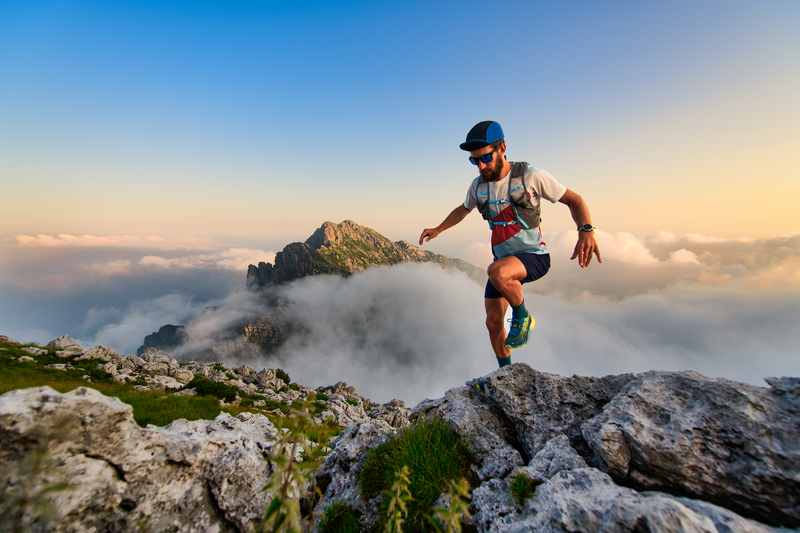
95% of researchers rate our articles as excellent or good
Learn more about the work of our research integrity team to safeguard the quality of each article we publish.
Find out more
MINI REVIEW article
Front. Immunol. , 29 January 2021
Sec. Alloimmunity and Transplantation
Volume 11 - 2020 | https://doi.org/10.3389/fimmu.2020.612737
This article is part of the Research Topic Novel Therapies for Tolerance Induction in Solid Organ and Bone Marrow Transplantation View all 14 articles
Pancreatic islet transplantation is a promising method for the treatment of type 1 and type 3 diabetes whereby replacement of islets may be curative. However, long-term treatment with immunosuppressive drugs (ISDs) remains essential for islet graft survival. Current ISD regimens carry significant side-effects for transplant recipients, and are also toxic to the transplanted islets. Pre-clinical efforts to induce immune tolerance to islet allografts identify ways in which the recipient immune system may be reeducated to induce a sustained transplant tolerance and even overcome autoimmune islet destruction. The goal of these efforts is to induce tolerance to transplanted islets with minimal to no long-term immunosuppression. Two most promising cell-based therapeutic strategies for inducing immune tolerance include T regulatory cells (Tregs) and donor and recipient hematopoietic mixed chimerism. Here, we review preclinical studies which utilize Tregs for tolerance induction in islet transplantation. We also review myeloablative and non-myeloablative hematopoietic stem cell transplantation (HSCT) strategies in preclinical and clinical studies to induce sustained mixed chimerism and allograft tolerance, in particular in islet transplantation. Since Tregs play a critical role in the establishment of mixed chimerism, it follows that the combination of Treg and HSCT may be synergistic. Since the success of the Edmonton protocol, the feasibility of clinical islet transplantation has been established and nascent clinical trials testing immune tolerance strategies using Tregs and/or hematopoietic mixed chimerism are underway or being formulated.
Type 1 diabetes (T1D) arises from an autoimmune attack of the insulin-producing, islet beta cells of the pancreas. Patients with T1D exhibit abnormalities in immune regulation that contribute to its etiology. Organ/tissue transplantation is complicated by adaptive CD4+ and CD8+ T cell responses that can contribute to allograft rejection (1–4). Owing to the combined specters of auto- and allo-immune responses, islet transplantation is one of the most challenging settings to prevent immune rejection.
Pharmacologic immunosuppressive drugs (ISDs) in islet transplantation traditionally target effector T cell proliferation and function to prevent graft rejection (5). However, most of these ISDs require life-long administration and have increased risk of multiple adverse reactions, including susceptibility to infection and incidence of secondary cancers (6, 7). In addition, survival of the transplanted islets is shortened due to direct toxic effects of the ISDs on islet β cells (8). One of the major goals in islet transplantation is the induction of immunosuppressive drug-free tolerance to the islet graft (9–11).
By virtue of their role in controlling alloreactive T cell responses to organ and tissue grafts, regulatory T cells (Tregs) are considered as promising alternatives to pharmacologic agents to promote engraftment and survival of the transplanted organs/tissues (12–14). Peripheral tolerance established by Tregs is crucial to prevent immune-mediated rejection of the transplanted graft (15, 16). Several preclinical studies have demonstrated induction of immune tolerance in different transplantation models such as heart, kidney, skin, liver, and islets (17–20). Multiple clinical trials are in progress evaluating the efficacy of recipient Tregs in organ transplantation tolerance (clinicaltrials.gov). One promising strategy in preclinical studies is the adoptive transfer of in vitro culture expanded Tregs to prevent the rejection of donor islet grafts (21, 22) and at least one clinical trial testing this approach is underway (NCT03444064). This phase I clinical trial aims to assess the safety and feasibility of autologous polyclonal Tregs in islet transplant patients. However, pre-clinical studies and clinical studies with recipient-derived Tregs in solid organ transplantation have shown that peripheral T cell tolerance is not necessarily durable and methods to enhance Treg function is an active area of research.
Another cell-based strategy for inducing islet allograft tolerance originates from studies which showed that the establishment of hematopoietic mixed chimerism between the donor and recipient results in donor allograft tolerance (Figure 1) (23, 24). Subsequent preclinical islet transplantation models which rely on mixed chimerism for tolerance induction have developed clinically translatable approaches (25–28). Encouragingly, clinical trials of combined kidney and hematopoietic cell transplantation (HCT) from living donors have demonstrated that immune tolerance to solid organs is possible by establishing hematopoietic mixed chimerism. Over 80% of HLA-matched patients enrolled in these trials are completely off ISDs (29, 30).
Figure 1 Treg and hematopoietic mixed chimerism as clinical strategies for tolerance induction. The left half of the figure shows direct effect of Tregs in inducing peripheral tolerance by regulating different immune cells such as dendritic cells and T cells to suppress alloreactivity. The adoptive transfer of different types of Tregs that been used in preclinical studies to support mechanisms of peripheral islet tolerance including polyclonal Tregs, antigen-specific Tregs, and engineered Tregs. These studies suggest Tregs might be used to reduce or eliminate systemic immunosuppression. The right half shows establishment of mixed hematopoietic chimerism through combined donor islet and hematopoietic stem cell transplantation. This is a state of coexistence of donor and recipient hematopoietic cell precursors with evidence to indicate that both mechanisms of central deletion of alloreactive responses and peripheral tolerance pathways regulate allograft tolerance. The administration of exogenous Tregs have been used to promote mixed hematopoietic chimerism and tolerance in preclinical studies. Treg are necessary for sustained chimerism and tolerance in these models and human clinical studies have shown Treg exert allo-antigen specific regulation in the setting of mixed chimerism. Ag, antigen; BMT, bone marrow transplantation; CTL, cytotoxic T lymphocytes; DC, dendritic cell; dTregs, donor-derived regulatory T cells; rTregs, recipient-derived regulatory T cells; T, T cell; Teff, effector T cell.
In the case of islet transplantation for T1D, experiments in preclinical murine models first reported almost 20 years ago have reproducibly shown that the establishment of hematopoietic mixed chimerism not only provides durable allograft tolerance but also prevents autoimmune islet destruction (31). A major problem in the translation of combined islet and HCT has been the traditionally toxic conditioning required for HCT, but the bone marrow transplantation field is rapidly evolving and significantly less toxic approaches have been developed or are in early phase clinical trials (32–34). Thus, combined islet and HCT is a promising area of translational investigation.
Since Tregs play a critical role in the establishment of tolerance in the setting of hematopoietic mixed chimerism (Figure 1), it is important to better understand Tregs in this setting. It is also possible that a combined immune therapy of Tregs and HCT may be synergistic (35).
Tregs are a small subsets of CD4+ T cells, characterized by the surface expression of CD4 and CD25, and the expression of the transcription factor forkhead box protein 3 (Foxp3) which is critical for their function (36). Tregs are well-known for their suppressive function and are responsible for safeguarding against various autoimmune diseases, including T1D (37, 38). This review focuses on major exogenously-administered Tregs that have been used in bone marrow and islet transplantation settings with a special emphasis on the classical CD4+CD25+Foxp3+ Tregs.
Non-obese diabetic (NOD) mice spontaneously develop autoimmune diabetes and share many features of human T1D (39, 40). Preclinical studies in NOD mice have shown that Treg can prevent autoimmune diabetes (41–43). The NOD mice which have defective CD28/B7 costimulation pathway and are prone to exacerbated T1D pathology showed delayed diabetes progression when injected with CD25+ Tregs (44). Moreover, adoptive transfer of islet-specific Tregs reversed Treg defect in CD28 deficient NOD mice and successfully prevented the disease progression (45). These series of findings suggest ex vivo-expanded Tregs as a way to satisfy Treg deficiency in the treatment of T1D.
T1D is characterized by presence of defective Tregs function and activation, particularly in the IL-2 pathway which can also affect Treg function (44–47). The role of IL-2 signaling in Treg development, metabolism, and function has been discussed in a recent review (48). Defective IL-2 signaling is associated with impaired Treg metabolism and diminished suppressive function (49). Ex vivo expansion of Tregs derived from T1D patients may be a principle way to correct for any inborn deficiency and these Tregs have been tested for their safety in phase 1 clinical trials with no evidence of therapy-related adverse events reported (50). Another clinical study has shown similar safety in pediatric T1D patients, and suggests disease modulation with observed reduced daily insulin requirement in treated patients (51, 52).
Treg therapy can be applied in two settings in islet transplantation: promoting islet survival in initial engraftment and inducing peripheral tolerance to eliminate immunosuppression.
The most common implantation site for clinical islet transplantation is within the liver via hepatic portal vein infusion (53). It is estimated that >50% of the initial islet mass that is infused is lost within the first few days due to local inflammatory changes and coagulation at the islet implantation site; the phenomena is termed as instant blood-mediated inflammatory reaction (IBMIR) (54, 55). The addition of Tregs at the time of islet infusion has been explored as a method for reducing initial islet graft loss and improving islet engraftment (56–58).
In addition to potentially changing the inflammation at the islet implantation site, experiments in which Tregs are either co-cultured, co-aggregated, or co-infused with islets have shown that Tregs appear to affect the islets themselves (59–62). In a preclinical study, co-culture of Tregs with the pancreatic islets altered production of inflammatory chemokines such as CCL2, CCL5, CXCL9, and CXCL10, produced by the islets themselves, benefitting islet graft survival after implantation under the kidney subcapsule (60). TGF-β secreted from the Tregs have been shown to improve islet viability and function in islet-Treg coculture experiment (63). Tregs might therefore improve islet viability and potentially reduce their immunogenicity.
A number of studies have provided preclinical evidence that Tregs incorporated into the islet graft itself or co-administered with the islet allograft can inhibit adaptive immune responses (59, 61, 64). In one example, Takemoto et al. constructed co-aggregates of BALB/c islets and C57BL/6 Tregs and transplanted into the liver of C57BL/6 mice where a long-term survival of the allogenic islets was observed for over 100 days without any immunosuppression (65).
Adoptive transfer of recipient-derived Tregs in preclinical models has shown to be effective in preventing islet allograft rejection through the establishment of transplant tolerance (Figure 1). Polyclonal Tregs have been used either to protect islets from direct contact-mediated immune attack or to modulate systemic immune response (59–61, 65, 66). Zhang et al. adaptably transferred donor antigen-specific Tregs in mice and found a profound synergistic effect with rapamycin in the islet allograft transplant setting (67). In another study by Lee et al, adoptive transfer of donor-reactive Tregs in T cell depleted mice resulted in indefinite survival of islet allografts. Moreover, in vitro expanded Tregs have been shown to delay porcine islet xenograft rejection in humanized mice by inhibiting graft-infiltrating effector T cells (64). In addition, multiple studies have demonstrated that local co-injection of islets and Tregs promotes islet engraftment (59, 60, 65).
An essential question remains unanswered in studies that examine the use of Treg therapy: which is better, donor or recipient Treg? This has perhaps been shaped by the perception that the only available source of clinical-grade Tregs is from the recipient, but Tregs can potentially be obtained from cadaveric spleen and bone marrow for clinical use.
It would be reasonable to hypothesize that both donor and recipient Tregs may reduce inflammation during islet transplantation. Perhaps donor Tregs would be more effective because of alloreactive responses to recipient MHC Class II expressed by local APCs or other cells. Likewise, recipient Tregs co-cultured with islets themselves might be more effective in changing islet profiles, as recipient Tregs may be more able to exert effector function through alloreactive TCR responses.
In case of Treg modulatory effects on adaptive immune responses, recipient Tregs might be favored as their initial alloreactive responses to islet tissue could locally shape the recipient adaptive immune response to allow alloreactive Tregs to persist and expand. Alternatively, donor Tregs might be more able to modulate adaptive responses by early and critical interactions with infiltrating recipient immune cells. In T1D patients, it is possible that recipient Tregs may also have deficiencies that could be avoided with the use of donor Treg therapy, however other methods such as ex vivo expansion or genetic modification of T1D Tregs are being explored (68–70). Alternatively, both donor or third-party Tregs could be utilized.
Outside of HCT, clinical trials with Tregs have generally shown an excellent safety profile but generally unclear efficacy. This may be because many studies do not use lymphodepletion, which may help with Treg engraftment (71, 72). Furthermore, the persistence of Tregs may be affected by the concomitant use of immunosuppressive drugs (73), with some evidence pointing to low dose IL-2 and rapamycin as a more effective strategy than other immunosuppressive regimins (74). Likewise, the use of low dose IL-2 and protein engineered IL-2 derivatives is being explored (75). Other promising methods of inducing Tregs in vivo such as the administration of tolerogenic CD11c+ DCs or pharmacologic stimulation of Treg are well described elsewhere (76–78).
Gene modification techniques have been proposed as alternative strategies to produce more active and efficacious Tregs in a large scale, involving two approaches: engineering Tregs with T cell receptor (TCR) (79–81) or chimeric antigen receptor (CAR) (82–84). Islet antigen-specific Tregs, generated using lentiviral-mediated TCR gene transfer, were capable of inhibiting effector T cells through antigen-specific suppression (81). This demonstrates the potential applicability of islet antigen-specific Tregs in the prevention of diabetes progression as well as in islet transplant settings. Islet antigen-specific Tregs generated using lentiviral transduction showed strong suppressive activity in an antigen-specific manner, providing a proof-of-concept for the potential use of TCR gene transfer technology-enhanced Treg activity in islet transplantation (81). Thus, gene transfer technology is likely to be adapted to enhance the therapeutic efficacy of Tregs while avoiding the pan-immunosuppression effect of polyclonal Tregs.
CAR Tregs are genetically engineered cells which express single chain variable fragment that recognizes specific antigen on target cells in an TCR-independent fashion. Recently, CAR Tregs have received growing attention in different transplantation models (84). Insulin-specific CAR Tregs generated using retroviral transduction were shown by Tenspolde et al. to be functionally stable and suppressive in vivo (85). The adoptive transfer of ex vivo expanded recipient Tregs transiently expressing CAR to target the MHC-I of donor islets in murine models showed improved initial allograft engraftment and survival, with donor-specific tolerance mechanisms observed (86). These studies suggest CAR Tregs could exert site-specific and localized immunosuppression.
The use of bone marrow to induce donor-specific tolerance has been tested in different solid organ transplantation models in preclinical and clinical studies in living donor transplantation (87–89). In a seminal study nearly 20 years ago, Sykes showed in murine models that immunological tolerance to allogeneic islets could be achieved in NOD mice with established disease through the bone marrow mixed chimerism across MHC barriers generated using a non-lethal dose of irradiation and a combination of anti-CD4, anti-CD8, anti-Thy1.2, and anti-CD40L mAbs (31). Since then, a number of studies have explored different conditioning regimens including those with different radiation doses or chemotherapy (fludarabine, cyclophosphamide, or busulfan) without irradation (26, 90, 91). A common thread to these studies, lymphodepletion was generally required for alloreactive graft tolerance and sustained chimerism (92).
One remarkable finding of a number of studies that explored NOD recipients is that the mixed chimerism induced from donors was sufficient to overcome autoimmune islet attack. Zeng et al. explored how the degree of MHC mismatch might affect autoimmunity. They showed that increased MHC mismatch from NOD recipients more effectively protects against autoimmune islet rejection (93). It is likely that human cadaveric donors of human islets will be HLA-mismatched, a major question in the clinical translation is which HLA alleles might be overlapping or not.
Oura et al. evaluated islet allograft survival in non-human primates using MHC-mismatched cynomolgus monkeys and found that islet allograft rejection is prevented as long as mixed hematopoietic chimerism is obtained. This is different from tolerance to kidneys transplanted into the same monkeys that were obtained even with a transient mixed chimerism (25, 94). This suggests that islet allografts may be more immunogenic or complicated than solid organ allografts in terms of tolerance induction in humans.
One of the major issues with bone marrow transplantation is the intensity of conditioning which has evolved over the past decades with some approaches such as the use of total lymphoid irradiation and antithymocyte globulin (TLI/ATG) have a good safety profile in combined organ and HCT (95). Current developments in safer conditioning in sickle cell are also being explored (96, 97). One of the most promising approach for newer and far less toxic HCT is the use of monoclonal antibodies against hematopoietic stem cell niche constituents instead of radiation or chemotherapy that is now being explored in patients with immune deficiency (98–100). Another complication is graft versus host disease (GVHD) (101) which in part is in large part mediated by donor T cells (102, 103). Early trial results of an ongoing phase 2 clinical trial of Treg therapy given at the time of HCT reinforce their capacity to prevent GVHD (104, 105). Aside from GVHD, dysregulated immunity can be a complication of GVHD which can include viral reactivation of cytomegalovirus (CMV) or Epstein bar virus (EBV) as well as susceptibility to pathogens or opportunistic infections (106, 107). Studies in the HCT setting have not shown an increased risk of viral reactivation with Treg therapy. Treg may help to regulate viral latency (108). In combined kidney and HCT studies in the HLA-matched and haploidentical setting, CMV reactivation might occur more frequently than in kidney transplant alone and appears controlled with antiviral medications (95), however the risks of immune dysregulation in the fully HLA-matched deceased donor setting remains unknown.
Following bone marrow transplantation, Tregs have been used to to prevent GVHD and to prolong allograft survival through the induction of mixed chimerism in combined marrow and organ transplantation (109). In this regard, studies report development of transplant tolerance by Tregs in the setting of mixed chimerism (35, 110), the dependency of tolerance on the presence of recipient Tregs (111), as well as the need for donor Tregs to prevent GVHD (112). In our murine studies, recipient Tregs have been shown to promote hematopoietic engraftment after HCT (86). Multiple other studies have shown that the addition of Tregs to conditioning increases donor hematopoietic engraftment (105, 113–115). It is interesting to observe a long-term graft tolerance even with the incorporation of Tregs which disappear shortly after infusion (73, 116). These findings suggest that the long-term graft survival might be due to the ability of the transferred Tregs to induce infectious tolerance. Recent studies show that both the donor and recipient Tregs contribute to suppress the alloreactive responses after HCT (105, 117, 118). The integration of Treg therapy into combined organ or islet transplantation is therefore a potentially non-toxic method for improving tolerance induction and establishing mixed hematopoietic chimerism. We are currently testing this in an ongoing trial in combined kidney and HCT from living donors (NCT03943238).
Finally, in the context of islet transplantation with concomitant HCT to induced mixed chimerism, donor Tregs are likely more effective in preventing graft-versus-host disease (GVHD) based on preclinical models in which donor Tregs were found to prevent GVHD when given at the time of HCT (112).
Clinical islet transplantation for T1D patients with severe hypoglycemia unawareness is an approved therapy in the majority of advanced nations (119). This population has severe morbidity and mortality; therefore, clinical trials are needed. Clinical trials integrating Treg therapy and/or hematopoietic mixed chimerism into islet transplantation have been limited. An ongoing clinical trial (NCT03444064) is testing the integration of autologous polyclonal Tregs in T1D patients who are receiving the conventional Edmonton islet transplantation protocol. Another clinical trial (NCT03162237) of islet xenotransplantation is currently underway and involves transplantation of 10,000 islet equivalent (IEQ) of porcine islets and infusion of 2 million/kg autologous Tregs in the recipients receiving induction immunotherapy with belatacept and maintenance immunotherapy with tacrolimus and mycophenolate mofetil. In the only report of combined islet and hematopoietic transplantation, a small six patient phase 1 trial integrating an infusion of cadaveric hematopoietic stem cells intravenously post-transplant did not successfully lead to donor chimerism or graft tolerance, but showed that the infusion of bone marrow cells from a cadaveric source is safe and potentially feasible (120).
Since the success of the Edmonton protocol in showing the benefit of islet transplantation to patients with hypoglycemia unawareness, the major challenge of achieving and maintaining tolerance remains. The integration of cell therapy approaches such as Treg therapy, mixed hematopoietic chimerism, or a combination of both remain promising.
All authors contributed to the article and approved the submitted version.
This study was supported by the Leona M. and Harry B. Helmsley Charitable Trust, Juvenile Diabetes Research Foundation United States of America, and the National Institute of Diabetes and Digestive and Kidney Diseases (grant no. P301P30DK11607401).
The authors declare that the research was conducted in the absence of any commercial or financial relationships that could be construed as a potential conflict of interest.
The authors thank Blake Mosher, Kent Jensen, and Preksha Bhagchandani for providing feedback on the manuscript.
1. Ingulli E. Mechanism of cellular rejection in transplantation. Pediatr Nephrol (2010) 25(1):61. doi: 10.1007/s00467-008-1020-x
2. Akatsuka Y, Nishida T, Kondo E, Miyazaki M, Taji H, Iida H, et al. Identification of a polymorphic gene, BCL2A1, encoding two novel hematopoietic lineage-specific minor histocompatibility antigens. J Exp Med (2003) 197(11):1489–500. doi: 10.1084/jem.20021925
3. Spierings E, Vermeulen CJ, Vogt MH, Doerner LE, Falkenburg JF, Mutis T, et al. Identification of HLA class II-restricted HY-specific T-helper epitope evoking CD4+ T-helper cells in HY-mismatched transplantation. Lancet (2003) 362(9384):610–5. doi: 10.1016/S0140-6736(03)14191-8
4. Haskins K, McDuffie M. Acceleration of diabetes in young NOD mice with a CD4+ islet-specific T cell clone. Science (1990) 249(4975):1433–6. doi: 10.1126/science.2205920
5. Grinyó JM, Cruzado JM. Mycophenolate mofetil and calcineurin-inhibitor reduction: recent progress. Am J Transplant (2009) 9(11):2447–52. doi: 10.1111/j.1600-6143.2009.02812.x
6. Pepper AR, Bruni A, Shapiro AMJ. Clinical islet transplantation: is the future finally now? Curr Opin Organ Transplant (2018) 23(4):428–39. doi: 10.1097/MOT.0000000000000546
7. Nanji SA, Shapiro AMJ. Islet Transplantation in Patients with Diabetes Mellitus. BioDrugs (2004) 18(5):315–28. doi: 10.2165/00063030-200418050-00004
8. Roep BO, Stobbe I, Duinkerken G, van Rood JJ, Lernmark A, Keymeulen B, et al. Auto- and alloimmune reactivity to human islet allografts transplanted into type 1 diabetic patients. Diabetes (1999) 48(3):484–90. doi: 10.2337/diabetes.48.3.484
9. Fisher JD, Zhang W. In situ recruitment of regulatory T cells promotes donor-specific tolerance in vascularized composite allotransplantation. Sci Adv (2020) 6: (11):eaax8429. doi: 10.1126/sciadv.aax8429
10. Oura T, Cosimi AB, Kawai T. Chimerism-based tolerance in organ transplantation: preclinical and clinical studies. Clin Exp Immunol (2017) 189: (2):190–6. doi: 10.1111/cei.12969
11. Hering BJ, Clarke WR, Bridges ND, Eggerman TL, Alejandro R, Bellin MD, et al. Phase 3 trial of transplantation of human islets in type 1 diabetes complicated by severe hypoglycemia. Diabetes Care (2016) 39(7):1230–40. doi: 10.2337/dc15-1988
12. Safinia N, Grageda N, Scotta C, Thirkell S, Fry LJ, Vaikunthanathan T, et al. Cell Therapy in Organ Transplantation: Our Experience on the Clinical Translation of Regulatory T Cells. Front Immunol (2018) 9:354. doi: 10.3389/fimmu.2018.00354
13. Martin-Moreno PL, Tripathi S, Chandraker A. Regulatory T Cells and Kidney Transplantation. Clin J Am Soc Nephrol CJASN (2018) 13(11):1760–4. doi: 10.2215/CJN.01750218
14. Romano M, Fanelli G, Albany CJ, Giganti G, Lombardi G. Past, Present, and Future of Regulatory T Cell Therapy in Transplantation and Autoimmunity. Front Immunol (2019) 10:43. doi: 10.3389/fimmu.2019.00043
15. Ashour HM, Niederkorn JY. Peripheral tolerance via the anterior chamber of the eye: role of B cells in MHC class I and II antigen presentation. J Immunol (Baltimore Md 1950) (2006) 176(10):5950–7. doi: 10.4049/jimmunol.176.10.5950
16. Sakaguchi S, Miyara M, Costantino CM, Hafler DA. FOXP3+ regulatory T cells in the human immune system. Nat Rev Immunol (2010) 10(7):490–500. doi: 10.1038/nri2785
17. Vaikunthanathan T, Safinia N. Regulatory T cells: tolerance induction in solid organ transplantation. Clin Exp Immunol (2017) 189: (2):197–210. doi: 10.1111/cei.12978
18. Long E, Wood KJ. Regulatory T cells in transplantation: transferring mouse studies to the clinic. Transplantation (2009) 88(9):1050–6. doi: 10.1097/TP.0b013e3181bb7913
19. Li W, Carper K, Zheng XX, Kuhr CS, Reyes JD, Liang Y, et al. The role of Foxp3+ regulatory T cells in liver transplant tolerance. Transplant Proc (2006) 38(10):3205–6. doi: 10.1016/j.transproceed.2006.10.093
20. Hu Y, Zhou H, Gao B. Role of regulatory T cells in CD47/donor-specific transfusion-induced immune tolerance in skin-heart transplantation mice. Transpl Infect Dis (2019) 21: (1):e13012. doi: 10.1111/tid.13012
21. Yolcu ES, Zhao H, Bandura-Morgan L, Lacelle C, Woodward KB, Askenasy N, et al. Pancreatic islets engineered with SA-FasL protein establish robust localized tolerance by inducing regulatory T cells in mice. J Immunol (Baltimore Md 1950) (2011) 187(11):5901–9. doi: 10.4049/jimmunol.1003266
22. Graca L, Le Moine A, Lin C-Y, Fairchild PJ, Cobbold SP, Waldmann H. Donor-specific transplantation tolerance: the paradoxical behavior of CD4+ CD25+ T cells. Proc Natl Acad Sci (2004) 101(27):10122–6. doi: 10.1073/pnas.0400084101
23. Sykes M, Sachs DH. Mixed allogeneic chimerism as an approach to transplantation tolerance. Immunol Today (1988) 9(1):23–7. doi: 10.1016/0167-5699(88)91352-7
24. Sykes M. Mixed chimerism and transplant tolerance. Immunity (2001) 14(4):417–24. doi: 10.1016/S1074-7613(01)00122-4
25. Oura T, Ko DS, Boskovic S, O’Neil JJ, Chipashvili V, Koulmanda M, et al. Kidney Versus Islet Allograft Survival After Induction of Mixed Chimerism With Combined Donor Bone Marrow Transplantation. Cell Transplant (2016) 25(7):1331–41. doi: 10.3727/096368915X688966
26. Wu T, Levay-Young B, Heuss N, Sozen H, Kirchhof N, Sutherland DE, et al. Inducing tolerance to MHC-matched allogeneic islet grafts in diabetic NOD mice by simultaneous islet and bone marrow transplantation under nonirradiative and nonmyeloablative conditioning therapy. Transplantation (2002) 74(1):22–7. doi: 10.1097/00007890-200207150-00005
27. Ikebukuro K, Adachi Y, Yamada Y, Fujimoto S, Seino Y, Oyaizu H, et al. Treatment of streptozotocin-induced diabetes mellitus by transplantation of islet cells plus bone marrow cells via portal vein in rats. Transplantation (2002) 73(4):512–8. doi: 10.1097/00007890-200202270-00004
28. Ikebukuro K, Adachi Y, Suzuki Y, Iwasaki M, Nakano K, Koike Y, et al. Synergistic effects of injection of bone marrow cells into both portal vein and bone marrow on tolerance induction in transplantation of allogeneic pancreatic islets. Bone Marrow Transplant (2006) 38(10):657–64. doi: 10.1038/sj.bmt.1705500
29. Scandling JD, Busque S, Dejbakhsh-Jones S, Benike C, Millan MT, Shizuru JA, et al. Tolerance and chimerism after renal and hematopoietic-cell transplantation. New Engl J Med (2008) 358(4):362–8. doi: 10.1056/NEJMoa074191
30. Scandling J, Busque S, Shizuru J, Lowsky R, Hoppe R, Dejbakhsh-Jones S, et al. Chimerism, graft survival, and withdrawal of immunosuppressive drugs in HLA matched and mismatched patients after living donor kidney and hematopoietic cell transplantation. Am J Transplant (2015) 15(3):695–704. doi: 10.1111/ajt.13091
31. Nikolic B, Takeuchi Y, Leykin I, Fudaba Y, Smith RN, Sykes M. Mixed hematopoietic chimerism allows cure of autoimmune diabetes through allogeneic tolerance and reversal of autoimmunity. Diabetes (2004) 53(2):376–83. doi: 10.2337/diabetes.53.2.376
32. Ishida T, Hishizawa M, Kato K, Tanosaki R, Fukuda T, Taniguchi S, et al. Allogeneic hematopoietic stem cell transplantation for adult T-cell leukemia-lymphoma with special emphasis on preconditioning regimen: a nationwide retrospective study. Blood J Am Soc Hematol (2012) 120(8):1734–41. doi: 10.1182/blood-2012-03-414490
33. Yu SP, Wei Z, Wei L. Preconditioning strategy in stem cell transplantation therapy. Trans Stroke Res (2013) 4(1):76–88. doi: 10.1007/s12975-012-0251-0
34. Li Y, Sedello A, Domen J. Tolerance Induction by Myeloid Progenitor Cells Does Not Require Lethal Preconditioning or Hematopoietic Stem Cell Transplantation. J Heart Lung Transplant (2019) 38(4):S40. doi: 10.1016/j.healun.2019.01.082
35. Ruiz P, Maldonado P, Hidalgo Y, Sauma D, Rosemblatt M, Bono MR. Alloreactive Regulatory T Cells Allow the Generation of Mixed Chimerism and Transplant Tolerance. Front Immunol (2015) 6:596. doi: 10.3389/fimmu.2015.00596
36. Rudensky AY. Regulatory T cells and Foxp3. Immunol Rev (2011) 241(1):260–8. doi: 10.1111/j.1600-065X.2011.01018.x
37. Chatenoud L, Salomon B, Bluestone JA. Suppressor T cells–they’re back and critical for regulation of autoimmunity! Immunol Rev (2001) 182:149–63. doi: 10.1034/j.1600-065X.2001.1820112.x
38. Brusko T, Atkinson M. Treg in type 1 diabetes. Cell Biochem Biophys (2007) 48(2-3):165–75. doi: 10.1007/s12013-007-0018-5
39. Nakayama M, Abiru N, Moriyama H, Babaya N, Liu E, Miao D, et al. Prime role for an insulin epitope in the development of type 1 diabetes in NOD mice. Nature (2005) 435(7039):220–3. doi: 10.1038/nature03523
40. Kishimoto H, Sprent J. A defect in central tolerance in NOD mice. Nat Immunol (2001) 2(11):1025–31. doi: 10.1038/ni726
41. Salomon B, Lenschow DJ, Rhee L, Ashourian N, Singh B, Sharpe A, et al. B7/CD28 costimulation is essential for the homeostasis of the CD4+CD25+ immunoregulatory T cells that control autoimmune diabetes. Immunity (2000) 12(4):431–40. doi: 10.1016/S1074-7613(00)80195-8
42. Bluestone JA, Tang Q. Therapeutic vaccination using CD4+CD25+ antigen-specific regulatory T cells. Proc Natl Acad Sci U States America (2004) 101 Suppl 2:14622–6. doi: 10.1073/pnas.0405234101
43. Tritt M, Sgouroudis E, d’Hennezel E, Albanese A, Piccirillo CA. Functional waning of naturally occurring CD4+ regulatory T-cells contributes to the onset of autoimmune diabetes. Diabetes (2008) 57(1):113–23. doi: 10.2337/db06-1700
44. Okubo Y, Torrey H, Butterworth J, Zheng H, Faustman DL. Treg activation defect in type 1 diabetes: correction with TNFR2 agonism. Clin Trans Immunol (2016) 5(1):e56. doi: 10.1038/cti.2015.43
45. Garg G, Tyler JR, Yang JH, Cutler AJ, Downes K, Pekalski M, et al. Type 1 diabetes-associated IL2RA variation lowers IL-2 signaling and contributes to diminished CD4+ CD25+ regulatory T cell function. J Immunol (2012) 188(9):4644–53. doi: 10.4049/jimmunol.1100272
46. McClymont SA, Putnam AL, Lee MR, Esensten JH, Liu W, Hulme MA, et al. Plasticity of human regulatory T cells in healthy subjects and patients with type 1 diabetes. J Immunol (Baltimore Md 1950) (2011) 186(7):3918–26. doi: 10.4049/jimmunol.1003099
47. Long SA, Cerosaletti K, Bollyky PL, Tatum M, Shilling H, Zhang S, et al. Defects in IL-2R signaling contribute to diminished maintenance of FOXP3 expression in CD4+ CD25+ regulatory T-cells of type 1 diabetic subjects. diabetes (2010) 59(2):407–15. doi: 10.2337/db09-0694
48. Malek TR. The Biology of Interleukin-2. Annu Rev Immunol (2008) 26(1):453–79. doi: 10.1146/annurev.immunol.26.021607.090357
49. Fan MY, Low JS, Tanimine N, Finn KK, Priyadharshini B, Germana SK, et al. Differential Roles of IL-2 Signaling in Developing versus Mature Tregs. Cell Rep (2018) 25(5):1204–13.e4. doi: 10.1016/j.celrep.2018.10.002
50. Bluestone JA, Buckner JH, Fitch M, Gitelman SE, Gupta S, Hellerstein MK, et al. Type 1 diabetes immunotherapy using polyclonal regulatory T cells. Sci Trans Med (2015) 7(315):315ra189. doi: 10.1126/scitranslmed.aad4134
51. Marek-Trzonkowska N, Mysliwiec M, Dobyszuk A, Grabowska M, Techmanska I, Juscinska J, et al. Administration of CD4+CD25highCD127- regulatory T cells preserves beta-cell function in type 1 diabetes in children. Diabetes Care (2012) 35(9):1817–20. doi: 10.2337/dc12-0038
52. Marek-Trzonkowska N, Myśliwiec M, Dobyszuk A, Grabowska M, Derkowska I, Juścińska J, et al. Therapy of type 1 diabetes with CD4+CD25highCD127-regulatory T cells prolongs survival of pancreatic islets — Results of one year follow-up. Clin Immunol (2014) 153(1):23–30. doi: 10.1016/j.clim.2014.03.016
53. Rickels MR, Robertson RP. Pancreatic islet transplantation in humans: recent progress and future directions. Endocr Rev (2019) 40(2):631–68. doi: 10.1210/er.2018-00154
54. Kanak M, Saravanan P, Levy M. Inflammatory response and its impact on outcome of islet transplantation. CellR4 (2019) 7:e2739. doi: 10.32113/cellr4_20198_2735
55. Wang J, Sun Z, Gou W, Adams DB, Cui W, Morgan KA, et al. α-1 antitrypsin enhances islet engraftment by suppression of instant blood-mediated inflammatory reaction. Diabetes (2017) 66(4):970–80. doi: 10.2337/db16-1036
56. Yoon IH, Chung H, Kim HJ, Nam HY, Shin JS, Kim YH, et al. Peri-graft porcine-specific CD4(+) FoxP3(+) regulatory T cells by CD40-CD154 blockade prevented the rejection of porcine islet graft in diabetic mice. Xenotransplantation (2019) 26: (5):e12533. doi: 10.1111/xen.12533
57. Gregori S, Casorati M, Amuchastegui S, Smiroldo S, Davalli AM, Adorini L. Regulatory T cells induced by 1 alpha,25-dihydroxyvitamin D3 and mycophenolate mofetil treatment mediate transplantation tolerance. J Immunol (Baltimore Md 1950) (2001) 167(4):1945–53. doi: 10.4049/jimmunol.167.4.1945
58. Gagliani N, Jofra T, Valle A, Stabilini A, Morsiani C, Gregori S, et al. Transplant tolerance to pancreatic islets is initiated in the graft and sustained in the spleen. Am J Transplant (2013) 13(8):1963–75. doi: 10.1111/ajt.12333
59. Pathak S, Pham TT, Jeong J-H, Byun Y. Immunoisolation of pancreatic islets via thin-layer surface modification. J Controlled Release (2019) 305:176–93. doi: 10.1016/j.jconrel.2019.04.034
60. Chen D, Zhang N, Fu S, Schröppel B, Guo Q, Garin A, et al. CD4+ CD25+ regulatory T-cells inhibit the islet innate immune response and promote islet engraftment. Diabetes (2006) 55(4):1011–21. doi: 10.2337/diabetes.55.04.06.db05-1048
61. Khattar M, Deng R, Kahan BD, Schroder PM, Phan T, Rutzky LP, et al. Novel sphingosine-1-phosphate receptor modulator KRP203 combined with locally delivered regulatory T cells induces permanent acceptance of pancreatic islet allografts. Transplantation (2013) 95(7):919–27. doi: 10.1097/TP.0b013e3182842396
62. Kim J, Hope CM, Gantumur N, Perkins GB, Stead SO, Yue Z, et al. Encapsulation of Human Natural and Induced Regulatory T-Cells in IL-2 and CCL1 Supplemented Alginate-GelMA Hydrogel for 3D Bioprinting. Adv Funct Mater (2020) 30(15):2000544. doi: 10.1002/adfm.202000544
63. Choi B, Kim S-H. Regulatory T Cells Promote Pancreatic Islet Function and Viability via TGF-β1 in vitro and in vivo. Korean J Clin Lab Sci (2018) 50(3):304–12. doi: 10.15324/kjcls.2018.50.3.304
64. Yi S, Ji M, Wu J, Ma X, Phillips P, Hawthorne WJ, et al. Adoptive transfer with in vitro expanded human regulatory T cells protects against porcine islet xenograft rejection via interleukin-10 in humanized mice. Diabetes (2012) 61(5):1180–91. doi: 10.2337/db11-1306
65. Takemoto N, Konagaya S, Kuwabara R, Iwata H. Coaggregates of Regulatory T Cells and Islet Cells Allow Long-term Graft Survival in Liver Without Immunosuppression. Transplantation (2015) 99(5):942–7. doi: 10.1097/TP.0000000000000579
66. Lee K, Nguyen V, Lee KM, Kang SM, Tang Q. Attenuation of donor-reactive T cells allows effective control of allograft rejection using regulatory T cell therapy. Am J Transplant (2014) 14(1):27–38. doi: 10.1111/ajt.12509
67. Zhang D, Zhang W, Ng TW, Wang Y, Liu Q, Gorantla V, et al. Adoptive cell therapy using antigen-specific CD4–CD8– T regulatory cells to prevent autoimmune diabetes and promote islet allograft survival in NOD mice. Diabetologia (2011) 54(8):2082–92. doi: 10.1007/s00125-011-2179-4
68. Grinberg-Bleyer Y, Baeyens A, You S, Elhage R, Fourcade G, Gregoire S, et al. IL-2 reverses established type 1 diabetes in NOD mice by a local effect on pancreatic regulatory T cells. J Exp Med (2010) 207(9):1871–8. doi: 10.1084/jem.20100209
69. Visperas A, Vignali DA. Are regulatory T cells defective in type 1 diabetes and can we fix them? J Immunol (2016) 197(10):3762–70. doi: 10.4049/jimmunol.1601118
70. Hull CM, Peakman M, Tree TI. Regulatory T cell dysfunction in type 1 diabetes: what’s broken and how can we fix it? Diabetologia (2017) 60(10):1839–50. doi: 10.1007/s00125-017-4377-1
71. Tang Q, Vincenti F. Transplant trials with Tregs: perils and promises. J Clin Invest (2017) 127(7):2505–12. doi: 10.1172/JCI90598
72. Romano M, Tung SL, Smyth LA, Lombardi G. Treg therapy in transplantation: a general overview. Transplant Int (2017) 30(8):745–53. doi: 10.1111/tri.12909
73. Singh K, Stempora L, Harvey RD, Kirk AD, Larsen CP, Blazar BR, et al. Superiority of rapamycin over tacrolimus in preserving nonhuman primate Treg half-life and phenotype after adoptive transfer. Am J Transplant (2014) 14(12):2691–703. doi: 10.1111/ajt.12934
74. Hu M, Hawthorne WJ, Nicholson L, Burns H, Qian YW, Liuwantara D, et al. Low-Dose Interleukin-2 Combined With Rapamycin Led to an Expansion of CD4(+)CD25(+)FOXP3(+) Regulatory T Cells and Prolonged Human Islet Allograft Survival in Humanized Mice. Diabetes (2020) 69: (8):1735–48. doi: 10.2337/db19-0525
75. Levin AM, Bates DL, Ring AM, Krieg C, Lin JT, Su L, et al. Exploiting a natural conformational switch to engineer an interleukin-2 ‘superkine’. Nature (2012) 484(7395):529–33. doi: 10.1038/nature10975
76. Domogalla MP, Rostan PV, Raker VK, Steinbrink K. Tolerance through Education: How Tolerogenic Dendritic Cells Shape Immunity. Front Immunol (2017) 8(1764):1764. doi: 10.3389/fimmu.2017.01764
77. Kushwah R, Hu J. Role of dendritic cells in the induction of regulatory T cells. Cell Biosci (2011) 1(1):20. doi: 10.1186/2045-3701-1-20
78. Maldonado RA, von Andrian UH. Chapter 4 - How Tolerogenic Dendritic Cells Induce Regulatory T Cells. In: Alt FW, Austen KF, Honjo T, Melchers F, Uhr JW, Unanue ER, editors. Adv Immunol. Academic Press, Elsevier (2010). p. 111–65. doi: 10.1016/B978-0-12-380995-7.00004-5
79. Brusko TM, Koya RC, Zhu S, Lee MR, Putnam AL, McClymont SA, et al. Human antigen-specific regulatory T cells generated by T cell receptor gene transfer. PloS One (2010) 5(7):e11726. doi: 10.1371/journal.pone.0011726
80. Tsang JY-S, Tanriver Y, Jiang S, Xue S-A, Ratnasothy K, Chen D, et al. Conferring indirect allospecificity on CD4+ CD25+ Tregs by TCR gene transfer favors transplantation tolerance in mice. J Clin Invest (2008) 118(11):3619–28. doi: 10.1172/JCI33185
81. Hull CM, Nickolay LE, Estorninho M, Richardson MW, Riley JL, Peakman M, et al. Generation of human islet-specific regulatory T cells by TCR gene transfer. J Autoimmun (2017) 79:63–73. doi: 10.1016/j.jaut.2017.01.001
82. McGovern JL, Wright GP, Stauss HJ. Engineering Specificity and Function of Therapeutic Regulatory T Cells. Front Immunol (2017) 8:1517. doi: 10.3389/fimmu.2017.01517
83. Noyan F, Zimmermann K, Hardtke-Wolenski M, Knoefel A, Schulde E, Geffers R, et al. Prevention of allograft rejection by use of regulatory T cells with an MHC-specific chimeric antigen receptor. Am J Transplant (2017) 17(4):917–30. doi: 10.1111/ajt.14175
84. Zhang Q, Lu W, Liang C-L, Chen Y, Liu H, Qiu F, et al. Chimeric antigen receptor (CAR) Treg: a promising approach to inducing immunological tolerance. Front Immunol (2018) 9:2359. doi: 10.3389/fimmu.2018.02359
85. Tenspolde M, Zimmermann K, Weber LC, Hapke M, Lieber M, Dywicki J, et al. Regulatory T cells engineered with a novel insulin-specific chimeric antigen receptor as a candidate immunotherapy for type 1 diabetes. J Autoimmun (2019) 103:102289. doi: 10.1016/j.jaut.2019.05.017
86. Pierini A, Iliopoulou BP, Peiris H, Perez-Cruz M, Baker J, Hsu K, et al. T cells expressing chimeric antigen receptor promote immune tolerance. JCI Insight (2017) 2(20):e92865. doi: 10.1172/jci.insight.92865
87. Zeevi A, Pavlick M, Banas R, Bentlejewski C, Spichty K, Rao A, et al. Three years of follow-up of bone marrow-augmented organ transplant recipients: the impact on donor-specific immune modulation. Transplant Proc NIH Public Access (1997) p:1205. doi: 10.1016/S0041-1345(96)00552-0
88. Thomas J, Carver M, Cunningham P, Park K, Gonder J, Thomas F. Promotion of incompatible allograft acceptance in rhesus monkeys given posttransplant antithymocyte globulin and donor bone marrow. I. In vivo parameters and immunohistologic evidence suggesting microchimerism. Transplantation (1987) 43(3):332–8. doi: 10.1097/00007890-198703000-00002
89. Fontes P, Rao AS, Demetris AJ, Zeevi A, Trucco M, Carroll P, et al. Bone marrow augmentation of donor-cell chimerism in kidney, liver, heart, and pancreas islet transplantation. Lancet (1994) 344(8916):151. doi: 10.1016/S0140-6736(94)92756-1
90. Luo B, Nanji SA, Schur CD, Pawlick RL, Anderson CC, Shapiro AM. Robust tolerance to fully allogeneic islet transplants achieved by chimerism with minimal conditioning. Transplantation (2005) 80(3):370–7. doi: 10.1097/01.tp.0000167724.38038.ae
91. Li H, Inverardi L, Molano RD, Pileggi A, Ricordi C. Nonlethal conditioning for the induction of allogeneic chimerism and tolerance to islet allografts. Transplantation (2003) 75(7):966–70. doi: 10.1097/01.TP.0000058516.74246.71
92. Lee BW, Lee JI, Oh SH, Ahn YR, Chae HY, Lee MS, et al. A more persistent tolerance to islet allografts through bone marrow transplantation in minimal nonmyeloablative conditioning therapy. Transplant Proc (2005) 37(5):2266–9. doi: 10.1016/j.transproceed.2005.03.044
93. Wang M, Racine J, Zhang M, Wu T, Deng R, Johnston H, et al. MHC-mismatched chimerism is required for induction of transplantation tolerance in autoimmune nonobese diabetic recipients. J Immunol (2014) 193(4):2005–15. doi: 10.4049/jimmunol.1401137
94. Oura T, Hotta K, Rosales I, Dehnadi A, Kawai K, Lee H, et al. Addition of Anti-CD40 Monoclonal Antibody to Nonmyeloablative Conditioning With Belatacept Abrogated Allograft Tolerance Despite Induction of Mixed Chimerism. Transplantation (2019) 103(1):168–76. doi: 10.1097/TP.0000000000002417
95. Scandling JD, Busque S, Lowsky R, Shizuru J, Shori A, Engleman E, et al. Macrochimerism and clinical transplant tolerance. Hum Immunol (2018) 79(5):266–71. doi: 10.1016/j.humimm.2018.01.002
96. Gaziev J, Isgro A, Sodani P, Paciaroni K, De Angelis G, Marziali M, et al. Haploidentical HSCT for hemoglobinopathies: improved outcomes with TCRαβ+/CD19+-depleted grafts. Blood Adv (2018) 2(3):263–70. doi: 10.1182/bloodadvances.2017012005
97. Oevermann L, Schulte JH, Hundsdörfer P, Hakimeh D, Kogel F, Lang P, et al. HLA-haploidentical hematopoietic stem cell transplantation in pediatric patients with hemoglobinopathies: current practice and new approaches. Bone Marrow Transplant (2019) 54(2):743–8. doi: 10.1038/s41409-019-0598-x
98. Nelson AS, Marsh RA, Myers KC, Davies SM, Jodele S, O’Brien TA, et al. A Reduced-Intensity Conditioning Regimen for Patients with Dyskeratosis Congenita Undergoing Hematopoietic Stem Cell Transplantation. Biol Blood Marrow Transplant (2016) 22(5):884–8. doi: 10.1016/j.bbmt.2016.01.026
99. Straathof KC, Rao K, Eyrich M, Hale G, Bird P, Berrie E, et al. Haemopoietic stem-cell transplantation with antibody-based minimal-intensity conditioning: a phase 1/2 study. Lancet (2009) 374(9693):912–20. doi: 10.1016/S0140-6736(09)60945-4
100. Czechowicz A, Kraft D, Weissman IL, Bhattacharya D. Efficient transplantation via antibody-based clearance of hematopoietic stem cell niches. Science (2007) 318(5854):1296–9. doi: 10.1126/science.1149726
101. Sakoda Y, Hashimoto D, Asakura S, Takeuchi K, Harada M, Tanimoto M, et al. Donor-derived thymic-dependent T cells cause chronic graft-versus-host disease. Blood (2007) 109(4):1756–64. doi: 10.1182/blood-2006-08-042853
102. Wagner JE, Thompson JS, Carter SL, Kernan NA. Effect of graft-versus-host disease prophylaxis on 3-year disease-free survival in recipients of unrelated donor bone marrow (T-cell Depletion Trial): a multi-centre, randomised phase II–III trial. Lancet (2005) 366(9487):733–41. doi: 10.1016/S0140-6736(05)66996-6
103. Barrett A, Mavroudis D, Tisdale J, Molldrem J, Clave E, Dunbar C, et al. T cell-depleted bone marrow transplantation and delayed T cell add-back to control acute GVHD and conserve a graft-versus-leukemia effect. Bone Marrow Transplant (1998) 21(6):543–51. doi: 10.1038/sj.bmt.1701131
104. Meyer EH, Laport G, Xie BJ, MacDonald K, Heydari K, Sahaf B, et al. Transplantation of donor grafts with defined ratio of conventional and regulatory T cells in HLA-matched recipients. JCI Insight (2019) 4(10):e127244. doi: 10.1172/jci.insight.127244
105. Di Ianni M, Falzetti F, Carotti A, Terenzi A, Castellino F, Bonifacio E, et al. Tregs prevent GVHD and promote immune reconstitution in HLA-haploidentical transplantation. Blood J Am Soc Hematol (2011) 117(14):3921–8. doi: 10.1182/blood-2010-10-311894
106. Somekh I, Marquardt B, Liu Y, Rohlfs M, Hollizeck S, Karakukcu M, et al. Novel mutations in RASGRP1 are associated with immunodeficiency, immune dysregulation, and EBV-induced lymphoma. J Clin Immunol (2018) 38(6):699–710. doi: 10.1007/s10875-018-0533-8
107. Kruizinga MD, van Tol MJ, Bekker V, Netelenbos T, Smiers FJ, Bresters D, et al. Risk factors, treatment, and immune dysregulation in autoimmune cytopenia after allogeneic hematopoietic stem cell transplantation in pediatric patients. Biol Blood Marrow Transplant (2018) 24(4):772–8. doi: 10.1016/j.bbmt.2017.12.782
108. Almanan M, Raynor J, Sholl A, Wang M, Chougnet C, Cardin RD, et al. Tissue-specific control of latent CMV reactivation by regulatory T cells. PloS Pathog (2017) 13(8):e1006507. doi: 10.1371/journal.ppat.1006507
109. Pilat N, Granofszky N, Wekerle T. Combining Adoptive Treg Transfer with Bone Marrow Transplantation for Transplantation Tolerance. Curr Transplant Rep (2017) 4(4):253–61. doi: 10.1007/s40472-017-0164-7
110. Joffre O, Santolaria T, Calise D, Al Saati T, Hudrisier D, Romagnoli P, et al. Prevention of acute and chronic allograft rejection with CD4+CD25+Foxp3+ regulatory T lymphocytes. Nat Med (2008) 14(1):88–92. doi: 10.1038/nm1688
111. Hongo D, Tang X, Dutt S, Nador RG, Strober S. Interactions between NKT cells and Tregs are required for tolerance to combined bone marrow and organ transplants. Blood J Am Soc Hematol (2012) 119(6):1581–9. doi: 10.1182/blood-2011-08-371948
112. Pillai AB, George TI, Dutt S, Strober S. Host natural killer T cells induce an interleukin-4–dependent expansion of donor CD4+ CD25+ Foxp3+ T regulatory cells that protects against graft-versus-host disease. Blood J Am Soc Hematol (2009) 113(18):4458–67. doi: 10.1182/blood-2008-06-165506
113. Wekerle T, Sykes M. Mixed chimerism and transplantation tolerance. Annu Rev Med (2001) 52(1):353–70. doi: 10.1146/annurev.med.52.1.353
114. Nguyen VH, Shashidhar S, Chang DS, Ho L, Kambham N, Bachmann M, et al. The impact of regulatory T cells on T-cell immunity following hematopoietic cell transplantation. Blood J Am Soc Hematol (2008) 111(2):945–53. doi: 10.1182/blood-2007-07-103895
115. Nguyen VH, Zeiser R, Negrin RS. Role of Naturally Arising Regulatory T Cells in Hematopoietic Cell Transplantation. Biol Blood Marrow Transplant (2006) 12(10):995–1009. doi: 10.1016/j.bbmt.2006.04.009
116. Guo H, Zhang H, Lu L, Ezzelarab MB, Thomson AW. Generation, cryopreservation, function and in vivo persistence of ex vivo expanded cynomolgus monkey regulatory T cells. Cell Immunol (2015) 295(1):19–28. doi: 10.1016/j.cellimm.2015.02.006
117. Kinsella FA, Zuo J, Inman CF, Pearce H, Maggs L, Eldershaw SE, et al. Mixed chimerism established by hematopoietic stem cell transplantation is maintained by host and donor T regulatory cells. Blood Adv (2019) 3(5):734–43. doi: 10.1182/bloodadvances.2018025502
118. He KM, Ma Y, Wang S, Min WP, Zhong R, Jevnikar A, et al. Donor double-negative Treg promote allogeneic mixed chimerism and tolerance. Eur J Immunol (2007) 37(12):3455–66. doi: 10.1002/eji.200737408
119. Shapiro AJ, Pokrywczynska M, Ricordi C. Clinical pancreatic islet transplantation. Nat Rev Endocrinol (2017) 13(5):268–77. doi: 10.1038/nrendo.2016.178
Keywords: Tregs, islet transplantation, hematopoietic stem cells, mixed chimerism, transplant tolerance
Citation: Pathak S and Meyer EH (2021) Tregs and Mixed Chimerism as Approaches for Tolerance Induction in Islet Transplantation. Front. Immunol. 11:612737. doi: 10.3389/fimmu.2020.612737
Received: 30 September 2020; Accepted: 14 December 2020;
Published: 29 January 2021.
Edited by:
Michaela Lucas, University of Western Australia, AustraliaReviewed by:
Astrid Gertrude Suzanne Van Halteren, Leiden University Medical Center, NetherlandsCopyright © 2021 Pathak and Meyer. This is an open-access article distributed under the terms of the Creative Commons Attribution License (CC BY). The use, distribution or reproduction in other forums is permitted, provided the original author(s) and the copyright owner(s) are credited and that the original publication in this journal is cited, in accordance with accepted academic practice. No use, distribution or reproduction is permitted which does not comply with these terms.
*Correspondence: Everett H. Meyer, ZXZtZXllckBzdGFuZm9yZC5lZHU=
Disclaimer: All claims expressed in this article are solely those of the authors and do not necessarily represent those of their affiliated organizations, or those of the publisher, the editors and the reviewers. Any product that may be evaluated in this article or claim that may be made by its manufacturer is not guaranteed or endorsed by the publisher.
Research integrity at Frontiers
Learn more about the work of our research integrity team to safeguard the quality of each article we publish.