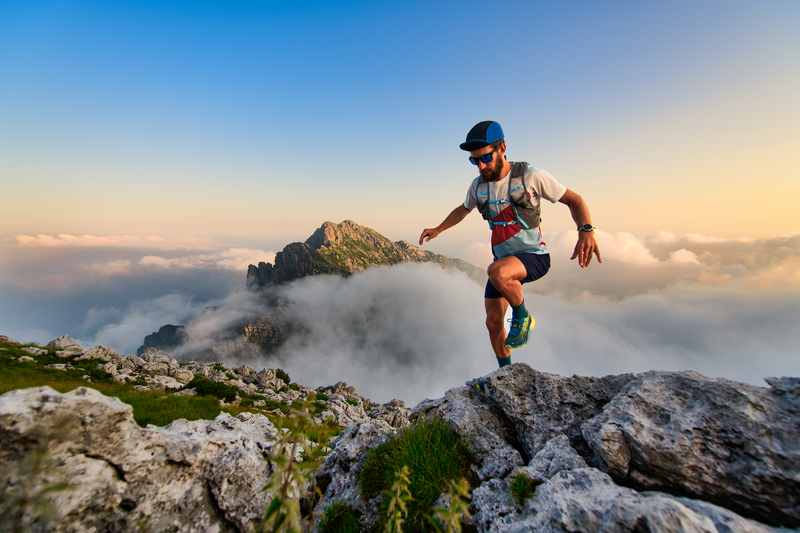
95% of researchers rate our articles as excellent or good
Learn more about the work of our research integrity team to safeguard the quality of each article we publish.
Find out more
REVIEW article
Front. Immunol. , 02 February 2021
Sec. Cancer Immunity and Immunotherapy
Volume 11 - 2020 | https://doi.org/10.3389/fimmu.2020.619236
This article is part of the Research Topic Advances in Human Immune System (HIS) Mouse Models for Studying Human Hematopoiesis and Cancer Immunotherapy View all 19 articles
Immunotherapy has established itself as a promising tool for cancer treatment. There are many challenges that remain including lack of targets and some patients across various cancers who have not shown robust clinical response. One of the major problems that have hindered the progress in the field is the dearth of appropriate mouse models that can reliably recapitulate the complexity of human immune-microenvironment as well as the malignancy itself. Immunodeficient mice reconstituted with human immune cells offer a unique opportunity to comprehensively evaluate immunotherapeutic strategies. These immunosuppressed and genetically modified mice, with some overexpressing human growth factors, have improved human hematopoietic engraftment as well as created more functional immune cell development in primary and secondary lymphoid tissues in these mice. In addition, several new approaches to modify or to add human niche elements to further humanize these immunodeficient mice have allowed a more precise characterization of human hematopoiesis. These important refinements have opened the possibility to evaluate not only human immune responses to different tumor cells but also to investigate how malignant cells interact with their niche and most importantly to test immunotherapies in a more preclinically relevant setting, which can ultimately lead to better success of these drugs in clinical trials.
HSCs are rare (one in 106 bone marrow cells) undifferentiated multipotent stem cells with the ability to perpetuate themselves for indefinite time through self-renewal. These HSCs differentiate to all the downstream mature blood cell lineages that control the homeostasis balance, immune function, and response to infections. Since the pioneering work of Till and McCulloch in the early 1960s, the understanding of HSCs has progressed enormously from observational to functional studies. The regenerative potential of HSCs was further established with the development of various clonal repopulation assays pioneered by Becker et al. (1), McCulloch et al. (2, 3), Moore and Metcalf (4, 5), Griffin and Löwenberg (6) and Dicke et al. (7). Even though our understanding of hematopoiesis largely comes from the mouse system, our ability to characterize human HSCs has improved. Since the discovery of a ligand for L-selectin (namely CD34) by Civin et al. (8), that for decades became the cornerstone, cell surface marker for enriching human HSCs, is still being used alongside newly well-established antigens (such as CD38, CD45RA, CD90, and CD49f) (9–15). However, the concept of SCID-repopulating cell (SRC) assay by Dick and colleagues was the game changing idea that enabled to accurately measure the primitive HSC populations using NOD/SCID mice (16, 17). Altogether, thanks to the use of robust in vivo and in vitro clonal assays, xenotransplantation as well as refined sorting strategies using cell surface antigens, significant insights toward understanding the human hematopoietic hierarchy have been made. Today it is well established that human HSCs are present within the CD34+CD38−CD90+CD45RA−CD49f+ fraction of the hematopoietic compartment, with an SRC frequency of one in 10 cells (15). There is also emerging evidence suggesting the existence of some HSCs within the CD34- faction of the human bone marrow cells, however the frequency of these HSCs is low (18–21).
Gaining knowledge of human physiology and pathophysiology has been often hampered by restricted access to human tissues or limited to performing in vitro assays. Over the last few decades, advances made from inbred wild-type to more state-of-the-art genetically engineered humanized strains have enabled researchers to gain novel insights into the complex biological underpinnings of human hematopoiesis. The development of humanized mouse models started with the identification of the severe combined immunodeficient (Scid; mutation in Prkdcscid) mice that lacked B and T cells (22). These mice were shown to allow human T and B cell reconstitution (23). However, the SCID mouse model has limited usage due to the spontaneous generation of mouse T and B cells during aging (also known as leakiness) and high levels of host NK cell activity, that limits the engraftment of human cells. Further attempts to modify the SCID mouse model were made to increase the human hematopoietic cell engraftment which eventually led to the development of the non-obese diabetic-SCID (NOD-SCID) mice. This hybrid model was developed by backcrossing the SCID mice onto the non-obese diabetic (NOD) mouse background, which resulted in immunological multi-dysfunction, including defective NK cell activity and complement-dependent hemolytic activity (24). The NOD background is supportive of human cells due to a specific mutation in Sirpa that confers high affinity to human CD47, resulting in host macrophage tolerance to human cells (25). These NOD-Scid mice supported high levels of human cell engraftment compared to other non-NOD derived immunodeficient mice. However, the use of this hybrid NOD-SCID mice remained limited due to relatively short life span (median survival = 257 days) as well as the residual activity of NK cell and some of the other innate components (26, 27) of the immune system, thereby impeding the engraftment of the human cells. These mice also have a propensity to developing thymic lymphomas with age, similar to SCID mice.
The next revolution in the development of advanced immunodeficient mice occurred with the backcrossing of NOD-Scid mice with either truncated (NOG) or deleted (NSG) interleukin-2 receptor (IL-2R) common γ chain. Both of these models have deficiency in the IL-2R γ-chain that leads to the multiple defects in the innate as well as the adaptive immune system and prevents NK cell development (28–30). In addition, the lack of the IL-2Rγc activity limits the spontaneous development of thymoma, therefore allowing for long-term human cell engraftment experiments (median survival >89 weeks) (31). Although NOD-Scid, NOG, and NSG mice are the three most commonly used models for xenotransplantation, there are also other strains that have been developed over the years. For example, Rag-deficient mice that lack the expression of functional Rag1 or Rag2 proteins cannot perform somatic recombination of the T cell receptor (TCR) and immunoglobulin (Ig) genes, and the absence of either of these genes results in T and B cell deficiency. Mice that carry either the Rag1 or Rag2 mutations have very similar phenotypes and are also commonly used nowadays (BALB/c-Rag2−/−Il2rγ−/− or BRG and the NOD-Rag1−/−Il2rγ−/− or NRG mice). Despite these improvements, all of the hybrid Il2rγ–/– mice strains still require conditioning with either sublethal radiation or with a myeloablative agent such as bulsulfan prior to cell injection of low number of human cells for optimal engraftment. Further detailed reviews on the historical development of the different mouse models have been extensively covered elsewhere (32–34). Instead, we will critically discuss the recent advancements and pitfalls concerning the development of the different human blood cells in these mice, particularly focusing on their use to investigate cancer immunotherapies (Figure 1). Nevertheless, a brief description of such models described here is summarized in Table 1.
Figure 1 Schematic representation of Immunodeficient mouse models and humanization strategies used to establish preclinical models. Tumor tissues from cancer patients are either injected (for hematological cancers) or implanted subcutaneously or orthotopically into immunodeficient mice with or without humanization. Scaffold implantation approach has also be used for hematological cancers in mice. Immunodeficient mouse models are either used for understanding the normal hematopoiesis or pathophysiology of the cancer tissues and for testing theurapeutic strategies. There are varying degrees of benefits for using different immunodeficient mouse models as depecited in the panel (+/−). IT, Immunotherapy, GVHD, Graft vs host disease, I.V., Intravenous route, BM, bone marrow, HSPC, hematopoietic stem and progenitor cells. Illustration was created with Biorender.com.
Table 1 Summary of the immunodeficient mouse strains developed over the last few decades described in this review.
Despite the successes seen over the last few decades in improving immunodeficient mouse models, significant challenges still remain. For example, NSG mice only allow low human myeloid cell development with high proportion of immature B cells (56, 57) with minimal production of antigen-specific IgG class antibodies (58) and very reduced production of erythrocytes (59). In fact, human cells that have proven most difficult to generate in these immunodeficient mice have been the erythrocytic and megakaryocytic lineages in which their de novo production from human hematopoietic stem and progenitor cells (HSPCs) normally peaks at 5–7 weeks following the xenotransplantation. This skewing in the lineage development of human cells could be multifactorial. The insufficient cross-reactivity of mouse cytokines to human cells may explain, at least in part, the poor development of these cell lineages. Certainly, humanized mice do not allow an efficient production of human megakaryocyte erythroid progenitors (MEPs). However, this deficiency is not corrected in Rag2−/−Il2ry−/− mice expressing human thrombopoietin (TPO). Interestingly, this mouse strain generated increased levels of human cell engraftment, as well as higher multilineage differentiation of HSCs, with an increased ratio of myelomonocytic versus lymphoid cells (38). In addition, injection of plasmid DNA-encoding human erythropoietin (EPO) and IL-3 was found to modestly improve human red blood cell (RBC) reconstitution in NSG mice that were engrafted with CD34+ HSPCs (60). It is worth to note that phagocytosis of human RBCs and platelets by murine macrophages has been shown to play a larger role in the elimination of such human cells. This could explain the absence of these cell types in the peripheral blood of the humanized mice. Interestingly, human late-stage immature erythroid cells (CD235a+CD45− nucleated normoblasts) are normally detected in the mouse BM; however, as these cells reach their maturity to form RBC, they are efficiently eliminated by murine macrophages (59, 61). As previously mentioned, NOD SIRPα is capable of cross-reacting with human CD47, suggesting that the rejection of human RBCs in humanized mice is unlikely to be induced by the inability of human CD47 to interact with the recipient SIRPα. Indeed, it was demonstrated that the rejection rates of human RBCs were significantly higher than CD47 knockout mouse RBCs, indicating that the rejection of human RBCs in immunodeficient mice is mainly induced by CD47-SIRPα independent mechanisms, such as xenoantigens present on human RBCs that can activate host macrophages (62, 63). Interestingly, intravenous injection of liposome-encapsulated clodronate into mice was shown to deplete recipient macrophages, resulting in robust, albeit transient, generation of both human RBCs and platelets (59, 61).
The recent generation of NSGW41 (with partial deficiency in Kit function) mice (Table 1) has definitely helped to improve human erythropoiesis and platelet formation in murine microenvironment (55, 64). Furthermore, human thrombopoiesis was observed in these mice following xenotransplantation, with increased proportions of human mature thrombocytes and erythroblasts in the murine BM. In addition, the morphology and composition of de novo generated human erythroblasts were found to be similar to that observed in the human BM. The most likely mechanism resulting in enhanced BM erythropoiesis in kit mutant mice is the cell intrinsic impairment of erythropoiesis in these mice (65). Interestingly, human erythro- and megakaryopoiesis were also found to be enhanced in an another recently developed immunodeficient mouse model (namely MISTRG mice), following the xenotransplantation of human HSPCs (66). The authors of this work postulated that the expression of human cytokines from the endogenous murine loci (replacing murine encoding cytokines), by providing a more physiologic expression of human cytokines, synergistically promotes a more competitive human hematopoiesis including the efficient development of human RBCs and platelets (66).
In a healthy human adult individual, myeloid cells comprise ~55–70% of all leukocytes both in the peripheral blood and BM. As discussed earlier, the current common immunodeficient mouse models poorly recapitulate the normal myelopoiesis that is observed in human hematopoietic tissues, particularly the human macrophages that are poorly generated in mice. Cytokines play a key role not only in the maintenance of human HSCs but also in their differentiation to mature myeloid progeny. One of the main reasons responsible for poor human myelopoiesis in mice could be the evolutionary divergence between human and mouse cytokines, that are species-specific (i.e., the mouse cytokines do not function in human cells). Early attempts were made to resolve these limitations which included the direct delivery of human cytokines (recombinant cytokines or plasmids encoding for cytokines) into the immunodeficient mice (60, 67, 68). However, these methods are expensive and not suitable for long-term experiments. Further quest for a “better” humanized mouse model led to the development of transgenic mice that are generated by overexpression of human cytokine-encoding genes under the control of a strong constitutive promoter. The NOD-SCID-SGM3 and NSG-SGM3 mouse strains (see Table 1) that overexpress SCF, GM-CSF, and IL-3 under the cytomegalovirus (CMV) promoter have been shown to increase the level of human myeloid cells upon engraftment with human HSPCs (43, 69). The latter mouse strain was also found to facilitate a more rapid reconstitution of T cells, with improved B cell differentiation and increased levels of NK cells as compared to the parental NSG strain. However, the supra-physiological levels of these cytokines in these mice were shown to almost abolish the human platelets that are normally detected in NSG mice at a low level, and more importantly cause exhaustion of the engrafted human HSCs (69). A closely related strain such as NOG-IL3/CSF2 (NOG-EXL) mice that overexpresses human IL-3 and GM-CSF, was also shown to have increased levels of human myeloid cells, in particular basophils, mast cells, and myeloid derived dendritic cells (DCs). However, this mouse strain appears to preserve the human HSPCs (44). It is unclear why this mouse strain does not seem to exhaust the human HSPCs. One of the reasons for such a behavior could be the level of the transgene expression that is driven by different promoters in these models (i.e. SRα instead of the CMV promoter) (44). However, BRG mice with human IL3 and CSF2 knock-in genes (BRG-IL3/CSF2) were found to have no significant increase in human T, B, and NK cells as well as human HSPCs, CD34+CD33+ myeloid progenitors, total CD33+ myeloid cells, CD14+ monocytes/macrophages and CD66+ granulocytes in the bone marrow, blood, and spleen of these mice (36). However, BRG-IL3/CSF2 mice were shown to support the development and replacement of human alveolar marcophages (36). This may suggest that additional factors other than only the lack of cross-reactivity of the cytokines between the species, are also important for generating robust human myelopoiesis in hematopoietic tissues in mice. In another model, a transgenic NOG mouse was generated using human IL6 cDNA under the control of the CMV promoter (NOG-IL6). These mice generated significant levels of CD14+HLA-DRlo/neg human monocytic myeloid derived suppressor cells, following HSPC xenotransplantation. In addition, infiltration of human CD163+ tumor-associated macrophages was evidently detected in a head and neck squamous cell carcinoma engrafted model (45).To specifically increase macrophage development, human macrophage colony stimulating factor (M-CSF; NSG-CSF1) gene was overexpressed in NSG mice (50), which when crossed with NSG-SGM3 mice, led to the generation of NSG-SGM3-CSF1 (QUAD) mice. Interestingly, these mice showed increased human myeloid and mature macrophage development when engrafted with human HSPCs (33). In order to further refine the expression of these critical cytokines, Rongvaux and colleagues used 129×BALB/c-Rag2−/−Il2rγ−/− mice to knock-in human IL3, CSF2, TPO (MITRG) and in addition SIRPA (MISTRG mice; see Table 1) in these mice. These mice allowed a robust development of human myelopoiesis and showed a strong human innate immune response to viral and bacterial infections. The authors also observed a functional human NK cell development that could be attributed to the production of IL-15 by the human monocytes developed in these mice (41). However, these mice eventually developed severe anemia as a result of the high human cell engraftment, which resulted in shorter lifespan of these mice. This was due to the destruction of mouse RBCs as a result of the enhanced human myeloid cell function (41).
Another innate immune cell population that displays suboptimal development in immunodeficient mice are the NK cells. Early effort to boost NK cell formation was demonstrated when BRG mice engrafted with human HSPCs were injected with recombinant IL-15/IL-15-receptor complexes that induced extensive NK cell proliferation and differentiation. This resulted in accumulation of CD16+KIR+ NK cells in these mice (70). This study led to the generation of NOG-IL15 mice (see Table 1). The high levels of circulating IL-15 cytokines in these mice were shown to support the survival of human peripheral blood-derived NK cells (46). Interestingly, human SIRPA and IL15 knock-in RAG (SRG-IL15) mice have more physiological levels of IL-15 but upon poly I:C stimulation these mice can express high levels of IL-15, resulting in an efficient functional human NK cells that can mediate antibody-dependent cellular cytotoxicity (40). Furthermore, compared to the all above innate immune cells, human CD123+ plasmacytoid and myeloid CD11c+ DCs are efficiently developed in most immunodeficient mouse models (71) even in NOD-Scid mice (72) without the requirement of exogenous human cytokines or the use of additional human transgene expression. Nevertheless, administration of recombinant FLT3-L was demonstrated to induce HSPC differentiation towards fully functional human CD141+ and CD1c+ DCs (73). As CD141+ DCs are endowed with the capacity to cross-present viral antigens after their uptake of necrotic virus-infected cells, produce high levels of IL-12, and induce Th1 responses, CD1c+ DCs, on the other hand, play a key role in driving adaptive immune responses to extracellular pathogens, owing to their capacity to promote Th2 and Th17 responses. Both DC fractions can serve important bridges for the elaboration of more complex human immune response studies in these immunodeficient mice.
Recent efforts to further enhance human immune system development and function in humanized mouse models have focused on improving specific innate immune cell lineages. An early study using mice with partial immunodeficiency has shown incomplete human B cell development, with large proportion of engrafted B cells having an immature (CD10+) and B1-type phenotype (CD5+) (74). Subsequent reports that used NSG mice demonstrated improved B cell function including class switching in which the VH‐DH‐JH composition was seen to be similar to the mice engrafted with cord blood cells. In these mice, the production of IgM, IgA, and IgG antibodies were also observed (75, 76). The general view in the field appears to be that B cell maturation in human HSPC engrafted mice increases with time, reaching up to 60% of the B cells having a mature phenotype (CD20+CD10−) after 24 weeks (57). Human de novo B cells isolated from NSG mice have also been shown to undergo somatic hypermutation (77). In fact, several studies have observed the production of antigen-specific human IgM and IgG antibodies in humanized mice in response to infection or immunization with varied degrees of responses (74, 78–80). Some of these humanized mice generated functional neutralizing antibodies in a model of Dengue virus infection (79). Furthermore, these mice have also been used to test the selection process of human autoreactive B cells. Indeed, Lang and colleagues used BRG mice that express a synthetic self-antigen specific constant region of human Igκ and demonstrated that developing human autoreactive (κ+) B cells undergo central tolerance by both receptor editing as well as clonal deletion (81). Other studies have supported this notion of autoreactive human B cell development, therefore confirming the intact central B cell tolerance in immunodeficient mice (82, 83). Therefore, humanized mouse models could also be used to elucidate some aspects of the molecular mechanisms of autoimmunity.
To further enhance class switching and somatic hypermutation, Flavell and colleagues generated immunodeficient Rag2−/−Il2rγ−/− mice in which IL6 was integrated into its orthologous mouse locus (39). These RGSKI-IL6 mice expressing human IL-6 not only allowed the improvement of thymopoiesis and peripheral T cell reconstitution but also showed significant increase in total IgG and antigen-specific IgG levels. The authors of this work also observed higher frequencies of memory and IgG producing B cells. Interestingly, once these mice were challenged with ovalbumin (OVA), the OVA-specific antibodies displayed high frequency of somatic mutations (39). This mouse model then led to the development of the MISTRG6 mice, that enabled further improvement of B cell development. Consequently, MISTRG6 mouse model was used to investigate the entire spectrum of human plasma cell neoplasia (42). Recently, IL-6 transgenic strain was created on the NSG background using human IL6 BAC (NSG-IL6) (33). These NSG-IL6 mice engrafted with human HSPCs displayed increased levels of CD3+ and Th17 T cells compared to NSG mice. In addition, higher plasma levels of IgM and IgG were observed in these mice (33). In summary, human IL6-expressing immunodeficient mice could be a useful tool to evaluate the elicitation of antigen-specific antibody responses.
Human conventional T cells’ response relies on the ability of their TCRs to recognize a specific peptide (and subsequently bind) in the context of an MHC molecule that is solely expressed in the thymus during their initial developmental phase. To examine human immune responses in immunodeficient mice, leukocytes can be engrafted by intravenous or intraperitoneal injection of human peripheral blood mononuclear cells (PBMCs) into mice. This model is less laborious and time consuming compared to the de novo generation of leukocytes through BM xenotransplantation using HSPCs. Although human leukocytes, in particular T cells, can be found circulating in the murine peripheral blood within days following the injection; however, this process can lead to a rapid xenogeneic graft versus host disease (GvHD) (84, 85). One of the major drawbacks of this system includes the limited T cell repertoire and prevalence of uncontrolled B cell activation with the production of human xenoreactive antibodies (86, 87). However, some of these concerns can be circumvented by using new mouse strains that are for the major histocompatibility complex (MHC) class I/II [such as, NSG-B2Mnull and NSG-(KbDb)null(IAnull) mice]. Injection of human PBMCs into these strains enables long-term engraftment of human CD4+ and CD8+ T cells without causing acute GvHD (49, 51). Alternatively, xenogeneic GvHD in immunodeficient mice can be eliminated by a more classical approach that enables the reconstitution of human immune system including T cells through the transplantation of HSPCs. This method enables the generation of human T cells that can be detected in murine thymus at around 12 weeks, depending upon the level of human cell engraftment in the mouse BM. However, this process can be accelerated when human HSPC transplantation is performed in new-born mouse (88–90), that perhaps occurs as a result of a faster seeding of primitive precursors in the mouse thymus. On the other hand, adult NSG-SGM3 mice also have improved T cell development (69). The exact mechanism and the particular changes responsible for this improved lymphopoiesis remain unknown. It is worth to note that this process still requires an extended developmental time, but interestingly different human T cell populations and diverse T cell receptor variable beta chain (TCR Vβ) repertoire can be generated in these mice (89, 91, 92). However, one of the caveats in human T cell development in humanized NSG murine microenvironment is that Th1, Th2, and Th17 cells were found at very low levels (51, 93), although the quantification of these cells was performed following an in vitro stimulation.
Development of human T cells in NOD-Scid and Rag-deficient derived mice undergoes maturation as well as selection via recognition of mouse MHC molecules expressed by thymus mouse epithelial cells and DCs. As these human T cells are educated and restricted solely to murine MHC, therefore they are not suitable for studies investigating T cell responses with human antigen presenting cells (APCs), which express human MHC receptors. To acquire the immune sensitivities with high diversity for human cells, education of T cells must be performed by the human MHC molecules in a murine environment. This major issue was addressed by transplanting human HSPCs isolated from human fetal liver into NOD-Scid mice that were implanted with human liver and thymus (under the mouse kidney capsule) from the same fetus (BLT model). This BLT model demonstrated that human T cells developed in the human thymus (implanted in mouse) were human Human Leukocyte antigen and generated immune response, with development of a mucosal system similar to humans (94–96). Despite these advantages, this model is still time consuming and technically difficult, and mice can still develop xenogeneic GvHD (97). In a recent development, NSG-HLA-A2/HHD mice expressing human HLA class I showed the development of virus-specific HLA-A2-restricted human T cell responses to Dengue virus infection (79). In a subsequent study, this mouse model generated functionally HLA-restricted cytotoxic T cells against Epstein–Barr virus (EBV) virus, therefore suggesting that the recognition and education of human HLA can take place in NSG mouse with a functional thymus. Furthermore, interferon gamma (IFNγ)-producing human T cells against multiple EBV HLA-A2 epitopes were observed showing similar patterns of reactivity to that detected in human infections, including the development of CD8+CD45RO+ memory T cells (51). These two reports described that HLA expressed in murine thymus allows human T cells to be positively selected and in fact these cells can induce HLA-restricted immune responses in murine environment. There are other HLA-transgenic immunodeficient mice that have recently been established, such as NSG mice expressing HLA-A*0201 and A*2402. This study showed that human cytotoxic T cells upon stimulation with Wilms’ tumor 1 (WT1) peptide in in vitro conditions, produced IFNγ and cytotoxic activity against leukemic cells in an antigen-specific and HLA-restricted manner (52). In another report, NSG‐HLA‐A2/DR1 mice were used to study human antiviral adaptive responses during hepatotropic virus infection (98). It was reported that these mice allowed the engraftment of functional human Th1, Th2, and Th17 T cells, and some of these T cells were polyfunctional effector cells (CD62L− CCR7-HLA‐DR+). Interestingly, these cells secreted a variety of cytokines such as IFNγ, tumor necrosis factor alpha (TNFα), IL-4 and IL-17 (98). The HLA‐DR4 single knock‐in mice (DRAG mice; NRG-HLA-A2-DR4; see Table 1) offer further improved polyfunctionality and antigen specificity of effector CD8+ cells over the HLA‐A2 NSG mice (99). Interestingly, these mice were also shown to allow the generation of high levels of CD4+CXCR5+PD1+ T follicular helper cells that were efficiently disseminated into the gut‐associated lymphoid tissues (100). Therefore, HLA class I and II transgenic NSG mouse models may serve as a preclinical tool to develop effective immunotherapy against human malignancies.
Even though the above mentioned immunodeficient mouse models have been useful, they still present various challenges. One of the biggest caveats is cross-species differences that exist between the engrafted human cells and mouse microenvironment (including immune-microenvironment) they reside in, and how this may influence various environmental clues that mediate cell–cell interactions, hematopoietic cell homing, survival, and expansion, among others. Recently, integration of biomaterials with immunodeficient mouse models has enabled researchers to generate a more physiological human microenvironment namely ‘humanized ectopic scaffold niche’, that provides architectural support for cell attachment and subsequent human BM-like tissue development. These humanized scaffold-based approaches can potentially enable the researchers to closely mimic the multicellular aspects of the human bone in a mouse model (101–104). This approach can provide an important tool to potentially generate patient-specific human microenvironment including endothelium in immunodeficient mice that can be used to unravel not only the role of human tumor microenvironments, disease pathology but can potentially be used to gather physiological response to various immunotherapies.
Immunotherapies are considered to be the cornerstone of current therapeutic strategies against cancer, along with surgery, radiation, cytotoxic chemotherapy, and targeted drug therapy (105). Current strategies of immunotherapies are summarized in Figure 2. Intrinsic therapeutic modulation of the adaptive and innate components of the human immune system to enhance recognition that subsequently leads to improved response against tumors is widely adopted strategy in the clinical fight targeting different types of cancers. There are more than 3,000 types of cancer immunotherapies either in development or currently assessed in clinical trials, that include antibody-based immunotherapy, oncolytic virus therapy, cytokine therapy, cellular therapy (CAR-T, CAR-NK, TCR-T and DC-based), cancer vaccines and the combination of immunotherapy that targets multiple immune components (106). Although, these therapies focused on unleashing the immune system are considered a breakthrough; however, durable clinical responses only occur in a subset of patients. Furthermore, the development of novel therapeutics is tightly restricted by the use of human samples before moving to clinical trials, which is generally slow and costly. Therefore, considerable scientific resources are still needed in preclinical research to identify novel and improved approaches to cancer cell‐specific immune response as well as testing of therapeutic strategies. Over the last half century, syngeneic mouse tumor models were the major in vivo systems used for understanding cancer immunology and associated immunotherapies. However, the paradigm shifted with the dramatic advances in our understanding of the interactions between tumor development and the immune microenvironment. Furthermore, immunotherapies such as immunomodulatory agents do not always react with mouse molecules due to cross species barriers. Together all these factors led to the increased humanization of immunodeficient mouse models in which both the human immune cells and human tumors are cohabiting with each other. However, these models are quite complex, and the fact that obtaining human immune cells as well as the malignant cells from the same patient still remains difficult, researchers are currently using HLA mismatched cord blood derived HSPCs or healthy donor derived PBMCs for humanization along with patient derived tumor cells or cancer cell lines (Figure 1). Even with these caveats, the use of these humanized models has emerged as an invaluable tool in providing a system not only to study normal human immune system interactions with the malignant cellular components but have also accelerated the development of the cancer immunotherapies. These preclinical model systems have enabled to better predict the clinical outcomes of immunotherapy regimens before moving to clinical trials.
Figure 2 Current immunotherapeutic strategies in cancers. Cancer immunotherapies target the patients own immune system by restoring and stimulating various immune components that ultimately had the potential to inhibit cancer growth and/or eradicate cancer. The various types of immunotherapies that are currently being used in the clinic or are under development include antibody-based immunotherapy, oncolytic virus therapy, cytokine therapy, cellular therapy, cancer vaccines. These therapies are also being used as combination therapies that target multiple immune components or with other established therapeutic options such as chemotherapy.
To date, one of the most popular immunodeficient mouse models used in the field of cancer biology as well as immuno-oncology is the NSG mice. These models have enabled the generation of patient derived xenografts (PDXs) particularly in hematological malignancies where they have played an instrumental role in the identification and understanding of leukemia initiating cells (101, 107) (Figure 1). Furthermore, these humanized mouse models have enabled personalized tests on the sensitivity and specificity of the tumors to many therapeutic regimens. Among various cellular immunotherapies, anti-CD19 directed CAR-T cells that were shown to eliminate the CD19+ B cell leukemia in NSG PDX model, resulted in prolonged survival of these mice, and this data set the basis for the first clinical trial and later on, approval of the first CAR T cell products by the FDA (108). In a recent development focusing on cellular therapies that could pave the way for off-the shelf cellular therapies, Li and colleagues used NSG mice to demonstrate the feasibility of using the next generation iPSC-derived CAR-NK cells as a treatment option for mesothelin-over expressing ovarian tumors (109). Monoclonal antibody (92R) tested in NSG mice to target T cell acute lymphoblastic leukemia cells strongly inhibited tumor growth via binding to the C-C chemokine receptor type 9 (CCR9) N-terminal domain. This leukemia killing response was observed in a model with compromised NK and complement activities, suggesting that other mechanisms (such as phagocytosis or apoptosis) might also be playing a role in tumor destruction (110).
One of the major drawbacks of these PDXs established in NSG model is the lack of immunosurveillance by the host. Considerable efforts that led to the generation of NSG-PBMC models have been used widely to study single and combination therapies. For example, IgG-based BCMA-T cell bispecific antibody (EM801) targeting B cell maturation antigen (BCMA) induced myeloma cell death by autologous T cells (111). Anti-tumor activity of ICBs (nivolumab, pembrolizumab, atezolizumab, cetuximab, and urelumab) was also observed in the PBMC-humanized mouse models (112–114). Interestingly, the differences in tumor growth were not significantly different between mono- and combination therapies; however, the humanized model was suitable to develop mechanistic studies that supported this combination in the subsequent clinical studies (113). This initial experience represented the first proof of concept report to demonstrate that immunotherapy combination strategies can be modeled in preclinical settings involving humanized mouse models. On the other hand, multiple DC-based vaccine tested in NSG-PBMC mouse model enabled assessment of melanoma antigen recognized by T cells-1 (MART-1)-specific immune responses and suppressive functions on melanoma cells (115). Although the ease of generating these mice allows for their use in tumor-bearing PDXs, challenges arise with regard to the onset of xenogeneic robust GvHD, which occurs concomitant with T cell expansion (116, 117). The limited window of time (approximately 2–3 weeks) for taking observation could enable the investigations into T cell-mediated anti-tumor mechanisms; however, the use of NSG or NOG MHC knockout mice or alternatively xenotransplantation of purified CD8+ T cells may enable long-term immune response studies (49, 118, 119). Using ICB-based (PD-1) immunotherapy in these mouse models, specific CD8+ T cell population was identified that produced anti-tumor effect in an HLA restricted manner without needing to worry about xenogeneic GvHD (120).
HSPC-engrafted human immune system (HIS) models provide powerful tools for long-term studies and are increasingly being used for understanding T cell-mediated immunotherapy mechanisms. The caveat of this HSPC-HIS (NSG and NOG) model is the biased differentiation of HSPCs towards the lymphoid lineage, with the limited development of myeloid cells. However, despite this limitation, HSPC-HIS mice have successfully provided a model system to study T cell-dependent immunotherapy mechanisms (such as blocking PD-1, CTLA-4 alone or in combination with oncolytic viruses) and benefit from long experimental time scales (121–123). In cord blood derived HSPC-generated humanized mouse model, PD-1 blocking (using nivolumab) inhibited the growth of MDAMB-231 tumor cells and CRC172 tumor cells by enhancing anti-tumor T cell response, increasing GrB+ or IFNγ+ CD8+ cells in tumors and reducing frequency of Treg and myeloid cells. However, combination of histone deacetylase (HDAC) inhibitors OKI-179 and nivolumab further inhibited cancer cell growth, therefore indicating that HDAC inhibitors could improve anti-tumor immune responses in cancers (124). In another study, a combination of anti-CD278 mAb and cyclophosphamide controlled the growth of breast cancer in NSG humanized mice. Administration of a neutralizing anti-CD278 mAb reduced human Treg proportions and numbers and improved CD4+ T cell proliferation therefore highlighting the crucial implication of innate immunity in treatment efficacy, opening new perspectives for the treatment of breast cancer (125). In another study, transplantation of allogeneic but HLA partially matched donor HSPCs and tumor cells (non‐small cell lung cancer, sarcoma, bladder cancer, and triple‐negative breast cancer) in NSG mice demonstrated significant tumor growth delay following pembrolizumab (anti‐PD‐1) therapy (121). In a different approach, Haworth and colleagues reconstituted NSG mice with human HSPCs that lead to the generation of matured human CD3+ T cells, which can be isolated, genetically modified and then reinfused into the same mice (89). It is worth to note that no clinical symptoms of GvHD were observed in these mice, and therefore this model might represent a better system for long term evaluation of cellular immunotherapies such as CAR-T cells.
Until recently, the development of more balanced humanization by improving the differentiation and survival of various human immune cell populations has been a major goal; the function of these human immune cells in murine system was overlooked. These issues have led to the development of so called “second generation” immunodeficient models that express human transgenes. These models (e.g. NSG-SGM3, NOG-EXL, NSG HLA-A2 and MISTRG) not only allow more myeloid and NK cell development apart from lymphoid reconstitution, but also enable the function of engrafted human immune cells thus, improving the utility and translatability of these models in immunotherapy related studies (Figure 1). NOG-IL2 transgenic mice were able to overcome the previous problems and recapitulated the clinical responses of patient-derived TILs (126). In a preclinical model using HSPC transplanted humanized BRGS mice, Capasso and colleagues established breast cancer and colon cancer PDXs, and showed strong suppressive function of PD-1 blockade that resulted in tumor growth inhibition with increased CD8 IFNγ+ tumor infiltrating T cells (124). Transgenic mice that express the human HLA-A2 allele, commonly detected in the Caucasian population, were developed to study immunologic interactions between human T cells and tumor cells (51, 127). Studies investigating the antigen-specific HLA-restricted response against Epstein–Barr virus (EBV)-associated tumors, found CD8+ (and CD4+) effector-to-target ratio-dependent cytotoxicity against EBV-infected lymphomas (128). The humanized transgenic NSG-SGM3 mice have shown enhanced human Treg cell differentiation, and these Treg cells were functional and were able to suppress the proliferation of T cells in PDX generated from HSPCs (93). Notably, similar data of T cell suppression by Tregs was reported in PDX models for autoimmune conditions such as aplastic anemia (129).
MISTRG mice that have recently been developed show robust differentiation of human innate as well as adaptive immune cells and have been used to study acute myeloid leukemia and diffuse large B-cell lymphoma (41, 130). Interestingly, human macrophages generated in MISTRG infiltrated a human tumor xenograft in a manner resembling that observed in tumors obtained from patients (41). Therefore, these MISTRG mice recapitulate the role of macrophages in tumor development and fulfil a critical need for models that enable study of the interaction between human tumors and human innate immune microenvironment. The major advantage of these humanized systems is the ability to observe the immune surveillance against human cancer tissues. However, one caveat of these models is that the reconstituted human immune system lacks physiological maturity due to the absence of human thymus tissue. To address these concerns, BLT mouse model developed, harbors an almost intact functional human immune system therefore providing a powerful tool to study cancer immunotherapy (131). In a recent study, implantation of human lung tissue into NSG mice pre-humanized with BM/liver/thymus resulted in robust antigen-specific humoral and T cell responses against cytomegalovirus infection (132). In a different study, Jin and colleagues developed an autologous NSG BLT-based model with a fully competent human immune system. Following the humanization, mice were transplanted with edited fetal HSPCs that recapitulated human B-ALL like disease. This model system was used to test autologous anti-CD19-CAR T cells. This preclinical model, though complex in its generation, was highly adapted to evaluate human CAR T cell efficacy, resistance and toxicity (133). These second-generation humanization strategies provide a promising tool for understanding the tumor immune microenvironment interactions and enable greater translational potential and expanded opportunities for therapeutic evaluation. However, BLT mice do require human fetal tissues which are difficult to source especially if needed in large quantities. However, surplus neonatal tissue can be a good viable alternative that can be used for mouse humanization (134).
Although immunodeficient mouse models along with increased humanization have been instrumental in gaining understanding of the cancer biology and develop various targeted immunotherapies, these models do have limitations that need to be addressed to create more appropriate model system that will fulfil the need of current and future research. There is an urgent need to develop new strategies that will enable us to further the humanization of these mice, particularly focusing on the development of comprehensive and functional human immune system. One of the ways to improve on the current sub-optimal development of human immune cells is to further characterize and identify the cytokines as well as growth factors that do not cross react between mouse and human. It is also important to identify and integrate novel approaches that would enable autologous experiments, where malignant tissues and immune cells from the same individual are used, that would provide more accurate understanding of disease progression, tumor-immune interactions and efficacy of personalized therapeutic options. Improving the humanization of these mice will also enable us to shed more light on the basic tumor immunology, particularly the roles of the immunosuppressive tumor immune-microenvironment and tumor neoantigens that often shape the development of cancer and influence therapeutic efficacy. Altogether, having an appropriate humanized preclinical model will enable speedier assessment of the efficacy and safety of novel therapeutic approaches and will ultimately provide a faster bench to beside transition.
All authors listed have made a substantial, direct, and intellectual contribution to the work and approved it for publication.
This work was supported by the Francis Crick Institute (to DB), which receives its core funding from Cancer Research UK (FC0010045), the UK Medical Research Council (FC0010045), and the Wellcome Trust (FC001045) and by the UK Biotechnology and Biological Sciences Research Council (BB/S017097/1; to FA-A). We would like to thank Blood Cancer UK for their support to SAM.
The authors declare that the research was conducted in the absence of any commercial or financial relationships that could be construed as a potential conflict of interest.
1. Becker AJ, Mc CE, Till JE. Cytological demonstration of the clonal nature of spleen colonies derived from transplanted mouse marrow cells. Nature (1963) 197:452–4. doi: 10.1038/197452a0
2. Buick RN, Till JE, McCulloch EA. Colony assay for proliferative blast cells circulating in myeloblastic leukaemia. Lancet (1977) 1(8016):862–3. doi: 10.1016/s0140-6736(77)92818-5
3. McCulloch EA. Stem cells in normal and leukemic hemopoiesis (Henry Stratton Lecture, 1982). Blood (1983) 62(1):1–13. doi: 10.1182/blood.V62.1.1.1
4. Moore MA, Williams N, Metcalf D. In vitro colony formation by normal and leukemic human hematopoietic cells: characterization of the colony-forming cells. J Natl Cancer Inst (1973) 50(3):603–23. doi: 10.1093/jnci/50.3.603
5. Metcalf D, Moore MA, Warner NL. Colony formation in vitro by myelomonocytic leukemic cells. J Natl Cancer Inst (1969) 43(4):983–1001. doi: 10.1093/jnci/43.4.983
6. Griffin JD, Lowenberg B. Clonogenic cells in acute myeloblastic leukemia. Blood (1986) 68(6):1185–95. doi: 10.1182/blood.V68.6.1185.1185
7. Dicke KA, Spitzer G, Ahearn MJ. Colony formation in vitro by leukaemic cells in acute myelogenous leukaemia with phytohaemagglutinin as stimulating factor. Nature (1976) 259(5539):129–30. doi: 10.1038/259129a0
8. Civin CI, Strauss LC, Brovall C, Fackler MJ, Schwartz JF, Shaper JH. Antigenic analysis of hematopoiesis. III. A hematopoietic progenitor cell surface antigen defined by a monoclonal antibody raised against KG-1a cells. J Immunol (1984) 133(1):157–65.
9. Bhatia M, Wang JC, Kapp U, Bonnet D, Dick JE. Purification of primitive human hematopoietic cells capable of repopulating immune-deficient mice. Proc Natl Acad Sci U S A (1997) 94(10):5320–5. doi: 10.1073/pnas.94.10.5320
10. Cashman JD, Lapidot T, Wang JC, Doedens M, Shultz LD, Lansdorp P, et al. Kinetic evidence of the regeneration of multilineage hematopoiesis from primitive cells in normal human bone marrow transplanted into immunodeficient mice. Blood (1997) 89(12):4307–16. doi: 10.1182/blood.V89.12.4307
11. Hogan CJ, Shpall EJ, Keller G. Differential long-term and multilineage engraftment potential from subfractions of human CD34+ cord blood cells transplanted into NOD/SCID mice. Proc Natl Acad Sci U S A (2002) 99(1):413–8. doi: 10.1073/pnas.012336799
12. Baum CM, Weissman IL, Tsukamoto AS, Buckle AM, Peault B. Isolation of a candidate human hematopoietic stem-cell population. Proc Natl Acad Sci U S A (1992) 89(7):2804–8. doi: 10.1073/pnas.89.7.2804
13. Murray L, Chen B, Galy A, Chen S, Tushinski R, Uchida N, et al. Enrichment of human hematopoietic stem cell activity in the CD34+Thy-1+Lin- subpopulation from mobilized peripheral blood. Blood (1995) 85(2):368–78. doi: 10.1182/blood.V85.2.368.368
14. Majeti R, Park CY, Weissman IL. Identification of a hierarchy of multipotent hematopoietic progenitors in human cord blood. Cell Stem Cell (2007) 1(6):635–45. doi: 10.1016/j.stem.2007.10.001
15. Notta F, Doulatov S, Laurenti E, Poeppl A, Jurisica I, Dick JE. Isolation of single human hematopoietic stem cells capable of long-term multilineage engraftment. Science (2011) 333(6039):218–21. doi: 10.1126/science.1201219
16. Guenechea G, Gan OI, Dorrell C, Dick JE. Distinct classes of human stem cells that differ in proliferative and self-renewal potential. Nat Immunol (2001) 2(1):75–82. doi: 10.1038/83199
17. Larochelle A, Vormoor J, Hanenberg H, Wang JC, Bhatia M, Lapidot T, et al. Identification of primitive human hematopoietic cells capable of repopulating NOD/SCID mouse bone marrow: implications for gene therapy. Nat Med (1996) 2(12):1329–37. doi: 10.1038/nm1296-1329
18. Bhatia M, Bonnet D, Murdoch B, Gan OI, Dick JE. A newly discovered class of human hematopoietic cells with SCID-repopulating activity. Nat Med (1998) 4(9):1038–45. doi: 10.1038/2023
19. Danet GH, Luongo JL, Butler G, Lu MM, Tenner AJ, Simon MC, et al. C1qRp defines a new human stem cell population with hematopoietic and hepatic potential. Proc Natl Acad Sci U S A (2002) 99(16):10441–5. doi: 10.1073/pnas.162104799
20. Wang J, Kimura T, Asada R, Harada S, Yokota S, Kawamoto Y, et al. SCID-repopulating cell activity of human cord blood-derived CD34- cells assured by intra-bone marrow injection. Blood (2003) 101(8):2924–31. doi: 10.1182/blood-2002-09-2782
21. Anjos-Afonso F, Currie E, Palmer HG, Foster KE, Taussig DC, Bonnet D. CD34(-) cells at the apex of the human hematopoietic stem cell hierarchy have distinctive cellular and molecular signatures. Cell Stem Cell (2013) 13(2):161–74. doi: 10.1016/j.stem.2013.05.025
22. Bosma GC, Custer RP, Bosma MJ. A severe combined immunodeficiency mutation in the mouse. Nature (1983) 301(5900):527–30. doi: 10.1038/301527a0
23. Mosier DE, Gulizia RJ, Baird SM, Wilson DB. Transfer of a functional human immune system to mice with severe combined immunodeficiency. Nature (1988) 335(6187):256–9. doi: 10.1038/335256a0
24. Shultz LD, Schweitzer PA, Christianson SW, Gott B, Schweitzer IB, Tennent B, et al. Multiple defects in innate and adaptive immunologic function in NOD/LtSz-scid mice. J Immunol (1995) 154(1):180–91.
25. Takenaka K, Prasolava TK, Wang JC, Mortin-Toth SM, Khalouei S, Gan OI, et al. Polymorphism in Sirpa modulates engraftment of human hematopoietic stem cells. Nat Immunol (2007) 8(12):1313–23. doi: 10.1038/ni1527
26. Pearson T, Markees TG, Serreze DV, Pierce MA, Marron MP, Wicker LS, et al. Genetic disassociation of autoimmunity and resistance to costimulation blockade-induced transplantation tolerance in nonobese diabetic mice. J Immunol (2003) 171(1):185–95. doi: 10.4049/jimmunol.171.1.185
27. Serreze DV, Gaedeke JW, Leiter EH. Hematopoietic stem-cell defects underlying abnormal macrophage development and maturation in NOD/Lt mice: defective regulation of cytokine receptors and protein kinase C. Proc Natl Acad Sci U S A (1993) 90(20):9625–9. doi: 10.1073/pnas.90.20.9625
28. Ishikawa F, Yasukawa M, Lyons B, Yoshida S, Miyamoto T, Yoshimoto G, et al. Development of functional human blood and immune systems in NOD/SCID/IL2 receptor {gamma} chain(null) mice. Blood (2005) 106(5):1565–73. doi: 10.1182/blood-2005-02-0516
29. Ito M, Hiramatsu H, Kobayashi K, Suzue K, Kawahata M, Hioki K, et al. NOD/SCID/gamma(c)(null) mouse: an excellent recipient mouse model for engraftment of human cells. Blood (2002) 100(9):3175–82. doi: 10.1182/blood-2001-12-0207
30. Shultz LD, Lyons BL, Burzenski LM, Gott B, Chen X, Chaleff S, et al. Human lymphoid and myeloid cell development in NOD/LtSz-scid IL2R gamma null mice engrafted with mobilized human hemopoietic stem cells. J Immunol (2005) 174(10):6477–89. doi: 10.4049/jimmunol.174.10.6477
31. Prochazka M, Gaskins HR, Shultz LD, Leiter EH. The nonobese diabetic scid mouse: model for spontaneous thymomagenesis associated with immunodeficiency. Proc Natl Acad Sci U S A (1992) 89(8):3290–4. doi: 10.1073/pnas.89.8.3290
32. Saito Y, Shultz LD, Ishikawa F. Understanding Normal and Malignant Human Hematopoiesis Using Next-Generation Humanized Mice. Trends Immunol (2020) 41(8):706–20. doi: 10.1016/j.it.2020.06.004
33. Shultz LD, Keck J, Burzenski L, Jangalwe S, Vaidya S, Greiner DL, et al. Humanized mouse models of immunological diseases and precision medicine. Mamm Genome (2019) 30(5-6):123–42. doi: 10.1007/s00335-019-09796-2
34. Theocharides AP, Rongvaux A, Fritsch K, Flavell RA, Manz MG. Humanized hemato-lymphoid system mice. Haematologica (2016) 101(1):5–19. doi: 10.3324/haematol.2014.115212
35. Goldman JP, Blundell MP, Lopes L, Kinnon C, Di Santo JP, Thrasher AJ. Enhanced human cell engraftment in mice deficient in RAG2 and the common cytokine receptor gamma chain. Br J Haematol (1998) 103(2):335–42. doi: 10.1046/j.1365-2141.1998.00980.x
36. Willinger T, Rongvaux A, Takizawa H, Yancopoulos GD, Valenzuela DM, Murphy AJ, et al. Human IL-3/GM-CSF knock-in mice support human alveolar macrophage development and human immune responses in the lung. Proc Natl Acad Sci U S A (2011) 108(6):2390–5. doi: 10.1073/pnas.1019682108
37. Strowig T, Rongvaux A, Rathinam C, Takizawa H, Borsotti C, Philbrick W, et al. Transgenic expression of human signal regulatory protein alpha in Rag2-/-gamma(c)-/- mice improves engraftment of human hematopoietic cells in humanized mice. Proc Natl Acad Sci U S A (2011) 108(32):13218–23. doi: 10.1073/pnas.1109769108
38. Rongvaux A, Willinger T, Takizawa H, Rathinam C, Auerbach W, Murphy AJ, et al. Human thrombopoietin knockin mice efficiently support human hematopoiesis in vivo. Proc Natl Acad Sci U S A (2011) 108(6):2378–83. doi: 10.1073/pnas.1019524108
39. Yu H, Borsotti C, Schickel JN, Zhu S, Strowig T, Eynon EE, et al. A novel humanized mouse model with significant improvement of class-switched, antigen-specific antibody production. Blood (2017) 129(8):959–69. doi: 10.1182/blood-2016-04-709584
40. Herndler-Brandstetter D, Shan L, Yao Y, Stecher C, Plajer V, Lietzenmayer M, et al. Humanized mouse model supports development, function, and tissue residency of human natural killer cells. Proc Natl Acad Sci U S A (2017) 114(45):E9626–E34. doi: 10.1073/pnas.1705301114
41. Rongvaux A, Willinger T, Martinek J, Strowig T, Gearty SV, Teichmann LL, et al. Development and function of human innate immune cells in a humanized mouse model. Nat Biotechnol (2014) 32(4):364–72. doi: 10.1038/nbt.2858
42. Das R, Strowig T, Verma R, Koduru S, Hafemann A, Hopf S, et al. Microenvironment-dependent growth of preneoplastic and malignant plasma cells in humanized mice. Nat Med (2016) 22(11):1351–7. doi: 10.1038/nm.4202
43. Nicolini FE, Cashman JD, Hogge DE, Humphries RK, Eaves CJ. NOD/SCID mice engineered to express human IL-3, GM-CSF and Steel factor constitutively mobilize engrafted human progenitors and compromise human stem cell regeneration. Leukemia (2004) 18(2):341–7. doi: 10.1038/sj.leu.2403222
44. Ito R, Takahashi T, Katano I, Kawai K, Kamisako T, Ogura T, et al. Establishment of a human allergy model using human IL-3/GM-CSF-transgenic NOG mice. J Immunol (2013) 191(6):2890–9. doi: 10.4049/jimmunol.1203543
45. Hanazawa A, Ito R, Katano I, Kawai K, Goto M, Suemizu H, et al. Generation of Human Immunosuppressive Myeloid Cell Populations in Human Interleukin-6 Transgenic NOG Mice. Front Immunol (2018) 9:152. doi: 10.3389/fimmu.2018.00152
46. Katano I, Nishime C, Ito R, Kamisako T, Mizusawa T, Ka Y, et al. Long-term maintenance of peripheral blood derived human NK cells in a novel human IL-15- transgenic NOG mouse. Sci Rep (2017) 7(1):17230. doi: 10.1038/s41598-017-17442-7
47. Pearson T, Shultz LD, Miller D, King M, Laning J, Fodor W, et al. Non-obese diabetic-recombination activating gene-1 (NOD-Rag1 null) interleukin (IL)-2 receptor common gamma chain (IL2r gamma null) null mice: a radioresistant model for human lymphohaematopoietic engraftment. Clin Exp Immunol (2008) 154(2):270–84. doi: 10.1111/j.1365-2249.2008.03753.x
48. Covassin L, Laning J, Abdi R, Langevin DL, Phillips NE, Shultz LD, et al. Human peripheral blood CD4 T cell-engrafted non-obese diabetic-scid IL2rgamma(null) H2-Ab1 (tm1Gru) Tg (human leucocyte antigen D-related 4) mice: a mouse model of human allogeneic graft-versus-host disease. Clin Exp Immunol (2011) 166(2):269–80. doi: 10.1111/j.1365-2249.2011.04462.x
49. Brehm MA, Kenney LL, Wiles MV, Low BE, Tisch RM, Burzenski L, et al. Lack of acute xenogeneic graft- versus-host disease, but retention of T-cell function following engraftment of human peripheral blood mononuclear cells in NSG mice deficient in MHC class I and II expression. FASEB J (2019) 33(3):3137–51. doi: 10.1096/fj.201800636R
50. Lee J, Brehm MA, Greiner D, Shultz LD, Kornfeld H. Engrafted human cells generate adaptive immune responses to Mycobacterium bovis BCG infection in humanized mice. BMC Immunol (2013) 14:53. doi: 10.1186/1471-2172-14-53
51. Shultz LD, Saito Y, Najima Y, Tanaka S, Ochi T, Tomizawa M, et al. Generation of functional human T-cell subsets with HLA-restricted immune responses in HLA class I expressing NOD/SCID/IL2r gamma(null) humanized mice. Proc Natl Acad Sci U S A (2010) 107(29):13022–7. doi: 10.1073/pnas.1000475107
52. Najima Y, Tomizawa-Murasawa M, Saito Y, Watanabe T, Ono R, Ochi T, et al. Induction of WT1-specific human CD8+ T cells from human HSCs in HLA class I Tg NOD/SCID/IL2rgKO mice. Blood (2016) 127(6):722–34. doi: 10.1182/blood-2014-10-604777
53. Ono R, Watanabe T, Kawakami E, Iwasaki M, Tomizawa-Murasawa M, Matsuda M, et al. Co-activation of macrophages and T cells contribute to chronic GVHD in human IL-6 transgenic humanised mouse model. EBioMedicine (2019) 41:584–96. doi: 10.1016/j.ebiom.2019.02.001
54. Wunderlich M, Chou FS, Link KA, Mizukawa B, Perry RL, Carroll M, et al. AML xenograft efficiency is significantly improved in NOD/SCID-IL2RG mice constitutively expressing human SCF, GM-CSF and IL-3. Leukemia (2010) 24(10):1785–8. doi: 10.1038/leu.2010.158
55. Cosgun KN, Rahmig S, Mende N, Reinke S, Hauber I, Schafer C, et al. Kit regulates HSC engraftment across the human-mouse species barrier. Cell Stem Cell (2014) 15(2):227–38. doi: 10.1016/j.stem.2014.06.001
56. Chen Q, He F, Kwang J, Chan JK, Chen J. GM-CSF and IL-4 stimulate antibody responses in humanized mice by promoting T, B, and dendritic cell maturation. J Immunol (2012) 189(11):5223–9. doi: 10.4049/jimmunol.1201789
57. Lang J, Kelly M, Freed BM, McCarter MD, Kedl RM, Torres RM, et al. Studies of lymphocyte reconstitution in a humanized mouse model reveal a requirement of T cells for human B cell maturation. J Immunol (2013) 190(5):2090–101. doi: 10.4049/jimmunol.1202810
58. Jangalwe S, Shultz LD, Mathew A, Brehm MA. Improved B cell development in humanized NOD-scid IL2Rgamma(null) mice transgenically expressing human stem cell factor, granulocyte-macrophage colony-stimulating factor and interleukin-3. Immun Inflammation Dis (2016) 4(4):427–40. doi: 10.1002/iid3.124
59. Hu Z, Van Rooijen N, Yang YG. Macrophages prevent human red blood cell reconstitution in immunodeficient mice. Blood (2011) 118(22):5938–46. doi: 10.1182/blood-2010-11-321414
60. Chen Q, Khoury M, Chen J. Expression of human cytokines dramatically improves reconstitution of specific human-blood lineage cells in humanized mice. Proc Natl Acad Sci U S A (2009) 106(51):21783–8. doi: 10.1073/pnas.0912274106
61. Hu Z, Yang YG. Full reconstitution of human platelets in humanized mice after macrophage depletion. Blood (2012) 120(8):1713–6. doi: 10.1182/blood-2012-01-407890
62. Burlak C, Twining LM, Rees MA. Terminal sialic acid residues on human glycophorin A are recognized by porcine kupffer cells. Transplantation (2005) 80(3):344–52. doi: 10.1097/01.tp.0000162974.94890.9f
63. Jin R, Greenwald A, Peterson MD, Waddell TK. Human monocytes recognize porcine endothelium via the interaction of galectin 3 and alpha-GAL. J Immunol (2006) 177(2):1289–95. doi: 10.4049/jimmunol.177.2.1289
64. Rahmig S, Kronstein-Wiedemann R, Fohgrub J, Kronstein N, Nevmerzhitskaya A, Bornhauser M, et al. Improved Human Erythropoiesis and Platelet Formation in Humanized NSGW41 Mice. Stem Cell Rep (2016) 7(4):591–601. doi: 10.1016/j.stemcr.2016.08.005
65. Sharma Y, Astle CM, Harrison DE. Heterozygous kit mutants with little or no apparent anemia exhibit large defects in overall hematopoietic stem cell function. Exp Hematol (2007) 35(2):214–20. doi: 10.1016/j.exphem.2006.10.001
66. Song Y, Rongvaux A, Taylor A, Jiang T, Tebaldi T, Balasubramanian K, et al. A highly efficient and faithful MDS patient-derived xenotransplantation model for pre-clinical studies. Nat Commun (2019) 10:366. doi: 10.1038/s41467-018-08166-x
67. Li Y, Chen Q, Zheng D, Yin L, Chionh YH, Wong LH, et al. Induction of functional human macrophages from bone marrow promonocytes by M-CSF in humanized mice. J Immunol (2013) 191(6):3192–9. doi: 10.4049/jimmunol.1300742
68. Wang JC, Lapidot T, Cashman JD, Doedens M, Addy L, Sutherland DR, et al. High level engraftment of NOD/SCID mice by primitive normal and leukemic hematopoietic cells from patients with chronic myeloid leukemia in chronic phase. Blood (1998) 91(7):2406–14. doi: 10.1182/blood.V91.7.2406
69. Wunderlich M, Chou FS, Sexton C, Presicce P, Chougnet CA, Aliberti J, et al. Improved multilineage human hematopoietic reconstitution and function in NSGS mice. PLoS One (2018) 13(12):e0209034. doi: 10.1371/journal.pone.0209034
70. Huntington ND, Legrand N, Alves NL, Jaron B, Weijer K, Plet A, et al. IL-15 trans-presentation promotes human NK cell development and differentiation in vivo. J Exp Med (2009) 206(1):25–34. doi: 10.1084/jem.20082013
71. Helft J, Anjos-Afonso F, van der Veen AG, Chakravarty P, Bonnet D, Reis e Sousa C. Dendritic Cell Lineage Potential in Human Early Hematopoietic Progenitors. Cell Rep (2017) 20(3):529–37. doi: 10.1016/j.celrep.2017.06.075
72. Palucka AK, Gatlin J, Blanck JP, Melkus MW, Clayton S, Ueno H, et al. Human dendritic cell subsets in NOD/SCID mice engrafted with CD34+ hematopoietic progenitors. Blood (2003) 102(9):3302–10. doi: 10.1182/blood-2003-02-0384
73. Ding Y, Wilkinson A, Idris A, Fancke B, O’Keeffe M, Khalil D, et al. FLT3-ligand treatment of humanized mice results in the generation of large numbers of CD141+ and CD1c+ dendritic cells in vivo. J Immunol (2014) 192(4):1982–9. doi: 10.4049/jimmunol.1302391
74. Novelli EM, Ramirez M, Leung W, Civin CI. Human hematopoietic stem/progenitor cells generate CD5+ B lymphoid cells in NOD/SCID mice. Stem Cells (1999) 17(5):242–52. doi: 10.1002/stem.170242
75. Brainard DM, Seung E, Frahm N, Cariappa A, Bailey CC, Hart WK, et al. Induction of robust cellular and humoral virus-specific adaptive immune responses in human immunodeficiency virus-infected humanized BLT mice. J Virol (2009) 83(14):7305–21. doi: 10.1128/JVI.02207-08
76. Quach TD, Hopkins TJ, Holodick NE, Vuyyuru R, Manser T, Bayer RL, et al. Human B-1 and B-2 B Cells Develop from Lin-CD34+CD38lo Stem Cells. J Immunol (2016) 197(10):3950–8. doi: 10.4049/jimmunol.1600630
77. Chang H, Biswas S, Tallarico AS, Sarkis PT, Geng S, Panditrao MM, et al. Human B-cell ontogeny in humanized NOD/SCID gammac(null) mice generates a diverse yet auto/poly- and HIV-1-reactive antibody repertoire. Genes Immun (2012) 13(5):399–410. doi: 10.1038/gene.2012.16
78. Biswas S, Chang H, Sarkis PT, Fikrig E, Zhu Q, Marasco WA. Humoral immune responses in humanized BLT mice immunized with West Nile virus and HIV-1 envelope proteins are largely mediated via human CD5+ B cells. Immunology (2011) 134(4):419–33. doi: 10.1111/j.1365-2567.2011.03501.x
79. Jaiswal S, Pearson T, Friberg H, Shultz LD, Greiner DL, Rothman AL, et al. Dengue virus infection and virus-specific HLA-A2 restricted immune responses in humanized NOD-scid IL2rgammanull mice. PLoS One (2009) 4(10):e7251. doi: 10.1371/journal.pone.0007251
80. Sango K, Joseph A, Patel M, Osiecki K, Dutta M, Goldstein H. Highly active antiretroviral therapy potently suppresses HIV infection in humanized Rag2-/-gammac-/- mice. AIDS Res Hum Retroviruses (2010) 26(7):735–46. doi: 10.1089/aid.2009.0136
81. Lang J, Ota T, Kelly M, Strauch P, Freed BM, Torres RM, et al. Receptor editing and genetic variability in human autoreactive B cells. J Exp Med (2016) 213(1):93–108. doi: 10.1084/jem.20151039
82. Cantaert T, Schickel JN, Bannock JM, Ng YS, Massad C, Oe T, et al. Activation-Induced Cytidine Deaminase Expression in Human B Cell Precursors Is Essential for Central B Cell Tolerance. Immunity (2015) 43(5):884–95. doi: 10.1016/j.immuni.2015.10.002
83. Ippolito GC, Hoi KH, Reddy ST, Carroll SM, Ge X, Rogosch T, et al. Antibody repertoires in humanized NOD-scid-IL2Rgamma(null) mice and human B cells reveals human-like diversification and tolerance checkpoints in the mouse. PLoS One (2012) 7(4):e35497. doi: 10.1371/journal.pone.0035497
84. King M, Pearson T, Shultz LD, Leif J, Bottino R, Trucco M, et al. A new Hu-PBL model for the study of human islet alloreactivity based on NOD-scid mice bearing a targeted mutation in the IL-2 receptor gamma chain gene. Clin Immunol (2008) 126(3):303–14. doi: 10.1016/j.clim.2007.11.001
85. Pearson T, Greiner DL, Shultz LD. Creation of “humanized” mice to study human immunity. Curr Protoc Immunol (2008). 81:15.21.1–15.21.21 doi: 10.1002/0471142735.im1521s81. Chapter 15:Unit 15 21.
86. Abedi MR, Christensson B, Islam KB, Hammarstrom L, Smith CI. Immunoglobulin production in severe combined immunodeficient (SCID) mice reconstituted with human peripheral blood mononuclear cells. Eur J Immunol (1992) 22(3):823–8. doi: 10.1002/eji.1830220329
87. Garcia S, Dadaglio G, Gougeon ML. Limits of the human-PBL-SCID mice model: severe restriction of the V beta T-cell repertoire of engrafted human T cells. Blood (1997) 89(1):329–36. doi: 10.1182/blood.V89.1.329.329_329_336
88. Brehm MA, Cuthbert A, Yang C, Miller DM, DiIorio P, Laning J, et al. Parameters for establishing humanized mouse models to study human immunity: analysis of human hematopoietic stem cell engraftment in three immunodeficient strains of mice bearing the IL2rgamma(null) mutation.. Clin Immunol (2010) 135(1):84–98. doi: 10.1016/j.clim.2009.12.008
89. Haworth KG, Ironside C, Norgaard ZK, Obenza WM, Adair JE, Kiem HP. In Vivo Murine-Matured Human CD3(+) Cells as a Preclinical Model for T Cell-Based Immunotherapies. Mol Ther Methods Clin Dev (2017) 6:17–30. doi: 10.1016/j.omtm.2017.05.004
90. Traggiai E, Chicha L, Mazzucchelli L, Bronz L, Piffaretti JC, Lanzavecchia A, et al. Development of a human adaptive immune system in cord blood cell-transplanted mice. Science (2010) 304(55667):104–7. doi: 10.1126/science.1093933
91. Brugman MH, Wiekmeijer AS, van Eggermond M, Wolvers-Tettero I, Langerak AW, de Haas EF, et al. Development of a diverse human T-cell repertoire despite stringent restriction of hematopoietic clonality in the thymus. Proc Natl Acad Sci U S A (2015) 112(44):E6020–7. doi: 10.1073/pnas.1519118112
92. Hiramatsu H, Nishikomori R, Heike T, Ito M, Kobayashi K, Katamura K, et al. Complete reconstitution of human lymphocytes from cord blood CD34+ cells using the NOD/SCID/gammacnull mice model. Blood (2003) 102(3):873–80. doi: 10.1182/blood-2002-09-2755
93. Billerbeck E, Barry WT, Mu K, Dorner M, Rice CM, Ploss A. Development of human CD4+FoxP3+ regulatory T cells in human stem cell factor-, granulocyte-macrophage colony-stimulating factor-, and interleukin-3-expressing NOD-SCID IL2Rgamma(null) humanized mice. Blood (2011) 117(11):3076–86. doi: 10.1182/blood-2010-08-301507
94. Denton PW, Nochi T, Lim A, Krisko JF, Martinez-Torres F, Choudhary SK, et al. IL-2 receptor gamma-chain molecule is critical for intestinal T-cell reconstitution in humanized mice. Mucosal Immunol (2012) 5(5):555–66. doi: 10.1038/mi.2012.31
95. Lan P, Tonomura N, Shimizu A, Wang S, Yang YG. Reconstitution of a functional human immune system in immunodeficient mice through combined human fetal thymus/liver and CD34+ cell transplantation. Blood (2006) 108(2):487–92. doi: 10.1182/blood-2005-11-4388
96. Melkus MW, Estes JD, Padgett-Thomas A, Gatlin J, Denton PW, Othieno FA, et al. Humanized mice mount specific adaptive and innate immune responses to EBV and TSST-1. Nat Med (2006) 12(11):1316–22. doi: 10.1038/nm1431
97. Greenblatt MB, Vrbanac V, Tivey T, Tsang K, Tager AM, Aliprantis AO. Graft versus host disease in the bone marrow, liver and thymus humanized mouse model. PLoS One (2012) 7(9):e44664. doi: 10.1371/journal.pone.0044664
98. Billerbeck E, Horwitz JA, Labitt RN, Donovan BM, Vega K, Budell WC, et al. Characterization of human antiviral adaptive immune responses during hepatotropic virus infection in HLA-transgenic human immune system mice. J Immunol (2013) 191(4):1753–64. doi: 10.4049/jimmunol.1201518
99. Majji S, Wijayalath W, Shashikumar S, Pow-Sang L, Villasante E, Brumeanu TD, et al. Differential effect of HLA class-I versus class-II transgenes on human T and B cell reconstitution and function in NRG mice. Sci Rep (2016) 6:28093. doi: 10.1038/srep28093
100. Allam A, Majji S, Peachman K, Jagodzinski L, Kim J, Ratto-Kim S, et al. TFH cells accumulate in mucosal tissues of humanized-DRAG mice and are highly permissive to HIV-1. Sci Rep (2015) 5:10443. doi: 10.1038/srep10443
101. Abarrategi A, Mian SA, Passaro D, Rouault-Pierre K, Grey W, Bonnet D. Modeling the human bone marrow niche in mice: From host bone marrow engraftment to bioengineering approaches. J Exp Med (2018) 215(3):729–43. doi: 10.1084/jem.20172139
102. Abarrategi A, Foster K, Hamilton A, Mian SA, Passaro D, Gribben J, et al. Versatile humanized niche model enables study of normal and malignant human hematopoiesis. J Clin Invest (2017) 127(2):543–8. doi: 10.1172/JCI89364
103. Lee J, Li M, Milwid J, Dunham J, Vinegoni C, Gorbatov R, et al. Implantable microenvironments to attract hematopoietic stem/cancer cells. Proc Natl Acad Sci U S A (2012) 109(48):19638–43. doi: 10.1073/pnas.1208384109
104. Reinisch A, Hernandez DC, Schallmoser K, Majeti R. Generation and use of a humanized bone-marrow-ossicle niche for hematopoietic xenotransplantation into mice. Nat Protoc (2017) 12(10):2169–88. doi: 10.1038/nprot.2017.088
105. Waldman AD, Fritz JM, Lenardo MJ. A guide to cancer immunotherapy: from T cell basic science to clinical practice. Nat Rev Immunol (2020) 20: 651–68 doi: 10.1038/s41577-020-0306-5
106. Yang Y. Cancer immunotherapy: harnessing the immune system to battle cancer. J Clin Invest (2015) 125(9):3335–7. doi: 10.1172/JCI83871
107. Meyer LH, Debatin KM. Diversity of human leukemia xenograft mouse models: implications for disease biology. Cancer Res (2011) 71(23):7141–4. doi: 10.1158/0008-5472.CAN-11-1732
108. Barrett DM, Zhao Y, Liu X, Jiang S, Carpenito C, Kalos M, et al. Treatment of advanced leukemia in mice with mRNA engineered T cells. Hum Gene Ther (2011) 22(12):1575–86. doi: 10.1089/hum.2011.070
109. Li Y, Hermanson DL, Moriarity BS, Kaufman DS. Human iPSC-Derived Natural Killer Cells Engineered with Chimeric Antigen Receptors Enhance Anti-tumor Activity. Cell Stem Cell (2018) 23(2):181–92>e5. doi: 10.1016/j.stem.2018.06.002
110. Somovilla-Crespo B, Martin Monzon MT, Vela M, Corraliza-Gorjon I, Santamaria S, Garcia-Sanz JA, et al. 92R Monoclonal Antibody Inhibits Human CCR9(+) Leukemia Cells Growth in NSG Mice Xenografts. Front Immunol (2018) 9:77. doi: 10.3389/fimmu.2018.00077
111. Seckinger A, Delgado JA, Moser S, Moreno L, Neuber B, Grab A, et al. Target Expression, Generation, Preclinical Activity, and Pharmacokinetics of the BCMA-T Cell Bispecific Antibody EM801 for Multiple Myeloma Treatment. Cancer Cell (2017) 31(3):396–410. doi: 10.1016/j.ccell.2017.02.002
112. Hartkopf AD, Taran FA, Wallwiener M, Walter CB, Kramer B, Grischke EM, et al. PD-1 and PD-L1 Immune Checkpoint Blockade to Treat Breast Cancer. Breast Care (Basel) (2016) 11(6):385–90. doi: 10.1159/000453569
113. Sanmamed MF, Rodriguez I, Schalper KA, Onate C, Azpilikueta A, Rodriguez-Ruiz ME, et al. Nivolumab and Urelumab Enhance Antitumor Activity of Human T Lymphocytes Engrafted in Rag2-/-IL2Rgammanull Immunodeficient Mice. Cancer Res (2015) 75(17):3466–78. doi: 10.1158/0008-5472.CAN-14-3510
114. Lutterbuese R, Raum T, Kischel R, Hoffmann P, Mangold S, Rattel B, et al. T cell-engaging BiTE antibodies specific for EGFR potently eliminate KRAS- and BRAF-mutated colorectal cancer cells. Proc Natl Acad Sci U S A (2010) 107(28):12605–10. doi: 10.1073/pnas.1000976107
115. Hu Z, Xia J, Fan W, Wargo J, Yang YG. Human melanoma immunotherapy using tumor antigen-specific T cells generated in humanized mice. Oncotarget (2016) 7(6):6448–59. doi: 10.18632/oncotarget.7044
116. Ali N, Flutter B, Sanchez Rodriguez R, Sharif-Paghaleh E, Barber LD, Lombardi G, et al. Xenogeneic graft-versus-host-disease in NOD-scid IL-2Rgammanull mice display a T-effector memory phenotype. PLoS One (2012) 7(8):e44219. doi: 10.1371/journal.pone.0044219
117. Alcantar-Orozco EM, Gornall H, Baldan V, Hawkins RE, Gilham DE. Potential limitations of the NSG humanized mouse as a model system to optimize engineered human T cell therapy for cancer. Hum Gene Ther Methods (2013) 24(5):310–20. doi: 10.1089/hgtb.2013.022
118. Inoue M, Senju S, Hirata S, Irie A, Baba H, Nishimura Y. An in vivo model of priming of antigen-specific human CTL by Mo-DC in NOD/Shi-scid IL2rgamma(null) (NOG) mice. Immunol Lett (2009) 126(1-2):67–72. doi: 10.1016/j.imlet.2009.08.001
119. Yaguchi T, Kobayashi A, Inozume T, Morii K, Nagumo H, Nishio H, et al. Human PBMC-transferred murine MHC class I/II-deficient NOG mice enable long-term evaluation of human immune responses. Cell Mol Immunol (2018) 15(11):953–62. doi: 10.1038/cmi.2017.106
120. Ashizawa T, Iizuka A, Nonomura C, Kondou R, Maeda C, Miyata H, et al. Antitumor Effect of Programmed Death-1 (PD-1) Blockade in Humanized the NOG-MHC Double Knockout Mouse. Clin Cancer Res (2017) 23(1):149–58. doi: 10.1158/1078-0432.CCR-16-0122
121. Wang M, Yao LC, Cheng M, Cai D, Martinek J, Pan CX, et al. Humanized mice in studying efficacy and mechanisms of PD-1-targeted cancer immunotherapy. FASEB J (2018) 32(3):1537–49. doi: 10.1096/fj.201700740R
122. Kuryk L, Moller AW, Jaderberg M. Combination of immunogenic oncolytic adenovirus ONCOS-102 with anti-PD-1 pembrolizumab exhibits synergistic antitumor effect in humanized A2058 melanoma huNOG mouse model. Oncoimmunology (2019) 8(2):e1532763. doi: 10.1080/2162402X.2018.1532763
123. Tsoneva D, Minev B, Frentzen A, Zhang Q, Wege AK, Szalay AA. Humanized Mice with Subcutaneous Human Solid Tumors for Immune Response Analysis of Vaccinia Virus-Mediated Oncolysis. Mol Ther Oncolytics (2017) 5:41–61. doi: 10.1016/j.omto.2017.03.001
124. Capasso A, Lang J, Pitts TM, Jordan KR, Lieu CH, Davis SL, et al. Characterization of immune responses to anti-PD-1 mono and combination immunotherapy in hematopoietic humanized mice implanted with tumor xenografts. J Immunother Cancer (2019) 7(1):37. doi: 10.1186/s40425-019-0518-z
125. Burlion A, Ramos RN, Kc P, Sendeyo K, Corneau A, Menetrier-Caux C, et al. A novel combination of chemotherapy and immunotherapy controls tumor growth in mice with a human immune system. Oncoimmunology (2019) 8(7):1596005. doi: 10.1080/2162402X.2019.1596005
126. Jespersen H, Lindberg MF, Donia M, Soderberg EMV, Andersen R, Keller U, et al. Clinical responses to adoptive T-cell transfer can be modeled in an autologous immune-humanized mouse model. Nat Commun (2017) 8(1):707. doi: 10.1038/s41467-017-00786-z
127. Strowig T, Gurer C, Ploss A, Liu YF, Arrey F, Sashihara J, et al. Priming of protective T cell responses against virus-induced tumors in mice with human immune system components. J Exp Med (2009) 206(6):1423–34. doi: 10.1084/jem.20081720
128. Ruhl J, Citterio C, Engelmann C, Haigh T, Dzionek A, Dreyer J, et al. Heterologous prime-boost vaccination protects against EBV antigen-expressing lymphomas. J Clin Invest (2019) 129(5):2071–87. doi: 10.1172/JCI125364
129. Lim SP, Costantini B, Mian SA, Perez Abellan P, Gandhi S, Martinez Llordella M, et al. Treg sensitivity to FasL and relative IL-2 deprivation drive idiopathic aplastic anemia immune dysfunction. Blood (2020) 136(7):885–97. doi: 10.1182/blood.2019001347
130. Stelling A, Hashwah H, Bertram K, Manz MG, Tzankov A, Muller A. The tumor suppressive TGF-beta/SMAD1/S1PR2 signaling axis is recurrently inactivated in diffuse large B-cell lymphoma. Blood (2018) 131(20):2235–46. doi: 10.1182/blood-2017-10-810630
131. Smith DJ, Lin LJ, Moon H, Pham AT, Wang X, Liu S, et al. Propagating Humanized BLT Mice for the Study of Human Immunology and Immunotherapy. Stem Cells Dev (2016) 25(24):1863–73. doi: 10.1089/scd.2016.0193
132. Wahl A, De C, Abad Fernandez M, Lenarcic EM, Xu Y, Cockrell AS, et al. Precision mouse models with expanded tropism for human pathogens. Nat Biotechnol (2019) 37(10):1163–73. doi: 10.1038/s41587-019-0225-9
133. Jin CH, Xia J, Rafiq S, Huang X, Hu Z, Zhou X, et al. Modeling anti-CD19 CAR T cell therapy in humanized mice with human immunity and autologous leukemia. EBioMedicine (2019) 39:173–81. doi: 10.1016/j.ebiom.2018.12.013
Keywords: immunodeficient mice models, immunotherapy, human hematopoiesis, xenotransplantation models, immune reconstitution
Citation: Mian SA, Anjos-Afonso F and Bonnet D (2021) Advances in Human Immune System Mouse Models for Studying Human Hematopoiesis and Cancer Immunotherapy. Front. Immunol. 11:619236. doi: 10.3389/fimmu.2020.619236
Received: 19 October 2020; Accepted: 18 December 2020;
Published: 02 February 2021.
Edited by:
Tim Willinger, Karolinska Institutet, SwedenReviewed by:
Anthony Rongvaux, Fred Hutchinson Cancer Research Center, United StatesCopyright © 2021 Mian, Anjos-Afonso and Bonnet. This is an open-access article distributed under the terms of the Creative Commons Attribution License (CC BY). The use, distribution or reproduction in other forums is permitted, provided the original author(s) and the copyright owner(s) are credited and that the original publication in this journal is cited, in accordance with accepted academic practice. No use, distribution or reproduction is permitted which does not comply with these terms.
*Correspondence: Dominique Bonnet, ZG9taW5pcXVlLmJvbm5ldEBjcmljay5hYy51aw==
†These authors have contributed equally to this work
Disclaimer: All claims expressed in this article are solely those of the authors and do not necessarily represent those of their affiliated organizations, or those of the publisher, the editors and the reviewers. Any product that may be evaluated in this article or claim that may be made by its manufacturer is not guaranteed or endorsed by the publisher.
Research integrity at Frontiers
Learn more about the work of our research integrity team to safeguard the quality of each article we publish.