brighton L, Gilmour MI, et al. Diesel exhaust enhanced susceptibility to influenza infection is associated with decreased surfactant protein expression. Inhalation Toxicol (2007) 19(14):1121–33. doi: 10.1080/08958370701665426
158. Harrod KS, Jaramillo RJ, Rosenberger CL, Wang SZ, Berger JA, McDonald JD, et al. Increased susceptibility to RSV infection by exposure to inhaled diesel engine emissions. Am J Respir Cell Mol Biol (2003) 28(4):451–63. doi: 10.1165/rcmb.2002-0100OC
159. White MR, Crouch E, Vesona J, Tacken PJ, Batenburg JJ, Leth-Larsen R, et al. Respiratory innate immune proteins differentially modulate the neutrophil respiratory burst response to influenza A virus. Am J Physiol-Lung Cell Mol Physiol (2005) 289(4):L606–L16. doi: 10.1152/ajplung.00130.2005
160. Sano H, Chiba H, Iwaki D, Sohma H, Voelker DR, Kuroki Y, et al. and D bind CD14 by different mechanisms. J Biol Chem (2000) 275(29):22442–51. doi: 10.1074/jbc.M001107200
161. Murakami S, Iwaki D, Mitsuzawa H, Sano H, Takahashi H, Voelker DR, et al. Surfactant protein A inhibits peptidoglycan-induced tumor necrosis factor-α secretion in U937 cells and alveolar macrophages by direct interaction with Toll-like receptor 2. J Biol Chem (2002) 277(9):6830–7. doi: 10.1074/jbc.M106671200
162. Hussain S, Wright JR, William J, Martin I. Surfactant Protein A Decreases Nitric Oxide Production by Macrophages in a Tumor Necrosis Factor-α–Dependent Mechanism. Am J Respiratory Cell Mol Biol (2003) 28(4):520–7. doi: 10.1165/rcmb.2002-0072OC
163. Minutti CM, García-Fojeda B, Sáenz A, de las Casas-Engel M, Guillamat-Prats R, de Lorenzo A, et al. Surfactant Protein A Prevents IFN-γ/IFN-γ Receptor Interaction and Attenuates Classical Activation of Human Alveolar Macrophages. J Immunol (2016) 197(2):590–8. doi: 10.4049/jimmunol.1501032
164. Tino MJ, Wright JR. Surfactant proteins A and D specifically stimulate directed actin-based responses in alveolar macrophages. Am J Physiol-Lung Cell Mol Physiol (1999) 276(1):L164–L74. doi: 10.1152/ajplung.1999.276.1.L164
165. Wright JR, Youmans DC. Pulmonary surfactant protein-A stimulates chemotaxis of alveolar macrophage. Am J Physiol (1993) 264(4):L338–L44. doi: 10.1152/ajplung.1993.264.4.L338
166. Schagat TL, Wofford JA, Greene KE, Wright JR. Surfactant protein A differentially regulates peripheral and inflammatory neutrophil chemotaxis. Am J Physiol Lung Cell Mol (2003) 284(1):L140–7. doi: 10.1152/ajplung.00125.2002
167. Crouch EC, Persson A, Griffin GL, Chang D, Senior RM. Interactions of pulmonary surfactant protein-D (SP-D) with human blood leukocytes. Am J Respiratory Cell Mol Biol (1995) 12(4):410–5. doi: 10.1165/ajrcmb.12.4.7695920
168. Thawer S, Auret J, Schnoeller C, Chetty A, Smith K, Darby M, et al. Surfactant Protein-D Is Essential for Immunity to Helminth Infection. PLoS Pathog (2016) 12(2):e1005461. doi: 10.1371/journal.ppat.1005461
169. Giustiniani J, de Masson A, Marie-Cardine A, Bouaziz J-D, Garbar C, Bagot M, et al. Identification of CD245 as myosin 18A, a receptor for surfactant A: A novel pathway for activating human NK lymphocytes effector function. AACR (2016) 5(5):e1127493. doi: 10.1158/1538-7445.AM2016-3231
170. Hortobagyi L, Kierstein S, Krytska K, Zhu X, Das AM, Poulain F, et al. Surfactant protein D inhibits TNF-alpha production by macrophages and dendritic cells in mice. J Allergy Clin Immunol (2008) 122(3):521–8. doi: 10.1016/j.jaci.2008.05.002
171. Brinker KG, Garner H, Wright JR. Surfactant protein A modulates the differentiation of murine bone marrow-derived dendritic cells. Am J Physiol-Lung Cell Mol Physiol (2003) 284(1):L232–L41. doi: 10.1152/ajplung.00187.2002
172. Hansen S, Lo B, Evans K, Neophytou P, Holmskov U, Wright JR. Surfactant protein D augments bacterial association but attenuates major histocompatibility complex class II presentation of bacterial antigens. Am J Respir Cell Mol Biol (2007) 36(1):94–102. doi: 10.1165/rcmb.2006-0195OC
173. Atochina EN, Gow AJ, Beck JM, Haczku A, Inch A, Kadire H, et al. Delayed clearance of Pneumocystis carinii infection, increased inflammation, and altered nitric oxide metabolism in lungs of surfactant protein-D knockout mice. J Infect Dis (2004) 189(8):1528–39. doi: 10.1086/383130
174. Borron P, Veldhuizen RAW, Lewis JE, Possmayer F, Caveney A, Inchley K, et al. Surfactant associated protein-a inhibits human lymphocyte proliferation and IL-2 production. Am J Respiratory Cell Mol Biol (1996) 15(1):115–21. doi: 10.1165/ajrcmb.15.1.8679215
175. Borron PJ, Mostaghel EA, Doyle C, Walsh ES, McHeyzer-Williams MG, Wright JR. Pulmonary surfactant proteins a and D directly suppress CD3(+)/CD4(+) cell function: Evidence for two shared mechanisms(1). J Immunol (2002) 169(10):5844–50. doi: 10.4049/jimmunol.169.10.5844
176. Mukherjee S, Giamberardino C, Thomas J, Evans K, Goto H, Ledford JG, et al. Surfactant Protein A Integrates Activation Signal Strength To Differentially Modulate T Cell Proliferation. J Immunol (2012) 188(3):957–67. doi: 10.4049/jimmunol.1100461
177. Borron PJ, Crouch EC, Lewis JF, Wright JR, Possmayer F, Fraher LJ. Recombinant rat surfactant-associated protein D inhibits human T lymphocyte proliferation and IL-2 production. J Immunol (Baltimore Md 1950) (1998) 161(9):4599–603.
178. Yang S, Milla C, Panoskaltsis-Mortari A, Ingbar DH, Blazar BR, Haddad IY. Human surfactant protein a suppresses T cell–dependent inflammation and attenuates the manifestations of idiopathic pneumonia syndrome in mice. Am J Respiratory Cell Mol Biol (2001) 24(5):527–36. doi: 10.1165/ajrcmb.24.5.4400
179. Lin K-W, Jen KY, Suarez CJ, Crouch EC, Perkins DL, Finn PW. Surfactant Protein D-Mediated Decrease of Allergen-Induced Inflammation Is Dependent upon CTLA4. J Immunol (2010) 184(11):6343–9. doi: 10.4049/jimmunol.0901947
180. Djiadeu P, Kotra LP, Sweezey N, Palaniyar N. Surfactant protein D delays Fas- and TRAIL-mediated extrinsic pathway of apoptosis in T cells. Apoptosis Int J Programmed Cell Death (2017) 22(5):730–40. doi: 10.1007/s10495-017-1348-4
181. Djiadeu P, Farmakovski N, Azzouz D, Kotra LP, Sweezey N, Palaniyar N. Surfactant protein D regulates caspase-8-mediated cascade of the intrinsic pathway of apoptosis while promoting bleb formation. Mol Immunol (2017) 92:190–8. doi: 10.1016/j.molimm.2017.10.016
182. Wilkinson TM, Li CKF, Chui CSC, Huang AKY, Perkins M, Liebner JC, et al. Preexisting influenza-specific CD4+ T cells correlate with disease protection against influenza challenge in humans. Nat Med (2012) 18(2):274–80. doi: 10.1038/nm.2612
183. Watson A, Spalluto CM, McCrae C, Cellura D, Burke H, Cunoosamy D, et al. Dynamics of IFN-β Responses during Respiratory Viral Infection. Insights for Therapeutic Strategies. Am J Respir Crit Care Med (2020) 201(1):83–94. doi: 10.1164/rccm.201901-0214OC
184. Ju CR, Liu W, Chen RC. Serum surfactant protein D: biomarker of chronic obstructive pulmonary disease. Dis Mark (2012) 32(5):281–7. doi: 10.1155/2012/509063
185. Zaky DSE, Naiem M, Eid HA, Adawy ZR, Abd-Elraheem SE, Mohamed ZAZ. Circulating surfactant protein-D as a biomarker of severity in stable chronic obstructive pulmonary diseases. Egyptian J Chest Dis Tuberculosis (2014) 63(3):553–9. doi: 10.1016/j.ejcdt.2014.03.011
186. Erpenbeck VJ, Schmidt R, Günther A, Krug N, Hohlfeld JM. Surfactant protein levels in bronchoalveolar lavage after segmental allergen challenge in patients with asthma. Allergy (2006) 61(5):598–604. doi: 10.1111/j.1398-9995.2006.01062.x
187. Hallman M, Merritt TA, Akino T, Bry K. Surfactant protein A, phosphatidylcholine, and surfactant inhibitors in epithelial lining fluid. Am Rev Respiratory Dis (1991) 144:1376–84. doi: 10.1164/ajrccm/144.6.1376
188. Bersani I, Speer CP, Kunzmann S. Surfactant proteins A and D in pulmonary diseases of preterm infants. Expert Rev Anti-infective Ther (2012) 10(5):573–84. doi: 10.1586/eri.12.34
189. Mackay RA, Townsend JP, Calvert J, Anthony M, Wilkinson AR, Postle AD, et al. Increased surfactant protein-D levels in the airways of preterm neonates with sepsis indicated responses to infectious challenges. Acta Paediatr (Oslo Norway 1992) (2019) 108(5):870–6. doi: 10.1111/apa.14630
190. Sato A, Ikegami M. SP-B and SP-C Containing New Synthetic Surfactant for Treatment of Extremely Immature Lamb Lung. PLoS One (2012) 7(7):e39392. doi: 10.1371/journal.pone.0039392
191. Kotecha S, Davies PL, Clark HW, McGreal EP. Increased prevalence of low oligomeric state surfactant protein D with restricted lectin activity in bronchoalveolar lavage fluid from preterm infants. Thorax (2013) 68(5):460–7. doi: 10.1136/thoraxjnl-2012-202729
192. Manicone AM. Role of the pulmonary epithelium and inflammatory signals in acute lung injury. Expert Rev Clin Immunol (2009) 5(1):63–75. doi: 10.1586/1744666X.5.1.63
193. Griese M, Wiesener A, Lottspeich F, von Bredow C. Limited proteolysis of surfactant protein D causes a loss of its calcium-dependent lectin functions. Biochim Biophys Acta (BBA) Mol Basis Dis (2003) 1638(2):157–63. doi: 10.1016/S0925-4439(03)00063-2
194. von Bredow C, Wiesener A, Griese M. Proteolysis of surfactant protein D by cystic fibrosis relevant proteases. Lung (2003) 181(2):79–88. doi: 10.1007/s00408-003-1008-z
195. Alcorn JF, Wright JR. Degradation of pulmonary surfactant protein D by Pseudomonas aeruginosa elastase abrogates innate immune function. J Biol Chem (2004) 279(29):30871–9. doi: 10.1074/jbc.M400796200
196. Rubio F, Cooley J, Accurso FJ, Remold-O’Donnell E. Linkage of neutrophil serine proteases and decreased surfactant protein-A (SP-A) levels in inflammatory lung disease. Thorax (2004) 59(4):318–23. doi: 10.1136/thx.2003.014902
197. Postle AD, Mander A, Reid KB, Wang JY, Wright SM, Moustaki M, et al. Deficient hydrophilic lung surfactant proteins A and D with normal surfactant phospholipid molecular species in cystic fibrosis. Am J Respir Cell Mol Biol (1999) 20(1):90–8. doi: 10.1165/ajrcmb.20.1.3253
198. McKelvey MC, Weldon S, McAuley DF, Mall MA, Taggart CC. Targeting proteases in cystic fibrosis lung disease: paradigms, progress, and potential. Am J Respir Crit Care Med (2019) 201(2):141–7. doi: 10.1164/rccm.201906-1190PP
199. Griese M, Essl R, Schmidt R, Rietschel E, Ratjen F, Ballmann M, et al. Pulmonary surfactant, lung function, and endobronchial inflammation in cystic fibrosis. Am J Respir Crit Care Med (2004) 170(9):1000–5. doi: 10.1164/rccm.200405-575OC
200. Hartl D, Tirouvanziam R, Laval J, Greene CM, Habiel D, Sharma L, et al. Innate Immunity of the Lung: From Basic Mechanisms to Translational Medicine. J Innate Immun (2018) 10(5-6):487–501. doi: 10.1159/000487057
201. Spalluto CM, Watson A, Mccrae C, Cellura D, Burke H, Cunoosamy D, et al. Dynamics of IFN-β responses during respiratory viral infection: insights for therapeutic strategies. ERJ Open Research (2019) 5(suppl 2):PP126.
202. Monk PD, Marsden RJ, Tear VJ, Brookes J, Batten TN, Mankowski M, et al. Safety and efficacy of inhaled nebulised interferon beta-1a (SNG001) for treatment of SARS-CoV-2 infection: a randomised, double-blind, placebo-controlled, phase 2 trial. Lancet Respir Med. (2020). doi: 10.1016/S2213-2600(20)30511-7
203. Salgado D, Fischer R, Schillberg S, Twyman RM, Rasche S. Comparative evaluation of heterologous production systems for recombinant pulmonary surfactant protein D. Front Immunol (2014) 5:623. doi: 10.3389/fimmu.2014.00623
204. Haagsman HP, Sargeant T, Hauschka PV, Benson BJ, Hawgood S. Binding of calcium to SP-A, a surfactant-associated protein. Biochemistry (1990) 29(38):8894–900. doi: 10.1021/bi00490a003
205. Brown-Augsburger P, Chang D, Rust K, Crouch EC. Biosynthesis of surfactant protein D. Contributions of conserved NH2-terminal cysteine residues and collagen helix formation to assembly and secretion. J Biol Chem (1996) 271(31):18912–9. doi: 10.1074/jbc.271.31.18912
206. Sato A, Whitsett JA, Scheule RK, Ikegami M. Surfactant protein-d inhibits lung inflammation caused by ventilation in premature newborn lambs. Am J Respir Crit Care Med (2010) 181(10):1098–105. doi: 10.1164/rccm.200912-1818OC
207. Leth-Larsen R, Garred P, Jensenius H, Meschi J, Hartshorn K, Madsen J, et al. A common polymorphism in the SFTPD gene influences assembly, function, and concentration of surfactant protein D. J Immunol (Baltimore Md 1950) (2005) 174(3):1532–8. doi: 10.4049/jimmunol.174.3.1532
208. Sorensen GL, Hoegh SV, Leth-Larsen R, Thomsen TH, Floridon C, Smith K, et al. Multimeric and trimeric subunit SP-D are interconvertible structures with distinct ligand interaction. Mol Immunol (2009) 46(15):3060–9. doi: 10.1016/j.molimm.2009.06.005
209. Clark HW. Untapped Therapeutic Potential of Surfactant Proteins: Is There a Case for Recombinant SP-D Supplementation in Neonatal Lung Disease? Neonatology (2010) 97(4):380–7. doi: 10.1159/000297770
210. Bill RM. Playing catch-up with Escherichia coli: using yeast to increase success rates in recombinant protein production experiments. Front Microbiol (2014) 5:85. doi: 10.3389/fmicb.2014.00085
211. Watson A, Sørensen GL, Holmskov U, Whitwell HJ, Madsen J, Clark H. Generation of novel trimeric fragments of human SP-A and SP-D after recombinant soluble expression in E. coli. Immunobiology (2020) 225(4):151953. doi: 10.1016/j.imbio.2020.151953
212. Strong P, Reid KBM, Clark H. Intranasal delivery of a truncated recombinant human SP-D is effective at down-regulating allergic hypersensitivity in mice sensitized to allergens of Aspergillus fumigatus. Clin Exp Immunol (2002) 130(1):19–24. doi: 10.1046/j.1365-2249.2002.01968.x
Keywords: surfactant protein A, surfactant protein D, recombinant fragment of human SP-D (rfhSP-D), inflammation, immunoregulation, coronavirus disease 2019, severe acute respiratory syndrome coronavirus 2 (2019-nCoV), therapeutic
Citation: Watson A, Madsen J and Clark HW (2021) SP-A and SP-D: Dual Functioning Immune Molecules With Antiviral and Immunomodulatory Properties. Front. Immunol. 11:622598. doi: 10.3389/fimmu.2020.622598
Received: 28 October 2020; Accepted: 14 December 2020;
Published: 19 January 2021.
Edited by:
Jean Sylvia Marshall, Dalhousie University, CanadaReviewed by:
Kenneth Reid, University of Oxford, United KingdomCopyright © 2021 Watson, Madsen and Clark. This is an open-access article distributed under the terms of the Creative Commons Attribution License (CC BY). The use, distribution or reproduction in other forums is permitted, provided the original author(s) and the copyright owner(s) are credited and that the original publication in this journal is cited, in accordance with accepted academic practice. No use, distribution or reproduction is permitted which does not comply with these terms.
*Correspondence: Alastair Watson, YS5zLndhdHNvbkBzb3Rvbi5hYy51aw==; orcid.org/0000-0002-6735-2567
Disclaimer: All claims expressed in this article are solely those of the authors and do not necessarily represent those of their affiliated organizations, or those of the publisher, the editors and the reviewers. Any product that may be evaluated in this article or claim that may be made by its manufacturer is not guaranteed or endorsed by the publisher.
Research integrity at Frontiers
Learn more about the work of our research integrity team to safeguard the quality of each article we publish.