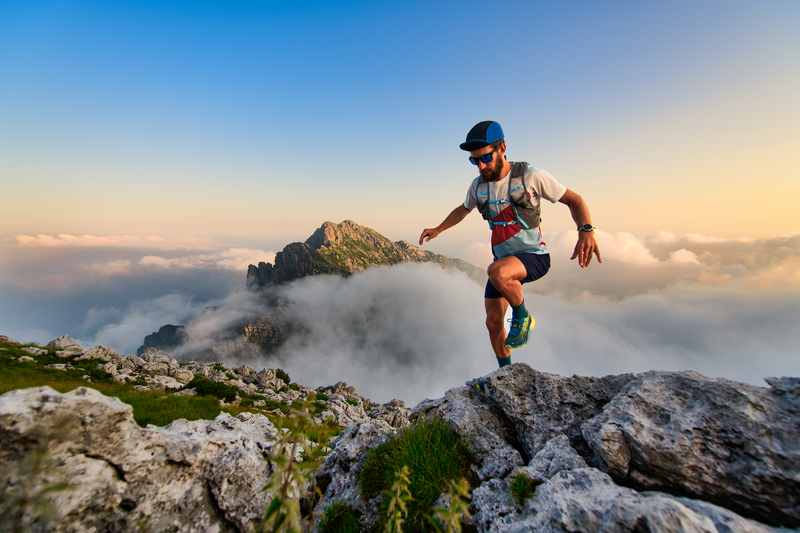
95% of researchers rate our articles as excellent or good
Learn more about the work of our research integrity team to safeguard the quality of each article we publish.
Find out more
REVIEW article
Front. Immunol. , 16 February 2021
Sec. Comparative Immunology
Volume 11 - 2020 | https://doi.org/10.3389/fimmu.2020.622377
This article is part of the Research Topic Gastrointestinal Immunity and Crosstalk with Internal Organs in Fish View all 13 articles
The development of mucosal vaccines against pathogens is currently a highly explored area of research in both humans and animals. This is due to the fact that mucosal vaccines have the potential to best elicit protective responses at these mucosal surfaces, which represent the frontline of host defense, thus blocking the pathogen at its initial replication sites. However, in order to provide an efficient long-lasting protection, these mucosal vaccines have to be capable of eliciting an adequate systemic immune response in addition to local responses. In aquaculture, the need for mucosal vaccines has further practical implications, as these vaccines would avoid the individual manipulation of fish out of the water, being beneficial from both an economic and animal welfare point of view. However, how B and T cells are organized in teleost fish within these mucosal sites and how they respond to mucosally delivered antigens varies greatly when compared to mammals. For this reason, it is important to establish which mucosally delivered antigens have the capacity to induce strong and long-lasting B and T cell responses. Hence, in this review, we have summarized what is currently known regarding the adaptive immune mechanisms that are induced both locally and systemically in fish after mucosal immunization through different routes of administration including oral and nasal vaccination, anal intubation and immersion vaccination. Finally, based on the data presented, we discuss how mucosal vaccination strategies could be improved to reach significant protection levels in these species.
Vaccination is a cost-efficient method to prevent the development of infectious diseases in humans and animals. Vaccine design aims to achieve long-term protection against a specific antigen by inducing an antigen-specific memory response in cells of the adaptive immune system, B and T cells, that will respond faster and with greater strength to a second encounter with this antigen. For this, antigen-presenting cells (APCs) are involved in digesting and degrading the antigen to provide antigen-specific activation of T cells, both CD4+ helper T cells and CD8+ cytotoxic T cells, the latter with the capacity to destroy virus-infected or tumor cells. B cells act as APCs and can also present the antigen to T cells, receiving in return activation signals from T helper cells in thymus-dependent (TD) responses or being activated by the antigen itself in the case of thymus-independent (TI) antigens. Upon activation, B cells start a differentiation process that leads them to a plasmablast state and eventually to become plasma cells, cells with a greater capacity to secrete immunoglobulins (Igs). Secreted Igs are referred to as antibodies. Throughout this differentiation process, memory B cells are also generated, as do memory T cells, also generated during the activation of T cells. In order to rationally design effective vaccines, it is fundamental to understand how these elements implicated in generating adaptive immunity respond to different vaccine formulations (1, 2). In mammals, different subsets of memory T lymphocytes have been described, including central memory T cells that remain in lymph and blood, tissue-resident memory T cells that reside within the tissues, and effector memory T cells that circulate amongst blood and peripheral tissues (3). In this context, CD4 tissue-resident memory T cells seem to play a key role in maintaining long-term protective immunity to mucosal pathogens as demonstrated in mice mucosally immunized with intranasal live attenuated vaccines against influenza virus (4) and coronaviruses (5). Regarding memory B lymphocytes, they are mainly found in the spleen and lymph nodes in mice and human, but can be also maintained as tissue-resident memory B cells at the sites of infection (6). Interestingly, intranasal live-attenuated influenza vaccines not only induced hemagglutinin-reactive plasmablasts but also memory B cells in the mediastinal lymph nodes (7). Therefore, the capacity of antigens to induce the generation of pathogen-specific tissue-resident memory B and T cells at mucosal surfaces seems as an important point to take into account when designing novel mucosal vaccines.
Most of the commercially available vaccines for both humans and animals are delivered through injection. However, in the past years, the interest and demand for the development of mucosal vaccines has considerably grown, taking into account that most infectious agents colonize the host through mucosal surfaces and it is at these sites that the initial replication steps occur (8). In this respect, having established that mucosal immunization has a superior ability to induce mucosal immune responses than systemic immunization (9), the search for effective mucosal vaccines is not only prompted from a logistic point of view, but also to produce vaccines that can efficiently block the pathogens at these early replicating sites before they are further disseminated throughout the organism. Despite all these advantages, there are only a few commercially available mucosal vaccines for humans or animals. This highlights the many challenges that the development of effective mucosal vaccines encounter. On one side, the vaccine formulations have to be correctly absorbed at the proper mucosal surface and taken up by a mucosal cell that can efficiently present the antigen. On the other hand, a successful mucosal vaccine has to overcome the mucosal tolerance that tightly controls the mucosal immune system to avoid unwanted immune responses to commensals or food antigens (8).
Despite these difficulties, some mucosal vaccines have shown to overcome mucosal tolerance and be able to generate efficient systemic immune responses in addition to local responses, including the production of specific antibodies and cell-mediated immunity (10–12), being this the basis for their efficacy, generating high levels of protection in humans. This is the case of the attenuated oral vaccine for the prevention of typhoid fever, caused by Salmonella enterica serovar Typhi, which elicits cell-mediated immune responses, involving both CD4+ helper T lymphocytes and CD8+ cytotoxic T cells (13). It is important to mention that although CD8+ T lymphocytes are mainly associated with defense against viral infections, these cells also play an important role clearing intracellular bacterial infections (14). Along the same line, the killed bivalent whole-cell Vibrio cholerae O1 and O139 vaccine produces a high increase of antibody titers and a strong O antigen–specific B memory response after oral immunization (15). In the case of mucosal vaccines against viruses, the intranasal administration of inactivated and live-attenuated influenza vaccines induce protective antibodies capable of inhibiting hemagglutination in serum (16). In this case, however, only the live-attenuated and not the inactivated vaccine generated influenza-specific CD4+ and CD8+ T cells (16). Additionally, the protective immune response to two oral live-attenuated rotavirus vaccines composed of a monovalent attenuated human rotavirus or five bovine–human rotaviruses is mostly due to rotavirus-specific immunoglobulin A (IgA) in serum (17). Oral vaccination has been also revealed successful to control infectious diseases in wildlife, including an oral vaccine against rabies used in foxes (18) and one against classical swine fever (CSF) administered to wild boar (19). Therefore, it seems that when mucosal vaccines are capable of inducing strong systemic adaptive immune effects, protection can be achieved.
In aquaculture, most commercially available vaccines are administrated by intraperitoneal injection, often providing a strong and long-lasting protection, especially in the case of antibacterial vaccines. However, this method involves fish handling which provokes a great amount of stress to the fish, which at the end results in reduced responsiveness to the vaccine and even in some unwanted mortalities. Furthermore, vaccination cannot be administered by injection at very early life stages, and it is precisely in these stages when fish are more susceptible to infectious diseases (20–22). For this reason, huge efforts are focused to find new approaches of mass vaccine administration through mucosal surfaces (e.g., oral immunization and immersion) as these routes have benefits from both an economic/practical and an animal welfare point of view (20–22).
Teleost fish lack well-organized lymphoid structures in their mucosal associated lymphoid tissues (MALTs) such as Peyer’s patches in the intestine or tonsils and adenoids in the nasal cavity but instead present diffuse immune cells scattered throughout the tissue. Although some authors accurately point out that this does not constitute a real MALT, for clarity, we will designate these immune cells as MALT as referred to in most fish immunology papers (23–25). In order to succeed in the development of mucosal vaccination for aquacultured fish, it is imperative that we understand how the regulatory constituents of the local immune system in teleost fish guarantee that antigens presented in MALTs produce appropriate systemic immune responses. In addition, teleost fish B cell responses also differ in many aspects from mammalian B2 cell (conventional B cell) responses, as fish B cells retain many innate features such as a strong phagocytic capacity and microbicidal activity (26), also described in mammalian innate B1 lymphocytes (27).
Teleost fish only express three antibody isotypes (IgM, IgD, and IgT/Z). Thus, fish B cells have never been shown to undergo class switch recombination, a process through which mammals replace the constant region of IgM by that of IgA, IgE, or IgG, antibodies with higher affinity and different effector functions (28, 29). In fact, in mammalian mucosal surfaces, it is IgA that plays a predominant immune role (30). Thus, in teleost fish, the majority of naïve B cells from central immune organs co-express IgM and IgD on the cell membrane, resembling naïve B cell subsets present in mammals (31). When these cells are activated, as in mammals, IgD is lost from the cell membrane, giving the rise to activated IgM+ B cells that have a plasmablast/plasma cell profile (32). However, unlike mammals, these cells do not differentiate further to IgA-secreting plasma cells. Given the lack of IgA in fish, the fish-specific IgT has been proposed as a functional equivalent of IgA based on recent findings. IgT+ B cells, which represent an independent B cell lineage, are more abundant than IgM+ B cells in mucosal surfaces such as the gut (33), the skin (34), and the gills (35), secreting polymeric IgT into the mucus to protect these tissues from pathogens. Finally, when fish were exposed to specific parasitic infections, IgT responses were shown to be predominant in the mucosal compartments in contrast to IgM responses that seemed restricted to systemic compartments (33–35). Despite this, systemic IgT responses have also been reported to both mucosal and systemic stimulations (36, 37), therefore the precise role of IgT in fish adaptive immunity is still largely unknown.
Interestingly, in mammals, some cells have been shown to undergo an unconventional class switch through which only IgD is produced (38, 39). These cells, that can secrete IgD, have been reported mainly in the upper respiratory tract (38, 39). Despite their identification, the precise role of IgD in mammals is still unknown. Thus far, circulating IgD has been shown to interact with basophils through a calcium-fluxing receptor inducing antimicrobial, opsonizing, inflammatory, and immunostimulating factors (39). Equivalent cells only expressing IgD on the cell membrane (IgD+ B cells) have been reported in channel catfish (Ictalurus punctatus) blood (40) and rainbow trout (Oncorhynchus mykiss) gills and intestine (41, 42), and secreted IgD has been identified as well in many fish species including catfish, rainbow trout, Atlantic salmon (Salmo salar), Atlantic cod (Gadus morhua), and grass carp (Ctenopharyngodon idella) (40, 43). Interestingly, in rainbow trout, IgD secreted by these gut IgD+ B cells, has been shown to coat the intestinal microbiota (42) as described for teleost IgM and IgT (33), suggesting that secreted IgD may play an evolutionary conserved role in mucosal homeostasis (42), as previously suggested in mice (44).
Cellular immunity also plays a key role in mucosal protection against pathogens through the generation of memory T cell subsets localized in mucosal tissues (45). For instance, in mice, an intratracheal vaccination strategy that combined Toll-like receptor (TLR) agonists with antigen-carrying lipid nanocapsules generated long-lived memory T cells not only in lungs but also in the intestinal and vaginal mucosa (45). Similarly, the pulmonary administration of a plasmid DNA vaccine induced robust systemic CD8+ T-cell responses in lungs and draining lymph nodes of mice equivalent to those generated by intramuscular vaccination (46). Recently, it has been shown in mice that the protective effect exerted by the intranasal administration of a recombinant murine cytomegalovirus expressing an MHC I restricted epitope from influenza A virus H1N1 was critically mediated by antigen-specific CD8+ T cells (47). In fish, a high number of T cells have been reported to be present in mucosal surfaces such as intestine and gills in several fish species and the role of CD8+ T cells mediating cellular protection against intracellular bacteria or viruses has been established [reviewed by Nakanishi et al. (48)]. However, to our knowledge, information regarding how T cells locally respond to mucosal vaccination in fish is missing.
Taking all these specific features of the fish immune system into account, the characterization of how mucosal immune cells respond to antigens locally, and then deliver activation signals to systemic immune tissues has special relevance to find out which type of mucosally delivered vaccine formulations are capable of providing strong and long-lasting B and T cell memory responses in these species. Consequently, we have focused this work on reviewing the adaptive immune responses elicited in fish upon mucosal immunization through different routes of administration including oral and nasal vaccination, anal intubation, and immersion vaccination. We have also highlighted the gaps found to evaluate T and B cell responses for some specific vaccines and routes of administration. Specifically, we have considered those studies in which at least one of the following responses has been reported: antibody response in serum, transcriptomic effect in genes related to adaptive immune response (e.g., IgM, IgT, IgD, MHC II, CD4, and CD8), as well as T cell-mediated immunity and B cell responses observed in mucosal and also in central tissues as thymus, peripheral blood, head kidney, and spleen. Finally, taking all this information into consideration, we discuss several strategies to improve the efficiency of mucosal vaccination in teleost fish.
Several studies have been focused on evaluating the local and systemic effects of oral immunization against bacteria and viruses in fish since this route of administration requires less fish handling, induces minimum stress, reduces side effects, and also allows vaccination at any stage of the life cycle in fish (49). Oral immunization of antigens can be administered by a great variety of ways including non-encapsulated or encapsulated feed, gavage (considered as an experimental way of delivering vaccines) or through the use of bioencapsulation vectors such as planktonic Crustacea (e.g., Daphnia), rotifers, and Artemia, all of them aimed at protecting the antigen from degradation until it reaches the most posterior segments of the digestive tract where immune induction has been shown to take place (21). These include the hindgut (50, 51) as well as the pyloric caeca, characterized in rainbow trout to be one of the most responsive gut segments to immunization (52).
Some vaccines designed against bacterial pathogens have been shown to produce high levels of serum antibodies when administrated in the fish feed (53–57). For instance, the level of protective antibodies elicited by a live attenuated Streptococcus agalactiae vaccine in Nile tilapia (Oreochromis niloticus) was significantly higher compared to the control group, reaching their maximum levels at 14–21 days after the immunization (55). In the case of the red tilapia hybrid (Oreochromis mossambicus x O. niloticus), fish vaccinated with formalin-killed St. agalactiae showed significantly higher IgM levels compared to controls, lasting up to 6 weeks after administration of the first booster dose (54). However, when a double booster regime was undertaken consistent higher levels of antibodies were reached until week 12, suggesting that this feed-based vaccination regime is a suitable strategy to obtain a long-lasting protection (54). Similarly, rainbow trout immunized with a non-microencapsulated live rifampicin-attenuated oral vaccine against Flavobacterium psychrophilum reached higher antibody titers than those obtained in fish vaccinated with the equivalent microencapsulated vaccine (53). Interestingly, a similar antibody response was observed between fish vaccinated orally and those intraperitoneally injected with the non-encapsulated vaccine (53). In the same way, oral vaccination of Atlantic salmon (S. salar) with live attenuated Piscirickettsia salmonis using a bioadhesive MicroMatrixTM cationic polysaccharide formulation produced systemic anti-P. salmonis IgM with a magnitude slightly higher than that of sera obtained from intraperitoneally vaccinated fish (57). In this study, specific antibodies were also detected in the intestinal mucosa of the oral vaccine-treated group at 350 degree-days post-vaccination (57).
In addition to these studies that show that some oral vaccination strategies are capable of producing systemic in addition to mucosal antibodies (57), there are very few data available on the characterization of B and T cell responses in central or mucosal tissues after oral immunization by means of transcriptional or functional analysis. In this sense, a study performed in gilthead sea bream (Sparus aurata) showed that the levels of specific IgM antibodies in the serum and IgT antibodies in skin mucus were significantly increased in orally vaccinated animals when challenged with Photobacterium damselae subsp. piscicida (37). In this case, challenged fish that had been orally vaccinated showed increased transcription of secreted IgT in intestine and head kidney, and much higher in spleen when compared to challenged unvaccinated fish (37). Regarding IgM, the transcription of membrane IgM was significantly upregulated in spleen, whereas the transcription of both membrane and secreted IgM forms were significantly downregulated in the intestine (37).
As an alternative to being a route for vaccination per se, oral vaccination has been proposed as a booster to acquire longer protection to intraperitoneally administered antigens. In this sense, the production of specific IgM antibodies was significantly increased when Atlantic salmon, rainbow trout and coho salmon (Oncorhynchus kisutch) were boosted orally after having been vaccinated with an injectable mono or polyvalent vaccine against salmonid rickettsial septicemia (SRS) (56). The oral boosters not only increased the magnitude of the response but also maintained the antibody response for longer, up to 2800–3200 degree-days (56).
The development of oral vaccines against viruses has been the aim of many studies in recent years (49, 50, 52, 58–63). Some of these studies have shown that oral antiviral vaccines can increase both systemic and mucosal immune responses to a previously administered intraperitoneal vaccine. Specifically, the administration of alginate-encapsulated antigens of infectious pancreatic necrosis virus (IPNV) in the feed after intraperitoneal vaccination in Atlantic salmon induced not only a significant higher antibody response following the first and the second booster, but also a significant higher CD4 and GATA3 expression in head kidney and spleen, known to be associated with T helper 2 responses that induce the secretion of Igs by B cells (61). Additionally, IgT transcription was also significantly increased in the intestine of fish orally immunized with the alginate-encapsulated vaccine (61). In some other cases, the antiviral vaccine was capable of inducing a strong immune response on its own. For example, in rainbow trout, detectable levels of anti-IPNV neutralizing antibodies were observed 90 days after administering along with the feed, for three consecutive days, 10 µg of an alginate-encapsulated DNA vaccine coding for the VP2 gene of IPNV (pcDNA-VP2) (59). Furthermore, IgM and IgT transcription was also significantly increased in response to this vaccine in kidney at day 30 post-vaccination (59). When this oral DNA vaccine was administered directly into the esophagus, neutralizing antibodies were also evident at 2, 3, 4, and 8 weeks post-vaccination (62). Additionally, IgM and IgT mRNA levels strongly increased in pyloric caeca, and to a lesser extent in head kidney (60). Accordingly, the pyloric caeca region was the area of the digestive tract in which a significant increase in the number of both IgM+ and IgT+ lymphocytes was more evident in response to this oral alginate-encapsulated IPNV DNA vaccine by means of immunohistochemistry analysis (52). Similarly, anti-IPNV antibodies were also detected in the sera of rainbow trout orally vaccinated with a DNA vaccine encapsulated in alginate microparticles as well as in chitosan/tripolyphosphate nanoparticles (58). In this case, the transcription levels of IgM, IgT, and CD4 (related to the presence of T helper cells) were dependent on the vaccine dose and were significantly upregulated in rainbow trout head kidney of all orally vaccinated fish groups compared to controls (58).
A significant increase in the production of antibodies against the infectious hematopoietic necrosis virus (IHNV) was detected in serum samples of rainbow trout vaccinated with an alginate microsphere encapsulated-DNA vaccine compared to sera from unvaccinated fish (50). Remarkably, this vaccine also induced the expression of several markers of the adaptive immune response, such as CD4, CD8, IgM, and IgT in the kidney and the spleen in a dose-dependent manner, although it should be noted that the vaccine doses that had to be used to achieve these responses were much higher than those used in the case of the oral alginate-encapsulated IPNV DNA vaccine (50). In 2017, a description of mucosal and systemic immune responses to IHNV induced by an orally delivered yeast expressing the G of IHNV on the cell surface was reported (63). In this work, the oral vaccine provoked a significant upregulation of CD8, IgM, and IgT mRNA levels in the hindgut, spleen, and head kidney from rainbow trout, with stronger levels reached in the intestine than in the other tissues (63).
The capacity of orally administered antiviral vaccines to induce antibodies has also been demonstrated in several species of carp (64–68). A single oral immunization using a formalin-inactivated crucian carp hematopoietic necrosis virus (CHNV) was not sufficient to induce measurable serum antibody responses in ginbuna crucian carp (Carassius auratus langsdorfii) (67). However, when fish were orally boosted 7 days after the initial oral immunization, significant antibody titers were reached between 30 and 60 days post-booster (67). Remarkably, orally immunized had higher antibody titers compared to non-immunized controls, and also significant cytotoxic peripheral blood lymphocytes (PBLs) against fibroblastic target cells fish and intraperitoneally infected when challenged with the live virus (67). In another study, VP6-specific serum antibodies were detected in grass carp (Ctenopharyngodon idella) during 2–8 weeks after vaccination with a recombinant baculovirus containing the vp6 gene from grass carp reovirus (GCRV) (68). Similarly, when common carp (Cyprinus carpio) and koi (C. carpio koi) were orally immunized with a recombinant Lactobacillus plantarum expressing the G protein of spring viremia of carp virus (SVCV) combined with the ORF81 protein of koi herpesvirus (KHV), significant levels of specific IgM were detected against the two viruses (64). Following a different approach, when common carp (C. carpio var. Jian) larvae were fed with Artemia coated with recombinant Saccharomyces cerevisiae expressing the cyprinid herpesvirus-3 (CyHV-3) envelope antigen pORF65 high levels of specific anti-pORF65 antibodies were induced, in addition to higher levels of IgM and CD8α transcription in the spleen (66). Similarly, the oral gavage of S. cerevisiae expressing CyHV-3 ORF131 evoked the production of specific serum IgM as well as the increase of the IgT1 mRNA levels in the spleen and the head kidney (65). However, no differences were observed between carps immunized with vaccine and the control yeast, attributing the increase of the IgT expression to the mucosal modulator of the yeast rather than to the expressed protein in this microorganism (65).
There are two methods to immunize fish by immersion: i) dip vaccination in which fish are immersed in water containing a relatively high dose of vaccine for few seconds or minutes; and ii) bath vaccination in which fish are introduced in a more diluted vaccine solution for a longer period of time (69). Although the great advantage of immersion vaccination is that the antigen is taken up through the skin, the intestine and gills, very large amounts of antigen are required and usually the levels of protection reached are not high, possible due to the fact that only a limited amount of antigen reaches the immune effector sites in these mucosal tissues (70). Despite this, being a method suitable to vaccinate a large number of fish at the same time, it has been used to administer many commercial antibacterial vaccines for fish. Commercial immersion vaccines commonly used in aquaculture include vaccines such as that against pasteurellosis for sea bream or against vibriosis for sea bass (Dicentrarchus labrax) and turbot (Scophthalmus maximus).
Most studies focused on establishing the local and systemic effects of immersion vaccines has been carried out with antibacterial vaccines, specifically against Yersinia ruckeri, F. psychrophilum, Listonella (formerly Vibrio) anguillarum, and Edwardsiella tarda. In most of these studies, except for those performed with E. tarda, the vaccines were administered by the dip methodology. Thus, dip vaccination of rainbow trout against enteric red mouth disease (ERM) using a commercial Y. ruckeri bacterin biotype 1 (AQUAVAC ERM vaccine, MSD Animal Health) (1:10 diluted-vaccine for 5 min) has shown to significantly increase Y. ruckeri-specific IgM antibody titers at 4, 8, and 26 weeks post-vaccination (71). Similarly, a booster vaccination using different dilutions (1:100 and 1:1,000) of the commercial vaccine AQUAVAC RELERA (MSD Animal Health) which combines Y. ruckeri biotypes 1 and 2, significantly increased antibody levels compared to fish vaccinated with only one dose (72). Interestingly, both commercial vaccines when administered by dip vaccination (1:10 diluted-vaccine for 30 s) increased the expression of adaptive immune genes in the later phase of infection, and also the occurrence of CD8+ and IgM+ cells in the spleen and head kidney, thus correlating the cellular changes with protection of vaccinated fish (73).
Significant levels of F. psychrophilum-specific antibodies were also reported in dip vaccinated rainbow trout (1:10 diluted-vaccine for 3 min) in several studies (70, 74). In addition, the administration of a polyvalent whole cell vaccine containing formalin-inactivated F. psychrophilum (1:10 diluted-vaccine for 30 s) elicited a low, although significant, increment of serum IgT at 6 weeks post-vaccination in rainbow trout (75). In this study, the number of IgT+ cells detected in spleen and head kidney of immersion vaccinated fish was also significantly higher in vaccinated fish. Intriguingly, the expression of CD8α in the head-kidney at day 2 and the levels of CD4-1 and CD8α transcription in the spleen at day 7 were significantly downregulated in vaccinated fish, suggesting the mobilization of both helper and cytotoxic T cells from these organs. However, IgT and IgM transcription levels were significantly upregulated in the hindgut at day 2 (75).
Dip vaccination of sea bass with a whole killed L. anguillarum serotype O1 and O2 bivalent commercial vaccine (MICROViB, Microtek International) also produced an increase of specific IgM antibodies (76). In zebrafish (Danio rerio) dip vaccinated for 8 min with attenuated L. anguillarum, the specific antibody levels did not rise significantly following vaccination (77). A recent study analyzed the response of Atlantic cod (G. morhua) to a dip vaccination against L. anguillarum (1:10 diluted-vaccine for 30 s), revealing changes in the levels of transcription of genes related to adaptive immunity processes as established by means of a transcriptome analysis in head kidney (78). Thus, genes related to T cell activity (CD8, TRGC1, TNFSF11, EOMES, PERF1, UNC13D), and B cell activity (IGLC2) were positively differentially expressed in vaccinated fish (78). Specifically, TRGC1 is a T cell receptor gamma and TNFSF11 is involved in the regulation of T cell-dependent immune responses. On the other hand, the increase in EOMES, PERF1, and UNC13D levels observed in vaccinated fish suggest the specific activation of cytotoxic T cells that preferentially express these genes (78).
Regarding the immune response elicited by vaccines administered by bath, the immunization of Japanese flounder (Paralichthys olivaceus) with a formalin-inactivated form of E. tarda produced a significantly higher upregulation of MHC II, TCRα, CD4-1, and IgT transcription in the skin and gills than that observed in spleen and kidney (79). On the contrary, the expression of IgM was much higher in lymphoid organs than in mucosal tissues (79).
The efficacy of antiviral vaccines administered by bath to induce B and T cell responses has been also demonstrated in fish (80–82). For instance, a significant specific antibody response was detected in carp 28 and 36 days after bath vaccination for 30 min with a KHV vaccine which consisted in inactivated Escherichia coli expressing glycoprotein-25 (80). In another study, carbon nanotubes were tested as vaccine carriers to enhance the efficacy of an immersion vaccine (81, 82). Specifically, functionalized single-walled carbon nanotubes (SWCNTs) used as carriers of a DNA vaccine encoding a matrix protein of SVCV and expressing a green fluorescent protein (pEGFP-M) produced a significant increase of antibody levels and an upregulation of IgM, IgZ1, CD8β, CD4, MHC I, and MHC II transcription levels in carp immunized by bath twice for 10 h after a 3 day interval (81), suggesting the activation of both cytotoxic and helper T lymphocytes upon immunization. Similarly, bath vaccination of Mandarin fish (Siniperca chuatsi) for 10 h with a subunit vaccine system encoding the major capsid protein (MCP) of the infectious spleen and kidney necrosis virus (ISKNV) based on SWCNTs produced higher levels of antibody as well as higher IgM mRNA levels than those obtained in groups vaccinated with the naked vaccine (82).
After the identification of the nasal-associated lymphoid tissue (NALT), some studies have emphasized the importance of nasal immunity to both infections and intranasal immunizations (83). In this respect, the efficacy of nasal vaccines has been uniquely demonstrated in rainbow trout (25, 84, 85), and as a consequence, only a few studies have identified the mucosal and systemic mechanisms elicited by vaccines administered through this route (23, 25).
There is only one study focused on how fish respond locally and systemically to an antibacterial vaccine administered through the nasal cavity (23). In this study, an inactivated vaccine against Y. ruckeri administered either nasally or intraperitoneally in rainbow trout produced an increase of the nasal IgM repertoire diversity, indicating that both local and systemic antigen exposures are capable of inducing B cell responses in the NALT (23). In the case of splenic B cells, nasal vaccination decreased both IgM and IgT clonotype diversity. On the other hand, specific serum IgM and IgT titers were significantly risen after intraperitoneal vaccination but not after nasal immunization (23).
Concerning antiviral vaccines, the administration of a live attenuated IHNV vaccine into the olfactory cup, was reported to elicit an antiviral adaptive immune response in the head kidney and the olfactory organ similar to that triggered by IHNV intramuscular vaccination (25). Moreover, the expression of IgM mRNA levels were significantly higher in the olfactory tissue at day 14 post-immunization in comparison to day 4, indicating the capacity of this vaccine to induce an adaptive immune response (25).
Some studies have explored the effects of anal immunization. Although this immunization strategy offers many disadvantages from a practical point of view, it can be considered as a way to investigate how the intestinal mucosa directly reacts to antigens without having to protect the antigen from degradation in the upper digestive segments (86, 87). In 2000, a study addressed how rainbow trout responded to fluorescein isothiocyanate (FITC) conjugated to keyhole limpet hemocyanin (KLH) when was administered intraperitoneally or anally (88). The results showed that fish injected with FITC-KLH developed higher antibody level of mucosal and serum anti-FITC antibodies when compared to fish immunized anally (88). Recently, the anal route has been used to study specific B cell responses to two model antigens, TNP-KLH (2,4,6-trinitrophenyl KLH), a TD antigen and TNP-LPS, a TI antigen (87). This study demonstrated that, in the absence of additional adjuvants, rainbow trout preferentially responded to anally administered TNP-LPS, while the response to TNP-KLH was much weaker. Thus, TNP-LPS elicited TNP-specific serum IgM, and a significant increase in the number of total and TNP-specific IgM-secreting cells in both spleen and kidney, with the kidney being the site where most of these cells were found at later time points. In the spleen, a proliferative response of both IgM+ B and T cells was also clearly visible at early time points, while weaker proliferative responses were observed in the kidney. At transcriptional level, a significant decrease in FOXP3A, FOXP3B, CD4, and CD8 mRNA levels was detected in the intestine of TNP-LPS-immunized fish, which suggested a local downregulation of different T cell subsets (87).
Few data is available concerning the effect of antibacterial vaccines administered anally. A L. anguillarum inactivated vaccine as well as the extracellular products obtained when producing the vaccine were shown to increase antibody titers in carp 21 days after anal intubation (89).
A formalin-inactivated CHNV administered by anal intubation produced a significant upregulation of TCRß mRNA levels in the kidney and the intestine of ginbuna crucian carp when compared to control unvaccinated fish (90). This result led the authors to hypothesize that this vaccine was effective at triggering systemic and local T cell responses. Additionally, an increase of the percentage of proliferating CD8+ cells was detected in the posterior portion of the hindgut, pointing to this part of the intestine as an important site for generating virus-specific CD8+ cytotoxic T cells upon anal vaccination (90).
In some studies different routes of mucosal administration have been compared in order to identify which delivery method reached the higher adaptive immune responses at both local and systemic levels.
In 1986, a study performed in carp showed that a formalin-killed L. anguillarum vaccine administered by anal immunization increased antigen-specific antibodies in serum, and after anal boosting, similar levels of serum antibodies were reached compared with two consecutive intramuscular injections (91). An enhancement of antigen-specific Ig titers in skin mucus was also detected after anal immunization (91). However, no significant antibody titers were elicited in serum after oral immunization, not even when bacteria were administered daily with the food (91). In rainbow trout, protection against L. anguillarum correlated positively with serum antibody levels after, but not prior to, boosting by oral, immersion or injection with a formalin-killed vaccine (92). In the case of African catfish (Clarias gariepinus), the analysis of serum samples after vaccination with a formalin-inactivated L. anguillarum O2 bacterin by four delivery routes showed that fish vaccinated by intraperitoneal injection obtained the highest antibody levels, followed by fish immunized by anal intubation, oral administration and immersion (93). However, the levels of antibodies reached in skin mucus were higher upon anal intubation, followed by immersion, oral intubation, and intraperitoneal injection (93), demonstrating that mucosal vaccines have a superior capacity to induce mucosal responses. In the case of barramundi (Lates calcarifer), fish injected intraperitoneally with an experimental Vibrio harveyi inactivated vaccine displayed significantly higher serum antibody activity than immersed and intubated fish (94). In eel (Anguilla anguilla), the bivalent formalin and heat-inactivated vaccine against two eel-pathogenic serovars of Vibrio vulnificus induced the production of significant antibody levels in plasma as well as in the skin and gut mucus despite the via of administration (oral, anal, immersion, or intraperitoneal vaccination) (95). Interestingly, in all cases, the production of IgM was faster in mucus while antibody titers were higher and lasted longer in plasma (95). In rainbow trout, no significant increase of IgM titers in serum, gill or skin mucus was observed 28 days after immunization of fish with live attenuated F. psychrophilum by anal intubation or immersion, unlike fish vaccinated intraperitoneally (96). However, the levels of secretory IgD and IgT expression were significantly upregulated in gills and intestine of fish immunized by the immersion and anal intubation route, respectively (96). In another study, the oral or anal administration of rainbow trout with a Y. ruckeri O1 bacterin induced protection against ERM, without affecting the levels of Y. ruckeri specific antibodies in plasma. The authors attributed this protection to an immune response mounted locally in the intestine (97). On the contrary, significantly higher antibody levels in serum were detected 45 days post-immunization in mrigal (Cirrhinus mrigala) anally or orally vaccinated against E. tarda in comparison to those detected in control fish or in fish vaccinated by immersion and intraperitoneal injection (98). In skin and gill mucus, significantly higher antibodies levels were obtained in the oral and immersion groups (98). In gut mucus, significantly higher values of antibodies were detected in the immersion group compared to the rest of the treatments (98). However, oral and immersion immunization routes offered better protection of mrigal compared to other antigen delivery routes (98).
No significant differences were observed in serum antibody levels in rainbow trout intraperitoneally, anally, and orally immunized with viral hemorrhagic septicemia virus (VHSV) (99). The vaccine consisted in lyophilized virus surrounded by polyethylene glycol (PEG) and then extruded under low temperature (99). In olive flounder fingerlings, specific anti-VHSV antibody titers were significantly enhanced in serum, and also in the skin and intestinal mucus following vaccination with a poly lactic-co-glycolic acid (PLGA) or chitosan encapsulated formalin-inactivated VHSV vaccine administered together with the feed or by immersion (100, 101). Additionally, a significant upregulation of IgM, IgT, pIgR, MHC I, MHC II transcripts was detected in the kidney, skin, and intestine of vaccinated fish (100, 101).
Bath and oral immunizations of grouper (Epinephelus coioides) larvae with a binary ethylenimine (BEI)-inactivated nervous necrosis virus (NNV) vaccine induced the transcription of IgM, IgT, MHC I, MHC II, and CD8 not only in viscera but also in mucosal tissues at specific time points (102), suggesting the involvement of both local and systemic B and T cells.
There are many mucosally administered vaccines that are only capable of triggering local immune responses and have been shown to be incapable of providing fish with a sufficient level of protection upon pathogen encounter [reviewed in (21)]. Thus, although in many of the studies mentioned through this work the levels of protection conferred by the vaccines have not been studied, it seems clear that those mucosal vaccines that are capable of eliciting strong systemic B and T cell responses are better suited to confer protection, and these are the ones we have focused on in this paper. However, it should be noted, that most of these studies in which adaptive immune responses to vaccines were undertaken, antibody production was measured and very few of them analyzed T cell responses, despite their relevance, especially in the case of antiviral vaccines. Additionally, all determinations of specific antibody titers focused on the identification of IgM specific antibodies, as no studies to date have reported the presence of antigen-specific IgT or IgD antibodies in fish. Therefore, the development of additional immunological tools in different fish species that would allow us to have a wider view of how B and T cell responses are activated in response to vaccine antigens seems essential to establish immunological correlates of protection. Only through a complete understanding of how antibodies and cellular responses correlate to protection, we will be able to rationally design effective mucosal vaccines in the future.
To increase the efficacy of non-optimal mucosal vaccine formulations, two aspects have to be taken into account. The formulation of the antigen has to be optimized to allow it to reach mucosal effector sites in an unaltered way in sufficient amount. For oral vaccines, this implies reaching the more distal gut segments such as hindgut (50) or pyloric caeca (52). However, knowledge on how the antigen is taken up at these mucosal sites and presented to cells of the adaptive system is still scarce. These studies are essential to understand to which cell types the antigen should be directed. For oral vaccines, encapsulation has been the most commonly used method to protect antigens from degradation and increase their uptake in the gut after oral immunization, whereas a fewer number of studies have used alternative strategies such as antigen expression in live feed (58). The other aspect that should be taken into account to increase the efficacy of mucosal vaccines, is the fact that these vaccines should circumvent mucosal tolerance, a mechanism through which the immune response in these mucosal surfaces is tightly regulated to avoid a continuous response to innocuous antigens. To this aim, inclusion of adjuvants may help to increase the immunogenicity of these antigens and bypass mucosal tolerance. However, this implies a greater knowledge of these regulatory systems as well as a search for adjuvants suited for mucosal deliver in fish. For instance, in mammals, the most commonly used mucosal adjuvants are toxin-based adjuvants (e.g., enterotoxigenic E. coli heat-labile toxin and cholera toxin), immunostimulatory adjuvants (e.g., monophosphoryl lipid, CpG, and QS21, a substance extracted from the bark of Quillaja saponaria), particulate adjuvants (e.g., emulsions and highly immunogenic immune stimulating complex) (103), or microbiota-derived components such as flagellin (104). Very few information is available regarding the adjuvant potential of these molecules in fish. The adjuvant potential of the E. coli LT (R192G/L211A) toxoid (dmLT) was first tested in carp in combination with an oral DNA vaccine against SVCV showing no efficacy (105). However, taking into account that a specific adjuvant may not be suitable for one antigen, but could be effective in combination with one of a different nature, Martín-Martín et al. (106) established a simple protocol designed to initially evaluate the effects of potential oral adjuvants for two different pathogens. The results pointed to dmLT as an preferential adjuvant for oral antibacterial vaccines in rainbow trout, since the combination of the toxoid with VHSV only produced minor transcriptional effects, while the effects were much more pronounced when combined with Aeromonas salmonicida (106). The adjuvant effect of Q. saponaria saponin (QSS) has been also tested in fish, particularly on the protection of turbot fry against L. anguillarum (107). The results showed that turbot immersed in seawater containing QSS for 10 min and then vaccinated in a formalin-inactivated L. anguillarum MN suspension for 30 min produced a significant expression of IgM mRNA in skin, spleen, and kidney at 14 days post-immunization in comparison to fish vaccinated without QSS by immersion or intraperitoneal injection (107). Moreover, a higher serum antibody titer was also demonstrated after 14 days in the vaccinated fish although at levels lower than those reached in the intraperitoneally immunized fish (107). Another strategy which has been proposed for aquacultured fish is the use of proteins derived from the host rather than foreign antigens as adjuvants (108). This is the case of sea bass fed with a commercial vaccine against L. anguillarum and Vibrio ordalii (AQUAVAC Vibrio Oral, ISPAH) adjuvanted with a fish-self recombinant cytokine, such as the tumor necrosis factor α (rTNFα). The supplementation of this vaccine with rTNFα significantly enhanced disease resistance against vibriosis, enhancing the infiltration of T cells into the gut epithelium, upregulating the expression of the IgT gene in the hindgut but not raising specific IgM titers in serum (108).
Regarding microbiota-derived components, the immune-stimulating effects of β-glucans on the fish immune system when administered on their own have become evident using different administration routes (109). Recently, immersion vaccination of gilbel carp (Carassius auratus gibelio) with β-glucans and β-propiolactone-inactivated cyprinid herpesvirus 2 protected against a viral challenge more efficiently than fish vaccinated in the absence of β-glucans (110). In this work, the levels of transcription of IL-2 and IFN-γ2 in head kidney and spleen as well as of IgM and IgZ in the spleen were higher when β-glucans were included (110). In rainbow trout, a recombinant flagellin from the salmonid pathogen Y. ruckeri induced a strong antibody response when intraperitoneally injected (111). Thus, it would be interesting to study how fish B and T cells respond to bacterial flagellin when administered as a mucosal adjuvant and whether this protein is able to promote adaptive immune responses in fish as previously observed in mice (104).
It is worthwhile to mention that, in the last years, not only microbiota-derived components but also live microorganisms, designated as immunobiotics, have been shown to modulate the mucosal immune system. Therefore, immunobiotics have been considered as a potential alternative to enhance the response to vaccination (112, 113). For example, the nasal administration of Lactobacillus rhamnosus CRL1505 as an adjuvant improved the humoral and cellular adaptive immune responses induced by influenza virus infection or vaccination in mice (112). Regarding this strategy, few data have been reported in fish. Yeast has been proposed as vehicle for oral antigen delivery due to its immunomodulatory properties and potential to boost local immune responses, acting as an adjuvant (49). Thus, in rainbow trout, the oral administration of Pichia pastoris yeast expressing a green fluorescence protein (GFP) was shown to exert a rapid local innate immune response in the intestine increasing IgT transcription in the midgut at 24 h post-treatment, and a subsequent systemic response, increasing the transcription of IgM and IgT in the spleen after 7 days (49). In this scenario, it is plausible that the use of immunobiotics can be explored in the future as adjuvants for novel fish mucosal vaccine formulations.
Taking into account that pathogens enter the organisms through mucosal surfaces where they initiate their replication and having established that mucosal vaccines have a superior ability to trigger mucosal immunity than systemic vaccines, mucosal vaccines seem as a great alternative to vaccines administered through injection. However, it does seem that these vaccines must be capable of eliciting robust systemic immune effects in order to provide long-lasting memory B and T cell responses. Although in fish, mucosal vaccines also provide numerous advantages from a practical point of view, almost no oral vaccines and very few immersion vaccines are available in the market for aquacultured species, many of them intended as boosters and not as vaccines on their own. The efficacy of these vaccines depends not only on the immunogenicity of the antigen itself (that may be increased by the addition of suited adjuvants) but also on the availability of this antigen for mucosal immune cells. In this sense, in mammals and also in fish, recent strategies to develop effective mucosal vaccines have been aimed at directing the antigens to specific antigen sampling cells within the mucosa (10, 114, 115). However, these strategies require a much better knowledge of how immune responses are organized in mucosal surfaces in fish, as well as a full phenotypical and functional characterization of the cells implicated in this response. Thus, the design of efficient mucosal vaccines for fish still requires investigation of many aspects. In parallel, it is also essential to develop new immunological tools that can help us evaluate the response to these vaccine formulations and establish correlates of protection. Despite all these gaps, it seems obvious that those mucosal vaccines capable of inducing not only local, but also systemic B and T cell responses are better placed to confer high protection levels. Thus, in this review we have highlighted this information, as it could be used as a starting point to continue the optimization towards economically profitable mucosal vaccines.
EM-A collected all the information included in this review and wrote the manuscript with help and contributions from PD-R and CT. All authors contributed to the article and approved the submitted version.
This work was supported by the European Research Council (ERC Consolidator Grant 2016 725061 TEMUBLYM), by the Spanish Ministry of Science, Innovation, and Universities (project AGL2017-85494-C2-1-R) and by the Comunidad de Madrid (grant 2016-T1/BIO-1672). EM-A was recipient of a Juan de la Cierva-Incorporación Postdoctoral Contract (IJC2018-035843-I) funded by the Spanish Ministry of Science, Innovation and Universities.
The authors declare that the research was conducted in the absence of any commercial or financial relationships that could be construed as a potential conflict of interest.
1. Amanna IJ, Slifka MK. Contributions of humoral and cellular immunity to vaccine-induced protection in humans. Virology (2011) 411(2):206–15. doi: 10.1016/j.virol.2010.12.016
2. Kang S-M, Compans RW. Host responses from innate to adaptive immunity after vaccination: molecular and cellular events. Mol Cells (2009) 27(1):5–14. doi: 10.1007/s10059-009-0015-1
3. Takamura S. Niches for the long-term maintenance of tissue-resident memory T cells. Front Immunol (2018) 9:1214. doi: 10.3389/fimmu.2018.01214
4. Zens KD, Chen JK, Farber DL. Vaccine-generated lung tissue-resident memory T cells provide heterosubtypic protection to influenza infection. JCI Insight (2016) 1(10):e85832. doi: 10.1172/jci.insight.85832
5. Zhao J, Zhao J, Mangalam AK, Channappanavar R, Fett C, Meyerholz DK, et al. Airway memory CD4(+) T cells mediate protective immunity against emerging respiratory coronaviruses. Immunity (2016) 44(6):1379–91. doi: 10.1016/j.immuni.2016.05.006
6. Palm AE, Henry C. Remembrance of things past: long-term B cell memory after infection and vaccination. Front Immunol (2019) 10:1787. doi: 10.3389/fimmu.2019.01787
7. Jegaskanda S, Mason RD, Andrews SF, Wheatley AK, Zhang R, Reynoso GV, et al. Intranasal live Influenza vaccine priming elicits localized B cell responses in mediastinal lymph nodes. J Virol (2018) 92(9):e01970-17. doi: 10.1128/jvi.01970-17
8. Chen K, Cerutti A. Vaccination strategies to promote mucosal antibody responses. Immunity (2010) 33(4):479–91. doi: 10.1016/j.immuni.2010.09.013
9. Neutra MR, Kozlowski PA. Mucosal vaccines: the promise and the challenge. Nat Rev Immunol (2006) 6(2):148–58. doi: 10.1038/nri1777
10. Fujkuyama Y, Tokuhara D, Kataoka K, Gilbert RS, McGhee JR, Yuki Y, et al. Novel vaccine development strategies for inducing mucosal immunity. Expert Rev Vaccines (2012) 11(3):367–79. doi: 10.1586/erv.11.196
11. Li M, Wang Y, Sun Y, Cui H, Zhu SJ, Qiu HJ. Mucosal vaccines: strategies and challenges. Immunol Lett (2020) 217:116–25. doi: 10.1016/j.imlet.2019.10.013
12. Lycke N. Recent progress in mucosal vaccine development: potential and limitations. Nat Rev Immunol (2012) 12(8):592–605. doi: 10.1038/nri3251
13. Sztein MB. Cell-mediated immunity and antibody responses elicited by attenuated Salmonella enterica Serovar Typhi strains used as live oral vaccines in humans. Clin Infect Dis (2007) 45 Suppl 1:S15–19. doi: 10.1086/518140
14. Wong P, Pamer EG. CD8 T cell responses to infectious pathogens. Annu Rev Immunol (2003) 21:29–70. doi: 10.1146/annurev.immunol.21.120601.141114
15. Harris JB. Cholera: immunity and prospects in vaccine development. J Infect Dis (2018) 218(suppl_3):S141–s146. doi: 10.1093/infdis/jiy414
16. Hoft DF, Babusis E, Worku S, Spencer CT, Lottenbach K, Truscott SM, et al. Live and inactivated influenza vaccines induce similar humoral responses, but only live vaccines induce diverse T-cell responses in young children. J Infect Dis (2011) 204(6):845–53. doi: 10.1093/infdis/jir436
17. Ward RL. Rotavirus vaccines: how they work or don’t work. Expert Rev Mol Med (2008) 10:e5. doi: 10.1017/s1462399408000574
18. Freuling CM, Hampson K, Selhorst T, Schröder R, Meslin FX, Mettenleiter TC, et al. The elimination of fox rabies from Europe: determinants of success and lessons for the future. Philos Trans R Soc Lond B Biol Sci (2013) 368(1623):20120142. doi: 10.1098/rstb.2012.0142
19. Rossi S, Staubach C, Blome S, Guberti V, Thulke H-H, Vos A, et al. Controlling of CSFV in European wild boar using oral vaccination: a review. Front Microbiol (2015) 6:1141. doi: 10.3389/fmicb.2015.01141
20. Adams A. Progress, challenges and opportunities in fish vaccine development. Fish Shellfish Immunol (2019) 90:210–4. doi: 10.1016/j.fsi.2019.04.066
21. Embregts CW, Forlenza M. Oral vaccination of fish: lessons from humans and veterinary species. Dev Comp Immunol (2016) 64:118–37. doi: 10.1016/j.dci.2016.03.024
22. Plant KP, LaPatra SE. Advances in fish vaccine delivery. Dev Comp Immunol (2011) 35(12):1256–62. doi: 10.1016/j.dci.2011.03.007
23. Magadán S, Jouneau L, Boudinot P, Salinas I. Nasal vaccination drives modifications of nasal and systemic antibody repertoires in rainbow trout. J Immunol (2019) 203(6):1480–92. doi: 10.4049/jimmunol.1900157
24. Salinas I, Zhang YA, Sunyer JO. Mucosal immunoglobulins and B cells of teleost fish. Dev Comp Immunol (2011) 35(12):1346–65. doi: 10.1016/j.dci.2011.11.009
25. Tacchi L, Musharrafieh R, Larragoite ET, Crossey K, Erhardt EB, Martin SAM, et al. Nasal immunity is an ancient arm of the mucosal immune system of vertebrates. Nat Commun (2014) 5:5205. doi: 10.1038/ncomms6205
26. Li J, Barreda DR, Zhang YA, Boshra H, Gelman AE, Lapatra S, et al. B lymphocytes from early vertebrates have potent phagocytic and microbicidal abilities. Nat Immunol (2006) 7(10):1116–24. doi: 10.1038/ni1389
27. Gao J, Ma X, Gu W, Fu M, An J, Xing Y, et al. Novel functions of murine B1 cells: active phagocytic and microbicidal abilities. Eur J Immunol (2012) 42(4):982–92. doi: 10.1002/eji.201141519
28. Cain KD, Jones DR, Raison RL. Antibody-antigen kinetics following immunization of rainbow trout (Oncorhynchus mykiss) with a T-cell dependent antigen. Dev Comp Immunol (2002) 26(2):181–90. doi: 10.1016/s0145-305x(01)00063-5
29. Stavnezer J, Amemiya CT. Evolution of isotype switching. Semin Immunol (2004) 16(4):257–75. doi: 10.1016/j.smim.2004.08.005
30. Macpherson AJ, McCoy KD, Johansen FE, Brandtzaeg P. The immune geography of IgA induction and function. Mucosal Immunol (2008) 1(1):11–22. doi: 10.1038/mi.2007.6
31. Díaz-Rosales P, Muñoz-Atienza E, Tafalla C. Role of teleost B cells in viral immunity. Fish Shellfish Immunol (2019) 86:135–42. doi: 10.1016/j.fsi.2018.11.039
32. Granja AG, Tafalla C. Different IgM(+) B cell subpopulations residing within the peritoneal cavity of vaccinated rainbow trout are differently regulated by BAFF. Fish Shellfish Immunol (2019) 85:9–17. doi: 10.1016/j.fsi.2017.10.003
33. Zhang YA, Salinas I, Li J, Parra D, Bjork S, Xu Z, et al. IgT, a primitive immunoglobulin class specialized in mucosal immunity. Nat Immunol (2010) 11(9):827–35. doi: 10.1038/ni.1913
34. Xu Z, Parra D, Gómez D, Salinas I, Zhang YA, von Gersdorff Jørgensen L, et al. Teleost skin, an ancient mucosal surface that elicits gut-like immune responses. Proc Natl Acad Sci U S A (2013) 110(32):13097–102. doi: 10.1073/pnas.1304319110
35. Xu Z, Takizawa F, Parra D, Gómez D, von Gersdorff Jørgensen L, LaPatra SE, et al. Mucosal immunoglobulins at respiratory surfaces mark an ancient association that predates the emergence of tetrapods. Nat Commun (2016) 7:10728. doi: 10.1038/ncomms10728
36. Castro R, Jouneau L, Pham HP, Bouchez O, Giudicelli V, Lefranc MP, et al. Teleost fish mount complex clonal IgM and IgT responses in spleen upon systemic viral infection. PloS Pathog (2013) 9(1):e1003098. doi: 10.1371/journal.ppat.1003098
37. Piazzon MC, Galindo-Villegas J, Pereiro P, Estensoro I, Calduch-Giner JA, Gómez-Casado E, et al. Differential Modulation of IgT and IgM upon parasitic, bacterial, viral, and dietary challenges in a perciform fish. Front Immunol (2016) 7:637. doi: 10.3389/fimmu.2016.00637
38. Arpin C, de Bouteiller O, Razanajaona D, Fugier-Vivier I, Brière F, Banchereau J, et al. The normal counterpart of IgD myeloma cells in germinal center displays extensively mutated IgVH gene, Cmu-Cdelta switch, and lambda light chain expression. J Exp Med (1998) 187(8):1169–78. doi: 10.1084/jem.187.8.1169
39. Chen K, Xu W, Wilson M, He B, Miller NW, Bengtén E, et al. Immunoglobulin D enhances immune surveillance by activating antimicrobial, proinflammatory and B cell-stimulating programs in basophils. Nat Immunol (2009) 10(8):889–98. doi: 10.1038/ni.1748
40. Edholm ES, Bengtén E, Stafford JL, Sahoo M, Taylor EB, Miller NW, et al. Identification of two IgD+ B cell populations in channel catfish, Ictalurus punctatus. J Immunol (2010) 185(7):4082–94. doi: 10.4049/jimmunol.1000631
41. Castro R, Bromage E, Abós B, Pignatelli J, González Granja A, Luque A, et al. CCR7 is mainly expressed in teleost gills, where it defines an IgD+IgM- B lymphocyte subset. J Immunol (2014) 192(3):1257–66. doi: 10.4049/jimmunol.1302471
42. Perdiguero P, Martín-Martín A, Benedicenti O, Díaz-Rosales P, Morel E, Muñoz-Atienza E, et al. Teleost IgD+IgM– B cells mount clonally expanded and mildly mutated intestinal IgD responses in the absence of lymphoid follicles. Cell Rep (2019) 29(13):4223–4235.e4225. doi: 10.1016/j.celrep.2019.11.101
43. Ramírez-Gómez F, Greene W, Rego K, Hansen JD, Costa G, Kataria P, et al. Discovery and characterization of secretory IgD in rainbow trout: secretory IgD is produced through a novel splicing mechanism. J Immunol (2012) 188(3):1341–9. doi: 10.4049/jimmunol.1101938
44. Choi JH, Wang KW, Zhang D, Zhan X, Wang T, Bu CH, et al. IgD class switching is initiated by microbiota and limited to mucosa-associated lymphoid tissue in mice. Proc Natl Acad Sci U S A (2017) 114(7):E1196–e1204. doi: 10.1073/pnas.1621258114
45. Li AV, Moon JJ, Abraham W, Suh H, Elkhader J, Seidman MA, et al. Generation of effector memory T cell-based mucosal and systemic immunity with pulmonary nanoparticle vaccination. Sci Transl Med (2013) 5(204):204ra130. doi: 10.1126/scitranslmed.3006516
46. Bivas-Benita M, Bar L, Gillard GO, Kaufman DR, Simmons NL, Hovav AH, et al. Efficient generation of mucosal and systemic antigen-specific CD8+ T-cell responses following pulmonary DNA immunization. J Virol (2010) 84(11):5764–74. doi: 10.1128/jvi.02202-09
47. Zheng X, Oduro JD, Boehme JD, Borkner L, Ebensen T, Heise U, et al. Mucosal CD8+ T cell responses induced by an MCMV based vaccine vector confer protection against influenza challenge. PLoS Pathog (2019) 15(9):e1008036. doi: 10.1371/journal.ppat.1008036
48. Nakanishi T, Shibasaki Y, Matsuura Y. T Cells in Fish. Biology (2015) 4(4):640–63. doi: 10.3390/biology4040640
49. Embregts CWE, Reyes-Lopez F, Pall AC, Stratmann A, Tort L, Lorenzen N, et al. Pichia pastoris yeast as a vehicle for oral vaccination of larval and adult teleosts. Fish Shellfish Immunol (2019) 85:52–60. doi: 10.1016/j.fsi.2018.07.033
50. Ballesteros NA, Alonso M, Saint-Jean SR, Pérez-Prieto SI. An oral DNA vaccine against infectious haematopoietic necrosis virus (IHNV) encapsulated in alginate microspheres induces dose-dependent immune responses and significant protection in rainbow trout (Oncorrhynchus mykiss). Fish Shellfish Immunol (2015) 45(2):877–88. doi: 10.1016/j.fsi.2015.05.045
51. Rombout JH, Abelli L, Picchietti S, Scapigliati G, Kiron V. Teleost intestinal immunology. Fish Shellfish Immunol (2011) 31(5):616–26. doi: 10.1016/j.fsi.2010.09.001
52. Ballesteros NA, Castro R, Abós B, Rodríguez Saint-Jean SS, Pérez-Prieto SI, Tafalla C. The pyloric caeca area is a major site for IgM(+) and IgT(+) B cell recruitment in response to oral vaccination in rainbow trout. PLoS One (2013) 8(6):e66118. doi: 10.1371/journal.pone.0066118
53. Ghosh B, Bridle AR, Nowak BF, Cain KD. Assessment of immune response and protection against bacterial coldwater disease induced by a live-attenuated vaccine delivered orally or intraperitoneally to rainbow trout, Oncorhynchus mykiss (Walbaum). Aquaculture (2015) 446:242–9. doi: 10.1016/j.aquaculture.2015.04.035
54. Ismail MS, Siti-Zahrah A, Syafiq MRM, Amal MNA, Firdaus-Nawi M, Zamri-Saad M. Feed-based vaccination regime against streptococcosis in red tilapiaOreochromis niloticus x Oreochromis mossambicus. BMC Vet Res (2016) 12(1):194. doi: 10.1186/s12917-016-0834-1
55. Li LP, Wang R, Liang WW, Huang T, Huang Y, Luo FG, et al. Development of live attenuated Streptococcus agalactiae vaccine for tilapia via continuous passage in vitro. Fish Shellfish Immunol (2015) 45(2):955–63. doi: 10.1016/j.fsi.2015.06.014
56. Tobar I, Arancibia S, Torres C, Vera V, Soto P, Carrasco C, et al. Successive oral immunizations against Piscirickettsia salmonis and infectious salmon anemia virus are required to maintain a long-term protection in farmed salmonids. Front Immunol (2015) 6:244. doi: 10.3389/fimmu.2015.00244
57. Tobar JA, Jerez S, Caruffo M, Bravo C, Contreras F, Bucarey SA, et al. Oral vaccination of Atlantic salmon (Salmo salar) against salmonid rickettsial septicaemia. Vaccine (2011) 29(12):2336–40. doi: 10.1016/j.vaccine.2010.12.107
58. Ahmadivand S, Soltani M, Behdani M, Evensen Ø, Alirahimi E, Hassanzadeh R, et al. Oral DNA vaccines based on CS-TPP nanoparticles and alginate microparticles confer high protection against infectious pancreatic necrosis virus (IPNV) infection in trout. Dev Comp Immunol (2017) 74:178–89. doi: 10.1016/j.dci.2017.05.004
59. Ballesteros NA, Rodriguez Saint-Jean S, Perez-Prieto SI. Food pellets as an effective delivery method for a DNA vaccine against infectious pancreatic necrosis virus in rainbow trout (Oncorhynchus mykiss, Walbaum). Fish Shellfish Immunol (2014) 37(2):220–8. doi: 10.1016/j.fsi.2014.02.003
60. Ballesteros NA, Saint-Jean SSR, Encinas PA, Pérez-Prieto SI, Coll JM. Oral immunization of rainbow trout to infectious pancreatic necrosis virus (IPNV) induces different immune gene expression profiles in head kidney and pyloric ceca. Fish Shellfish Immunol (2012) 33(2):174–85. doi: 10.1016/j.fsi.2012.03.016
61. Chen L, Klaric G, Wadsworth S, Jayasinghe S, Kuo T-Y, Evensen Ø, et al. Augmentation of the antibody response of Atlantic salmon by oral administration of alginate-encapsulated IPNV antigens. PLoS One (2014) 9(10):e109337. doi: 10.1371/journal.pone.0109337
62. de las Heras AI, Rodríguez Saint-Jean S, Pérez-Prieto SI. Immunogenic and protective effects of an oral DNA vaccine against infectious pancreatic necrosis virus in fish. Fish Shellfish Immunol (2010) 28(4):562–70. doi: 10.1016/j.fsi.2009.12.006
63. Zhao J-Z, Xu L-M, Liu M, Cao Y-S, LaPatra SE, Yin J-S, et al. Preliminary study of an oral vaccine against infectious hematopoietic necrosis virus using improved yeast surface display technology. Mol Immunol (2017) 85:196–204. doi: 10.1016/j.molimm.2017.03.001
64. Cui LC, Guan XT, Liu ZM, Tian CY, Xu YG. Recombinant Lactobacillus expressing G protein of spring viremia of carp virus (SVCV) combined with ORF81 protein of koi herpesvirus (KHV): a promising way to induce protective immunity against SVCV and KHV infection in cyprinid fish via oral vaccination. Vaccine (2015) 33(27):3092–9. doi: 10.1016/j.vaccine.2015.05.002
65. Liu Z, Wu J, Ma Y, Hao L, Liang Z, Ma J, et al. Protective immunity against CyHV-3 infection via different prime-boost vaccination regimens using CyHV-3 ORF131-based DNA/protein subunit vaccines in carp Cyprinus carpio var. Jian. Fish Shellfish Immunol (2020) 98:342–53. doi: 10.1016/j.fsi.2020.01.034
66. Ma Y, Liu Z, Hao L, Wu J, Qin B, Liang Z, et al. Oral vaccination using Artemia coated with recombinant Saccharomyces cerevisiae expressing cyprinid herpesvirus-3 envelope antigen induces protective immunity in common carp (Cyprinus carpio var. Jian) larvae. Res Vet Sci (2020) 130:184–92. doi: 10.1016/j.rvsc.2020.03.013
67. Sato A, Okamoto N. Induction of virus-specific cell-mediated cytotoxic responses of isogeneic ginbuna crucian carp, after oral immunization with inactivated virus. Fish Shellfish Immunol (2010) 29(3):414–21. doi: 10.1016/j.fsi.2010.04.017
68. Xue R, Liu L, Cao G, Xu S, Li J, Zou Y, et al. Oral vaccination of BacFish-vp6 against grass carp reovirus evoking antibody response in grass carp. Fish Shellfish Immunol (2013) 34(1):348–55. doi: 10.1016/j.fsi.2012.11.024
69. Bøgwald J, Dalmo RA. Review on immersion vaccines for fish: an update 2019. Microorganisms (2019) 7(12):627. doi: 10.3390/microorganisms7120627
70. Sudheesh PS, Cain KD. Optimization of efficacy of a live attenuated Flavobacterium psychrophilum immersion vaccine. Fish Shellfish Immunol (2016) 56:169–80. doi: 10.1016/j.fsi.2016.07.004
71. Raida MK, Nylén J, Holten-Andersen L, Buchmann K. Association between plasma antibody response and protection in rainbow trout Oncorhynchus mykiss immersion vaccinated against Yersinia ruckeri. PLoS One (2011) 6(6):e18832. doi: 10.1371/journal.pone.0018832
72. Chettri JK, Jaafar RM, Skov J, Kania PW, Dalsgaard I, Buchmann K. Booster immersion vaccination using diluted Yersinia ruckeri bacterin confers protection against ERM in rainbow trout. Aquaculture (2015) 440:1–5. doi: 10.1016/j.aquaculture.2015.01.027
73. Deshmukh S, Kania PW, Chettri JK, Skov J, Bojesen AM, Dalsgaard I, et al. Insight from molecular, pathological, and immunohistochemical studies on cellular and humoral mechanisms responsible for vaccine-induced protection of rainbow trout against Yersinia ruckeri. Clin Vaccine Immunol (2013) 20(10):1623–41. doi: 10.1128/CVI.00404-13
74. Ma J, Bruce TJ, Sudheesh PS, Knupp C, Loch TP, Faisal M, et al. Assessment of cross-protection to heterologous strains of Flavobacterium psychrophilum following vaccination with a live-attenuated coldwater disease immersion vaccine. J Fish Dis (2019) 42(1):75–84. doi: 10.1111/jfd.12902
75. Hoare R, Ngo TPH, Bartie KL, Adams A. Efficacy of a polyvalent immersion vaccine against Flavobacterium psychrophilum and evaluation of immune response to vaccination in rainbow trout fry (Onchorynchus mykiss L.). Vet Res (2017) 48(1):43. doi: 10.1186/s13567-017-0448-z
76. Angelidis P, Karagiannis D, Crump EM. Efficacy of a Listonella anguillarum (syn. Vibrio anguillarum) vaccine for juvenile sea bass Dicentrarchus labrax. Dis Aquat Organ (2006) 71(1):19–24. doi: 10.3354/dao071019
77. Zhang Z, Wu H, Xiao J, Wang Q, Liu Q, Zhang Y. Immune responses evoked by infection with Vibrio anguillarum in zebrafish bath-vaccinated with a live attenuated strain. Vet Immunol Immunopathol (2013) 154(3-4):138–44. doi: 10.1016/j.vetimm.2013.05.012
78. Solbakken MH, Jentoft S, Reitan T, Mikkelsen H, Jakobsen KS, Seppola M. Whole transcriptome analysis of the Atlantic cod vaccine response reveals subtle changes in adaptive immunity. Comp Biochem Phys D (2019) 31:100597. doi: 10.1016/j.cbd.2019.100597
79. Du Y, Tang X, Sheng X, Xing J, Zhan W. The influence of concentration of inactivated Edwardsiella tarda bacterin and immersion time on antigen uptake and expression of immune-related genes in Japanese flounder (Paralichthys olivaceus). Microb Pathog (2017) 103:19–28. doi: 10.1016/j.micpath.2016.12.011
80. Aonullah AA, Nuryati S, Alimuddin, Murtini S. Efficacy of koi herpesvirus DNA vaccine administration by immersion method on Cyprinus carpio field scale culture. Aquac Res (2017) 48(6):2655–62. doi: 10.1111/are.13097
81. Zhang C, Zheng Y-Y, Gong Y-M, Zhao Z, Guo Z-R, Jia Y-J, et al. Evaluation of immune response and protection against spring viremia of carp virus induced by a single-walled carbon nanotubes-based immersion DNA vaccine. Virology (2019) 537:216–25. doi: 10.1016/j.virol.2019.09.002
82. Zhao Z, Zhang C, Jia YJ, Qiu DK, Lin Q, Li NQ, et al. Immersion vaccination of Mandarin fish Siniperca chuatsi against infectious spleen and kidney necrosis virus with a SWCNTs-based subunit vaccine. Fish Shellfish Immunol (2019) 92:133–40. doi: 10.1016/j.fsi.2019.06.001
83. Das PK, Salinas I. Fish nasal immunity: From mucosal vaccines to neuroimmunology. Fish Shellfish Immunol (2020) 104:165–71. doi: 10.1016/j.fsi.2020.05.076
84. LaPatra S, Kao S, Erhardt EB, Salinas I. Evaluation of dual nasal delivery of infectious hematopoietic necrosis virus and enteric red mouth vaccines in rainbow trout (Oncorhynchus mykiss). Vaccine (2015) 33(6):771–6. doi: 10.1016/j.vaccine.2014.12.055
85. Salinas I, LaPatra SE, Erhardt EB. Nasal vaccination of young rainbow trout (Oncorhynchus mykiss) against infectious hematopoietic necrosis and enteric red mouth disease. Dev Comp Immunol (2015) 53(1):105–11. doi: 10.1016/j.dci.2015.05.015
86. Chen L, Evensen Ø, Mutoloki S. IPNV antigen uptake and distribution in Atlantic salmon following oral administration. Viruses (2015) 7(5):2507–17. doi: 10.3390/v7052507
87. Martín-Martín A, Simón R, Abós B, Díaz-Rosales P, Tafalla C. Rainbow trout mount a robust specific immune response upon anal administration of thymus-independent antigens. Dev Comp Immunol (2020) 109:103715. doi: 10.1016/j.dci.2020.103715
88. Cain KD, Jones DR, Raison RL. Characterisation of mucosal and systemic immune responses in rainbow trout (Oncorhynchus mykiss) using surface plasmon resonance. Fish Shellfish Immunol (2000) 10(8):651–66. doi: 10.1006/fsim.2000.0280
89. Joosten PHM, Kruijer WJ, Rombout JHWM. Anal immunisation of carp and rainbow trout with different fractions of a Vibrio anguillarum bacterin. Fish Shellfish Immunol (1996) 6(8):541–51. doi: 10.1006/fsim.1996.0051
90. Tajimi S, Kondo M, Nakanishi T, Nagasawa T, Nakao M, Somamoto T. Generation of virus-specific CD8(+) T cells by vaccination with inactivated virus in the intestine of ginbuna crucian carp. Dev Comp Immunol (2019) 93:37–44. doi: 10.1016/j.dci.2018.12.009
91. Rombout JW, Blok LJ, Lamers CH, Egberts E. Immunization of carp (Cyprinus carpio) with a Vibrio anguillarum bacterin: indications for a common mucosal immune system. Dev Comp Immunol (1986) 10(3):341–51. doi: 10.1016/0145-305x(86)90024-8
92. Palm RC Jr., Landolt ML, Busch RA. Route of vaccine administration: effects on the specific humoral response in rainbow trout Oncorhynchus mykiss. Dis Aquat Organ (1998) 33(3):157–66. doi: 10.3354/dao033157
93. Vervarcke S, Ollevier F, Kinget R, Michoel A. Mucosal response in African catfish after administration of Vibrio anguillarum O2 antigens via different routes. Fish Shellfish Immunol (2005) 18(2):125–33. doi: 10.1016/j.fsi.2004.06.004
94. Crosbie PB, Nowak BF. Immune responses of barramundi, Lates calcarifer (Bloch), after administration of an experimental Vibrio harveyi bacterin by intraperitoneal injection, anal intubation and immersion. J Fish Dis (2004) 27(11):623–32. doi: 10.1111/j.1365-2761.2004.00575.x
95. Esteve-Gassent MD, Fouz B, Amaro C. Efficacy of a bivalent vaccine against eel diseases caused by Vibrio vulnificus after its administration by four different routes. Fish Shellfish Immunol (2004) 16(2):93–105. doi: 10.1016/s1050-4648(03)00036-6
96. Makesh M, Sudheesh PS, Cain KD. Systemic and mucosal immune response of rainbow trout to immunization with an attenuated Flavobacterium psychrophilum vaccine strain by different routes. Fish Shellfish Immunol (2015) 44(1):156–63. doi: 10.1016/j.fsi.2015.02.003
97. Villumsen KR, Neumann L, Ohtani M, Strøm HK, Raida MK. Oral and anal vaccination confers full protection against enteric redmouth disease (ERM) in rainbow trout. PLoS One (2014) 9(4):e93845. doi: 10.1371/journal.pone.0093845
98. Qadiri SSN, Makesh M, Rajendran KV, Rathore G, Purushothaman CS. Specific immune response in mucosal and systemic compartments of Cirrhinus mrigala vaccinated against Edwardsiella tarda: in vivo kinetics using different antigen delivery routes. J World Aquac Soc (2019) 50(4):856–65. doi: 10.1111/jwas.12584
99. Adelmann M, Köllner B, Bergmann SM, Fischer U, Lange B, Weitschies W, et al. Development of an oral vaccine for immunisation of rainbow trout (Oncorhynchus mykiss) against viral haemorrhagic septicaemia. Vaccine (2008) 26(6):837–44. doi: 10.1016/j.vaccine.2007.11.065
100. Kole S, Qadiri SSN, Shin SM, Kim WS, Lee J, Jung SJ. PLGA encapsulated inactivated-viral vaccine: formulation and evaluation of its protective efficacy against viral haemorrhagic septicaemia virus (VHSV) infection in olive flounder (Paralichthys olivaceus) vaccinated by mucosal delivery routes. Vaccine (2019) 37(7):973–83. doi: 10.1016/j.vaccine.2018.12.063
101. Kole S, Qadiri SSN, Shin SM, Kim WS, Lee J, Jung SJ. Nanoencapsulation of inactivated-viral vaccine using chitosan nanoparticles: evaluation of its protective efficacy and immune modulatory effects in olive flounder (Paralichthys olivaceus) against viral haemorrhagic septicaemia virus (VHSV) infection. Fish Shellfish Immunol (2019) 91:136–47. doi: 10.1016/j.fsi.2019.05.017
102. Kai YH, Wu YC, Chi SC. Immune gene expressions in grouper larvae (Epinephelus coioides) induced by bath and oral vaccinations with inactivated betanodavirus. Fish Shellfish Immunol (2014) 40(2):563–9. doi: 10.1016/j.fsi.2014.08.005
103. Savelkoul HF, Ferro VA, Strioga MM, Schijns VE. Choice and design of adjuvants for parenteral and mucosal vaccines. Vaccines (Basel) (2015) 3(1):148–71. doi: 10.3390/vaccines3010148
104. Lee SE, Kim SY, Jeong BC, Kim YR, Bae SJ, Ahn OS, et al. A bacterial flagellin, Vibrio vulnificus FlaB, has a strong mucosal adjuvant activity to induce protective immunity. Infect Immun (2006) 74(1):694–702. doi: 10.1128/iai.74.1.694-702.2006
105. Embregts CWE, Rigaudeau D, Tacchi L, Pijlman GP, Kampers L, Veselý T, et al. Vaccination of carp against SVCV with an oral DNA vaccine or an insect cells-based subunit vaccine. Fish Shellfish Immunol (2019) 85:66–77. doi: 10.1016/j.fsi.2018.03.028
106. Martín-Martín A, Tejedor L, Tafalla C, Díaz-Rosales P. Potential of the Escherichia coli LT(R192G/L211A) toxoid as a mucosal adjuvant for rainbow trout (Oncorhynchus mykiss). Fish Shellfish Immunol (2020) 105:310–8. doi: 10.1016/j.fsi.2020.07.016
107. Wang Y, Wang X, Huang J, Li J. Adjuvant effect of Quillaja saponaria saponin (QSS) on protective efficacy and IgM generation in turbot (Scophthalmus maximus) upon immersion vaccination. Int J Mol Sci (2016) 17(3):325. doi: 10.3390/ijms17030325
108. Galindo-Villegas J, Mulero I, García-Alcazar A, Muñoz I, Peñalver-Mellado M, Streitenberger S, et al. Recombinant TNFα as oral vaccine adjuvant protects European sea bass against vibriosis: insights into the role of the CCL25/CCR9 axis. Fish Shellfish Immunol (2013) 35(4):1260–71. doi: 10.1016/j.fsi.2013.07.046
109. Petit J, Wiegertjes GF. Long-lived effects of administering β-glucans: indications for trained immunity in fish. Dev Comp Immunol (2016) 64:93–102. doi: 10.1016/j.dci.2016.03.003
110. Yan Y, Huo X, Ai T, Su J. β-glucan and anisodamine can enhance the immersion immune efficacy of inactivated cyprinid herpesvirus 2 vaccine in Carassius auratus gibelio. Fish Shellfish Immunol (2020) 98:285–95. doi: 10.1016/j.fsi.2020.01.025
111. Wangkahart E, Secombes CJ, Wang T. Studies on the use of flagellin as an immunostimulant and vaccine adjuvant in fish aquaculture. Front Immunol (2018) 9:3054. doi: 10.3389/fimmu.2018.03054
112. Tonetti FR, Islam MA, Vizoso-Pinto MG, Takahashi H, Kitazawa H, Villena J. Nasal priming with immunobiotic lactobacilli improves the adaptive immune response against influenza virus. Int Immunopharmacol (2020) 78:106115. doi: 10.1016/j.intimp.2019.106115
113. Zelaya H, Álvarez S, Kitazawa H, Villena J. Respiratory antiviral immunity and immunobiotics: beneficial effects on inflammation-coagulation interaction during influenza virus infection. Front Immunol (2016) 7:633. doi: 10.3389/fimmu.2016.00633
114. Fuglem B, Jirillo E, Bjerkås I, Kiyono H, Nochi T, Yuki Y, et al. Antigen-sampling cells in the salmonid intestinal epithelium. Dev Comp Immunol (2010) 34(7):768–74. doi: 10.1016/j.dci.2010.02.007
Keywords: teleost fish, mucosal vaccination, systemic immune responses, B cells, T cells, immunoglobulins
Citation: Muñoz-Atienza E, Díaz-Rosales P and Tafalla C (2021) Systemic and Mucosal B and T Cell Responses Upon Mucosal Vaccination of Teleost Fish. Front. Immunol. 11:622377. doi: 10.3389/fimmu.2020.622377
Received: 28 October 2020; Accepted: 29 December 2020;
Published: 16 February 2021.
Edited by:
Nan Wu, Chinese Academy of Sciences, ChinaReviewed by:
Uwe Fischer, Friedrich-Loeffler-Institute, GermanyCopyright © 2021 Muñoz-Atienza, Díaz-Rosales and Tafalla. This is an open-access article distributed under the terms of the Creative Commons Attribution License (CC BY). The use, distribution or reproduction in other forums is permitted, provided the original author(s) and the copyright owner(s) are credited and that the original publication in this journal is cited, in accordance with accepted academic practice. No use, distribution or reproduction is permitted which does not comply with these terms.
*Correspondence: Carolina Tafalla, tafalla@inia.es
†Present address: Estefanía Muñoz-Atienza, Grupo de Seguridad y Calidad de los Alimentos por Bacterias Lácticas, Bacteriocinas y Probióticos (Grupo SEGABALBP), Sección Departamental de Nutrición y Ciencia de los Alimentos (Nutrición, Bromatología, Higiene y Seguridad Alimentaria), Facultad de Veterinaria, Universidad Complutense de Madrid, Madrid, Spain
Disclaimer: All claims expressed in this article are solely those of the authors and do not necessarily represent those of their affiliated organizations, or those of the publisher, the editors and the reviewers. Any product that may be evaluated in this article or claim that may be made by its manufacturer is not guaranteed or endorsed by the publisher.
Research integrity at Frontiers
Learn more about the work of our research integrity team to safeguard the quality of each article we publish.