- 1Department of Biochemistry, Microbiology and Immunology, University of Ottawa, Ottawa, ON, Canada
- 2The Ottawa Hospital, Ottawa, ON, Canada
- 3uOttawa Center for Infection, Immunity and Inflammation (CI3), Ottawa, ON, Canada
In December 2019, the novel betacoronavirus Severe Acute Respiratory Disease Coronavirus 2 (SARS-CoV-2) was first detected in Wuhan, China. SARS-CoV-2 has since become a pandemic virus resulting in hundreds of thousands of deaths and deep socioeconomic implications worldwide. In recent months, efforts have been directed towards detecting, tracking, and better understanding human humoral responses to SARS-CoV-2 infection. It has become critical to develop robust and reliable serological assays to characterize the abundance, neutralization efficiency, and duration of antibodies in virus-exposed individuals. Here we review the latest knowledge on humoral immune responses to SARS-CoV-2 infection, along with the benefits and limitations of currently available commercial and laboratory-based serological assays. We also highlight important serological considerations, such as antibody expression levels, stability and neutralization dynamics, as well as cross-reactivity and possible immunological back-boosting by seasonal coronaviruses. The ability to accurately detect, measure and characterize the various antibodies specific to SARS-CoV-2 is necessary for vaccine development, manage risk and exposure for healthcare and at-risk workers, and for monitoring reinfections with genetic variants and new strains of the virus. Having a thorough understanding of the benefits and cautions of standardized serological testing at a community level remains critically important in the design and implementation of future vaccination campaigns, epidemiological models of immunity, and public health measures that rely heavily on up-to-date knowledge of transmission dynamics.
Introduction
In late 2019, a novel betacoronavirus with sustained human-to-human transmission emerged from China’s Hubei Province (1, 2). This new coronavirus was identified as Severe Acute Respiratory Syndrome Coronavirus 2 (SARS-CoV-2) and is currently responsible for the worldwide Coronavirus Disease 2019 (COVID-19) pandemic (3, 4). Currently, a large proportion of the global population remains in various forms of temporary confinement to limit the spread of this virus, leading to significant disruptions in international travel and local socioeconomic activities. Thus, there is a pressing need to better understand the nature and duration of immunity against SARS-CoV-2 infection since nearly all epidemiological models, future vaccination campaigns, and public health measures assume that SARS-CoV-2 convalescence imparts some degree of immunity (5–7). Based on previous serological studies of SARS-CoV (the agent responsible for the 2003 epidemic) and of the Middle East Respiratory Syndrome coronavirus (MERS), neutralizing antibodies are relatively short lived, detectable for approximately three years following infection (8–11). However, the duration of immunity to these specific CoVs is not known. But according to reinfections frequencies by seasonal coronaviruses (sCoVs), this immunity may only last a year (12). Given the global spread and prevalence of SARS-CoV-2, this lethal virus is expected to become endemic (13).
As the pandemic continues its course and convalescent individuals recover, there is an increasing demand to develop validated serological assays that assess the antibody-mediated immunity conferred by a SARS-CoV-2 infection. The utility of serological assays in COVID-19 is manifold. From an epidemiological perspective, a validated serological assay could be used to identify the proportion of individuals exposed to the virus in various populations, such that the evolving disease incidence can be closely monitored. Measuring population seroprevalence can also be used to evaluate the prevalence of asymptomatic transmission and risk factors for acquiring the disease, which remain key research priorities. Furthermore, reliable serological assays are required to determine whether antibody titers, and more importantly neutralizing antibody titers, correlate with sterilizing immunity to SARS-CoV-2. These immunological features could prove to be robust predictors of the efficacy of future vaccines candidates. At the patient level, serological testing can be used as an adjunct to the current PCR-based assays to improve diagnostic sensitivity. Lastly, serological testing will have profound clinical and epidemiological implications by determining the duration and magnitude of immunity conferred by SARS-CoV-2 infection, characterizing the risk of reinfection, and predicting whether a given vaccine will require further boosters (14, 15). Ultimately, accurate serological data will be crucial for understanding the epidemiological and clinical characteristics of COVID-19 that must be established to inform effective and ethical response strategies to the COVID-19 pandemic, especially as policymakers discuss future approaches to resume economic activities and re-open borders.
Serological tests commonly use blood, serum, plasma, or saliva to detect multiple isotypes of circulating antibodies generated by B lymphocytes. Various private, academic, and public health labs are currently developing platforms for SARS-CoV-2 serological testing, utilizing technologies such as classical immunoassays (mostly Enzyme-Linked Immunosorbent Assays; ELISA), chemiluminescent immunoassays (CLIA), flow cytometry-based methods, and various other approaches, all with varying degrees of automation ranging from manual to high-throughput systems (16–20). Furthermore, point-of-care (POC) lateral flow immunochromatographic assays (LFAs) are becoming increasingly popular for their ease of use and rapid detection capabilities (21, 22). Although all serological testing methods share a common function in detecting antibodies against SARS-CoV-2, major differences exist among tests depending on the viral antigens being targeted, the subclass of antibody being detected, and the overall accuracy and reliability.
The urgency to produce serological assays has led to a recent surge in protocols, testing devices, and literature, each with varying degrees of quality and reliability. Here we review current advances in knowledge regarding the antibody response towards SARS-CoV-2 infection. We then look at current commercial and laboratory-based serological assays for SARS-CoV-2 and discuss their strengths and limitations as they relate to cross-reactivity, sensitivity, and specificity. Lastly, we investigate which epidemiological characteristics of COVID-19 may be gleaned from existing serological data, and how these can be applied to public health policy domains such as vaccination, herd immunity modeling, and other public health interventions.
Down to the Basics: Antibody Classes and Class Switching
Multiple classes of antibodies (i.e., IgM, IgA, IgG, and IgE) are involved in antibody-mediated immune responses to viral infections (Figure 1). These classes are characterized by their intrinsic biophysical properties, functions, tissue distributions, and half-lives. Together with IgD, IgM immunoglobulins are normally the first to be expressed during naïve B cell development, comprising the majority of antibodies produced between B cell activation and class switching. IgM represents approximately 10% of all antibodies in the serum (24, 25). IgM antibodies demonstrate a relatively low affinity compared to IgG due to limited affinity maturation through somatic mutations. However, IgM antibodies demonstrate high avidity for the target antigen because they form pentamers that utilize multimeric interactions with the target antigen to facilitate neutralization (25). IgM antibodies are found mostly in circulation where they can facilitate antigen opsonization (26). Recent studies have also revealed diverse roles for secretory IgM in the mucosa of the gastrointestinal and respiratory tracts (27). Human IgA immunoglobulins, which can be further subdivided into the IgA1 and IgA2 subclasses (28), generally exceed levels of IgM in serum and are significantly more present in mucosal surfaces and secretions (i.e., saliva, breast milk, etc.) where they are central to mucosal immunity. IgA immunoglobulins form dimers upon secretion, which contributes to their increased avidity. Although IgA antibodies do not fix complement effectively like IgM, IgA antibodies secreted by plasma cells into the respiratory tract play a key role in mucosal immunity via pathogen neutralization, a process that facilitates aggregation and prevents the initial infection of host cells, thereby conferring sterilizing immunity to a pathogen (29, 30).
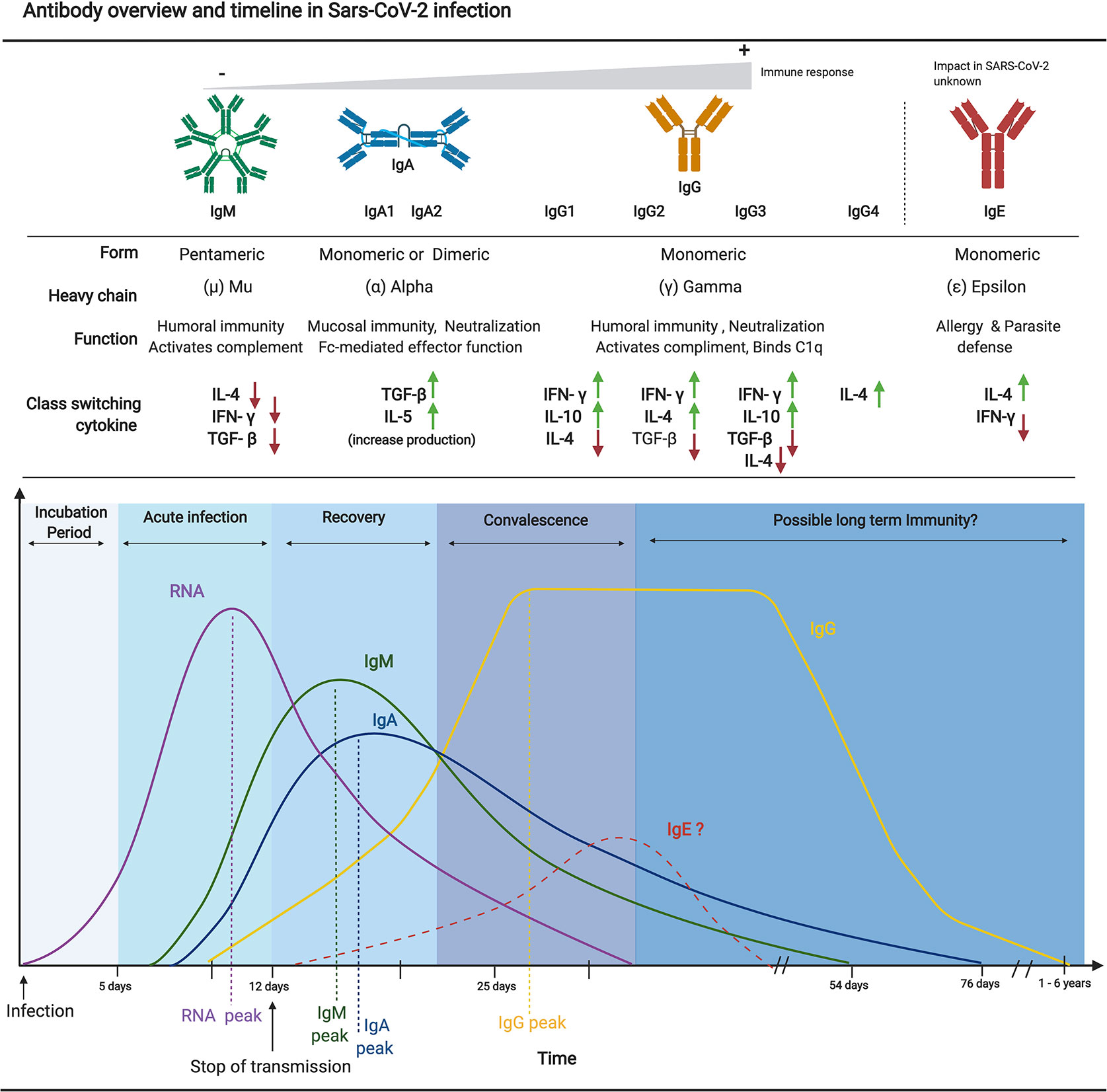
Figure 1 Overview of antibody isotype characteristics and an approximate timeline from SARS-CoV-2 infection to possible immunity. Each antibody isotype is represented with their typical form and associated heavy chain. A brief description of their main function as well as a representation of upregulated and downregulated cytokine necessary for each class switching is also included. The approximate timeline of appearance and subsequent decrease of each isotype in relation to the viral RNA is shown. The curves and values are based on recent serological studies discussed in this review. Since limited literature is available on the implication of IgE in the pathogenesis and antibody mediated immunity to SARS-CoV-2, as such the representation of the IgE timeline is purely hypothetical. Figures were generated using (23).
IgG antibodies start appearing later in the immune response because they undergo affinity maturation through somatic mutations, resulting in high affinity for the target antigen and a heightened capacity to neutralize pathogens (31). In addition to their role in neutralizing antigen, IgG antibodies also have other critically important roles, most notably Fc-mediated effector functions such as cell activations and antibody-dependent cellular cytotoxicity (ADCC) (32–34). IgG immunoglobulins are monomeric and represent about 75% of all antibodies in serum. They are associated with lasting immunity given their long half-life in blood and association with differentiated memory B cells (25). IgG can also bind C1q, activating the classical complement pathway of the innate immune system (35).
IgG antibodies can be subdivided into multiple subtypes (i.e., IgG1, IgG2, IgG3, and IgG4), each with slightly different roles in humoral immunity (32). For example, IgG1, IgG3, and occasionally IgG4 (upon repeated exposure) are secreted in response to protein antigens, while IgG2 almost solely responds to polysaccharide antigens (32). Given that different pathogens elicit different ratios of IgG subtypes, these can be used as characteristic profiles for monitoring the efficacy of vaccine designs with regards to correlates of protection (36, 37). Finally, IgE antibodies predominately mediate allergic reactions and immune responses against parasitic infections and comprise less than 0.01% of all total antibodies. IgE antibodies are monomeric and demonstrate a strong affinity for FcϵRI receptors expressed on numerous innate immune cells (e.g., mast cells, basophils, eosinophils), allowing for the generation of a generalized inflammatory response through innate immune system activation (38).
Current published data support that SARS-CoV-2 induces a classic viral response pattern, where IgM is the first isotype to appear, followed closely by IgA which peaks at 2-3 weeks post-symptom onset (PSO) before declining, and finally with IgG antibodies that remain detectable for several months PSO (39, 40). However, some studies have also reported the detection of virus-specific IgA responses preceding that of IgM, although the implications of this new pattern are not entirely understood (39, 41). Of particular interest, detectable levels of neutralizing antibodies against SARS-CoV-2 have been shown to start declining within three months of infection, especially among mild and asymptomatic cases (42–45). This, however, is not uncommon and resembles findings from patients infected with sCoVs (12, 46). Given the frequency of reinfections with sCoVs, this observation is likely a predictor of impermanent immunity and of heightened risk of reinfection in the short term.
SARS-CoV-2 Viral Antigens
The effectiveness of an antibody response is largely dependent on the capacity of antibodies raised against native viral antigens during a natural infection, or against antigens in a vaccine, to act when exposed to the virus. These antibodies can either be present in blood, or produced de novo by memory B cells and plasma cells upon re-exposure to the viral antigens (47). Antibodies play a direct role in neutralizing incoming virus to prevent reinfections (i.e., sterilizing immunity), or by tagging viral antigens expressed on the surface of infected cells thereby triggering downstream Fc effector functions. In the case of CoVs, the viral antigens to which most antibodies are directed against are the viral spike (S) and nucleocapsid (N) proteins (48, 49).
The SARS-CoV-2 S protein is a trimeric transmembrane glycoprotein that is exposed on the surface of virions and mediates viral entry into host cells (Figure 2A) (50). This S protein constitutes the primary target of all current leading vaccine candidates (51). This large, exposed protein is readily targeted by neutralizing antibodies, which indirectly creates selective pressure for the emergence of evasion mutations. The propensity for S to mutate may limit its future use in serological assays and vaccines, as antibodies directed against the current variant may not bind emerging mutated epitopes, resulting in reduced vaccine efficacy while producing more false negatives in serological assays.
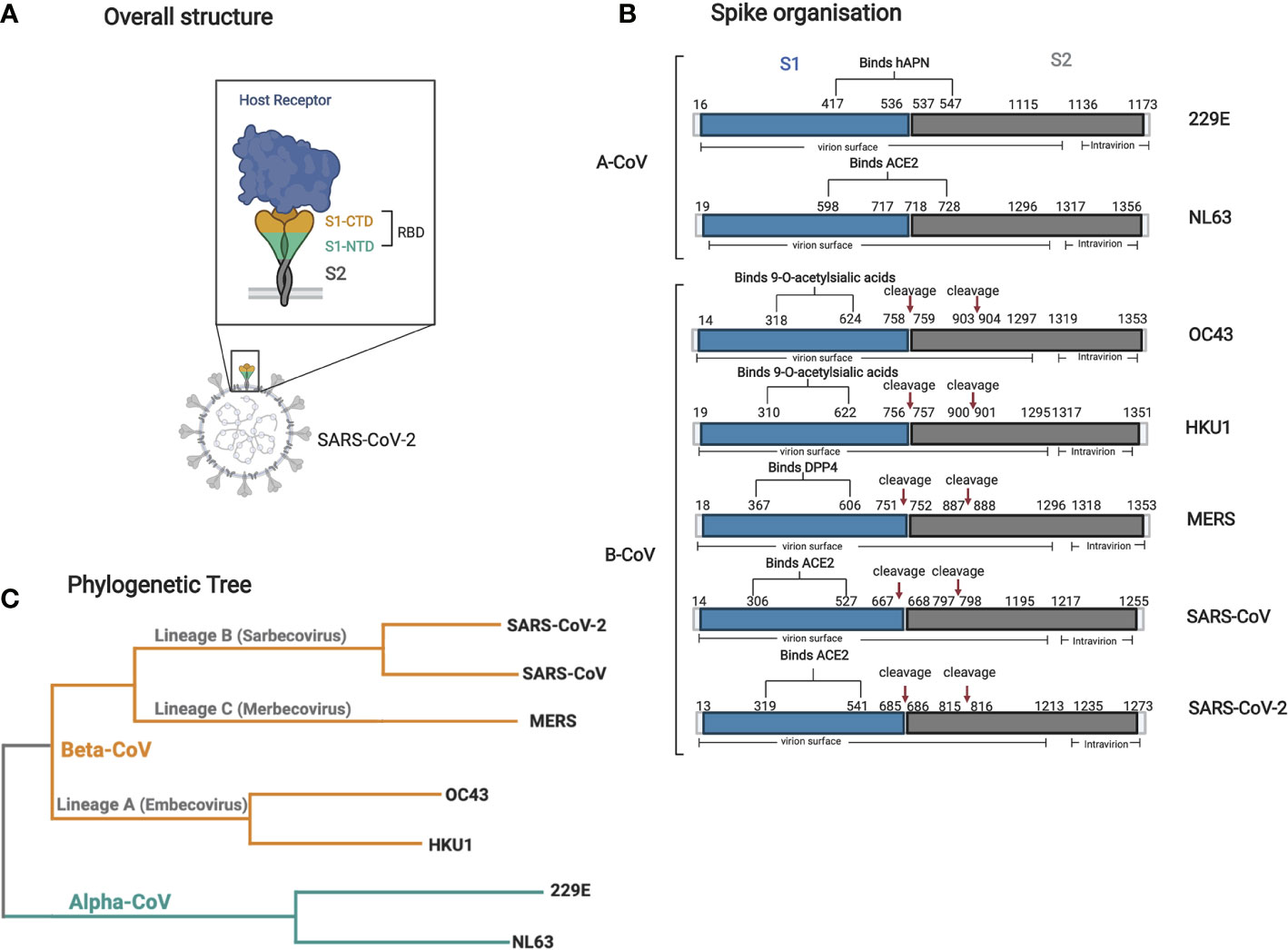
Figure 2 Structure and organization of the spike glycoprotein and phylogenetic tree of all seven human CoVs. (A) A cartoon structure of the Spike protein and its receptor (i.e., ACE2) is shown in relation to its localization on the virion surface. The S1 domain interacts directly with the receptor through its RBD via it’s C-terminal domain (CTD). (B) Graphical representation of the various spike human CoV proteins. The RBD, S1 (blue), and S2 (gray) domain locations and all other relevant sites (cleavage sites), and other topological features are shown with their respective amino acid sequence number. The information for each spike was obtained using Uniprot with the following accession numbers: 229E P15423, NL63 Q6Q1S2, HKU1 Q0ZME7, OC43 P36334, MERS K9N5Q8, SARS-CoV P59594, SARS-CoV-2 P0DTC2. (C) A phylogenetic tree based on the complete genome of all seven human CoVs was made using Clustal Omega multiple alignment tool using the reference genome sequenced from NCBI with the following accession numbers: 229E NC002645.1, NL63 NC005831.2, HKU1 NC006577.2, OC43 NC006213.1, MERS NC019843.3, SARS-CoV NC_004718.3, SARS-CoV-2 NC_045512.2. Figures were generated using (23).
The S protein is further divided into two functional subunits, S1 and S2. S1 is responsible for binding to the host cell surface receptor ACE2 through its receptor-binding domain (RBD) found within subunit S1-, while S2 is involved in the fusion between the viral envelope and cellular membranes upon attachment (52) (Figure 2B). Along with orchestrating viral entry into host cells, the RBD region of S1 is of specific importance as many antibodies raised against RBD have neutralizing potential. Indeed, numerous viral epitopes that that are targeted by neutralizing antibodies are located within this region (53–57).
The viral N protein is an abundant nucleoprotein that binds the viral RNA genome and is contained within the virion. Each N protein contains three highly conserved and distinct regions: an N-terminal RNA-binding domain, a central Serine/Arginine-rich linker, and a C-terminal dimerization domain (58). The N protein has many functions associated with viral RNA packaging, RNA transcription, and viral replication. Since the N protein is abundantly expressed during infection, it is capable of inducing high levels of antibody production, making it a suitable target for serological assays (59–62). However, given that the N protein is not involved in viral entry and is shielded from antibodies by the viral envelope, most N protein antibodies are not likely to be neutralizing (63, 64). This was demonstrated by one study which showed that immunization with the SARS-CoV N protein induced antibodies with undetectable neutralizing activity (65).
Testing for SARS-CoV-2 Antibodies
Serological tests are designed to detect the presence of antibodies against a given pathogen, in this case, SARS-CoV-2. A positive serological test result is indicative of a past exposure to one or several of the pathogen’s antigenic epitopes and therefore is not an indicator of an active infection. Furthermore, if the pathogen of interest shares antigenic epitope sequences with the proteins of other microbes or even that of vaccine antigens, a test can be reported as falsely positive. During a natural infection by SARS-CoV-2, the levels of viral RNA rapidly decrease during the second week and may become undetectable (66–68). Antibodies therefore become the primary and most accurate modality to detect a recently resolved or past infection (Figure 1). Serological tests are also critical for the detection of asymptomatic and previously undiagnosed infections in the population. This information is essential to guide public health interventions during an epidemic to mitigate the spread of a pathogen to the most vulnerable members of the population.
Serological tests can be broadly divided into two categories: Rapid Diagnostic Tests (RDTs) and non-rapid tests (Figure 3) (49). A list of clinical serological tests currently approved are presented in Table S1. RDTs are most commonly LFAs which detect the presence of antibodies against multiple SARS-CoV-2 antigens within a 30-min time window. LFAs for SARS-CoV-2 detection work through the addition of a liquid sample (e.g., blood or saliva) – potentially containing the target antibodies – to one side of the testing device. The sample then diffuses by capillary action to a conjugation pad, where viral antigens conjugated to a colorimetric detection molecule (e.g., colloidal gold) are deposited. If SARS-CoV-2 antibodies are present, they will capture and dislodge these antigens and then migrate by continued capillary flow to a nitrocellulose membrane where anti-human capture antibodies are immobilized, usually anti-IgG and anti-IgM. If anti-SARS-CoV-2 antibodies are present, the colloidal gold (or another detection agent) will accumulate on a thin strip of anti-IgG and anti-IgM to create a colored line (Figure 3) (69). LFAs do not require multiple steps, nor the addition of any solution other than the patient sample. LFAs provide fast, qualitative, and easy-to-understand readouts that are designed for usage at home or in a POC setting without the need for equipment (70). Drawbacks of LFAs include their higher cost-per-test rate, their inability to analyze multiple samples simultaneously, their general lack of quantitative data, and importantly, a several-fold reduced sensitivity when compared to non-rapid testing methods (71, 72). Although RDTs are theoretically ideal for POC usage, recent studies have demonstrated that many newly developed RDTs for SARS-CoV-2 have failed to meet the necessary standards for sensitivity and specificity when compared to non-rapid testing (71–76). Therefore, for research purposes, LFAs are not the ideal choice.
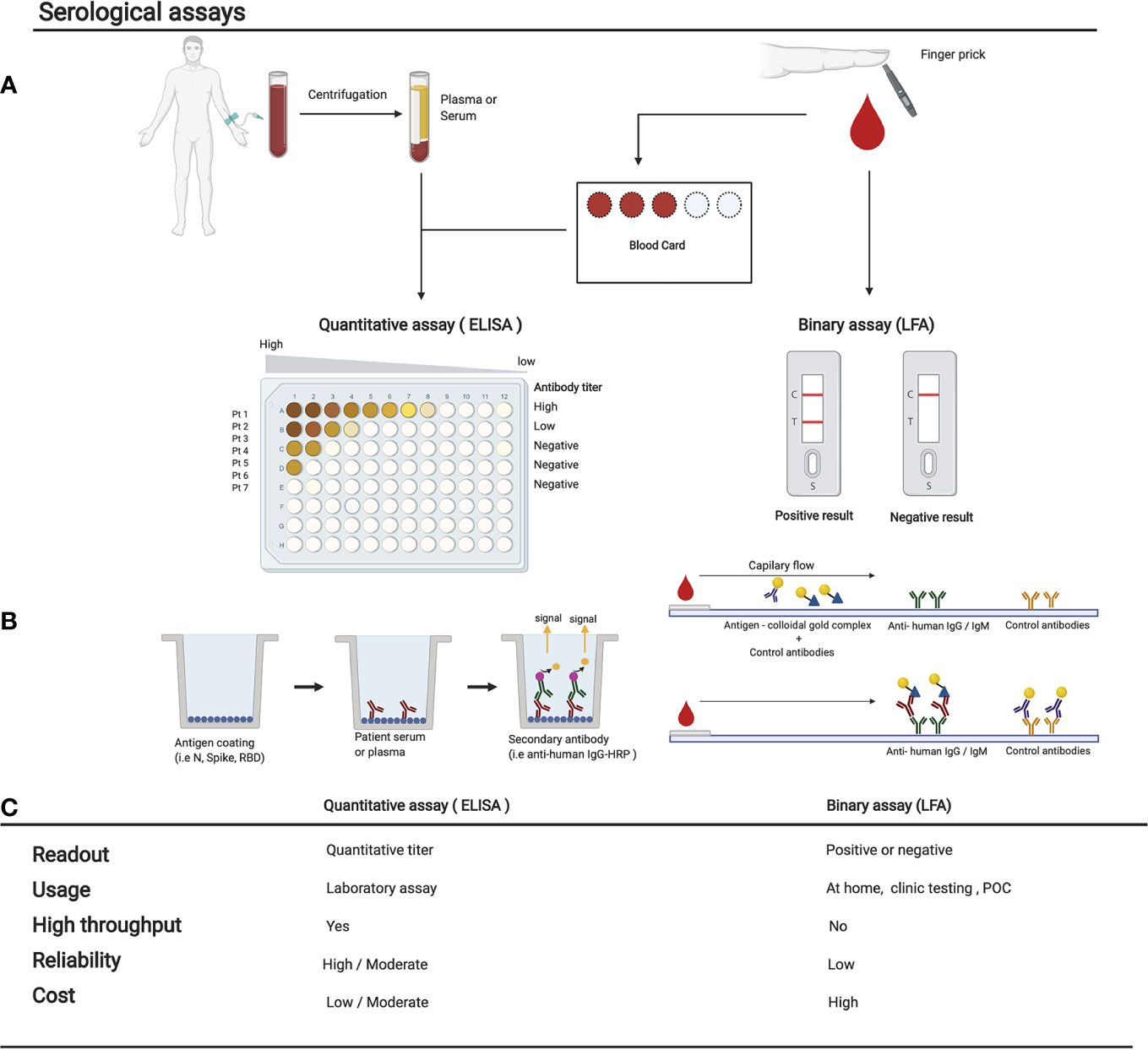
Figure 3 Comparison of various serological assays. (A) The sampling method and subsequent treatment of the blood before performing the serological assay is shown. Either a tube of blood is collected to isolate serum/plasma, or blood from a finger prick is used to fill a dried blood spot card or used directly in a LFA. Here we show the 2 main types of serological assays: on the left, a quantitative ELISA, or on the right, a binary result LFA. (B) The experimental procedure of each test is shown in their most simple form. Many variations are now available and are being used (see Table S1). (C) Comparison of the strengths and weaknesses of each method. POC, point of care; LFA, lateral flow assay. Figures were generated using (23).
Non-rapid serological testing methods include solid-phase immunoassays, microarrays, viral neutralizing tests, bead-based flow cytometry-based methods, and immunofluorescent microscopy, among others. These are all primarily laboratory tests that are carried out by trained personnel. Solid-phase immunoassays including ELISA, CLIA, Electrochemiluminescence Immunoassay (ECLIA), Enzyme-linked Fluorescent Assay (ELFA), and Dried Blood Spot ELISA (DBS-ELISA) are currently the most commonly used non-rapid, high-throughput methods for the detection of SARS-CoV-2 antibodies within a population (49).
Non-rapid tests generally involve the capture of primary SARS-CoV-2 antibodies within a saliva or blood sample to a solid support, like a dish or plate, coated with a SARS-CoV-2 antigen. This is followed by an initial wash step and the addition of a detection antibody, which is usually conjugated to a fluorophore or an enzyme like the horse radish peroxidase (HRP). Excess antibody is washed off and detection is performed. Colorimetric, fluorescent, and/or luminescent methods can be used as the final detection method depending on the detection antibody conjugate. Throughput can be easily scaled up using robotic liquid handlers.
Unlike LFAs, non-rapid tests can also provide valuable information on the quantity of each antibody within the samples (77). Non-rapid methods are generally much more sensitive than RDTs. Low-level antibody detection is especially important in the first 7 to 21 days of SARS-CoV-2 infection, when IgG levels are still rising, as well as >2 weeks post-infection when IgM antibodies begin to diminish (Figure 1) (67). Although most non-rapid tests currently use venous serum or plasma, or saliva samples in a liquid phase, DBS-ELISAs provide a practical alternative to venous samples by using only a few microliters of blood taken by pinprick and deposited onto an absorbent paper (Figure 3). Antibodies can then be eluted from a circular punch taken from the paper using a small amount of buffer. The ELISA is then performed in a standard fashion. This simple and convenient approach to collecting blood eliminates the need for a healthcare provider to perform venipuncture and provides an opportunity to conduct large-scale population-level seroprevalence studies using high throughput liquid handlers to perform DBS-ELISAs (78).
Neutralization Assays and Their Importance
Although serological tests can determine prior immune exposure to SARS-CoV-2, neutralization assays provide critical knowledge on whether the detected antibodies are capable of neutralizing the virus and providing likely protection upon subsequent exposure. Currently, the most reliable neutralization assays involve live authentic SARS-CoV-2 viruses produced in cell culture and therefore require all procedures to be carried out in a Biosafety Level 3 (BSL3) facility (79). A current challenge is to develop a reliable neutralization assay that can be carried out in a standard BSL2 laboratory, at home, or in the clinic. To circumvent biosafety containment requirements, researchers are currently developing lab-based assays using viruses that consist of a less-harmful or non-infectious virus, such as murine leukemia virus (MLV) or vesicular stomatitis virus (VSV), pseudotyped with the SARS-CoV-2 S glycoprotein (80, 81). Other options include assays that utilize purified ACE2 to determine the effect of neutralizing antibodies on the ACE2-Spike interaction without the requirement of live cells or viruses. One example of a neutralization assay is the cPass SARS-CoV-2 Neutralization Antibody Detection Kit from GenScript Biotech. This test kit is advertised to test for pan-Ig neutralizing antibodies using the SARS-CoV-2 RBD as the viral antigen for antibody capture (82). Other promising surrogate neutralization assays have been proposed which utilize an ELISA-based competition binding assay against ACE2 (83, 84). Antibodies against RBD have been shown to be the primary source of neutralizing antibodies against the virus (53, 85–88). However, it should be noted that not all antibodies that bind RBD demonstrate neutralization potential, and that anti-RBD antibodies capable of neutralization may only be present at very low concentrations in some individuals post-infection (89, 90). Furthermore, RBD is not the only viral antigen that is a target for antibody-mediated neutralization; additional non-RBD epitopes elsewhere on the S protein have also been shown to neutralize the virus when targeted by antibodies (57, 91, 92). One caveat of the aforementioned neutralization assays is that they provide limited information on possible Fc-dependent effector functions, which likely also play an important role in protecting against SARS-CoV-2 (93). Therefore, current single-antigen neutralization assays only detect a subset of the total pool of neutralizing antibodies.
Of note, several commercial serology tests use the N antigen for antibody capture (Table S1). Given the high abundance of anti-N antibodies, targeting this viral antigen has the potential to increase test sensitivity (94). However, N proteins are located on the interior surface of intact viruses, and thus remain inaccessible to circulating antibodies. Therefore, tests that use N are unlikely to identify neutralizing antibodies that provide sterilizing immunity upon infection. Nevertheless, effector functions of anti-N antibodies could still provide protection (64). Currently, the RBD and S proteins are the most reliable antigens for measuring the abundance of neutralizing antibodies.
Sensitivity and Specificity of Serological Assays for SARS-CoV-2
The quality and usefulness of a serological test is primarily evaluated by its degree of sensitivity and specificity (95). Sensitivity describes the ability of a serological test to provide a positive result from samples that contain antibodies against SARS-CoV-2 (“true positives”). Thus, a highly sensitive test would have a very low frequency of false negatives. Meanwhile, specificity describes the ability of a test to provide a negative result when a sample does not contain SARS-CoV-2 antibodies. Thus, a high specificity SARS-CoV-2 serological assay would have no or few false positives, including those resulting from cross-reactivity to any of the other six human CoVs (96, 97). The sensitivity and specificity of an assay are influenced by the cut-off point at which a test result is deemed positive. A receiver operating characteristic (ROC) curve is a useful graphical tool to visualize the relationship and trade-off between the sensitivity and specificity of an assay (98), but its details are beyond the scope of this review.
Serological tests that target IgM, which naturally has a lower affinity for the viral antigen than IgG, will be at a higher risk of producing false positives, and therefore should require a higher specificity threshold. Testing thresholds for specificity and sensitivity are arbitrary values established experimentally and differ between serological tests and methods. SARS-CoV-2 thresholds are primarily determined based on test results of negative control samples collected prior to the pandemic, as well as on positive control samples that have been confirmed by a certified clinical RT-PCR diagnostic test (99). Currently, there are no international reference standards for reporting test sensitivity and specificity, making it very challenging to compare the different serological tests and assays without carrying out a direct experimental comparison. Recent studies have sought to compare multiple testing kits with a small group of common samples (100–102). While this represents progress, what is ultimately required is a well-characterized set of standard sera that could be tested against any approved serology testing kit, allowing for the sensitivity and specificity of these kits to be compared (103). Other variables that require standardization for serological testing and kit comparisons include the length of time PSO for samples to be collected from patients, since the sensitivity and specificity of commercial tests can differ depending on the time at which the sample is collected (104), as well as the method by which samples are sometimes inactivated for lab safety (97, 105).
Sensitivity and specificity thresholds are also important for epidemiological considerations unique to certain situations and environments. These thresholds can be altered to allow for greater sensitivity of testing at the expense of specificity, or the opposite, whereby specificity is favored at the expense of sensitivity. For example, in a region with high SARS-CoV-2 seroprevalence, sensitivity may be prioritized over specificity to ensure the majority of positive cases are identified. The opposite is also true in low-prevalence regions (16). If the prevalence in a given region is very rare, then higher specificity and relatively lower sensitivity would be favored so that fewer patients would have false-positive results while still detecting the majority of true positives. It is essential to have the correct balance between sensitivity and specificity, as the epidemiological implications of disproportionate false negatives or false positives can be profound. A test with too many false positives will keep people isolated for longer than necessary, creating otherwise avoidable social and economic strains. A test with too many false negatives will result in the underestimation of disease prevalence, which may lead to a premature easing of disease containment policies and resurgent waves of infection as misidentified patients unknowingly continue to transmit the disease (16).
Dynamics of the Antibody-Mediated Immune Response to SARS-CoV-2
Understanding the temporal profile by which circulating antibody classes are produced following SARS-CoV-2 infection is essential for the interpretation and clinical application of serological test results. During a viral infection, plasma B lymphocytes of the adaptive immune system produce different classes of antibodies in response to temporally regulated cytokine expression in a process called class switching or class switch recombination (CSR) (106). A given infecting pathogen type normally induces a characteristic cytokine profile that is responsible for triggering CSR for the production of the various isotypes and subtypes that are optimally suited to neutralize that type of microbe. Individuals suffering from severe COVID-19 are known to exhibit a dysregulation of pro-inflammatory cytokine release, also known as a cytokine storm (107–110). How this large release in cytokines alters the humoral response compared to asymptomatic, mild or moderate COVID-19 cases that do not exhibit this same cytokine storm is not yet clear.
While it is expected that IgM immunoglobulins are the first class detected following infection by SARS-CoV-2, as supported by a number of studies (37, 39, 40, 111), others have paradoxically demonstrated IgG responses that precede the IgM response (38, 112–115). This surprising discrepancy is likely related to cross-reactivity with pre-existing immunity to sCoVs (40, 111, 116–118). Nevertheless, the largest body of evidence suggests that nearly all SARS-CoV-2 infected individuals begin to produce IgM, IgA, and then IgG by 1 to 2 weeks PSO (Figure 1) (39, 90, 114, 119, 120). In fact, IgM antibodies against the viral N protein have been detected as early as 1 to 7 days PSO in 85% of individuals (39). However, these figures vary considerably depending on the type of serological assay and target antigen used. For example, Long et al. detected IgM antibodies against N protein in only 12% to 40% of cases during this same time period (i.e., 1–7 days) (121). The majority of patients appeared to have seroconverted by day 14 PSO, with approximately 94% of infected individuals having detectable levels of IgM against the RBD of the SARS-CoV-2 S protein, and 88% against the N protein (114, 122). IgM antibodies decline rapidly at approximately 20 days PSO, becoming undetectable at 60 days PSO on average (Figure 1) (123, 124). The impermanence of the IgM response suggests that the diagnostic role for IgM serology is most relevant in the detection of current and recent infections, within the first 1 to 2 weeks PSO, at which point its sensitivity for the diagnostic of an active infection may actually exceed that of PCR (39, 125, 126).
IgG immunoglobulins broadly have the most significant implications with respect to serological testing and antibody responses, given its high affinity for the antigen, capacity for viral neutralization, ability to activate complement, and predominant role in long-term immunity following infection or vaccination. Indeed, serological studies on other human CoVs including SARS-CoV and MERS have found the IgG antibody class to yield assays with greater specificity compared to IgM, to be significantly longer-lasting in comparison to IgM and IgA (127, 128), and to have strong links to neutralization and patient outcomes (114, 122). During acute SARS-CoV-2 infection, class switching from IgM to IgG occurs relatively quickly, with a median time to IgG detection ranging from as early as 7 days (120, 121) to approximately 14 days (39, 125, 129). IgG production also peaks later and is much slower to decrease than IgM (124, 130). The duration and intensity of the reported IgG antibody response for SARS-CoV-2 varies according to several study parameters that include disease severity and outcome, and antigens used in the serology assays. One study demonstrated an important reduction in IgG over 8 weeks in both symptomatic and asymptomatic cases, with many patients becoming seronegative during the study period (40% asymptomatic, 12.9% symptomatic) (121). Such observations are also supported by a number of additional studies that also measured a decline of IgG antibodies after several weeks PSO (44, 131). However, most groups have demonstrated that IgG levels against SARS-CoV-2 remained relatively stable within a 3 to 5 month observation period PSO (43, 124, 130–135).
Interestingly, among individuals infected by SARS-CoV-2, detectable antibody subtypes included RBD-specific IgG1 & IgG3, but rarely IgG2 or IgG4 (136). If a consistent subtype ratio is reliably established, this could help in identifying true seropositive individuals (convalescent from SARS-CoV-2) as opposed to false-positives with cross-reactive antibodies (convalescent from other sCoVs). Similar to IgM, IgA antibodies are produced shortly after PSO, with a median time to detection of 1 to 2 weeks PSO (39, 40, 67, 128). However, while IgM peaks at approximately 10 to 12 days, IgA levels appear to be relatively more persistent, peaking at approximately 20 to 30 days PSO (39, 123, 124, 133). To date, there is no serological evidence for the induction of IgE production in patients with COVID-19.
Duration of SARS-CoV-2 Neutralizing Antibodies
Nearly all individuals who become infected with SARS-CoV-2 develop antibodies and neutralizing antibodies following infection, demonstrating a successful adaptive antibody-mediated immune response (43, 45, 87, 88, 110, 121, 123, 124, 130, 134, 137). This is consistent with non-human primate (NHP) studies where exposure to the live virus provided protection against reinfection without clinical illness, with corresponding neutralizing antibody responses (138, 139). Therefore, regardless of the discrepancies between studies regarding persistence or decline of total antibodies, it is imperative to note that persistence of IgG antibodies does not necessarily imply persistence of neutralizing antibodies during this same period. In fact, most studies report various intensities of decline in neutralizing antibodies after three months PSO, with disease severity being a factor strongly correlating with the decay rate of neutralization (42–44, 90, 121, 124, 140).
In contrast, data from some studies have indicated that neutralizing antibody titers remain stable ranging from 75 days to 6 months PSO in COVID-19 convalescent individuals with a broad spectrum of disease severity (130, 134, 141, 142). In particular, a large cohort study by Wajnberg et al. analyzed humoral responses in 30,032 antibody-positive individuals in New York City, and demonstrated relatively stable anti-S IgG antibody titers over five months, with these titers correlating with virus neutralization (130). Similar findings were demonstrated in a convalescent cohort study in China over a six month follow-up period with anti-S and anti-N IgG antibodies detectable in 70% of patients, with associated stability in neutralization titers, although these results are yet to be peer-reviewed (141).
One factor that could influence the persistence of neutralizing antibodies within specific cohorts is a high prevalence of the virus in a defined geographical region or in a specific subpopulation of individuals such as frontline healthcare workers. Regular re-exposure to the virus may help sustain higher antibody and neutralizing antibody levels. A second factor may be the persistence of antigens in tissues or as immune complexes on follicular dendritic cells. In fact, new evidence suggests that memory B cell responses continue to evolve in recovered individuals for at least six months after infection (143). During this time, somatic mutations accumulate to produce neutralizing antibodies with increased potency. This suggests that regardless of whether neutralizing antibodies wane over time, re-exposure to the virus is likely to stimulate memory B cells to mount a rapid and effective humoral response.
Taken together, while the specific conditions that influence the total duration of SARS-CoV-2 humoral immunity remain to be more precisely defined, decreasing antibody titers do not necessarily imply waning or defective immunity. In fact, antibody titers are expected to decrease following the resolution of an acute infection as a natural consequence of the depletion of short-lived plasma cells when immediate and sustained immune responses are no longer necessary (144, 145). Furthermore, the half-life of IgG in serum is about 26 days (146). Without continuous antibody output from plasma cells, antigen-specific antibodies will naturally decline. As such, while more severe COVID-19 symptoms may elicit longer protection for convalescent individuals, it is plausible that milder symptoms may provide much shorter windows of sterilizing immunity. However, it is established knowledge that adaptive immune responses rely on immunological memory from both B cells and T cells to not only prevent reinfections but also diminish disease severity; this is also the basis of vaccination.
Antibody-Dependent Enhancement (ADE)
Evidence demonstrating a positive association between high antibody titers and increased clinical severity of COVID-19 has raised the possibility that antibody-dependent enhancement (ADE) could, in some instances, contribute to an excessive immune response that exacerbates SARS-CoV-2 pathogenesis (123, 125, 147–149). ADE is a process in which antibodies bind to viruses to form virus-antibody complexes which potentiates and facilitates host cell entry via cell surface Fc receptors, causing infection of Fc-expressing cells such as B cells, dendritic cells, macrophages, and monocytes. Cellular Fc receptors bind to the constant region of antibodies that define the isotype (e.g., Fcγ receptors bind IgG). ADE has been shown to cause increased pathogenicity of some viruses such as Dengue virus, Ebola virus, and Zika virus (150–152). ADE has also been observed in certain human CoV challenges in immunized animals. These include MERS as well as SARS-CoV, where anti-S protein antibodies have potentiated viral entry via an ACE2 receptor-dependent mechanism, or independently of ACE2 by facilitating virus uptake via FcγRII (153–157). ADE has been observed to induce pro-inflammatory cytokine release from Fc-expressing immune cells in mice and NHPs (157–159). While there is no direct evidence yet to support this hypothesis in the context of COVID-19, the biphasic course of infection that has been described, in which severe hypoxia and respiratory distress typically manifest 7 to 14 days after onset of fever and viremia, coincides with the chronology of seroconversion and IgG class switching (160). Fortunately, animal studies thus far in immunized NHPs re-challenged with SARS-CoV-2 have not shown signs of ADE (149). However, these studies were limited to small numbers of animals and more studies are needed to understand if these animal models can successfully be used to understand ADE in humans. Furthermore, there have been two large-cohort studies published to date on the use of convalescent plasma in human patients, both deeming the incidence of serious adverse events to be low (161, 162). Cumulatively, this would suggest that ADE is unlikely to be a major cause of pathogenesis in SARS-CoV-2 infection in humans. Nevertheless, ADE should be investigated further as it could impact the efficacy and safety of serum therapy, as well as vaccination programs. In particular, if future vaccine candidates are to require booster shots because of impermanent immunity, ADE must be considered as repeated doses generate an increase in antibodies that could potentially contribute to ADE upon virus exposure.
Cross-Reactivity of SARS-CoV-2 Antibodies to Seasonal CoVs
A significant challenge in developing a specific SARS-CoV-2 serological assay is the potential for cross-reactivity of SARS-CoV-2 capture antigens with antibodies against other human CoVs (163). SARS-CoV-2 shares amino acid sequences and antigenic T and B cell epitopes with the highly prevalent sCoVs that cause the common cold including 229E, OC43, NL63, and HKU1, and also with the now rare MERS and extinct SARS-CoV that both cause severe and fatal respiratory disease (Figure 2) (62, 164–168). A recent prevalence survey of the sCoVs using RT-PCR revealed that OC43 is the most prevalent sCoV followed by NL63, HKU1, and finally 229E (169). While infection with the sCoVs induce antibody responses as would be expected, these wane over time and render the hosts susceptible to reinfection. An impressive study of the occurrence of reinfection for all four sCoVs over more than a 35 year span revealed that reinfections with the same sCoV occurred most frequently after 12 months (12). While sterilizing immunity to sCoVs is relatively short-lived, here we will review current knowledge about cross-reactivity of these antibodies to SARS-CoV-2 antigens.
Given that the prevalence of antibodies against all four sCoVs may be as high as 90%, as demonstrated in one sample of American adults (170), antigens used in SARS-CoV-2 serological assays may in some instances be detected by these naturally circulating and highly prevalent antibodies, thereby limiting test specificity and creating the potential for false-positive results. While the greatest probability for cross-reactivity exists between antibodies directed against SARS-CoV-2, SARS-CoV, and MERS, the latter two are exceedingly rare given the low case numbers of these infections (171, 172). Therefore, issues related to cross-reactivity between SARS-CoV-2 and the circulating sCoVs are of foremost concern.
Several serological studies have demonstrated cross-reactivity of SARS-CoV-2 S protein with SARS-CoV, MERS, and sCoVs (43, 53, 116, 118, 165, 173–175). The spike S2 domain is believed to be primarily responsible for this cross-recognition given its slightly higher level of sequence similarity than the other S domains (118). When comparing the amino acid sequence by both percent identity and percent similarity of all human CoVs, the S2 domain has the highest identity and similarity compared to full S, S1, RBD, or N domains (Tables 1 and 2) (173). Cross-reactivity between SARS-CoV-2 antibodies and the S2 domain of the SARS-CoV S protein has also been shown (176). Some studies examined the specificity of ELISAs and demonstrated cross-reactivity of sCoVs, MERS, and SARS-CoV sera only with the full SARS-CoV-2 S protein, and not with the S1 antigen (53, 173), which is in agreement with several other groups that failed to measure cross-reactivity of sCoV antibodies with SARS-CoV-2 RBD (62, 134, 176). Importantly, the lack of cross-reactivity demonstrated between the S1 subdomain of SARS-CoV-2 and sCoVs antibodies may point to its potential application as a target antigen for highly specific serological assays.
There may also be cross-reactivity issues that affect the specificity of tests that use the N protein. The N protein of SARS-CoV-2 shows 97% similarity to that of SARS-CoV, 75% to MERS, and 58% to 65% similarity to the sCoVs (Table 2). One previous study analyzed the cross-reactivity of the N protein between the various CoV groups (177). They found that antibodies against the seasonal alphacoronaviruses 229E and NL63 demonstrated cross-reactivity towards each other but did not cross-react with betacoronavirus antigens, which would include SARS-CoV-2. Meanwhile, betacoronavirus (NL63 and OC43) sera primarily cross-reacted with N proteins from other betacoronaviruses, with the exception of SARS-CoV (177). While the SARS-CoV-2 N antigen was not included in the study, this suggests that it is likely that other betacoronaviruses would similarly cross-react with SARS-CoV-2. Indeed, Ng et al. also showed that sCoV-reactive serum could bind to the SARS-CoV-2 N protein in their flow cytometry-based detection assay (20). Therefore, there is also the potential for cross-reactivity between pre-existing antibodies towards sCoVs and the N antigen of SARS-CoV-2. While the high abundance of the N protein otherwise makes it a promising candidate for diagnostic serological assays, the potential for poor specificity due to cross-reactivity with prevalent sCoVs may be a critical limitation to its use. Indeed, in a pre-print manuscript, Anderson et al. demonstrated in a cohort of 207 pre-pandemic samples that 5% reacted to the SARS-CoV-2 S proteins, 2% against the RBD, and 19% against N (116). A closer analysis of these samples revealed that most had antibodies against OC43, 229E, and NL63 but these were non-neutralizing.
Protection From SARS-CoV-2 Infections by sCoVs Antibodies
While there is strong evidence that antibodies raised against sCoVs antigens can bind to SARS-CoV-2 proteins and interfere with serological assays, there is conflicting information concerning the protective role of these sCoV antibodies. While most neutralizing antibodies target the RBD to disrupt binding to the host-expressed ACE2 receptor (61, 178, 179), cross-reactive sCoVs primarily target the S2 domain of the SARS-CoV-2 spike protein (118, 167). Furthermore, non-RBD S1 as well as S2-binding neutralizing antibodies have been identified for both SARS-CoV and SARS-CoV-2 (92, 165, 180–182). As of this moment, two studies have shown evidence that the presence of sCoV antibodies is associated with less severe COVID-19 symptoms (183, 184), while two more have shown some neutralizing activity in pre-pandemic samples (118, 165).
More specifically, Ng et al. showed that healthy individuals with recent sCoV exposure had antibodies capable of limiting SARS-CoV-2 entry into host cells in an experimental system (118). It has been proposed that the protection conferred by sCoV antibodies may also contribute to the age disparity in COVID-19 susceptibility (118, 185). Seroprevalence of sCoVs varies considerably between age groups, with especially high prevalence in very young children (<1 year of age), an observation that aligns well with the fewer number of severe cases of COVID-19 in children (186, 187). The high prevalence of protective sCoV antibodies in younger individuals provides a plausible explanation for why young adults under the age of 20 are estimated to be half as susceptible to COVID-19 as those above the age of 20, and why children comprise only 21% of symptomatic cases, compared to upwards of 69% for those above 70 years of age (188). This hypothesis, however, remains to be tested in a well-designed randomized clinical trial.
However, mounting evidence support that few to no cross-neutralizing sCoV antibodies do in fact exist (116, 117). Nevertheless, one must be cognizant that most neutralizing assays utilize spike-pseudotyped viruses or surrogate (virus-free) assays with purified antigen. It is possible that such experimental systems fail to measure the overall protection of sCoV pre-exposure as seen in a living person. Indeed, sCoV-induced cross-reactive T cell responses and Fc effector functions of antibodies may also play a role in COVID-19 severity and outcomes (62, 164, 166, 168).
Immunological Back-Boosting by sCoV Antibodies and the Original Antigenic Sin
As described above, cross-reactivity of sCoVs antibodies to SARS-CoV-2 antigens has now been well characterized. It is also well established that most people have had prior exposure and produce antibodies to several sCoVs. While there is some evidence that sCoV antibodies can neutralize SARS-CoV-2, so far neutralization appears to be weak if at all detectable. Given that most cross-reactive antibodies bind the S2 region of the SARS-CoV-2 spike and are non-neutralizing, a very interesting an important question arises: can this cross-recognition of antigens give rise to immunological imprinting?
Immunological imprinting, also called original antigenic sin, relates to the concept of mounting an antibody response to a new pathogen using memory cells recognizing past antigens over stimulating a de novo antibody response (189). Such responses have been shown for influenza and dengue virus and are associated with poor virus neutralization and can have profound consequences on vaccine efficacy (190–193). For SARS-CoV-2 infections, a number of studies have now reported back-boosting of non-neutralizing sCoV antibody production (116, 118, 167, 194, 195). These antibodies appear to be most prominently targeted against conserved epitopes in OC43 and HKU1, both betacoronaviruses (194, 195). Interestingly, none of these studies presented evidence that a sCoV antibody boost was associated with either protection against SARS-CoV-2 infection or COVID-19 severity. In fact, a negative correlation was observed in some studies, providing additional support to a disfavorable consequence of immunological imprinting (194, 195). Given that most of the leading SARS-CoV-2 vaccine candidates being currently developed use the full (S1-S2) spike protein as the primary viral antigen, special consideration needs to be given as to whether inclusion of the S2 domain will be a factor that that impedes vaccine efficacy.
Epidemiological Implications of Serology Testing for SARS-CoV-2
As the COVID-19 pandemic continues around the world, with second and third waves of infections already taking form, it is becoming increasingly important to monitor serological data at the population level. Effective and ethical response strategies to the COVID-19 pandemic can only be formulated once it is accurately determined if neutralizing antibodies are present, how effective those antibodies are at preventing disease and viral spread, and how long that immunity will last. Ongoing epidemiological considerations include the concepts of herd immunity, shield immunity, and immunity passports. However, these ideas remain largely based on the assumption that a humoral response implies lasting immunity, making their implementation for SARS-CoV-2 premature on an ethical basis. It must be reiterated that further functional serological studies must be performed to measure long-term effectiveness of humoral responses.
One of the most widely discussed epidemiological concepts surrounding COVID-19 is the possibility of achieving herd immunity. Herd immunity is a population-level phenomenon where the risk of infection for susceptible and disproportionately vulnerable individuals is mitigated by the presence and proximity of immune individuals. As more people develop immunity, the risk to susceptible population decreases, resulting in fewer opportunities for pathogen transmission (196). Herd immunity is particularly important for protecting those who cannot be effectively vaccinated, such as the very young and the immunocompromised (197). For COVID-19, where the majority of deaths and severe symptoms are observed in patients 60 and older (198), herd immunity will also play an important role in protecting the vulnerable elderly population.
In order to achieve protection, a minimum percentage of the population, known as the herd immunity threshold (HIT), must develop immunity. In its most basic form, the HIT is estimated with the formula (R0 – 1)/R0, where R0 (the basic reproduction number for an infectious disease) represents the number of secondary cases generated by each infected individual in a fully susceptible population (199). Early models investigating the localized outbreaks in China estimated R0 for COVID-19 to range from 1.4 to 6.49, with a mean value of 3.28 (threshold = 69.5%) (200). While estimates continue to vary, there is a general consensus that the average value of R0 for COVID-19 is approximately between 2 and 3, implying that a minimum of 50% to 67% of a population must achieve immune resistance before herd immunity can take effect (201).
Herd immunity can either be achieved through natural acquisition (i.e., natural herd immunity) or by controlled vaccination programs (202). Natural herd immunity assumes that convalescence imparts sterilizing immunity, and therefore widespread infection is necessary for widespread immunity. In the context of COVID-19, while natural herd immunity is theoretically possible, its pursuit is difficult to ethically justify given the high mortality and lasting morbidity caused by the virus, and it is also difficult to implement practically. Recent seroprevalence data shows that no country is even close to achieving herd immunity through natural acquisition (Table 3). In Sweden, where no official lockdown measures were enforced throughout the pandemic, as of May 2020, there was only a seroprevalence of 15% in Stockholm (compared to their predicted seroprevalence of 40%) (214). Furthermore, in several COVID-19 “hotspots” like Iran and New York City, where large numbers of cases were observed in short timespans, seroprevalence still never exceeded 25%. In the vast majority of other cities and countries, seroprevalence is usually much lower than 10% (Table 3). Most importantly, given the high risk of long-term morbidity due to tissue damage caused by COVID-19, naturally acquired herd immunity cannot be ethically pursued or encouraged (215–218). Therefore, the pursuit of natural herd immunity against SARS-CoV-2 is not justifiable in any form or manner and will be associated with very high immediate and long-term healthcare costs due to chronic disease.
A safer, more effective, and ethically sound alternative to acquiring natural herd immunity is to deploy controlled vaccination programs. Rigorously tested and formally approved vaccines offer a safe and effective method to quickly increase a population’s immunity to a harmful pathogen (219). Furthermore, vaccines are designed to elicit a neutralizing antibody response without the severe pathogenesis associated with the corresponding disease, in this case, COVID-19. Therefore, while it may take several more months for a safe and effective SARS-CoV-2 vaccine to be developed, tested and deployed, it is widely agreed upon that vaccine-acquired herd immunity is faster, safer, cheaper, and more effective than natural herd immunity. However, cautious optimism is warranted over vaccines. Based on knowledge acquired from SARS-CoV convalescent individuals, long-term protective immunity may last for only a few months (6, 9). With waning humoral immunity over time against a highly prevalent and infectious virus, maintaining herd immunity at the population level will almost certainly require booster shots and updated vaccines to maintain immunity against reinfections by SARS-CoV-2 and its inevitable genetic variants that are poised to emerge.
Conclusions
Significant progress has been made with respect to understanding the antibody-mediated immune response to SARS-CoV-2 infections. The applications and utility of serological assays are manifold, spanning from the development of screening modalities for epidemiological monitoring and drafting effective public health policy, to the creation of vaccines, and finally to the diagnosis of past infections.
However, the appropriate utilization of serological data requires an understanding of its limitations and ensuring these limitations are accounted for in the current and future pandemic response. For example, it remains unclear whether differences exist between the effectiveness and duration of immunity procured by a natural SARS-CoV-2 infection or vaccine-mediated immunity. Furthermore, existing serological testing approaches are widely varied in their sensitivity, specificity, and practicality, and require an adept understanding of the characteristics of each test in order to determine which should be suitably used in which context. Finally, studying the characteristics of the main SARS-CoV-2 antigens has revealed how some may be better suited for either vaccine development versus serological testing. However, cross-reactivity to sCoVs, the risk of ADE, and emergence of mutations will have profound implications on how these antigens should be employed in vaccination or screening technologies.
As the COVID-19 pandemic continues to impart substantial human suffering and economic losses throughout the world, various governments and stakeholders are experiencing increasing urgency and pressure to re-open commercial and social activities. Nevertheless, folding under this pressure has the risk of driving pre-emptive action based on inconclusive evidence, as large-scale policy mistakes such as encouraging natural herd immunity, the implementation of unstandardized serological assays, or the distribution of unproven immunity passports may reverse progress and incur unacceptable human and financial costs. Instead, this motivation to resolve the pandemic should prompt the thoughtful application of existing research, as well as support initiatives that seek to address the remaining evidence gaps within epidemiology and questions of long-term immunity to COVID-19. Such a grounded approach will be required if we are to create safe and effective solutions for the rapid diagnosis and prevention of COVID-19 and, ultimately, return to our daily activities without having to wear a mask.
Author Contributions
YG and MG equally carried out the literature review, prepared the figures and tables, and the drafting of the manuscript. GL and MD contributed to the drafting of the manuscript. M-AL contributed to the literature review and drafting of the manuscript. All authors contributed to the article and approved the submitted version.
Conflict of Interest
The authors declare that the research was conducted in the absence of any commercial or financial relationships that could be construed as a potential conflict of interest.
Acknowledgments
M-AL holds a Canada Research Chair in Molecular Virology and Intrinsic Immunity. This work was supported by a grant from the Canadian Institutes for Health Research (CIHR) to M-AL.
Supplementary Material
The Supplementary Material for this article can be found online at: https://www.frontiersin.org/articles/10.3389/fimmu.2020.610688/full#supplementary-material
References
1. Zhu N, Zhang D, Wang W, Li X, Yang B, Song J, et al. A Novel Coronavirus from Patients with Pneumonia in China, 2019. N Engl J Med (2020) 382(8):727–33. doi: 10.1056/NEJMoa2001017
2. Wu F, Zhao S, Yu B, Chen YM, Wang W, Song ZG, et al. A new coronavirus associated with human respiratory disease in China. Nature (2020) 579(7798):265–9. doi: 10.1038/s41586-020-2008-3
3. Sohrabi C, Alsafi Z, O’Neill N, Khan M, Kerwan A, Al-Jabir A, et al. World Health Organization declares global emergency: A review of the 2019 novel coronavirus (COVID-19). Int J Surg (2020) 76:71–6. doi: 10.1016/j.ijsu.2020.02.034
4. Hamid S, Mir MY, Rohela GK. Novel coronavirus disease (COVID-19): a pandemic (epidemiology, pathogenesis and potential therapeutics). New Microbes New Infect (2020) 35:100679. doi: 10.1016/j.nmni.2020.100679
5. Read JM, Bridgen JR, Cummings DA, Ho A, Jewell CP. Novel coronavirus 2019-nCoV: early estimation of epidemiological parameters and epidemic predictions. medRxiv (2020) 2020.01.23.20018549. doi: 10.1101/2020.01.23.20018549
6. Kissler SM, Tedijanto C, Goldstein E, Grad YH, Lipsitch M. Projecting the transmission dynamics of SARS-CoV-2 through the postpandemic period. Science (2020) 368(6493):860–8. doi: 10.1126/science.abb5793
7. Kucharski AJ, Russell TW, Diamond C, Liu Y, Edmunds J, Funk S, et al. Early dynamics of transmission and control of COVID-19: a mathematical modelling study. Lancet Infect Dis (2020) 20(5):553–8. doi: 10.1101/2020.01.31.20019901
8. Liu W, Fontanet A, Zhang PH, Zhan L, Xin ZT, Baril L, et al. Two-year prospective study of the humoral immune response of patients with severe acute respiratory syndrome. J Infect Dis (2006) 193(6):792–5. doi: 10.1086/500469
9. Wu LP, Wang NC, Chang YH, Tian XY, Na DY, Zhang LY, et al. Duration of antibody responses after severe acute respiratory syndrome. Emerg Infect Dis (2007) 13(10):1562–4. doi: 10.3201/eid1310.070576
10. Tang F, Quan Y, Xin ZT, Wrammert J, Ma MJ, Lv H, et al. Lack of peripheral memory B cell responses in recovered patients with severe acute respiratory syndrome: a six-year follow-up study. J Immunol (2011) 186(12):7264–8. doi: 10.4049/jimmunol.0903490
11. Payne DC, Iblan I, Rha B, Alqasrawi S, Haddadin A, Al Nsour M, et al. Persistence of Antibodies against Middle East Respiratory Syndrome Coronavirus. Emerg Infect Dis (2016) 22(10):1824–6. doi: 10.3201/eid2210.160706
12. Edridge AWD, Kaczorowska J, Hoste ACR, Bakker M, Klein M, Loens K, et al. Seasonal coronavirus protective immunity is short-lasting. Nat Med (2020) 26:1691–3. doi: 10.1101/2020.05.11.20086439
13. Shaman J, Galanti M. Will SARS-CoV-2 become endemic? Science (2020) 370(6516):527–9. doi: 10.1126/science.abe5960
14. Ohst C, Saschenbrecker S, Stiba K, Steinhagen K, Probst C, Radzimski C, et al. Reliable Serological Testing for the Diagnosis of Emerging Infectious Diseases. Adv Exp Med Biol (2018) 1062:19–43. doi: 10.1007/978-981-10-8727-1_3
15. Gaebler C, Nussenzweig MC. All eyes on a hurdle race for a SARS-CoV-2 vaccine. Nature (2020) 586(7830):501–2. doi: 10.1038/d41586-020-02926-w
16. Bryant JE, Azman AS, Ferrari MJ, Arnold BF, Boni MF, Boum Y, et al. Serology for SARS-CoV-2: Apprehensions, opportunities, and the path forward. Sci Immunol (2020) 5(47). doi: 10.1126/sciimmunol.abc6347
17. Grzelak L, Temmam S, Planchais C, Demeret C, Tondeur L, Huon C, et al. A comparison of four serological assays for detecting anti-SARS-CoV-2 antibodies in human serum samples from different populations. Sci Transl Med (2020) 12(559). doi: 10.1126/scitranslmed.abc3103
18. Amanat F, Stadlbauer D, Strohmeier S, Nguyen THO, Chromikova V, McMahon M, et al. A serological assay to detect SARS-CoV-2 seroconversion in humans. Nat Med (2020) 26:1033–6. doi: 10.1038/s41591-020-0913-5
19. Okba NMA, Muller MA, Li W, Wang C, GeurtsvanKessel CH, Corman VM, et al. Severe Acute Respiratory Syndrome Coronavirus 2-Specific Antibody Responses in Coronavirus Disease Patients. Emerg Infect Dis (2020) 26(7):1478–88. doi: 10.3201/eid2607.200841
20. Ng K, Faulkner N, Cornish G, Rosa A, Earl C, Wrobel A, et al. Pre-existing and de novo humoral immunity to SARS-CoV-2 in humans. bioRxiv (2020). 2020.05.14.095414. doi: 10.1101/2020.05.14.095414
21. Whitman JD, Hiatt J, Mowery CT, Shy BR, Yu R, Yamamoto TN, et al. Test performance evaluation of SARS-CoV-2 serological assays. medRxiv (2020). doi: 10.1101/2020.04.25.20074856
22. Koczula KM, Gallotta A. Lateral flow assays. Essays Biochem (2016) 60(1):111–20. doi: 10.1042/EBC20150012
23. Image created with BioRender (2020). Available at: https://biorender.com/.
24. Janeway CA Jr, Travers P, Walport M, Shlomchik MJ. Immunobiology: The Immune system in health and Disease. 5th ed. New York: Garland Science (2001).
25. Schroeder HW Jr., Cavacini L. Structure and function of immunoglobulins. J Allergy Clin Immunol (2010) 125(2 Suppl 2):S41–52. doi: 10.1016/j.jaci.2009.09.046
26. Boes M. Role of natural and immune IgM antibodies in immune responses. Mol Immunol (2000) 37(18):1141–9. doi: 10.1016/S0161-5890(01)00025-6
27. Planchais C, Mouquet H. Easy pan-detection of human IgA immunoglobulins. J Immunol Methods (2020) 484–5:112833. doi: 10.1016/j.jim.2020.112833
28. Breedveld A, van Egmond M. IgA and FcαRI: Pathological Roles and Therapeutic Opportunities. Front Immunol (2019) 10:553. doi: 10.3389/fimmu.2019.00553
29. Woof JM, Mestecky J. Mucosal immunoglobulins. Immunol Rev (2005) 206:64–82. doi: 10.1111/j.0105-2896.2005.00290.x
30. Freihorst J, Ogra PL. Mucosal immunity and viral infections. Ann Med (2001) 33(3):172–7. doi: 10.3109/07853890109002074
31. Zan H, Casali P. Immunoglobulin Somatic Hypermutation and Class-Switch DNA Recombination. In: Mackay IR, Rose NR, Diamond B, Davidson A, editors. Encyclopedia of Medical Immunology: Autoimmune Diseases. New York, NY: Springer New York (2014). doi: 10.1007/978-0-387-84828-0_556
32. Vidarsson G, Dekkers G, Rispens T. IgG subclasses and allotypes: from structure to effector functions. Front Immunol (2014) 5:520. doi: 10.3389/fimmu.2014.00520
33. Lu LL, Suscovich TJ, Fortune SM, Alter G. Beyond binding: antibody effector functions in infectious diseases. Nat Rev Immunol (2018) 18(1):46–61. doi: 10.1038/nri.2017.106
34. van Erp EA, Luytjes W, Ferwerda G, van Kasteren PB. Fc-Mediated Antibody Effector Functions During Respiratory Syncytial Virus Infection and Disease. Front Immunol (2019) 10(548). doi: 10.3389/fimmu.2019.00548
35. Holers VM. Complement and its receptors: new insights into human disease. Annu Rev Immunol (2014) 32:433–59. doi: 10.1146/annurev-immunol-032713-120154
36. Soininen A, Seppälä I, Nieminen T, Eskola J, Käyhty H. IgG subclass distribution of antibodies after vaccination of adults with pneumococcal conjugate vaccines. Vaccine (1999) 17(15-16):1889–97. doi: 10.1016/S0264-410X(98)00475-7
37. Isa MB, Martínez L, Giordano M, Passeggi C, de Wolff MC, Nates S. Comparison of immunoglobulin G subclass profiles induced by measles virus in vaccinated and naturally infected individuals. Clin Diagn Lab Immunol (2002) 9(3):693–7. doi: 10.1128/CDLI.9.3.693-697.2002
38. Amarasekera M. Immunoglobulin E in health and disease. Asia Pac Allergy (2011) 1(1):12–5. doi: 10.5415/apallergy.2011.1.1.12
39. Guo L, Ren L, Yang S, Xiao M, Chang D, Yang F, et al. Profiling Early Humoral Response to Diagnose Novel Coronavirus Disease (COVID-19). Clin Infect Dis (2020) 71(15):778–85. doi: 10.1093/cid/ciaa310
40. Padoan A, Sciacovelli L, Basso D, Negrini D, Zuin S, Cosma C, et al. IgA-Ab response to spike glycoprotein of SARS-CoV-2 in patients with COVID-19: A longitudinal study. Clin Chim Acta (2020) 507:164–6. doi: 10.1016/j.cca.2020.04.026
41. Jaaskelainen AJ, Kekalainen E, Kallio-Kokko H, Mannonen L, Kortela E, Vapalahti O, et al. Evaluation of commercial and automated SARS-CoV-2 IgG and IgA ELISAs using coronavirus disease (COVID-19) patient samples. Euro Surveill (2020) 25(18). doi: 10.2807/1560-7917.ES.2020.25.18.2000603
42. Long QX, Tang XJ, Shi QL, Li Q, Deng HJ, Yuan J, et al. Clinical and immunological assessment of asymptomatic SARS-CoV-2 infections. Nat Med (2020) 26:1200–4. doi: 10.1038/s41591-020-0965-6
43. Prevost J, Gasser R, Beaudoin-Bussieres G, Richard J, Duerr R, Laumaea A, et al. Cross-Sectional Evaluation of Humoral Responses against SARS-CoV-2 Spike. Cell Rep Med (2020) 1(7):100126. doi: 10.1016/j.xcrm.2020.100126
44. Seow J, Graham C, Merrick B, Acors S, Pickering S, Steel KJA, et al. Longitudinal observation and decline of neutralizing antibody responses in the three months following SARS-CoV-2 infection in humans. Nat Microbiol (2020) 5:1598–607. doi: 10.1038/s41564-020-00813-8
45. Beaudoin-Bussieres G, Laumaea A, Anand SP, Prevost J, Gasser R, Goyette G, et al. Decline of Humoral Responses against SARS-CoV-2 Spike in Convalescent Individuals. mBio (2020) 11(5). doi: 10.1128/mBio.02590-20
46. Galanti M, Shaman J. Direct Observation of Repeated Infections With Endemic Coronaviruses. J Infect Dis (2020). doi: 10.1101/2020.04.27.20082032
47. Palm A-KE, Henry C. Remembrance of Things Past: Long-Term B Cell Memory After Infection and Vaccination. Front Immunol (2019) 10(1787). doi: 10.3389/fimmu.2019.01787
48. Huang AT, Garcia-Carreras B, Hitchings MDT, Yang B, Katzelnick LC, Rattigan SM, et al. A systematic review of antibody mediated immunity to coronaviruses: kinetics, correlates of protection, and association with severity. Nat Commun (2020) 11(1):4704. doi: 10.1038/s41467-020-18450-4
49. Weissleder R, Lee H, Ko J, Pittet MJ. COVID-19 diagnostics in context. Sci Transl Med (2020) 12(546):eabc1931. doi: 10.1126/scitranslmed.abc1931
50. Tortorici MA, Veesler D. Structural insights into coronavirus entry. Adv Virus Res (2019) 105:93–116. doi: 10.1016/bs.aivir.2019.08.002
51. Krammer F. SARS-CoV-2 vaccines in development. Nature (2020) 586(7830):516–27. doi: 10.1038/s41586-020-2798-3
52. Walls AC, Park YJ, Tortorici MA, Wall A, McGuire AT, Veesler D. Structure, Function, and Antigenicity of the SARS-CoV-2 Spike Glycoprotein. Cell (2020) 181(2):281–92.e6. doi: 10.1016/j.cell.2020.02.058
53. Ju B, Zhang Q, Ge X, Wang R, Yu J, Shan S, et al. Potent human neutralizing antibodies elicited by SARS-CoV-2 infection. bioRxiv (2020) 2020.03.21.990770. doi: 10.1101/2020.03.21.990770
54. Yi C, Sun X, Ye J, Ding L, Liu M, Yang Z, et al. Key residues of the receptor binding motif in the spike protein of SARS-CoV-2 that interact with ACE2 and neutralizing antibodies. Cell Mol Immunol (2020) 17(6):621–30. doi: 10.1038/s41423-020-0458-z
55. Yuan M, Wu NC, Zhu X, Lee CD, So RTY, Lv H, et al. A highly conserved cryptic epitope in the receptor binding domains of SARS-CoV-2 and SARS-CoV. Science (2020) 368(6491):630–3. doi: 10.1126/science.abb7269
56. Lan J, Ge J, Yu J, Shan S, Zhou H, Fan S, et al. Structure of the SARS-CoV-2 spike receptor-binding domain bound to the ACE2 receptor. Nature (2020) 581(7807):215–20. doi: 10.1038/s41586-020-2180-5
57. Liu L, Wang P, Nair MS, Yu J, Rapp M, Wang Q, et al. Potent neutralizing antibodies against multiple epitopes on SARS-CoV-2 spike. Nature (2020) 584(7821):450–6. doi: 10.1038/s41586-020-2571-7
58. Kang S, Yang M, Hong Z, Zhang L, Huang Z, Chen X, et al. Crystal structure of SARS-CoV-2 nucleocapsid protein RNA binding domain reveals potential unique drug targeting sites. Acta Pharm Sin B (2020) 10(7):1228–38. doi: 10.1101/2020.03.06.977876
59. Ahmed SF, Quadeer AA, McKay MR. Preliminary Identification of Potential Vaccine Targets for the COVID-19 Coronavirus (SARS-CoV-2) Based on SARS-CoV Immunological Studies. Viruses (2020) 12(3). doi: 10.3390/v12030254
60. Liu SJ, Leng CH, Lien SP, Chi HY, Huang CY, Lin CL, et al. Immunological characterizations of the nucleocapsid protein based SARS vaccine candidates. Vaccine (2006) 24(16):3100–8. doi: 10.1016/j.vaccine.2006.01.058
61. Piccoli L, Park YJ, Tortorici MA, Czudnochowski N, Walls AC, Beltramello M, et al. Mapping Neutralizing and Immunodominant Sites on the SARS-CoV-2 Spike Receptor-Binding Domain by Structure-Guided High-Resolution Serology. Cell (2020) 183(4):1024–42.e21. doi: 10.1016/j.cell.2020.09.037
62. Shrock E, Fujimura E, Kula T, Timms RT, Lee IH, Leng Y, et al. Viral epitope profiling of COVID-19 patients reveals cross-reactivity and correlates of severity. Science (2020) 370(6520). doi: 10.1126/science.abd4250
63. Wajnberg A, Amanat F, Firpo A, Altman D, Bailey M, Mansour M, et al. SARS-CoV-2 infection induces robust, neutralizing antibody responses that are stable for at least three months. medRxiv (2020) 2020.07.14.20151126. doi: 10.1101/2020.07.14.20151126
64. McAndrews KM, Dowlatshahi DP, Dai J, Becker LM, Hensel J, Snowden LM, et al. Heterogeneous antibodies against SARS-CoV-2 spike receptor binding domain and nucleocapsid with implications for COVID-19 immunity. JCI Insight (2020) 5(18). doi: 10.1172/jci.insight.142386
65. Deming D, Sheahan T, Heise M, Yount B, Davis N, Sims A, et al. Vaccine efficacy in senescent mice challenged with recombinant SARS-CoV bearing epidemic and zoonotic spike variants. PloS Med (2006) 3(12):e525. doi: 10.1371/journal.pmed.0030525
66. Wolfel R, Corman VM, Guggemos W, Seilmaier M, Zange S, Muller MA, et al. Virological assessment of hospitalized patients with COVID-2019. Nature (2020) 581(7809):465–9. doi: 10.1038/s41586-020-2196-x
67. Wang Y, Zhang L, Sang L, Ye F, Ruan S, Zhong B, et al. Kinetics of viral load and antibody response in relation to COVID-19 severity. J Clin Invest (2020) 130(10):5235–44. doi: 10.1172/JCI138759
68. Borremans B, Gamble A, Prager KC, Helman SK, McClain AM, Cox C, et al. Quantifying antibody kinetics and RNA detection during early-phase SARS-CoV-2 infection by time since symptom onset. Elife (2020) 9. doi: 10.7554/eLife.60122
69. Biosciences I. Guide to Lateral Flow Immunoassays. (2020). Available at: https://fnkprddata.blob.core.windows.net/domestic/download/pdf/IBS_A_guide_to_lateral_flow_immunoassays.pdf.
70. Bissonnette L, Bergeron MG. Diagnosing infections–current and anticipated technologies for point-of-care diagnostics and home-based testing. Clin Microbiol Infect (2010) 16(8):1044–53. doi: 10.1111/j.1469-0691.2010.03282.x
71. Lisboa Bastos M, Tavaziva G, Abidi SK, Campbell JR, Haraoui L-P, Johnston JC, et al. Diagnostic accuracy of serological tests for covid-19: systematic review and meta-analysis. BMJ (2020) 370:m2516. doi: 10.1136/bmj.m2516
72. Pallett SJC, Rayment M, Patel A, Fitzgerald-Smith SAM, Denny SJ, Charani E, et al. Point-of-care serological assays for delayed SARS-CoV-2 case identification among health-care workers in the UK: a prospective multicentre cohort study. Lancet Respir Med (2020) 8(9):885–94. doi: 10.1016/S2213-2600(20)30315-5
73. Pickering S, Betancor G, Galao RP, Merrick B, Signell AW, Wilson HD, et al. Comparative assessment of multiple COVID-19 serological technologies supports continued evaluation of point-of-care lateral flow assays in hospital and community healthcare settings. PloS Pathog (2020) 16(9):e1008817. doi: 10.1101/2020.06.02.20120345
74. Patel EU, Bloch EM, Clarke W, Hsieh YH, Boon D, Eby Y, et al. Comparative performance of five commercially available serologic assays to detect antibodies to SARS-CoV-2 and identify individuals with high neutralizing titers. medRxiv (2020). doi: 10.1101/2020.08.31.20184788
75. Conklin SE, Martin K, Manabe YC, Schmidt HA, Keruly M, Klock E, et al. Evaluation of Serological SARS-CoV-2 Lateral Flow Assays for Rapid Point of Care Testing. medRxiv (2020) 2020.07.31.20166041. doi: 10.1101/2020.07.31.20166041
76. National SARS-CoV-2 Serology Assay Evaluation Group. Performance characteristics of five immunoassays for SARS-CoV-2: a head-to-head benchmark comparison. Lancet Infect Dis (2020) 20(12):1390–400. doi: 10.1016/S1473-3099(20)30634-4
77. Morrison A, Li Y, Loshak H. Serological Test for COVID-19. Ottawa: CADTH horizon Scan (2020). may 2020. Contract No.: 188.
78. McDade TW, McNally E, D’Aquila RT, Mustanski B, Miller A, Vaught L, et al. Enzyme immunoassay for SARS-CoV-2 antibodies in dried blood spot samples: A minimally-invasive approach to facilitate community- and population-based screening. medRxiv (2020) 2020.04.28.20081844. doi: 10.1101/2020.04.28.20081844
79. Center for Disease Control. Interim Laboratory Biosafety Guidelines for Handling and Processing Specimens Associated with Coronavirus Disease 2019 (COVID-19). CDC. (2020). Available at: https://www.cdc.gov/coronavirus/2019-ncov/lab/lab-biosafety-guidelines.html.
80. Nie J, Li Q, Wu J, Zhao C, Hao H, Liu H, et al. Establishment and validation of a pseudovirus neutralization assay for SARS-CoV-2. Emerg Microbes Infect (2020) 9(1):680–6. doi: 10.1080/22221751.2020.1743767
81. Schmidt F, Weisblum Y, Muecksch F, Hoffmann H-H, Michailidis E, Lorenzi JCC, et al. Measuring SARS-CoV-2 neutralizing antibody activity using pseudotyped and chimeric viruses. J Exp Med (2020) 217(11). doi: 10.1084/jem.20201181
82. GenScript. GenScript cPass™ SARS-CoV-2 Neutralization Antibody Detection Kit (2020). Available at: https://www.genscript.com/cpass-sars-cov-2-neutralization-antibody-detection-Kit.html.
83. Abe KT, Li Z, Samson R, Samavarchi-Tehrani P, Valcourt EJ, Wood H, et al. A simple protein-based surrogate neutralization assay for SARS-CoV-2. JCI Insight (2020) 5(19). doi: 10.1172/jci.insight.142362
84. Tan CW, Chia WN, Qin X, Liu P, Chen MI, Tiu C, et al. A SARS-CoV-2 surrogate virus neutralization test based on antibody-mediated blockage of ACE2-spike protein-protein interaction. Nat Biotechnol (2020) 38:1073–8. doi: 10.21203/rs.3.rs-24574/v1
85. Wang Q, Zhang Y, Wu L, Niu S, Song C, Zhang Z, et al. Structural and Functional Basis of SARS-CoV-2 Entry by Using Human ACE2. Cell (2020) 181(4):894–904.e9. doi: 10.1016/j.cell.2020.03.045
86. Seydoux E, Homad LJ, MacCamy AJ, Parks KR, Hurlburt NK, Jennewein MF, et al. Analysis of a SARS-CoV-2 infected individual reveals development of potent neutralizing antibodies to distinct epitopes with limited somatic mutation. Immunity (2020) 53(1):98–105.e5. doi: 10.1016/j.immuni.2020.06.001
87. Suthar MS, Zimmerman M, Kauffman R, Mantus G, Linderman S, Vanderheiden A, et al. Rapid generation of neutralizing antibody responses in COVID-19 patients. medRxiv (2020) 2020.05.03.20084442. doi: 10.1101/2020.05.03.20084442
88. Premkumar L, Segovia-Chumbez B, Jadi R, Martinez DR, Raut R, Markmann A, et al. The receptor binding domain of the viral spike protein is an immunodominant and highly specific target of antibodies in SARS-CoV-2 patients. Sci Immunol (2020) 5(48). doi: 10.1126/sciimmunol.abc8413
89. Shen C, Wang Z, Zhao F, Yang Y, Li J, Yuan J, et al. Treatment of 5 Critically Ill Patients With COVID-19 With Convalescent Plasma. Jama (2020) 323(16):1582–9. doi: 10.1001/jama.2020.4783
90. Robbiani DF, Gaebler C, Muecksch F, Lorenzi JCC, Wang Z, Cho A, et al. Convergent antibody responses to SARS-CoV-2 in convalescent individuals. Nature (2020) 584(7821):437–42. doi: 10.1038/s41586-020-2456-9
91. Brouwer PJM, Caniels TG, van der Straten K, Snitselaar JL, Aldon Y, Bangaru S, et al. Potent neutralizing antibodies from COVID-19 patients define multiple targets of vulnerability. Science (2020) 369(6504):643–50. doi: 10.1101/2020.05.12.088716
92. Chi X, Yan R, Zhang J, Zhang G, Zhang Y, Hao M, et al. A neutralizing human antibody binds to the N-terminal domain of the Spike protein of SARS-CoV-2. Science (2020) 369(6504):650–5. doi: 10.1126/science.abc6952
93. Schäfer A, Muecksch F, Lorenzi JCC, Leist SR, Cipolla M, Bournazos S, et al. Antibody potency, effector function and combinations in protection from SARS-CoV-2 infection in vivo. bioRxiv (2020) 2020.09.15.298067. doi: 10.1101/2020.09.15.298067
94. Burbelo PD, Riedo FX, Morishima C, Rawlings S, Smith D, Das S, et al. Detection of Nucleocapsid Antibody to SARS-CoV-2 is More Sensitive than Antibody to Spike Protein in COVID-19 Patients. medRxiv (2020) 2020.04.20.20071423. doi: 10.1101/2020.04.20.20071423
95. Trevethan R. Sensitivity, Specificity, and Predictive Values: Foundations, Pliabilities, and Pitfalls in Research and Practice. Front Public Health (2017) 5(307). doi: 10.3389/fpubh.2017.00307
96. FDA. EUA Authorized Serology Test Performance. FDA (2020). Available at: https://www.fda.gov/medical-devices/emergency-situations-medical-devices/eua-authorized-serology-test-performance.
97. Serodiagnostics for Severe Acute Respiratory Syndrome–Related Coronavirus-2: A Narrative Review. Ann Internal Med (2020) 173(6):450–60. doi: 10.7326/M20-2854
98. Zou KH, O’Malley AJ, Mauri L. Receiver-operating characteristic analysis for evaluating diagnostic tests and predictive models. Circulation (2007) 115(5):654–7. doi: 10.1161/CIRCULATIONAHA.105.594929
99. GISAID. Genomic epidemiology of novel coronavirus - Global subsampling. (GISAID (2020)). Available at: https://nextstrain.org/ncov/global?animate=2019-12-09,2020-04-10,1,1,15000&f_country=USA.
100. Haselmann V, Kittel M, Gerhards C, Thiaucourt M, Eichner R, Costina V, et al. Comparison of test performance of commercial anti-SARS-CoV-2 immunoassays in serum and plasma samples. Clin Chim Acta (2020) 510:73–8. doi: 10.1016/j.cca.2020.07.007
101. Traugott M, Aberle SW, Aberle JH, Griebler H, Karolyi M, Pawelka E, et al. Performance of Severe Acute Respiratory Syndrome Coronavirus 2 Antibody Assays in Different Stages of Infection: Comparison of Commercial Enzyme-Linked Immunosorbent Assays and Rapid Tests. J Infect Dis (2020) 222(3):362–6. doi: 10.1093/infdis/jiaa305
102. Jaaskelainen AJ, Kuivanen S, Kekalainen E, Ahava MJ, Loginov R, Kallio-Kokko H, et al. Performance of six SARS-CoV-2 immunoassays in comparison with microneutralisation. J Clin Virol (2020) 129:104512. doi: 10.1016/j.jcv.2020.104512
103. Adams E, Ainsworth M, Anand R, Andersson M, Auckland K, Baillie J, et al. Antibody testing for COVID-19: A report from the†National COVID Scientific Advisory Panel [version 1; peer review: 1 approved]. Wellcome Open Res (2020) 5(139). doi: 10.12688/wellcomeopenres.15927.1
104. Muecksch F, Wise H, Batchelor B, Squires M, Semple E, Richardson C, et al. Longitudinal analysis of clinical serology assay performance and neutralising antibody levels in COVID19 convalescents. medRxiv (2020) 2020.08.05.20169128. doi: 10.1101/2020.08.05.20169128
105. Hu X, An T, Situ B, Hu Y, Ou Z, Li Q, et al. Heat inactivation of serum interferes with the immunoanalysis of antibodies to SARS-CoV-2. J Clin Lab Anal (2020) 34(9):e23411. doi: 10.1002/jcla.23411
106. Xu Z, Zan H, Pone EJ, Mai T, Casali P. Immunoglobulin class-switch DNA recombination: induction, targeting and beyond. Nat Rev Immunol (2012) 12(7):517–31. doi: 10.1038/nri3216
107. Ye Q, Wang B, Mao J. The pathogenesis and treatment of the `Cytokine Storm’ in COVID-19. J Infect (2020) 80(6):607–13. doi: 10.1016/j.jinf.2020.03.037
108. Coperchini F, Chiovato L, Croce L, Magri F, Rotondi M. The cytokine storm in COVID-19: An overview of the involvement of the chemokine/chemokine-receptor system. Cytokine Growth Factor Rev (2020) 53:25–32. doi: 10.1016/j.cytogfr.2020.05.003
109. Pedersen SF, Ho YC. SARS-CoV-2: a storm is raging. J Clin Invest (2020) 130(5):2202–5. doi: 10.1172/JCI137647
110. Woodruff MC, Ramonell RP, Nguyen DC, Cashman KS, Saini AS, Haddad NS, et al. Extrafollicular B cell responses correlate with neutralizing antibodies and morbidity in COVID-19. Nat Immunol (2020) 21:1506–1516. doi: 10.1038/s41590-020-00814-z
111. Azkur AK, Akdis M, Azkur D, Sokolowska M, van de Veen W, Bruggen MC, et al. Immune response to SARS-CoV-2 and mechanisms of immunopathological changes in COVID-19. Allergy (2020) 75(7):1564–81. doi: 10.1111/all.14364
112. Long Q-x, Deng H-j, Chen J, Hu J, Liu B-z, Liao P, et al. Antibody responses to SARS-CoV-2 in COVID-19 patients: the perspective application of serological tests in clinical practice. medRxiv (2020). doi: 10.1101/2020.03.18.20038018.
113. Lee YL, Liao CH, Liu PY, Cheng CY, Chung MY, Liu CE, et al. Dynamics of anti-SARS-Cov-2 IgM and IgG antibodies among COVID-19 patients. J Infect (2020) 81(2):e55–e8. doi: 10.1016/j.jinf.2020.04.019
114. To KK, Tsang OT, Leung WS, Tam AR, Wu TC, Lung DC, et al. Temporal profiles of viral load in posterior oropharyngeal saliva samples and serum antibody responses during infection by SARS-CoV-2: an observational cohort study. Lancet Infect Dis (2020) 20(5):565–74. doi: 10.1016/S1473-3099(20)30196-1
115. Zhang W, Du RH, Li B, Zheng XS, Yang XL, Hu B, et al. Molecular and serological investigation of 2019-nCoV infected patients: implication of multiple shedding routes. Emerg Microbes Infect (2020) 9(1):386–9. doi: 10.1080/22221751.2020.1729071
116. Anderson EM, Goodwin EC, Verma A, Arevalo CP, Bolton MJ, Weirick ME, et al. Seasonal human coronavirus antibodies are boosted upon SARS-CoV-2 infection but not associated with protection. medRxiv (2020). doi: 10.1101/2020.11.06.20227215.
117. Poston D, Weisblum Y, Wise H, Templeton K, Jenks S, Hatziioannou T, et al. Absence of SARS-CoV-2 neutralizing activity in pre-pandemic sera from individuals with recent seasonal coronavirus infection. medRxiv (2020) 2020.10.08.20209650. doi: 10.1101/2020.10.08.20209650
118. Ng KW, Faulkner N, Cornish GH, Rosa A, Harvey R, Hussain S, et al. Preexisting and de novo humoral immunity to SARS-CoV-2 in humans. Science (2020) eabe1107. doi: 10.1126/science.abe1107
119. Jin Y, Wang M, Zuo Z, Fan C, Ye F, Cai Z, et al. Diagnostic value and dynamic variance of serum antibody in coronavirus disease 2019. Int J Infect Dis (2020) 94:49–52. doi: 10.1016/j.ijid.2020.03.065
120. Sun B, Feng Y, Mo X, Zheng P, Wang Q, Li P, et al. Kinetics of SARS-CoV-2 specific IgM and IgG responses in COVID-19 patients. Emerg Microbes Infect (2020) 9(1):940–8. doi: 10.1080/22221751.2020.1762515
121. Long QX, Liu BZ, Deng HJ, Wu GC, Deng K, Chen YK, et al. Antibody responses to SARS-CoV-2 in patients with COVID-19. Nat Med (2020) 26(6):845–8. doi: 10.1038/s41591-020-0897-1
122. Robbiani DF, Gaebler C, Muecksch F, Lorenzi JCC, Wang Z, Cho A, et al. Convergent Antibody Responses to SARS-CoV-2 Infection in Convalescent Individuals. bioRxiv (2020). doi: 10.1101/2020.05.13.092619
123. Seow J, Graham C, Merrick B, Acors S, Steel KJA, Hemmings O, et al. Longitudinal evaluation and decline of antibody responses in SARS-CoV-2 infection. medRxiv (2020) 2020.07.09.20148429. doi: 10.1101/2020.07.09.20148429
124. Isho B, Abe KT, Zuo M, Jamal AJ, Rathod B, Wang JH, et al. Persistence of serum and saliva antibody responses to SARS-CoV-2 spike antigens in COVID-19 patients. Sci Immunol (2020) 5(52). doi: 10.1101/2020.08.01.20166553
125. Zhao J, Yuan Q, Wang H, Liu W, Liao X, Su Y, et al. Antibody responses to SARS-CoV-2 in patients of novel coronavirus disease 2019. Clin Infect Dis (2020) 71(16):2027–34. doi: 10.1101/2020.03.02.20030189
126. Stavnezer J, Guikema JE, Schrader CE. Mechanism and regulation of class switch recombination. Annu Rev Immunol (2008) 26:261–92. doi: 10.1146/annurev.immunol.26.021607.090248
127. Meyer B, Drosten C, Müller MA. Serological assays for emerging coronaviruses: challenges and pitfalls. Virus Res (2014) 194:175–83. doi: 10.1016/j.virusres.2014.03.018
128. Hsueh PR, Huang LM, Chen PJ, Kao CL, Yang PC. Chronological evolution of IgM, IgA, IgG and neutralisation antibodies after infection with SARS-associated coronavirus. Clin Microbiol Infect (2004) 10(12):1062–6. doi: 10.1111/j.1469-0691.2004.01009.x
129. Xiang F, Wang X, He X, Peng Z, Yang B, Zhang J, et al. Antibody Detection and Dynamic Characteristics in Patients with COVID-19. Clin Infect Dis (2020) 71(8):1930–4. doi: 10.1093/cid/ciaa461
130. Wajnberg A, Amanat F, Firpo A, Altman DR, Bailey MJ, Mansour M, et al. Robust neutralizing antibodies to SARS-CoV-2 infection persist for months. Science (2020). doi: 10.1126/science.abd7728
131. Chen Y, Zuiani A, Fischinger S, Mullur J, Atyeo C, Travers M, et al. Quick COVID-19 Healers Sustain Anti-SARS-CoV-2 Antibody Production. Cell (2020) 183:1–12. doi: 10.1016/j.cell.2020.10.051
132. Ripperger TJ, Uhrlaub JL, Watanabe M, Wong R, Castaneda Y, Pizzato HA, et al. Detection, prevalence, and duration of humoral responses to SARS-CoV-2 under conditions of limited population exposure. medRxiv (2020) 2020.08.14.20174490. doi: 10.1101/2020.08.14.20174490
133. Isho B, Abe KT, Zuo M, Jamal AJ, Rathod B, Wang JH, et al. Mucosal versus systemic antibody responses to SARS-CoV-2 antigens in COVID-19 patients. medRxiv (2020) 2020.08.01.20166553. doi: 10.1101/2020.08.01.20166553
134. Iyer AS, Jones FK, Nodoushani A, Kelly M, Becker M, Slater D, et al. Persistence and decay of human antibody responses to the receptor binding domain of SARS-CoV-2 spike protein in COVID-19 patients. Sci Immunol (2020) 5(52). doi: 10.1126/sciimmunol.abe0367
135. Gudbjartsson DF, Norddahl GL, Melsted P, Gunnarsdottir K, Holm H, Eythorsson E, et al. Humoral Immune Response to SARS-CoV-2 in Iceland. N Engl J Med (2020) 383(18):1724–34. doi: 10.1056/NEJMoa2026116
136. Suthar MS, Zimmerman MG, Kauffman RC, Mantus G, Linderman SL, Hudson WH, et al. Rapid Generation of Neutralizing Antibody Responses in COVID-19 Patients. Cell Rep Med (2020) 1(3):100040. doi: 10.1101/2020.05.03.20084442
137. Kreer C, Zehner M, Weber T, Ercanoglu MS, Gieselmann L, Rohde C, et al. Longitudinal Isolation of Potent Near-Germline SARS-CoV-2-Neutralizing Antibodies from COVID-19 Patients. Cell (2020) 182(4):843–54.e12. doi: 10.1016/j.cell.2020.06.044
138. Deng W, Bao L, Liu J, Xiao C, Liu J, Xue J, et al. Primary exposure to SARS-CoV-2 protects against reinfection in rhesus macaques. Science (2020) 369(6505):818–23. doi: 10.1126/science.abc5343
139. Chandrashekar A, Liu J, Martinot AJ, McMahan K, Mercado NB, Peter L, et al. SARS-CoV-2 infection protects against rechallenge in rhesus macaques. Science (2020) 369(6505):812–7. doi: 10.1126/science.abc4776
140. Chen Y, Tong X, Li Y, Gu B, Yan J, Liu Y, et al. A comprehensive, longitudinal analysis of humoral responses specific to four recombinant antigens of SARS-CoV-2 in severe and non-severe COVID-19 patients. PloS Pathog (2020) 16(9):e1008796. doi: 10.1371/journal.ppat.1008796
141. Wu J, Liang B, Chen C, Wang H, Fang Y, Shen S, et al. SARS-CoV-2 infection induces sustained humoral immune responses in convalescent patients following symptomatic COVID-19. medRxiv (2020) 2020.07.21.20159178. doi: 10.1101/2020.07.21.20159178
142. Ripperger TJ, Uhrlaub JL, Watanabe M, Wong R, Castaneda Y, Pizzato HA, et al. Orthogonal SARS-CoV-2 Serological Assays Enable Surveillance of Low-Prevalence Communities and Reveal Durable Humoral Immunity. Immunity (2020) 53(5):925–33. doi: 10.1016/j.immuni.2020.10.004
143. Gaebler C, Wang Z, Lorenzi JCC, Muecksch F, Finkin S, Tokuyama M, et al. Evolution of Antibody Immunity to SARS-CoV-2. bioRxiv (2020). doi: 10.1101/2020.11.03.367391
144. Slifka MK, Ahmed R. Long-lived plasma cells: a mechanism for maintaining persistent antibody production. Curr Opin Immunol (1998) 10(3):252–8. doi: 10.1016/S0952-7915(98)80162-3
145. Nutt SL, Hodgkin PD, Tarlinton DM, Corcoran LM. The generation of antibody-secreting plasma cells. Nat Rev Immunol (2015) 15(3):160–71. doi: 10.1038/nri3795
146. Mankarious S, Lee M, Fischer S, Pyun KH, Ochs HD, Oxelius VA, et al. The half-lives of IgG subclasses and specific antibodies in patients with primary immunodeficiency who are receiving intravenously administered immunoglobulin. J Lab Clin Med (1988) 112(5):634–40.
147. Zhang B, Zhou X, Zhu C, Song Y, Feng F, Qiu Y, et al. Immune Phenotyping Based on the Neutrophil-to-Lymphocyte Ratio and IgG Level Predicts Disease Severity and Outcome for Patients With COVID-19. Front Mol Biosci (2020) 7:157. doi: 10.3389/fmolb.2020.00157
148. Lee WS, Wheatley AK, Kent SJ, DeKosky BJ. Antibody-dependent enhancement and SARS-CoV-2 vaccines and therapies. Nat Microbiol (2020) 5(10):1185–91. doi: 10.1038/s41564-020-00789-5
149. Arvin AM, Fink K, Schmid MA, Cathcart A, Spreafico R, Havenar-Daughton C, et al. A perspective on potential antibody-dependent enhancement of SARS-CoV-2. Nature (2020) 584:353–63. doi: 10.1038/s41586-020-2538-8
150. Negro F. Is antibody-dependent enhancement playing a role in COVID-19 pathogenesis? Swiss Med Wkly (2020) 150:w20249. doi: 10.4414/smw.2020.20249
151. Bardina SV, Bunduc P, Tripathi S, Duehr J, Frere JJ, Brown JA, et al. Enhancement of Zika virus pathogenesis by preexisting antiflavivirus immunity. Science (2017) 356(6334):175–80. doi: 10.1126/science.aal4365
152. Kuzmina NA, Younan P, Gilchuk P, Santos RI, Flyak AI, Ilinykh PA, et al. Antibody-Dependent Enhancement of Ebola Virus Infection by Human Antibodies Isolated from Survivors. Cell Rep (2018) 24(7):1802–15.e5. doi: 10.1016/j.celrep.2018.07.035
153. Wang SF, Tseng SP, Yen CH, Yang JY, Tsao CH, Shen CW, et al. Antibody-dependent SARS coronavirus infection is mediated by antibodies against spike proteins. Biochem Biophys Res Commun (2014) 451(2):208–14. doi: 10.1016/j.bbrc.2014.07.090
154. Wan Y, Shang J, Sun S, Tai W, Chen J, Geng Q, et al. Molecular Mechanism for Antibody-Dependent Enhancement of Coronavirus Entry. J Virol (2020) 94(5). doi: 10.1128/JVI.02015-19
155. Jaume M, Yip MS, Cheung CY, Leung HL, Li PH, Kien F, et al. Anti-severe acute respiratory syndrome coronavirus spike antibodies trigger infection of human immune cells via a pH- and cysteine protease-independent FcγR pathway. J Virol (2011) 85(20):10582–97. doi: 10.1128/JVI.00671-11
156. Zohar T, Alter G. Dissecting antibody-mediated protection against SARS-CoV-2. Nat Rev Immunol (2020) 1–3. doi: 10.1038/s41577-020-0359-5
157. Iwasaki A, Yang Y. The potential danger of suboptimal antibody responses in COVID-19. Nat Rev Immunol (2020) 20(6):339–41. doi: 10.1038/s41577-020-0321-6
158. Yasui F, Kai C, Kitabatake M, Inoue S, Yoneda M, Yokochi S, et al. Prior immunization with severe acute respiratory syndrome (SARS)-associated coronavirus (SARS-CoV) nucleocapsid protein causes severe pneumonia in mice infected with SARS-CoV. J Immunol (2008) 181(9):6337–48. doi: 10.4049/jimmunol.181.9.6337
159. Liu L, Wei Q, Lin Q, Fang J, Wang H, Kwok H, et al. Anti-spike IgG causes severe acute lung injury by skewing macrophage responses during acute SARS-CoV infection. JCI Insight (2019) 4(4). doi: 10.1172/jci.insight.123158
160. Siddiqi HK, Mehra MR. COVID-19 illness in native and immunosuppressed states: A clinical-therapeutic staging proposal. J Heart Lung Transplant (2020) 39(5):405–7. doi: 10.1016/j.healun.2020.03.012
161. Agarwal A, Mukherjee A, Kumar G, Chatterjee P, Bhatnagar T, Malhotra P. Convalescent plasma in the management of moderate covid-19 in adults in India: open label phase II multicentre randomised controlled trial (PLACID Trial). Bmj (2020) 371:m3939. doi: 10.1136/bmj.m3939
162. Joyner MJ, Bruno KA, Klassen SA, Kunze KL, Johnson PW, Lesser ER, et al. Safety Update: COVID-19 Convalescent Plasma in 20,000 Hospitalized Patients. Mayo Clin Proc (2020) 95(9):1888–97. doi: 10.1016/j.mayocp.2020.09.032
163. Su S, Wong G, Shi W, Liu J, Lai ACK, Zhou J, et al. Epidemiology, Genetic Recombination, and Pathogenesis of Coronaviruses. Trends Microbiol (2016) 24(6):490–502. doi: 10.1016/j.tim.2016.03.003
164. Braun J, Loyal L, Frentsch M, Wendisch D, Georg P, Kurth F, et al. SARS-CoV-2-reactive T cells in healthy donors and patients with COVID-19. Nature (2020) 587(7833):270–4. doi: 10.1038/s41586-020-2598-9
165. Song G, He WT, Callaghan S, Anzanello F, Huang D, Ricketts J, et al. Cross-reactive serum and memory B cell responses to spike protein in SARS-CoV-2 and endemic coronavirus infection. bioRxiv (2020). doi: 10.1101/2020.09.22.308965
166. Woldemeskel BA, Kwaa AK, Garliss CC, Laeyendecker O, Ray SC, Blankson JN. Healthy donor T-cell responses to common cold coronaviruses and SARS-CoV-2. J Clin Invest (2020) 130(12):6631–8. doi: 10.1172/JCI143120
167. Nguyen-Contant P, Embong AK, Kanagaiah P, Chaves FA, Yang H, Branche AR, et al. S Protein-Reactive IgG and Memory B Cell Production after Human SARS-CoV-2 Infection Includes Broad Reactivity to the S2 Subunit. mBio (2020) 11(5). doi: 10.1128/mBio.01991-20
168. Mateus J, Grifoni A, Tarke A, Sidney J, Ramirez SI, Dan JM, et al. Selective and cross-reactive SARS-CoV-2 T cell epitopes in unexposed humans. Science (2020) 370(6512):89–94. doi: 10.1126/science.abd3871
169. Masse S, Capai L, Villechenaud N, Blanchon T, Charrel R, Falchi A. Epidemiology and Clinical Symptoms Related to Seasonal Coronavirus Identified in Patients with Acute Respiratory Infections Consulting in Primary Care over Six Influenza Seasons (2014-2020) in France. Viruses (2020) 12(6). doi: 10.3390/v12060630
170. Gorse GJ, Patel GB, Vitale JN, O’Connor TZ. Prevalence of antibodies to four human coronaviruses is lower in nasal secretions than in serum. Clin Vaccine Immunol (2010) 17(12):1875–80. doi: 10.1128/CVI.00278-10
171. WHO. Middle East respiratory syndrome coronavirus (MERS-CoV). (2019). (WHO). Available at: https://www.who.int/emergencies/mers-cov/en/.
172. WHO. Summary of probable SARS cases with onset of illness from 1 November 2002 to 31 July 2003. WHO (2004). Available at: https://www.who.int/csr/sars/country/table2004_04_21/en/.
173. Okba NMA, Muller MA, Li W, Wang C, GeurtsvanKessel CH, Corman VM, et al. SARS-CoV-2 specific antibody responses in COVID-19 patients. medRxiv (2020) 2020.03.18.20038059. doi: 10.1101/2020.03.18.20038059
174. Hicks J, Klumpp-Thomas C, Kalish H, Shunmugavel A, Mehalko J, Denson JP, et al. Serologic cross-reactivity of SARS-CoV-2 with endemic and seasonal Betacoronaviruses. medRxiv (2020). doi: 10.1101/2020.06.22.20137695
175. Ladner JT, Henson SN, Boyle AS, Engelbrektson AL, Fink ZW, Rahee F, et al. Epitope-resolved profiling of the SARS-CoV-2 antibody response identifies cross-reactivity with an endemic human CoV. bioRxiv (2020) 2020.07.27.222943. doi: 10.1101/2020.07.27.222943
176. Lv H, Wu NC, Tsang OT, Yuan M, Perera R, Leung WS, et al. Cross-reactive Antibody Response between SARS-CoV-2 and SARS-CoV Infections. Cell Rep (2020) 31(9):107725. doi: 10.1016/j.celrep.2020.107725
177. Trivedi SU, Miao C, Sanchez JE, Caidi H, Tamin A, Haynes L, et al. Development and Evaluation of a Multiplexed Immunoassay for Simultaneous Detection of Serum IgG Antibodies to Six Human Coronaviruses. Sci Rep (2019) 9(1):1390. doi: 10.1038/s41598-018-37747-5
178. Zost SJ, Gilchuk P, Chen RE, Case JB, Reidy JX, Trivette A, et al. Rapid isolation and profiling of a diverse panel of human monoclonal antibodies targeting the SARS-CoV-2 spike protein. Nat Med (2020) 26(9):1422–7. doi: 10.1101/2020.05.12.091462
179. Ju B, Zhang Q, Ge J, Wang R, Sun J, Ge X, et al. Human neutralizing antibodies elicited by SARS-CoV-2 infection. Nature (2020) 584(7819):115–9. doi: 10.1038/s41586-020-2380-z
180. Duan J, Yan X, Guo X, Cao W, Han W, Qi C, et al. A human SARS-CoV neutralizing antibody against epitope on S2 protein. Biochem Biophys Res Commun (2005) 333(1):186–93. doi: 10.1016/j.bbrc.2005.05.089
181. Elshabrawy HA, Coughlin MM, Baker SC, Prabhakar BS. Human monoclonal antibodies against highly conserved HR1 and HR2 domains of the SARS-CoV spike protein are more broadly neutralizing. PloS One (2012) 7(11):e50366. doi: 10.1371/journal.pone.0050366
182. Wang C, Li W, Drabek D, Okba NMA, van Haperen R, Osterhaus A, et al. A human monoclonal antibody blocking SARS-CoV-2 infection. Nat Commun (2020) 11(1):2251. doi: 10.1038/s41467-020-16452-w
183. Sagar M, Reifler K, Rossi M, Miller NS, Sinha P, White L, et al. Recent endemic coronavirus infection is associated with less severe COVID-19. J Clin Invest (2020). doi: 10.1172/JCI143380
184. Dugas M, Grote-Westrick T, Vollenberg R, Lorentzen E, Brix T, Schmidt H, et al. Less severe course of COVID-19 is associated with elevated levels of antibodies against seasonal human coronaviruses OC43 and HKU1 (HCoV OC43, HCoV HKU1). medRxiv (2020) 2020.10.12.20211599. doi: 10.1101/2020.10.12.20211599
185. Sun K, Chen J, Viboud C. Early epidemiological analysis of the coronavirus disease 2019 outbreak based on crowdsourced data: a population-level observational study. Lancet Digital Health (2020) 2(4):e201–e8. doi: 10.1016/S2589-7500(20)30026-1
186. Shao X, Guo X, Esper F, Weibel C, Kahn JS. Seroepidemiology of group I human coronaviruses in children. J Clin Virol (2007) 40(3):207–13. doi: 10.1016/j.jcv.2007.08.007
187. Nickbakhsh S, Ho A, Marques DFP, McMenamin J, Gunson RN, Murcia PR. Epidemiology of Seasonal Coronaviruses: Establishing the Context for the Emergence of Coronavirus Disease 2019. J Infect Diseases (2020) 222(1):17–25. doi: 10.1093/infdis/jiaa185
188. Davies NG, Klepac P, Liu Y, Prem K, Jit M, Eggo RM. Age-dependent effects in the transmission and control of COVID-19 epidemics. Nat Med (2020) 26:1205–11. doi: 10.1101/2020.03.24.20043018
189. Vatti A, Monsalve DM, Pacheco Y, Chang C, Anaya JM, Gershwin ME. Original antigenic sin: A comprehensive review. J Autoimmun (2017) 83:12–21. doi: 10.1016/j.jaut.2017.04.008
190. Zompi S, Harris E. Original antigenic sin in dengue revisited. Proc Natl Acad Sci U S A (2013) 110(22):8761–2. doi: 10.1073/pnas.1306333110
191. Rothman AL. Immunity to dengue virus: a tale of original antigenic sin and tropical cytokine storms. Nat Rev Immunol (2011) 11(8):532–43. doi: 10.1038/nri3014
192. Meade P, Kuan G, Strohmeier S, Maier HE, Amanat F, Balmaseda A, et al. Influenza Virus Infection Induces a Narrow Antibody Response in Children but a Broad Recall Response in Adults. mBio (2020) 11(1). doi: 10.1128/mBio.03243-19
193. Devarajan P, Swain SL. Original Antigenic Sin: Friend or Foe in Developing a Broadly Cross-Reactive Vaccine to Influenza? Cell Host Microbe (2019) 25(3):354–5. doi: 10.1016/j.chom.2019.02.009
194. Aydillo T, Rombauts A, Stadlbauer D, Aslam S, Abelenda-Alonso G, Escalera A, et al. Antibody Immunological Imprinting on COVID-19 Patients. medRxiv (2020) 2020.10.14.20212662. doi: 10.1101/2020.10.14.20212662
195. Westerhuis BM, Aguilar-Bretones M, Raadsen MP, de Bruin E, Okba NMA, Haagmans BL, et al. Severe COVID-19 patients display a back boost of seasonal coronavirus-specific antibodies. medRxiv (2020) 2020.10.10.20210070. doi: 10.1101/2020.10.10.20210070
196. Randolph HE, Barreiro LB. Herd Immunity: Understanding COVID-19. Immunity (2020) 52(5):737–41. doi: 10.1016/j.immuni.2020.04.012
197. Fine P, Eames K, Heymann DL. “Herd immunity”: a rough guide. Clin Infect Dis (2011) 52(7):911–6. doi: 10.1093/cid/cir007
198. Severe Outcomes Among Patients with Coronavirus Disease 2019 (COVID-19) - United States, February 12-March 16, 2020. MMWR Morb Mortal Wkly Rep (2020) 69(12):343–6. doi: 10.15585/mmwr.mm6912e2
199. Anderson RM, May RM. Vaccination and herd immunity to infectious diseases. Nature (1985) 318(6044):323–9. doi: 10.1038/318323a0
200. Liu Y, Gayle AA, Wilder-Smith A, Rocklov J. The reproductive number of COVID-19 is higher compared to SARS coronavirus. J Travel Med (2020) 27(2). doi: 10.1093/jtm/taaa021
201. WHO. Report of the WHO-China Joint Mission on Coronavirus Disease 2019 (COVID-19) 2020 (2020). Available at: https://www.who.int/docs/default-source/coronaviruse/who-china-joint-mission-on-covid-19-final-report.pdf. 24 February 2020.
202. Brett T, Rohani P. COVID-19 herd immunity strategies: walking an elusive and dangerous tightrope. medRxiv (2020). doi: 10.1101/2020.04.29.20082065
203. Arora RK, Joseph A, Van Wyk J, Rocco S, Atmaja A, May E, et al. SeroTracker: a global SARS-CoV-2 seroprevalence dashboard. Lancet Infect Dis (2020). doi: 10.1016/S1473-3099(20)30631-9
204. Stringhini S, Wisniak A, Piumatti G, Azman AS, Lauer SA, Baysson H, et al. Seroprevalence of anti-SARS-CoV-2 IgG antibodies in Geneva, Switzerland (SEROCoV-POP): a population-based study. Lancet (2020) 396(10247):313–9. doi: 10.1016/S0140-6736(20)31304-0
205. Ward H, Atchison CJ, Whitaker M, Ainslie KEC, Elliott J, Okell LC, et al. Antibody prevalence for SARS-CoV-2 in England following first peak of the pandemic: REACT2 study in 100,000 adults. medRxiv (2020) 2020.08.12.20173690. doi: 10.1101/2020.08.12.20173690
206. Folkhälsomyndigheten. Nya resultat om antikroppar mot covid-19 i olika grupper i befolkningen. Folkhälsomyndigheten (2020). Available at: https://www.folkhalsomyndigheten.se/nyheter-och-press/nyhetsarkiv/2020/september/nya-resultat-om-antikroppar-mot-covid-19-i-olika-grupper-i-befolkningen/.
207. Centers for Disease Control and Prevention. Commercial laboratory Seroprevalence Survey Data. CDC (2020). Available at: https://www.cdc.gov/coronavirus/2019-ncov/cases-updates/commercial-lab-surveys.html#interpreting-serology-results.
208. Pollán M, Pérez-Gómez B, Pastor-Barriuso R, Oteo J, Hernán MA, Pérez-Olmeda M, et al. Prevalence of SARS-CoV-2 in Spain (ENE-COVID): a nationwide, population-based seroepidemiological study. Lancet (2020) 396(10250):535–44. doi: 10.1016/S0140-6736(20)32266-2
209. Héma-Quebec. Blood donor seroprevalence study : 2.23 % of quebec adults contracted covid-19. Héma-Québec (2020). Available at: https://www.hema-quebec.qc.ca/publications/communiques/archives/2020/communiques-2020/etude-seroprevalence-resultats.en.html.
210. Ontario). OafHPaPPH. Weekly epidemiologic summary: COVID-19 Seroprevalence in Ontario : March 27, 2020 to June 30, 2020. Toronto, ON: Queen’s printer for Ontario (2020). p. 10.
211. Skowronski DM, Sekirov I, Sabaiduc S, Zou M, Morshed M, Lawrence D, et al. Low SARS-CoV-2 sero-prevalence based on anonymized residual sero-survey before and after first wave measures in British Columbia, Canada, March-May 2020. medRxiv (2020) 2020.07.13.20153148. doi: 10.1101/2020.07.13.20153148
212. Valenti L, Bergna A, Pelusi S, Facciotti F, Lai A, Tarkowski M, et al. SARS-CoV-2 seroprevalence trends in healthy blood donors during the COVID-19 Milan outbreak. medRxiv (2020) 2020.05.11.20098442. doi: 10.1101/2020.05.11.20098442
213. Chang L, Hou W, Zhao L, Zhang Y, Wang Y, Wu L, et al. The prevalence of antibodies to SARS-CoV-2 among blood donors in China. medRxiv (2020) 2020.07.13.20153106. doi: 10.2139/ssrn.3633170
214. Orlowski EJW, Goldsmith DJA. Four months into the COVID-19 pandemic, Sweden’s prized herd immunity is nowhere in sight. J R Soc Med (2020) 113(8):292–8. doi: 10.1177/0141076820945282
215. Carfì A, Bernabei R, Landi F. Persistent Symptoms in Patients After Acute COVID-19. Jama (2020) 324(6):603–5. doi: 10.1001/jama.2020.12603
216. Yelin D, Wirtheim E, Vetter P, Kalil AC, Bruchfeld J, Runold M, et al. Long-term consequences of COVID-19: research needs. Lancet Infect Dis (2020) 20(10):1115–7. doi: 10.1016/S1473-3099(20)30701-5
217. Rimmer A. Covid-19: Impact of long term symptoms will be profound, warns BMA. BMJ (2020) 370:m3218. doi: 10.1136/bmj.m3218
218. Rapkiewicz AV, Mai X, Carsons SE, Pittaluga S, Kleiner DE, Berger JS, et al. Megakaryocytes and platelet-fibrin thrombi characterize multi-organ thrombosis at autopsy in COVID-19: A case series. EClinicalMedicine (2020) 24:100434. doi: 10.1016/j.eclinm.2020.100434
219. Papachristodoulou E, Kakoullis L, Parperis K, Panos G. Long-term and herd immunity against SARS-CoV-2: implications from current and past knowledge. Pathog Dis (2020) 78(3). doi: 10.1093/femspd/ftaa025
220. Johns Hopkins Center for Health Security. Serology-based tests for COVID-19. (2020). Available at: https://www.centerforhealthsecurity.org/resources/COVID-19/serology/Serology-based-tests-for-COVID-19.html#sec4.
221. US Food and Drug Administration. EUA Authorized Serology Test Performance. (2020). Available at: https://www.fda.gov/medical-devices/coronavirus-disease-2019-covid-19-emergency-use-authorizations-medical-devices/eua-authorized-serology-test-performance.
222. Hayes Emily L. FDA pulls plug on wayward coronavirus antibody tests. (2020). Available at: https://www.labpulse.com/index.aspx?sec=sup&sub=mic&pag=dis&ItemID=801254.
223. GenomeWeb. Coronavirus Test Tracker: Commercially Available COVID-19 Diagnostic Tests. (2020). Available at: https://www.360dx.com/coronavirus-test-tracker-launched-covid-19-tests.
Keywords: SARS-CoV-2, coronavirus, COVID-19, serology, humoral immunity, serological assays, original antigenic sin
Citation: Galipeau Y, Greig M, Liu G, Driedger M and Langlois M-A (2020) Humoral Responses and Serological Assays in SARS-CoV-2 Infections. Front. Immunol. 11:610688. doi: 10.3389/fimmu.2020.610688
Received: 26 September 2020; Accepted: 23 November 2020;
Published: 18 December 2020.
Edited by:
Edward K. L. Chan, University of Florida, United StatesReviewed by:
Matthew Miller, McMaster University, CanadaKevin John Selva, The University of Melbourne, Australia
Copyright © 2020 Galipeau, Greig, Liu, Driedger and Langlois. This is an open-access article distributed under the terms of the Creative Commons Attribution License (CC BY). The use, distribution or reproduction in other forums is permitted, provided the original author(s) and the copyright owner(s) are credited and that the original publication in this journal is cited, in accordance with accepted academic practice. No use, distribution or reproduction is permitted which does not comply with these terms.
*Correspondence: Marc-André Langlois, bGFuZ2xvaXNAdW90dGF3YS5jYQ==
†These authors have contributed equally to this work