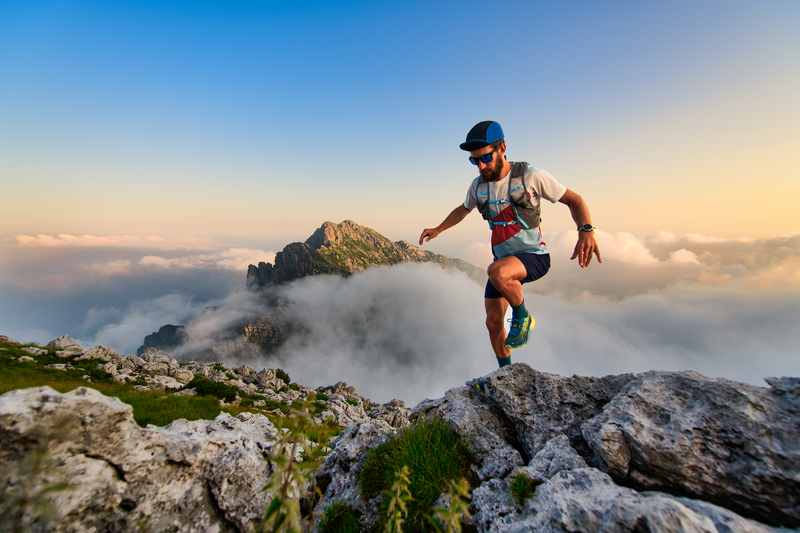
95% of researchers rate our articles as excellent or good
Learn more about the work of our research integrity team to safeguard the quality of each article we publish.
Find out more
MINI REVIEW article
Front. Immunol. , 14 December 2020
Sec. Alloimmunity and Transplantation
Volume 11 - 2020 | https://doi.org/10.3389/fimmu.2020.614578
This article is part of the Research Topic Novel Therapies for Tolerance Induction in Solid Organ and Bone Marrow Transplantation View all 14 articles
Application of cell-based immunotherapy in organ transplantation to minimize the burden of immunosuppressive medication and promote allograft tolerance has expanded significantly over the past decade. Adoptively transferred regulatory immune cells prolong allograft survival and transplant tolerance in pre-clinical models. Many cell products are currently under investigation in early phase human clinical trials designed to assess feasibility and safety. Despite rapid advances in manufacturing practices, defining the appropriate protocol that will optimize in vivo conditions for tolerance induction remains a major challenge and depends heavily on understanding the fate, biodistribution, functional stability and longevity of the cell product after administration. This review focuses on in vivo detection and monitoring of various regulatory immune cell types administered for allograft tolerance induction in both pre-clinical animal models and early human clinical trials. We discuss the current status of various non-invasive methods for tracking regulatory cell products in the context of organ transplantation and implications for enhanced understanding of the therapeutic potential of cell-based therapy in the broad context of control of immune-mediated inflammatory disorders.
Cell-based therapy using naturally occurring or genetically modified immune cells, having now been successfully translated to the clinic for cancer treatment, is undergoing clinical development to promote tolerance and prolong graft survival after solid organ transplantation. Cell products under active investigation for clinical use in kidney or liver transplantation include donor-antigen alloreactive regulatory T cells (darTreg) and polyclonally expanded Tregs (pTreg), regulatory macrophages (Mreg), regulatory dendritic cells (DCreg), and mesenchymal stromal cells (MSCs). Findings from the recent ONE Study, the largest multi-center consortium to date assessing adoptive cell therapy in kidney transplant patients, have confirmed the safety of infusing various regulatory immune cells, paving the way for further development (1). The main challenge in clinical testing of regulatory cell therapy, however, is that the in vivo fate and localization of the cell product remains largely unknown which leads to major gaps in understanding of tolerance induction mechanisms and hinders cell therapy protocol design. Non-invasive, accurate, and durable techniques to monitor exogenous cell products after infusion in both pre-clinical and clinical human studies are critical in addressing 1) variability in clinical outcomes, 2) potential cell toxicity and adverse side effects of infusion, 3) anatomic localization and 4) duration and magnitude of desired tolerogenic activity (2).
Historically, cellular staining and subsequent flow cytometry has been a reliable approach to detecting adoptively transferred cells (3–5) however, more advanced approaches to longitudinal in vivo cell monitoring using whole-body imaging with novel reporter systems, initially developed for cancer immunotherapy, are increasingly being incorporated into both pre-clinical and clinical transplant studies. This review will discuss current techniques used to track and monitor the major regulatory immune cells under clinical investigation for tolerance induction (Table 1) and how understanding the in vivo fate of these cell products has helped advance cell-based therapy in organ transplantation.
Table 1 Methods used to track and monitor regulatory immune cells adoptively transferred for transplant indications.
Naturally occurring Tregs are a rare, specialized subset of thymic-derived CD4+CD25+ T cells characterized by high expression of the transcription factor forkhead box P3 (Foxp3). In addition to these thymic Tregs (tTregs), naïve Foxp3-CD4+ T cells can differentiate in the periphery to become Foxp3+ cells, that are known as induced Tregs (iTregs) or peripheral Tregs. Distinctions between tTregs and iTregs have been reviewed recently (27). T cell receptors (TCRs) that recognize antigens to which an organism is chronically exposed promote the generation of iTregs (28, 29).
Tregs have been implicated extensively in tolerance induction and maintenance pathways. Their potential to regulate allograft rejection after transplantation is the most extensively evaluated of the regulatory cell types under current investigation. Given their paucity in the peripheral circulation in the healthy steady state, one salient issue in using Tregs to promote tolerance induction is whether these cells can persist or self-expand in host peripheral blood and tissue to exert a sustained therapeutic effect after administration. Localization and physical distribution of Tregs within allograft tissue, in particular, have been associated with enhanced immunomodulatory function in vivo, implicating the importance of cell homing in adoptive Treg cell therapy for transplant tolerance (30–32). As such, methodologies to track the fate of infused Tregs are critical and have been incorporated into both pre-clinical and early clinical studies (Table 2).
Early studies investigating ex vivo-expanded polyclonal Tregs adoptively transferred to skin- or pancreatic islet-engrafted mice relied on direct labeling of the ex vivo-expanded CD4+CD25+Foxp3+ cells with intracellular carboxyfluorescein succinimidyl ester (CFSE) dye or on Treg generated from green fluorescent protein (GFP) transgenic donors to study tissue homing and survival of these cells after intravenous administration (6, 7). Flow cytometric analysis detected labeled autologous (6) or darTregs (7) in peripheral blood, spleen, draining lymph nodes (LN) and allograft tissue, up to 60 to 98 days post-infusion demonstrating the persistence and trafficking of adoptively transferred autologous Tregs to secondary lymphoid organs. These studies also determined the cell surface molecules integral to Treg migration. E and P selectin ligands were found to be important in Treg homing to the graft, while chemokine receptors CCR7, CCR2, and CCR5 were required for their migration to secondary lymphoid tissue (8).
More recent studies in transplanted rodents have shifted towards non-invasive whole-body in vivo cell tracking and imaging of adoptively transferred Tregs. Tregs isolated and expanded ex vivo from luciferase transgenic rats were adoptively transferred into major histocompatibility complex (MHC)-mismatched vascularized composite allotransplant rat recipients and visually tracked using bioluminescence imaging (BLI) longitudinally. In contrast to the limited detection of labeled Tregs in cross-sectional samples of earlier studies, real-time in vivo imaging allowed Cheng et. al to identify migratory patterns of infused Tregs first to draining LNs and then to grafted tissue over a prolonged period of 42 weeks (9). A novel method using superparamagnetic nano-sized iron-oxide particle, IOPC-NH2, to label transferred T cells and magnetic resonance imaging (MRI) was developed by Liu et. al and successfully demonstrated localized infiltration of IOPC-NH2-labeled autologous T cells into allograft tissue within 24 h in a rat heart-lung transplant model (10). Radiolabeling of ex vivo-expanded Tregs with technetium-99m pertechnetate (99mTcO4-) was performed both directly and indirectly via retroviral transduction with a construct expressing the hNIS glycoprotein ion channel gene (11). These studies localized adoptively transferred labeled Tregs in spleen, liver, lungs, and the allograft after administration and skin transplantation in mice with the approach allowing longitudinal detection of transferred Tregs in vivo over time.
Tregs have been well-characterized in nonhuman primates (NHP) (20, 21, 33–35).The in vivo persistence and homing of adoptively transferred pTregs to secondary lymphoid organs demonstrated in rodent models have been corroborated by several NHP studies evaluating the survival, migration, and function of exogenous Tregs after administration. In vivo detection of ex vivo-expanded autologous or allogeneic Tregs infused systemically into non-transplanted cynomolgus or rhesus macaques was accomplished through direct CSFE or violet proliferation dye 450 (VPD450)-labeling and subsequent flow cytometric analysis of the labeled cells in peripheral blood, mesenteric and inguinal LNs, and spleen at various timepoints post-infusion (20, 21). Pharmacokinetic analysis of CSFE-labeled autologous Tregs detected an initial rapid phase of elimination from the peripheral blood between day 0 and day 3 post-infusion after which these transferred cells persisted at low levels in the blood up to 3 weeks (21). Persistence of these cells in secondary lymphoid organs was not as durable. Labeled autologous Tregs were detected in inguinal and mesenteric LNs harvested at days 1 to 2 post-infusion, but lost to detection by day 6 (21). Administration of concurrent immunosuppression (IS) therapy substantially increased survival of transferred autologous Tregs in peripheral blood and LNs. Labeled autologous Tregs persisted longer in peripheral blood and LNs in monkeys given rapamycin alone or with concurrent IL-2 and were detected in these compartments in greater numbers when compared to non-immunosuppressed conditions 50 to 84 days post-infusion (22, 23). These studies highlight the wide variability in survival of infused Tregs under numerous different conditions, including the presence and type of IS, as well as cell production techniques, particularly cryopreservation.
Studies in splenectomized, kidney-transplanted NHP treated early post-transplant with cyclophosphamide and then infused with ex vivo-expanded autologous Tregs support their efficacy in prolonging allograft survival and function. In addition, multiple Treg infusions in NHP pretreated with anti-thymocyte globulin (ATG) and post-operative rapamycin prolonged renal allograft survival (36). In contrast, Ezzelarab et. al failed to demonstrate enhanced heart allograft survival after adoptive transfer of autologous pTreg to ATG-treated heart allograft recipients, possibly reflecting, in part, reduced survival capacity of the pTreg product in vivo (37). Overall, in vivo detection of the transferred regulatory cells in the majority of these studies was limited, as they focused primarily on allograft survival outcomes.
While the majority of pre-clinical studies investigating the efficacy of Treg cell therapy have focused on polyclonal autologous and non-autologous Tregs, several groups have evaluated the potential of using chimeric antigen receptor (CAR) modified Tregs as a more potent and targeted cellular method of tolerance induction after transplantation. Investigators have demonstrated that adoptive transfer of genetically engineered donor HLA-specific CAR Tregs successfully prevents the rejection of transplanted allogeneic cells and graft tissue in humanized mouse models (12, 13, 38, 39). In vivo BLI utilizing the luciferase-GFP reporter system showed rapid and specific trafficking of adoptively transferred HLA-A2-specific CAR Tregs (12) or mAb-directed CAR Tregs targeted to H-2Dd (13) to transplanted skin or pancreatic islet allografts respectively, persisting up to 21 days after transfer. Additionally, both studies demonstrated that, compared to their polyclonal counterparts, CAR Tregs achieved a more targeted localization and longer persistence in allograft tissue.
In humans, early phase clinical testing of adoptively transferred autologous Tregs in transplant patients is well underway. Deuterium-labeled autologous pTregs were infused and tracked in the peripheral blood of 3 kidney transplant recipients on maintenance IS regimen of tacrolimus, mycophenolate mofetil ± prednisone with subclinical inflammation on 6-month surveillance biopsy (24). CD4+CD127loCD25+ Tregs were purified via FACS from peripheral blood and single cell suspensions from kidney biopsies. DNA was then extracted from all purified cells and subjected to gas chromatography and mass spectrometry (GC-MS) analysis to measure deuterium enrichment in circulating Tregs. Infused Tregs peaked within 7 days of infusion and were detected by deuterium signals at 30 days. Deuterium-labeled cells fell to the limit of detection within 3 months of infusion (24). In this study, infused Tregs demonstrated patterns of persistence and stability comparable to those observed in prior corresponding immunosuppressed NHP models and non-immunosuppressed type 1 diabetes mellitus patients receiving autologous pTreg therapy (40). Anatomic biodistribution of clinical grade Tregs after therapeutic infusion was ascertained in a non-transplant autoimmune hepatitis (AIH) clinical pilot study by radiolabeling of good manufacturing practice (GMP)-grade Tregs with 111Indium tropolonate (111In) (41). Serial gamma camera and SPECT-CT imaging taken at serial timepoints after infusion tracked the presence of transferred indium-labeled Tregs. 22% to 44% of infused Tregs migrated to the liver, spleen and bone marrow of 4 AIH patients for up to 72 h without any off-target organ localization (41). This provides an additional effective cell tracking method that can be implemented in current and future transplant Treg therapy human clinical studies to assess spatial distribution of infused cell therapy non-invasively in real-time.
The myeloid cell lineage includes multiple regulatory immune cell subsets under active investigation to induce and maintain transplant tolerance in solid organ transplantation, including DCregs, Mregs, and myeloid-derived suppressor cells (MDSCs) (42) (Table 3).
Table 3 Observations of adoptively transferred regulatory myeloid cell survival and migration in various species.
MDSCs comprise a heterogeneous population of immature myeloid progenitor cells that have been associated with modulation of T cell differentiation. There is evidence from pre-clinical rodent models that MDSCs may play a role in promotion of transplant tolerance by inducing Treg and inhibiting alloreactive T cell proliferation in an inducible nitric oxide synthase (iNOS)-dependent manner (43, 44) however, adoptive transfer of ex vivo-generated MSDCs has not been found in pre-clinical animal studies to improve allograft survival (45) and, as such, has not reached clinical testing in humans to date (46). Tracking of infused MDSCs has thus far been restricted to mouse cancer models with one study using a 64Cu-labeled CD11b-specific mAb and PET scanning (47).
Mregs, characterized by a CD14-CD63+HLA-DR+ phenotype and IL-10 production, have been demonstrated to suppress T cell proliferation in vitro (48). In a heterotopic heart transplant mouse model, administration of donor-specific Mregs significantly prolonged allograft survival in an iNOS-dependent manner (14). Mregs were tracked in vivo using donor-discriminatory Mreg staining and flow cytometry analysis of cells from recipient blood, spleen, liver, LN, BM, and lung suspensions at serial timepoints post-infusion. 24 h after administration, Mregs were readily detected in the blood, spleen, liver, and lung but not in LN or BM. Persistence of infused Mregs decreased in all tissue compartments thereafter up to 2 weeks, after which Mregs were no longer detectable (14). Notably, cross-dressing of recipient antigen-presenting cells (APCs) with donor-specific Ag was not observed in this study.
DCregs are another myeloid-derived immune cell subset whose tolerogenic properties have been well-characterized (49) and have thus, garnered significant attention for clinical testing and use in transplant tolerance induction therapy (50). Extensive pre-clinical testing in organ- and skin transplanted mouse models, has demonstrated that the adoptive transfer of ex vivo- generated autologous or donor-derived DCreg prolongs allograft survival and promotes donor Ag- specific tolerance. These effects have been achieved either in the absence of, or in combination with, short-term IS (51–57). Two reports have suggested that donor-derived DCreg can prime the alloimmune response (58, 59). In a heterotopic cardiac transplantation rat model, infused autologous DCregs were labeled with PKH-26 red fluorescent cell linker which allowed their detection in spleens of recipient rats using immunofluorescence imaging of histological sections 5 days after administration (15). The use of additional fluorochromes allowed elucidation of interferon-gamma production induction as a potential mechanism of immunoregulation. The lipophilic membrane dye PKH was also used to label rapamycin-treated autologous DCregs pulsed with alloAg that were also administered to heart transplanted mice (16). DCreg homing to spleen was unaffected by rapamycin treatment, but conferred the capacity to suppress alloAg-specific T cell proliferation. Donor discriminatory MHC staining and flow cytometry analysis have also been utilized to detect in vivo survival of infused donor-derived DCregs in heart-transplanted mice, which has been shown to be short-lived likely due to killing/removal by host natural killer (NK) cells (60). Thus, the therapeutic effect of pre-transplant infusion of donor-derived DCreg does not appear to depend on the in vivo persistence of intact donor DCreg which offers a potential advantage over other cell therapy approaches for which immunosuppressive ability that may depend on in vivo persistence of the transferred regulatory cells.
In a human study published in 2011, two kidney transplant recipients were infused with donor-derived Mreg pre-operatively and shown to successfully wean to low-dose tacrolimus monotherapy within 24 weeks of transplantation, with no evidence of adverse effect or rejection (25). A small proportion (12%) of adoptively transferred Mregs were radiolabeled using 111In prior to infusion allowing for in vivo Mreg tracking in real-time using SPECT-CT scanning. Scintigrams reconstructed from SPECT imaging demonstrated initial trapping of labeled Mregs in the pulmonary vasculature, but after 2.5 h re-distributed to the peripheral blood, liver, and spleen. 24 h after infusion, Mregs were no longer detectable in the lungs or peripheral blood and were seen to accumulate in lymphoid and non-lymphoid organs (25). Pre-transplant administration of Mreg therapy in two enrolled kidney transplant recipients was most recently assessed for safety and feasibility as part of the multi-center ONE study, however efficacy and in vivo cell tracking/distribution were not evaluated (1, 61).
Donor discriminatory HLA staining is being used to track donor-derived DCregs infused 7 days before transplant into prospective living donor liver transplant recipients. Detection of the donor DCreg and their products is enhanced by image-based flow cytometry methods that can directly visualize the expression of MHC Ags and other gene products of donor or recipient origin by APCs in the circulation and host lymph nodes (26).
MSCs are naturally occurring, bone marrow-derived precursor cells, unique in their activation and migration to inflammatory sites, including allograft tissue, where they can exert their immunoregulatory effects, including upregulation of Treg differentiation in the inflammatory microenvironment (48). Administration of ex vivo-expanded MSCs has now consistently proven to be effective in prolonging allograft survival in murine models of solid organ transplantation (62). For in vivo tracking, cell labeling with PKH -26 red fluorescence cell linker has been used in murine models infused with autologous or donor-derived MSCs 7 days before kidney or semi-allogeneic heart transplantation (18, 19). In kidney allografted mice, adoptively transferred autologous MSCs infused 1 day prior to kidney transplantation preferentially migrated to the spleen, correlated with better graft survival, whereas post-transplant administration of MSCs was associated with infiltration of the allograft and subsequent C3 complement deposition without any therapeutic effect on allograft function (18). In cardiac allografted mice, PKH-26+ donor-derived MSCs infused prior to transplantation localized to liver, lung, primary and secondary lymphoid organs after infusion with none detected in peripheral blood. Survival in lymphoid tissue and lung was short-lived as PKH-26+ MSCs were not detected in these compartments at day 7 and 21 timepoints, while transferred MSCs were still detected in liver at day 7 post-infusion.
Multiple human studies investigating the safety, feasibility, and efficacy of adoptively transferred MSCs in solid organ transplantation are currently ongoing (63–65). One large randomized, controlled trial using MSC-based induction therapy in living donor kidney transplantation has already demonstrated reduced incidences of acute rejection, lower rates of infection, and improved 1-year graft function (63). Cell tracking/localization experiments in published human studies are lacking, however the importance of tissue localization following MSC administration is bound to prompt current or future human studies to incorporate non-invasive in vivo detection methods of this infused regulatory cell product.
Cell-based therapies are increasingly being considered and investigated for minimization of IS and induction/maintenance of tolerance in solid organ transplantation. As such, gaps in understanding of the in vivo fate of adoptively transferred regulatory immune cells after administration need to be filled in order to advance translation of these treatments to the clinic. Current direct cell labeling and flow cytometric analyses of target cells using intracellular dyes or surface marker tags have been efficacious in determining persistence of transferred cells in pre-clinical animal models; however, they lack anatomic information and are cumbersome to apply routinely to human studies due to the need for frequent blood draws and/or tissue biopsies. Cell radiolabeling in conjugation with imaging modalities such as SPECT or MRI has proven to be a more effective strategy of longitudinal in vivo cell monitoring in humans given its non-invasive approach, but commonly used radionuclides are often severely limited by their short half-lives. Advanced multi-modal approaches utilizing a dual reporter gene/radiolabeling system and whole-body imaging would provide the highest resolution and sensitivity of monitoring infused cell therapy in the most comprehensive and non-invasive way.
As current early phase human studies investigating various regulatory immune cell products for transplant tolerance advance to higher stages of clinical testing, incorporating some method of in vivo monitoring of the infused regulatory cell products without detriment to their function/survival will become imperative to ensure patient safety and maximize therapeutic potential.
Both authors contributed to the content design, literature searches, writing of the manuscript, and manuscript review. All authors contributed to the article and approved the submitted version.
Work described in this article was supported in part by National Institutes of Health (NIH) grants R01 AI118777, U01 AI 136779, and U19 AI131453 to AT, and by an NIH institutional research training fellowship to LT (T32 AI074490). Additionally, the University of Pittsburgh holds a Physician-Scientist Institutional Award from the Burroughs Wellcome Fund, which supports LMT.
The authors declare that the research was conducted in the absence of any commercial or financial relationships that could be construed as a potential conflict of interest.
1. Sawitzki B, Harden PN, Reinke P, Moreau A, Hutchinson JA, Game DS, et al. Regulatory cell therapy in kidney transplantation (The ONE Study): a harmonised design and analysis of seven non-randomised, single-arm, phase 1/2A trials. Lancet (2020) 395:1627–39. doi: 10.1016/S0140-6736(20)30167-7
2. Du X, Chang S, Guo W, Zhang S, Chen ZK. Progress in Liver Transplant Tolerance and Tolerance-Inducing Cellular Therapies. Front Immunol (2020) 11:1326:1326. doi: 10.3389/fimmu.2020.01326
3. Rana J, Biswas M. Regulatory T cell therapy: Current and future design perspectives. Cell Immunol (2020) 356:104193. doi: 10.1016/j.cellimm.2020.104193
4. Scarfe L, Taylor A, Sharkey J, Harwood R, Barrow M, Comenge J, et al. Non-invasive imaging reveals conditions that impact distribution and persistence of cells after in vivo administration. Stem Cell Res Ther (2018) 9:332. doi: 10.1186/s13287-018-1076-x
5. Lee HW, Gangadaran P, Kalimuthu S, Ahn BC. Advances in Molecular Imaging Strategies for In Vivo Tracking of Immune Cells. BioMed Res Int (2016) 2016:1946585. doi: 10.1155/2016/1946585
6. Xia G, He J, Zhang Z, Leventhal JR. Targeting acute allograft rejection by immunotherapy with ex vivo-expanded natural CD4+ CD25+ regulatory T cells. Transplantation (2006) 82:1749–55. doi: 10.1097/01.tp.0000250731.44913.ee00007890-200612270-00042[pii
7. Golshayan D, Jiang S, Tsang J, Garin MI, Mottet C, Lechler RI. In vitro-expanded donor alloantigen-specific CD4+CD25+ regulatory T cells promote experimental transplantation tolerance. Blood (2007) 109:827–35. doi: 10.1182/blood-2006-05-025460
8. Zhang N, Schroppel B, Lal G, Jakubzick C, Mao X, Chen D, et al. Regulatory T cells sequentially migrate from inflamed tissues to draining lymph nodes to suppress the alloimmune response. Immunity (2009) 30:458–69. doi: 10.1016/j.immuni.2008.12.022
9. Cheng HY, Tay SKL, Wen CJ, Lin CF, Wang AY, Shih LY, et al. Bioimaging of alloantigen-stimulated regulatory T cells in rat vascularized composite allotransplantation. PloS One (2018) 13:e0203624. doi: 10.1371/journal.pone.0203624
10. Liu L, Ye Q, Wu Y, Hsieh WY, Chen CL, Shen HH, et al. Tracking T-cells in vivo with a new nano-sized MRI contrast agent. Nanomed Nanotechnol Biol Med (2012) 8:1345–54. doi: 10.1016/j.nano.2012.02.017
11. Sharif-Paghaleh E, Sunassee K, Tavare R, Ratnasothy K, Koers A, Ali N, et al. In vivo SPECT reporter gene imaging of regulatory T cells. PloS One (2011) 6:e25857. doi: 10.1371/journal.pone.0025857
12. Dawson NA, Lamarche C, Hoeppli RE, Bergqvist P, Fung VC, McIver E, et al. Systematic testing and specificity mapping of alloantigen-specific chimeric antigen receptors in regulatory T cells. JCI Insight (2019) 4:e123672. doi: 10.1172/jci.insight.123672
13. Pierini A, Iliopoulou BP, Peiris H, Perez-Cruz M, Baker J, Hsu K, et al. T cells expressing chimeric antigen receptor promote immune tolerance. JCI Insight (2017) 2:e92865. doi: 10.1172/jci.insight.92865
14. Riquelme P, Tomiuk S, Kammler A, Fandrich F, Schlitt HJ, Geissler EK, et al. IFN-gamma-induced iNOS expression in mouse regulatory macrophages prolongs allograft survival in fully immunocompetent recipients. Mol Ther (2013) 21:409–22. doi: 10.1038/mt.2012.168
15. Hill M, Thebault P, Segovia M, Louvet C, Beriou G, Tilly G, et al. Cell therapy with autologous tolerogenic dendritic cells induces allograft tolerance through interferon-gamma and epstein-barr virus-induced gene 3. Am J Transplant (2011) 11:2036–45. doi: 10.1111/j.1600-6143.2011.03651.x
16. Taner T, Hackstein H, Wang Z, Morelli AE, Thomson AW. Rapamycin-treated, alloantigen-pulsed host dendritic cells induce Ag-specific T cell regulation and prolong graft survival. Am J Transplant (2005) 5:228–36. doi: 10.1046/j.1600-6143.2004.00673.x
17. Divito SJ, Wang Z, Shufesky WJ, Liu Q, Tkacheva OA, Montecalvo A, et al. Endogenous dendritic cells mediate the effects of intravenously injected therapeutic immunosuppressive dendritic cells in transplantation. Blood (2010) 116:2694–705. doi: 10.1182/blood-2009-10-251058
18. Casiraghi F, Azzollini N, Todeschini M, Cavinato RA, Cassis P, Solini S, et al. Localization of mesenchymal stromal cells dictates their immune or proinflammatory effects in kidney transplantation. Am J Transplant (2012) 12:2373–83. doi: 10.1111/j.1600-6143.2012.04115.x
19. Casiraghi F, Azzollini N, Cassis P, Imberti B, Morigi M, Cugini D, et al. Pretransplant infusion of mesenchymal stem cells prolongs the survival of a semiallogeneic heart transplant through the generation of regulatory T cells. J Immunol (2008) 181:3933–46. doi: 10.4049/jimmunol.181.6.3933
20. Zhang H, Guo H, Lu L, Zahorchak AF, Wiseman RW, Raimondi G, et al. Sequential monitoring and stability of ex vivo-expanded autologous and nonautologous regulatory T cells following infusion in nonhuman primates. Am J Transplant (2015) 15:1253–66. doi: 10.1111/ajt.13113
21. Singh K, Stempora L, Harvey RD, Kirk AD, Larsen CP, Blazar BR, et al. Superiority of rapamycin over tacrolimus in preserving nonhuman primate Treg half-life and phenotype after adoptive transfer. Am J Transplant (2014) 14:2691–703. doi: 10.1111/ajt.12934
22. Guo H, Zhang H, Lu L, Ezzelarab MB, Thomson AW. Generation, cryopreservation, function and in vivo persistence of ex vivo expanded cynomolgus monkey regulatory T cells. Cell Immunol (2015) 295:19–28. doi: 10.1016/j.cellimm.2015.02.006
23. Furlan SN, Singh K, Lopez C, Tkachev V, Hunt DJ, Hibbard J, et al. IL-2 enhances ex vivo-expanded regulatory T-cell persistence after adoptive transfer. Blood Adv (2020) 4:1594–605. doi: 10.1182/bloodadvances.2019001248
24. Chandran S, Tang Q, Sarwal M, Laszik ZG, Putnam AL, Lee K, et al. Polyclonal Regulatory T Cell Therapy for Control of Inflammation in Kidney Transplants. Am J Transplant (2017) 17:2945–54. doi: 10.1111/ajt.14415
25. Hutchinson JA, Riquelme P, Sawitzki B, Tomiuk S, Miqueu P, Zuhayra M, et al. Cutting Edge: Immunological consequences and trafficking of human regulatory macrophages administered to renal transplant recipients. J Immunol (2011) 187:2072–8. doi: 10.4049/jimmunol.1100762
26. Macedo C, Tran L, Zahorchak AF, Dia H, Gu X, Ravichandran R, et al. Donor-derived regulatory dendritic cell infusion results in host cell cross-dressing and T cell subset changes in prospective living donor liver transplant recipients. Am J Transplant. doi: 10.1111/ajt.16393
27. Romano M, Fanelli G, Albany CJ, Giganti G, Lombardi G. Past, Present, and Future of Regulatory T Cell Therapy in Transplantation and Autoimmunity. Front Immunol (2019) 10:43. doi: 10.3389/fimmu.2019.00043
28. Allan SE, Alstad AN, Merindol N, Crellin NK, Amendola M, Bacchetta R, et al. Generation of potent and stable human CD4+ T regulatory cells by activation-independent expression of FOXP3. Mol Ther (2008) 16:194–202. doi: 10.1038/sj.mt.6300341
29. Seng A, Krausz KL, Pei D, Koestler DC, Fischer RT, Yankee TM, et al. Coexpression of FOXP3 and a Helios isoform enhances the effectiveness of human engineered regulatory T cells. Blood Adv (2020) 4:1325–39. doi: 10.1182/bloodadvances.2019000965
30. Issa F, Hester J, Milward K, Wood KJ. Homing of regulatory T cells to human skin is important for the prevention of alloimmune-mediated pathology in an in vivo cellular therapy model. PloS One (2012) 7:e53331. doi: 10.1371/journal.pone.0053331
31. Kendal AR, Chen Y, Regateiro FS, Ma J, Adams E, Cobbold SP, et al. Sustained suppression by Foxp3+ regulatory T cells is vital for infectious transplantation tolerance. J Exp Med (2011) 208:2043–53. doi: 10.1084/jem.20110767
32. Burrell BE, Nakayama Y, Xu J, Brinkman CC, Bromberg JS. Regulatory T cell induction, migration, and function in transplantation. J Immunol (2012) 189:4705–11. doi: 10.4049/jimmunol.1202027
33. Anderson A, Martens CL, Hendrix R, Stempora LL, Miller WP, Hamby K, et al. Expanded nonhuman primate Tregs exhibit a unique gene expression signature and potently downregulate alloimmune responses. Am J Transplant (2008) 8:2252–64. doi: 10.1111/j.1600-6143.2008.02376.x
34. Dons EM, Raimondi G, Cooper DK, Thomson AW. Non-human primate regulatory T cells: current biology and implications for transplantation. Transplantation (2010) 90:811–6. doi: 10.1097/TP.0b013e3181ebf782
35. Alonso-Guallart P, Zitsman JS, Stern J, Kofman SB, Woodland D, Ho SH, et al. Characterization, biology, and expansion of regulatory T cells in the Cynomolgus macaque for preclinical studies. Am J Transplant (2019) 19:2186–98. doi: 10.1111/ajt.15313
36. Ma A, Qi S, Song L, Hu Y, Dun H, Massicotte E, et al. Adoptive transfer of CD4+CD25+ regulatory cells combined with low-dose sirolimus and anti-thymocyte globulin delays acute rejection of renal allografts in Cynomolgus monkeys. Int Immunopharmacol (2011) 11:618–29. doi: 10.1016/j.intimp.2010.11.001
37. Ezzelarab MB, Zhang H, Guo H, Lu L, Zahorchak AF, Wiseman RW, et al. Regulatory T cell infusion can enhance memory T cell and alloantibody responses in lymphodepleted nonhuman primate heart allograft recipients. Am J Transplant (2016) 16:1999–2015. doi: 10.1111/ajt.13685
38. Boardman DA, Philippeos C, Fruhwirth GO, Ibrahim MA, Hannen RF, Cooper D, et al. Expression of a Chimeric Antigen Receptor Specific for Donor HLA Class I Enhances the Potency of Human Regulatory T Cells in Preventing Human Skin Transplant Rejection. Am J Transplant (2017) 17:931–43. doi: 10.1111/ajt.14185
39. Noyan F, Zimmermann K, Hardtke-Wolenski M, Knoefel A, Schulde E, Geffers R, et al. Prevention of Allograft Rejection by Use of Regulatory T Cells With an MHC-Specific Chimeric Antigen Receptor. Am J Transplant (2017) 17:917–30. doi: 10.1111/ajt.14175
40. Bluestone JA, Buckner JH, Fitch M, Gitelman SE, Gupta S, Hellerstein MK, et al. Type 1 diabetes immunotherapy using polyclonal regulatory T cells. Sci Transl Med (2015) 7:315ra189. doi: 10.1126/scitranslmed.aad4134
41. Oo YH, Ackrill S, Cole R, Jenkins L, Anderson P, Jeffery HC, et al. Liver homing of clinical grade Tregs after therapeutic infusion in patients with autoimmune hepatitis. JHEP Rep: Innovation Hepatol (2019) 1:286–96. doi: 10.1016/j.jhepr.2019.08.001
42. Rosborough BR, Raich-Regue D, Turnquist HR, Thomson AW. Regulatory myeloid cells in transplantation. Transplantation (2014) 97:367–79. doi: 10.1097/TP.0b013e3182a860de
43. Garcia MR, Ledgerwood L, Yang Y, Xu J, Lal G, Burrell B, et al. Monocytic suppressive cells mediate cardiovascular transplantation tolerance in mice. J Clin Invest (2010) 120:2486–96. doi: 10.1172/JCI41628
44. Dugast AS, Haudebourg T, Coulon F, Heslan M, Haspot F, Poirier N, et al. Myeloid-derived suppressor cells accumulate in kidney allograft tolerance and specifically suppress effector T cell expansion. J Immunol (2008) 180:7898–906. doi: 10.4049/jimmunol.180.12.7898
45. Ezzelarab MB, Perez-Gutierrez A, Humar A, Wijkstrom M, Zahorchak AF, Lu-Casto L, et al. Preliminary assessment of the feasibility of autologous myeloid-derived suppressor cell infusion in non-human primate kidney transplantation. Transpl Immunol (2019) 56:101225. doi: 10.1016/j.trim.2019.101225
46. Scalea JR, Tomita Y, Lindholm CR, Burlingham W. Transplantation Tolerance Induction: Cell Therapies and Their Mechanisms. Front Immunol (2016) 7:87. doi: 10.3389/fimmu.2016.00087
47. Hoffmann SHL, Reck DI, Maurer A, Fehrenbacher B, Sceneay JE, Poxleitner M, et al. Visualization and quantification of in vivo homing kinetics of myeloid-derived suppressor cells in primary and metastatic cancer. Theranostics (2019) 9:5869–85. doi: 10.7150/thno.33275
48. Wood KJ, Bushell A, Hester J. Regulatory immune cells in transplantation. Nat Rev Immunol (2012) 12:417–30. doi: 10.1038/nri3227
49. Morelli AE, Thomson AW. Tolerogenic dendritic cells and the quest for transplant tolerance. Nat Rev Immunol (2007) 7:610–21. doi: 10.1038/nri2132
50. Thomson AW, Zahorchak AF, Ezzelarab MB, Butterfield LH, Lakkis FG, Metes DM. Prospective clinical testing of regulatory dendritic cells in organ transplantation. Front Immunol (2016) 7:15. doi: 10.3389/fimmu.2016.00015
51. Huang H, Dawicki W, Zhang X, Town J, Gordon JR. Tolerogenic dendritic cells induce CD4+CD25hiFoxp3+ regulatory T cell differentiation from CD4+CD25-/loFoxp3- effector T cells. J Immunol (2010) 185:5003–10. doi: 10.4049/jimmunol.0903446
52. Raker VK, Domogalla MP, Steinbrink K. Tolerogenic Dendritic Cells for Regulatory T Cell Induction in Man. Front Immunol (2015) 6:569. doi: 10.3389/fimmu.2015.00569
53. Fu F, Li Y, Qian S, Lu L, Chambers F, Starzl TE, et al. Costimulatory molecule-deficient dendritic cell progenitors (MHC class II+, CD80dim, CD86-) prolong cardiac allograft survival in nonimmunosuppressed recipients. Transplantation (1996) 62:659–65. doi: 10.1097/00007890-199609150-00021
54. Zhou Y, Shan J, Guo Y, Li S, Long D, Li Y, et al. Effects of adoptive transfer of tolerogenic dendritic cells on allograft survival in organ transplantation models: an overview of systematic reviews. J Immunol Res (2016) 2016:5730674. doi: 10.1155/2016/5730674
55. Xia MJ, Shan J, Li YP, Zhou YN, Guo YJ, Sun GX, et al. Adoptive transfusion of tolerogenic dendritic cells prolongs the survival of liver allograft: a systematic review. J Evidence-Based Med (2014) 7:135–46. doi: 10.1111/jebm.12094
56. Sato K, Yamashita N, Yamashita N, Baba M, Matsuyama T. Regulatory dendritic cells protect mice from murine acute graft-versus-host disease and leukemia relapse. Immunity (2003) 18:367–79. doi: 10.1016/S1074-7613(03)00055-4
57. Eun SC, Baek RM, Park CG. Prolongation of the rat composite tissue allograft survival by the combination of tolerogenic immature dendritic cells and short-term treatment with FK506. Transpl Proc (2013) 45:1792–6. doi: 10.1016/j.transproceed.2013.01.021
58. de Kort H, Crul C, van der Wal AM, Schlagwein N, Stax AM, Bruijn JA, et al. Accelerated antibody-mediated graft loss of rodent pancreatic islets after pretreatment with dexamethasone-treated immature donor dendritic cells. Transplantation (2012) 94:903–10. doi: 10.1097/TP.0b013e31826acd01
59. Smyth LA, Ratnasothy K, Moreau A, Alcock S, Sagoo P, Meader L, et al. Tolerogenic donor-derived dendritic cells risk sensitization in vivo owing to processing and presentation by recipient APCs. J Immunol (2013) 190:4848–60. doi: 10.4049/jimmunol.1200870
60. Rubtsov YP, Niec RE, Josefowicz S, Li L, Darce J, Mathis D, et al. Stability of the regulatory T cell lineage in vivo. Science (2010) 329:1667–71. doi: 10.1126/science.1191996
61. Hutchinson JA, Ahrens N, Geissler EK. MITAP-compliant characterization of human regulatory macrophages. Transpl Int (2017) 30:765–75. doi: 10.1111/tri.12988
62. Podesta MA, Remuzzi G, Casiraghi F. Mesenchymal Stromal Cells for Transplant Tolerance. Front Immunol (2019) 10:1287. doi: 10.3389/fimmu.2019.01287
63. Tan J, Wu W, Xu X, Liao L, Zheng F, Messinger S, et al. Induction therapy with autologous mesenchymal stem cells in living-related kidney transplants: a randomized controlled trial. JAMA (2012) 307:1169–77. doi: 10.1001/jama.2012.316
64. Pan GH, Chen Z, Xu L, Zhu JH, Xiang P, Ma JJ, et al. Low-dose tacrolimus combined with donor-derived mesenchymal stem cells after renal transplantation: a prospective, non-randomized study. Oncotarget (2016) 7:12089–101. doi: 10.18632/oncotarget.7725
Keywords: cell therapy, adoptive transfer, cell tracking, regulatory T cell, mesenchymal stromal cell, regulatory myeloid cell, transplantation
Citation: Tran LM and Thomson AW (2020) Detection and Monitoring of Regulatory Immune Cells Following Their Adoptive Transfer in Organ Transplantation. Front. Immunol. 11:614578. doi: 10.3389/fimmu.2020.614578
Received: 06 October 2020; Accepted: 12 November 2020;
Published: 14 December 2020.
Edited by:
Federica Casiraghi, Mario Negri Pharmacological Research Institute (IRCCS), ItalyReviewed by:
Gilles Benichou, Harvard Medical School, United StatesCopyright © 2020 Tran and Thomson. This is an open-access article distributed under the terms of the Creative Commons Attribution License (CC BY). The use, distribution or reproduction in other forums is permitted, provided the original author(s) and the copyright owner(s) are credited and that the original publication in this journal is cited, in accordance with accepted academic practice. No use, distribution or reproduction is permitted which does not comply with these terms.
*Correspondence: Angus W. Thomson, dGhvbXNvbmF3QHVwbWMuZWR1
Disclaimer: All claims expressed in this article are solely those of the authors and do not necessarily represent those of their affiliated organizations, or those of the publisher, the editors and the reviewers. Any product that may be evaluated in this article or claim that may be made by its manufacturer is not guaranteed or endorsed by the publisher.
Research integrity at Frontiers
Learn more about the work of our research integrity team to safeguard the quality of each article we publish.