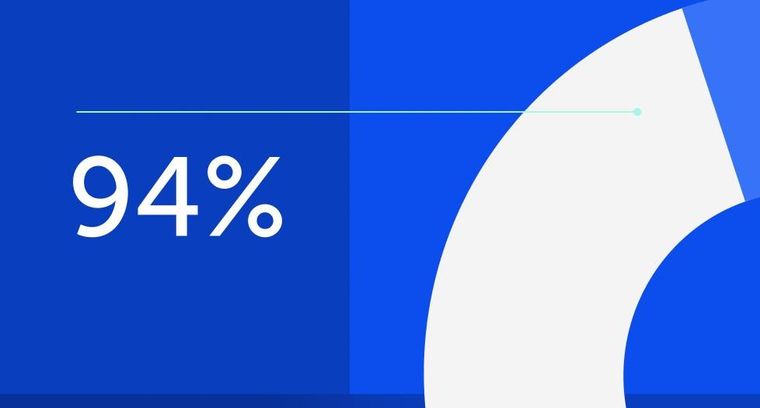
94% of researchers rate our articles as excellent or good
Learn more about the work of our research integrity team to safeguard the quality of each article we publish.
Find out more
ORIGINAL RESEARCH article
Front. Immunol., 07 December 2020
Sec. Nutritional Immunology
Volume 11 - 2020 | https://doi.org/10.3389/fimmu.2020.607609
This article is part of the Research TopicImpact of Early Life Nutrition on Immune System Development and Related Health Outcomes in Later LifeView all 18 articles
The impact of human milk (HM) or dairy milk-based formula (MF) on the large intestine’s metabolome was not investigated. Two-day old male piglets were randomly assigned to HM or MF diet (n = 26/group), from postnatal day (PND) 2 through 21 and weaned to a solid diet until PND 51. Piglets were euthanized at PND 21 and PND 51, luminal contents of the cecum, proximal (PC) and distal colons (DC), and rectum were collected and subjected to metabolomics analysis. Data analyses were performed using Metaboanalyst. In comparison to MF, the HM diet resulted in higher levels of fatty acids in the lumen of the cecum, PC, DC, and rectum at PND 21. Glutamic acid was greater in the lumen of cecum, PC, and DC relative to the MF group at PND 21. Also, spermidine was higher in the DC and rectal contents of HM relative to MF at PND 21. MF diet resulted in greater abundances of amino acids in the cecal lumen relative to HM diet at PND 21. Additionally, several sugar metabolites were higher in various regions of the distal gut of MF fed piglets relative to HM group at PND 21. In contrast, at PND 51, in various regions there were higher levels of erythritol, maltotriose, isomaltose in HM versus MF fed piglets. This suggests a post weaning shift in sugar metabolism that is impacted by neonatal diet. The data also suggest that infant diet type and host-microbiota interactions likely influence the lower gut metabolome.
Human milk (HM) contains a diversity of bioactive components including lipids, human milk oligosaccharides (HMOs), a variety of cytokines, and microbiota that can influence the child’s development, immune function, and microbiota colonization during early life (1–3). Although studies have indicated the positive impact of HM diet on immune function (4, 5), microbiota composition (6), and child’s growth (7), mechanisms behind these outcomes are poorly understood due to limitations associated with gut sample collection from infants. During early life, cow’s milk-based formula (MF) has been chosen as an alternative to human milk (8), but the degree to which MF feeding alters the gastrointestinal tract (GI) milieu relative to HM remains to be fully characterized.
The use of omics technologies such as metagenomics and metabolomics provide platforms to gain new insights about the mechanisms underlying diet-associated differences in the infant’s growth and overall health during the neonatal period. For instance, microbiota analysis of infant’s stool demonstrated that HM diet shapes microbiota colonization and enriches bacterial species Bifidobacteria and Bacteroides during exclusive HM feeding relative to formula diet (9, 10). Furthermore, previous studies using metabolomics investigated fecal and serum metabolite profiles of HM versus MF fed infants (11–14). While providing valuable insights, the GI bioregional aspects of HM and MF feeding have remained difficult to study.
We and others reported the use of animal models (primate and piglets) to investigate the impact of MF diet on gut microbiota, immune system, and metabolism (15–22). These models are valuable tools to explore the effects of neonatal regimes on gastrointestinal tract development and maturation (18, 23–25), since they allow the collection of multiple tissues and GI regions for large scale analysis which is limited in human studies (26). Our group developed a piglet model under controlled conditions (i.e., an isocaloric diet of HM or MF, vivarium housing), and have demonstrated that HM-fed piglets had a higher abundance of Bacteroides which is similar to the microbiota composition of breast-fed infants (17). Most recently, using the same piglet model our group reported that formula diet could alter the epithelial barrier integrity through disruption of tight junctions in the small intestine of formula-fed piglets compared to the HM-fed (18). These findings are indicative that a piglet model is a promising tool to evaluate the influence of neonatal diet on gut metabolism. Here, we present a comparative metabolomics analysis of the distal gastrointestinal tract of piglets fed HM or MF diet during the first 21 days of life and post-weaning neonatal diet at day 51.
The animal study was conducted in accordance with the ethical guidelines for animal research approved by the Institutional Animal Care and Use Committee at the University of Arkansas for Medical Sciences. The detailed experimental design as well as the diet composition were previously published (19). Briefly, White Dutch Landrace Duroc male piglets within 2-d old were randomly assigned to two groups (n = 26/group), fed an isocaloric diet of HM (Mother’s Milk Bank of North Texas), or a dairy-based MF (milk formula; Similac Advance powder; Ross products, Abbott Laboratories, Columbus, OH) to meet the nutrient requirements of growing pigs as per the guidelines published by the National Research Council (NRC) (27). At postnatal day (PND) 14 complementary food (i.e., solid pellets) (starter pellets; Teklad, TD 140608; Harlan Laboratories) was introduced to the piglets and weaned to ad libitum solid pellets from PND21 to PND51 (19). Piglets were immunized on PND 21 and PND 35 with oral administration of 100 µg of cholera toxin (C8052, Millipore Sigma) and 100 µg of cholera toxin subunit B (CTB; C9903, Millipore Sigma). Piglets also received The DAPTACEL [diphtheria, tetanus, pertussis (DTaP)] vaccine (0.5 mL; Arkansas Children’s Hospital pharmacy) by intramuscular injection. Control piglets received vehicle.
At PND 21 and 51 piglets were euthanized after anesthetization with isoflurane, followed by exsanguination. Cecum, proximal colon, distal colon, and rectum contents were collected within a scintillation vial by pinching the tissue and sliding the constriction toward the open end. All samples were immediately snap-frozen in liquid nitrogen and stored at −80°C until further analysis.
Cecum, PC, DC, and rectum contents were subjected to metabolomics analyses using gas chromatography/mass spectrometry (GC/MS) at the West Coast Metabolomics Center at University of California Davis. Approximately 4 mg of contents from experimental samples from each region were used to have a pool for quality control (QC) during the process of the metabolome data. Detailed GC/MS instrument conditions were reported previously (28). Briefly, a total of 0.5 µL of each sample was injected splitless into an Agilent 6890 GC equipped with a Gerstel automatic liner exchange system (ALEX) that includes a multipurpose sample (MPS2) dual rail, and a Gerstel CIS cold injection system (Gerstel, Muehlheim, Germany). The gas chromatograph was controlled using Leco ChromaTOF software. Constituted of helium mobile phase, the gas flow rate through a 30 m long, 0.25 mm i.d. Rtx-5Sil MS column (0.25 μm 95% dimethyl 5% diphenyl polysiloxane film) with additional 10 m integrated guard column (Restek, Bellefonte PA) was 1 mL/min. The transfer line temperature between gas chromatograph and mass spectrometer was set to 280°C. Electron impact was generated by a 70-eV ionization and with an ion source temperature of 250°C. Acquisition rate is 17 spectra/second, with a scan mass range of 85–500 Da. Compounds were identified by comparison with Fiehn lab BinBase database annotations (29), database identifier [i.e., InChI key (30)], the compound annotation metadata (i.e., retention index, quantification mass, BinBase identifier, and mass spectrum), and PubChem annotation (31). A list of peak heights, retention time and mass to charge (m/z) were obtained. 549 metabolites were detected in all samples, including 282 annotated and 267 unknown (non-annotated) metabolites. The unknown metabolites were excluded from the current analysis. The raw data was processed and analyzed in MetaboAnalyst 4.0 (32). On postnatal day 51, diet and immunization interactions were assessed by Permutational multivariate ANOVA (PERMANOVA) with 999 permutations (Supplemental Table 1). No Diet × immunization interaction was observed for cecum (P > 0.25), PC and DC (P ≥ 0.42), and for rectum content metabolites (P = 0.11). Therefore, control and immunized data were pooled in the analysis of the PND 51. The QC samples were subjected to multivariate analysis in MetaboAnalyst to check the precision of the metabolomics analysis. The supervised partial least squares discriminant analysis (PLS-DA) score plot for the QC samples (Supplemental Figure 1) showed the tight clustering of the QC samples indicating the precise outcome from the metabolites process. Metabolites peak intensities were normalized by the sum of all identified metabolites (33) and log transformed prior to multivariate statistical analysis (34). The PLS-DA score plots were used to see the overall difference between metabolite profiles of HM and MF groups followed by Pattern Hunter analysis in MetaboAnalyst to detect the significant differences in metabolites between groups. A metabolite was considered to be statistically different when P - value ≤ 0.05, Benjamini-Hochberg adjusted false discovery rate (FDR) ≤ 0.15, and variable importance in projection (VIP) score > 1.0 (34, 35). Based on the identification of the significantly altered metabolites in HM and MF-fed groups, we calculated the fold change (FC) for each metabolite.
Previously we have demonstrated that microbiota changes were predominant in the large intestine of piglets fed the MF diet relative to the HM group (17). Thus, to evaluate the impact of early diet on the large intestine metabolome, the cecum, proximal colon, distal colon, and rectum contents were examined at PND 21. The PLS-DA model of metabolite showed robust separation of dietary groups at PND 21 in cecal, PC, DC, and rectal regions of the gastrointestinal tract (Figures 1A–D).
Figure 1 Two-dimensional scores plot of partial least squares discriminant analysis (PLS-DA) model showing how distal gut content abundances of annotated metabolites can discriminate human milk (HM) versus milk formula (MF) feeding groups during the neonatal period in piglets. Panels depict (A) cecum (B), proximal colon (C), distal colon, and (D) rectal contents at postnatal day (PND) 21. PLS-DA scores (i.e., individual piglet scores) for PLS-DA components (dimensions) 1 and 2 are displayed. Shadows with color are 95% confidence regions. Pink circles indicate individual HM-fed piglets and green circles indicate MF-fed piglets. Sample numbers were n = 8–11 per group.
At PND 21, within the lumen of large intestine and rectum, a total of 123 cecal, 111 PC, 95 DC, and 62 rectal metabolites from diverse chemical classes including fatty acids, amino acids, lipids, carbohydrates, vitamins, steroids, and co-metabolites were significantly different between HM and MF diet-fed piglets (Tables 1–7 and Supplemental Table 2). The complete list of all detected metabolites (including non-annotated “unknown” metabolites) within each intestinal region is presented in the Supplementary Table 6.
Table 1 Average abundances [quantifier ion (quantion) intensities] of fatty acids significantly different when comparing human milk (HM) or milk formula (MF) diet groups, in cecum, proximal colon, distal colon, and rectum contents of piglets at postnatal day (PND) 21.
Table 2 Average abundances [quantifier ion (quantion) intensities] of polyamines significantly different when comparing human milk (HM) or milk formula (MF) diet groups, in cecum, proximal colon, distal colon, and rectum contents of piglets at postnatal day (PND) 21.
Table 3 Average abundances [quantifier ion (quantion) intensities] of sugar metabolites significantly different when comparing human milk (HM) or milk formula (MF) diet groups, in cecum, proximal colon, distal colon, and rectum contents of piglets at postnatal day (PND) 21.
Table 4 Average abundances [quantifier ion (quantion) intensities] of amino acids significantly different when comparing human milk (HM) or milk formula (MF) diet groups, in cecum, proximal colon, distal colon, and rectum contents of piglets at postnatal day (PND) 21.
Table 5 Average abundances [quantifier ion (quantion) intensities] of cholesterol and bile acids significantly different when comparing human milk (HM) or milk formula (MF) diet groups, in cecum, proximal colon, distal colon, and rectum contents of piglets at postnatal day (PND) 21.
Table 6 Average abundances [quantifier ion (quantion) intensities] of tryptophan metabolites significantly different when comparing human milk (HM) or milk formula (MF) diet groups, in cecum, proximal colon, distal colon, and rectum contents of piglets at postnatal day (PND) 21.
Table 7 Average abundances [quantifier ion (quantion) intensities] of sugar metabolites (erythritol, lyxose, xylitol, xylose, pentose, xylulose, ribose, maltotriose, isomaltose), tryptophan metabolites (indole-3-propionic acid), and fatty acids (behenic acid) significantly different when comparing human milk (HM) or milk formula (MF) diet groups, in cecum, proximal colon, distal colon, and rectum contents of piglets at postnatal day (PND) 51.
The fatty acids myristic, palmitic, linolenic, linoleic, oleic, and palmitoleic were the common metabolites identified throughout the lumen of cecum, PC, DC, and rectum at PND 21, which had greater abundance in the HM than in the MF group. In the lumen of cecum, the saturated fatty acid stearic acid was greater in the HM-fed group relative to the MF group (Table 1). In the PC and DC of HM fed piglets, the fatty acids cis-gondoic acid was higher relative to the MF group (Table 1). In addition, the fatty acids cis-gondoic had greater abundance in the DC lumen of HM than MF-fed piglets (Table 1). Spermidine was another metabolite common to the DC and rectal lumen that was higher in the HM compared to the MF-fed piglets (Table 2). However, Putrescine was lower in HM cecal lumen in comparison to MF group.
The carbohydrates 1, 5-anhydroglucitol, galactitol, sorbitol, and fructose were greater in the DC contents of HM-fed relative to MF-fed piglets, while the carbohydrates galactose-6-phosphate and raffinose had greater abundances in the cecal, PC, and DC lumen of MF relative to HM-fed piglets (Table 3). Isomaltose, ribitol, and maltotriose were greater in the cecal contents of MF relative to the HM group. In addition, 1, 5-anhydroglucitol, mannose and maltotriose were higher in rectal contents in MF group relative to HM group. The essential amino acids histidine, valine, and leucine were greater in the cecal lumen and rectal contents of MF-fed piglets relative to the HM group (Table 4). Additionally, threonine, isoleucine, and phenylalanine were greater in the rectal contents of the MF-fed group compared to HM-group. While the non-essential amino acids glycine and proline were greater in the rectal contents, and taurine and cysteine were greater in the cecal contents of MF-fed compared to the HM-fed piglets. In rectal contents, a higher abundance of the amino acids N-acetylornithine, and N-acetylaspartic acid was observed in the HM group (Table 3). However, glutamic acid was higher in the HM lumen of cecal, PC, and DC while N-acetyl aspartic acid was higher in PC, DC and rectal contents relative to MF-fed piglets.
Cholesterol was significantly higher in the MF group in cecal, PC, and DC lumen (Table 5). Interestingly, secondary bile acid deoxycholic acid had greater abundance throughout the 4 regions of the distal gut in comparison to HM-fed piglets. Also, the primary bile acid chenodeoxycholic acid was higher in the luminal contents of PC and DC in the MF group relative to the HM group.
The metabolites indole-3-propionic acid and 3-hydroxyphenylacetic acid had greater abundance in MF-fed piglets relative to the HM group in the cecal lumen. Within the DC lumen, 5-hydroxy-3-indoleacetic acid and tryptophan were higher in the HM than in the MF group. Additionally, the tryptophan metabolite 5-hydroxy-3-indoleacetic acid was greater in the rectum of the HM relative to the MF group (Table 6).
PLS-DA plots demonstrated that the distribution of metabolites had less separation between HM and MF groups at PND 51 (Figures 2A–D), except for the rectal contents that had a robust separation of the metabolite profile between HM and MF groups. At PND 51 between HM and MF fed piglets, 15 metabolites were significantly different in cecum and PC, 37 in DC, and 21 in the rectum by using the P < 0.05 and a VIP > 1.0 criteria (Supplemental Table 3). The lumen of the cecum of HM fed piglets had higher abundance of indole-3-propionic acid relative to the MF-fed piglets. The sugar alcohol erythritol was a common metabolite in the cecum, DC, and rectum, with higher abundance in the HM group in comparison to the MF group. Additionally, behenic acid was a common fatty acid in the DC and rectal lumen which was higher in the HM-fed relative to the MF-fed piglets at PND 51 (Table 7).
Figure 2 Two-dimensional scores plot of partial least squares discriminant analysis (PLS-DA) model showing how distal gut content abundances of annotated metabolites can discriminate human milk (HM) versus milk formula (MF) feeding groups during the neonatal period in piglets. Panels depict (A) cecum (B), proximal colon (C), distal colon, and (D) rectal contents at postnatal day (PND) 51. PLS-DA scores (i.e., individual piglet scores) for PLS-DA components (dimensions) 1 and 2 are displayed. Shadows with color are 95% confidence regions. Pink circles indicate individual HM-fed piglets and green circles indicate MF-fed piglets. Sample numbers were n = 9–15 per group.
At PND 21, serum metabolome revealed higher abundance of threonic acid and cysteine in the MF relative to the HM fed group. While palmitoleic acid was higher in the HM group. At PND 51, the HM diet resulted in greater abundances of sugar metabolites including maltotriose and xylitol, and greater indole-3-propionic acid relative to MF-fed group. The complete list of serum metabolites impacted by HM and MF diets are presented in the Supplemental Table 4.
The present study provides metabolite profiles in the cecum, colon, and rectal lumen of HM versus MF feeding regimens in a porcine model at PND 21 and PND 51. We found that diet has a pronounced effect on metabolite profiles in the lumen of the cecum, PC, DC, and rectum at PND 21 (pre-weaning) but an attenuated effect at PND 51 (~1-month post-weaning). We observed a greater number of metabolite changes in the luminal region of the cecum of HM-fed piglets compared to the MF group at PND 21. A greater abundance of fatty acids and polyamines was observed in HM, while amino acids were higher in MF at PND 21. The persistent effect of the neonatal diet was observed at PND 51 with altered sugar metabolism in HM versus MF fed piglets.
Of particular note was the observation that HM feeding impacted tryptophan metabolism differently than MF feeding, at PND 21. The majority of ingested protein is digested and absorbed by the small intestine (36); however, a significant amount of proteins and amino acids may reach the colon, which is degraded by different microbial species (37). Amino acids in the lower gut may also derive in part from the host (e.g., sloughed tissue, mucous, and epithelial cells from the lining of the intestines) (38–40). In the lumen of DC, tryptophan was higher in the HM-fed group. In addition, a derivative of indole-3-acetic acid (IAA), 5-hydroxy-3-indole acetic acid, was greater in the DC and rectum of HM-fed piglets. Interestingly, we have shown that IAA concentration was also higher in the feces of HM-fed infants at 3 months of age in comparison to formula fed infants (41). Bacteroides genera have been shown to convert tryptophan to indole-3-acetic acid. In support of this notion, we have reported a higher abundance of genera Bacteroides in infants fed human milk and a higher abundance of genera from class Bacteroidia in the rectal lumen of HM fed piglets (17, 41). These results suggest that tryptophan in the HM group is likely metabolized by distal gut microbiota. In addition, bioactive microbial tryptophan metabolites, indole, indole-3-propionic acid, and IAA have been reported to modulate inflammatory response by promoting IL-22 production in the gastrointestinal tract of mice through the activation of aryl hydrocarbon receptor (AhR) (42, 43). We speculate that the higher tryptophan metabolite levels with human milk feeding promotes the interaction with the host-microbiota which might dampen inflammation.
Neonatal diet also resulted in a divergent fatty acid profile at PND 21 in the large intestine. The human milk lipid profile is variable, and several factors including maternal age, lactation stage, metabolic disorders, maternal diet, among others can modulate the lipid composition (44). HM is composed of more than 200 fatty acids including high levels of oleic and linoleic acids, and these are likely obtained from the mother’s diet (45). Essential fatty acids such as linoleic and linolenic cannot be synthesized by the mammalian body from the precursor oleic acid due to the lack of specific enzymes (Δ12 and Δ15-desaturase and hydrogenase), thus adequate intake of these fatty acids through dietary regimens are needed (46). Furthermore, the fatty acid composition of monogastric animals (i.e., piglets) also depends on the dietary intake of fatty acids (47). In our study, throughout the 4 regions evaluated (from cecum to rectum) the linolenic and linoleic essential fatty acids were higher in the HM fed piglets relative to MF at PND 21. Additionally, other fatty acids, myristic, palmitic, oleic, and palmitoleic were common metabolites identified throughout the large intestine of HM-fed relative to the MF-fed group. Studies from our laboratory and others identified higher circulating fatty acids in the HM group. For example, palmitoleic acid was higher in HM-fed serum in comparison to MF-fed piglets (Supplemental Table 4), and free fatty acids such as palmitic acid, oleic acid, and stearic acid were higher in the plasma of infants fed HM relative to formula-fed (11). It is suggestive that fatty acids are delivered to infants from HM and in part from the mother’s diet. Dietary fatty acids have been shown to exert immunomodulatory effects during inflammatory conditions in humans (48) and in mouse models (49, 50). For example, linolenic acid had an anti-inflammatory effect by decreasing the secretion of the pro-inflammatory IL-6 in an intestinal model using the Caco-2-cell line (51). Additionally, essential fatty acids have been shown to be transferred from sow milk into the piglets’ enteric tissues, which might play a role in the immune response and in the epithelial integrity (52). For instance, polyunsaturated fatty acids supplementation to pregnant sows resulted in lower markers of inflammation in the post weaning period of piglets (53). These data, suggest that fatty acids from mothers’ milk exhibit immune protection to infants.
Human milk contains low levels of putrescine compared to spermine and spermidine in term and preterm milk (54). Interestingly, we observed a significantly lower level of putrescine in the lumen of the cecum while spermidine was significantly higher in the lumen of DC and rectum in HM relative to MF. It is possible that HM is the source for these polyamines observed in the distal gut and may provide benefits to infants by various mechanisms. For example, spermine and spermidine play a role in the maintenance of the colonic (55) and intestinal mucosa in mammals (56). Spermidine is considered essential for postnatal intestinal maturation and it has been reported to be higher in human milk than in formulas (57, 58). In addition, spermidine supplementation suppresses inflammatory DC function and systemic inflammation in the psoriasis mouse model (59). Interestingly, human infants fed dairy-based formula had greater levels of the pro-inflammatory molecules (IL8 and IL1β) in the feces compared to HM-fed infants at 1-month (60) and our most recent report suggested higher inflammatory status in MF than HM fed piglets (18). In addition, spermidine has been shown to play a role in autophagy to rejuvenate memory B cell response in older individuals (61). Reduced B cell function causes poor vaccination efficacy and likely a higher incidence of infections. Several studies have demonstrated that HM fed infants have stronger vaccine response and lower respiratory tract infections during the infancy period (1, 2, 62–64). Moreover, in the same piglets we observed stronger vaccine response in HM versus MF fed piglets (19). Also, infant formula supplemented with polyamines increased the number of Bifidobacterium species in the large intestine of mice resulting in greater mucin production (65). Thus, the greater level of spermidine upon human milk feeding may benefit the infants by maintaining colon health, microbiota composition, and immune function.
While human milk cholesterol content varies from 90 to 150 mg/L, infant formulas have lower cholesterol content between 20–40 mg/L originated from dairy milk fat (66). Adequate cholesterol dietary intake is essential, especially for growing infants, for the production of steroid hormones, brain development, and lipoprotein metabolism (67, 68). However, a balance between cholesterol absorption and synthesis is required for maintaining whole-body cholesterol homeostasis (69). Formula-fed infants (70, 71) and piglets (24, 72, 73) have been shown to have higher hepatic cholesterol synthesis and fecal bile acid excretion. Fecal sterol excretion followed by intestinal breakdown can be associated with reduced intestinal absorption of cholesterol (68). In the current piglet study, the greater cholesterol detected in the cecum and colon contents of the MF group might be associated with a feedback mechanism (e.g., increased cholesterol synthesis) in response to the low dietary cholesterol uptake. In addition, the cholesterol synthesized in the liver is converted to primary bile acids such as cholic acid (CA), and chenodeoxycholic acid (CDCA) (74). These primary bile acids synthesized from cholesterol in hepatocytes are conjugated to the amino acids taurine or glycine for further biliary secretion (75). In our study, the greater abundance of the bile acids CDCA in the PC and DC lumen was associated with higher levels of amino acids taurine and glycine in the cecal contents of the MF group. In the distal colon, solely gut bacterial bile salt hydrolase (BSH) deconjugates bile acids to form the secondary bile acids deoxycholic acid (DCA) and lithocholic acid (LCA) (76). Importantly, we observed higher DCA in all 4 regions of the distal gut with MF diet suggesting as one of the mechanisms of maintaining cholesterol homeostasis is likely by excretion of secondary bile acids. The implications of a high level of cholesterol and bile acids in the gut can be speculated based on previously published literature (77). For example, bile acids can regulate the epithelial barrier integrity through activation of the farnesoid X receptor (FXR) on intestinal epithelial cells (74). DCA has been shown to induce gut dysbiosis, disrupt bile acid enterohepatic circulation, and promote intestinal inflammation (78). In addition, taurine has been shown to activate Nlrp6 inflammasome and induce the release of the proinflammatory IL-18 by the intestinal epithelial cells (79). Moreover, the accumulation of DCA in the large intestine has been associated with passive absorption through the colon mucosa (76). Overall, these data suggest that cholesterol and bile acid homeostasis is impacted by the formula diet.
Glutamic acid (glutamate), glutamine, and taurine are the most abundant free amino acids (FAA) in human milk, accounting for approximately 50% of total FAA (80–82) while in dairy-based formulas taurine is the most prevalent FAA (83). In this study, throughout the distal gut regions, higher glutamic acid was detected in HM-fed piglets, likely derived from HM (82, 84). Glutamate intake through the HM diet might benefit the overall neonatal gut health since it has been reported to function as a major energy substrate for intestinal cells (84, 85). Thus, non-essential amino acids intake through human milk might supply infants with readily available nitrogen-compounds. Previous studies demonstrated that standard infant formulas have a lower concentration of free amino acid compared to breastmilk (80, 83) while hydrolysate formulas have a higher amount of amino acids relative to regular formulas (86). In our study, several amino acids (i.e., valine, cysteine, isoleucine, leucine, methionine, cysteine, glycine, histidine, and phenylalanine) were higher in the cecal and rectal contents of MF-fed piglets relative to HM at PND 21, likely due to higher amount of protein in formula. Interestingly, previous studies demonstrated higher levels of circulatory amino acids in formula-fed relative to breastfed infants likely due to higher protein intake with formula diet (11, 12, 87, 88). While we only observed higher cysteine levels in the serum of MF fed piglets (Supplemental Table 4), it is possible that in our piglets fasting conditions (8 h) were impacting the circulatory amino acid pool as most of the infant studies measured metabolites after 2–3 h of fasting (11).
Sugar metabolism was impacted by the formula diet relative to the HM diet in piglets. Several metabolites (UDP-glucuronic acid, lyxose, ribonic acid, maltrotriose, UDP-N-acetyl glucosamine, pyruvic acid, threonic acid, raffinose, melibiose, erythrose, xylulose, panose, maltose, mannose) were significantly higher in the MF group relative to the HM group in different regions of distal gut at 8 h of fasting. Interestingly, serum threonic acid (Supplemental Table 4) and urinary threonic acid, ribonic acid, and maltotriose (Supplemental Table 5) were also significantly higher in MF relative to HM piglets. Notably, galactose concentration was higher in infant formulas compared to mature human milk (89). In our piglet model MF diet has impacted the carbohydrate metabolism as observed by a higher abundance of galatcose-6-phosphate in the cecum and colon followed by higher glucose-1-phosphate in the cecum of MF-fed piglets at PND 21. Based on previous infant literature and our current data, it is suggestive that formula-fed piglets exhibited a trend to use more of the energy from carbohydrate while HM-fed piglets may use fat as the energy fuel during exclusive neonatal feeding (i.e., PND 21) (11, 13). Additionally, others demonstrated that carbohydrate intake was lower in breastfed infants at 3 and 6 months compared to formula-fed infants (90). Also, metabolites shared between urine and large intestine suggest that these could serve as biomarkers of host health and likely microbial metabolism.
Previous metabolomics studies of infants have shown that the introduction of complementary food minimizes metabolic profile differences in serum while there are clear metabolic changes upon exclusively HM or MF feeding in infants (11). Similarly, we observed less separation of metabolite profile at PND 51 between HM and MF fed piglets. However, sugar metabolites such as erythritol, lyxose, xylitol, xylose, pentose, xylulose, ribose, maltotriose, isomaltose were higher in HM fed relative to MF fed post-weaned piglets. In addition, maltotriose, xylitol followed a similar pattern in the serum of HM fed piglets (Supplemental Table 4) suggesting a shift toward carbohydrate metabolism in HM group post-weaning neonatal diet. Persistent effects on microbial metabolism of tryptophan to indole-3-propionic acid was also observed by a higher abundance of this metabolite in cecal lumen and serum of HM fed piglets (Supplemental Table 4).
The human milk fed to piglets was a pool from donors at 2 to 12 months of lactation, which is prone to variations on the milk composition including fatty acids. The different stages of lactation and the variability from the donor mothers might alter the distal tract metabolite profile. The components added to the HM and MF to maintain the requirement of a growing piglet may impact the luminal metabolome.
Overall, our results showed a distinct metabolome signature between HM and MF-fed during the first 21 days of life. The data presented at PND 21 suggest that human milk feeding may favor the fatty acid metabolism for energy source while MF feeding utilized the sugar breakdown as fuel which is similar with the findings in breastfed vs formula fed infants (11, 13). The greater polyamines and tryptophan pathway metabolites within the distal gut of the HM-fed group may indicate a robust immune response upon human milk than with formula feeding. Also, at PND 21 the higher cholesterol and bile acids in the distal gut of the MF-fed piglets relative to the HM group suggests an impact of formula on cholesterol homeostasis. In contrast, the addition of complementary food (PND 51) resulted in a metabolite profile not as distinguishable and likely shifted to carbohydrate metabolism in HM group. Thus, diet and host-microbiota interactions likely played a role in luminal metabolome (Figure 3). Future studies are needed to determine how host physiology (liver and gut tissue) and immune system are impacted at the molecular level by post-weaning neonatal diet.
Figure 3 Schematic overview shows the divergent metabolite profile derived from human milk (HM) and dairy-based milk-formula (MF) and their potential effects on neonates’ intestinal metabolism (A). Through metabolomics analysis higher fatty acids (myristic, palmitic, linolenic, linoleic, oleic, and palmitoleic acids), spermidine (polyamine), the glutamic amino acid, tryptophan and its derivatives, pyrimidines (thymine, pseudo-uridine, and uracil), and carbohydrates (sugars) were detected in different regions of the distal gastrointestinal tract (gut) [lumen of cecum, proximal colon (PC), distal colon (DC), and rectum] of HM-fed piglets (B). While cholesterol abundance, bile acids (chenodeoxycholic and deoxycholic), essential amino acids (histidine, valine, and leucine), non-essential amino acids (taurine and glycine), and carbohydrates were greater in the luminal distal gut of MF- fed piglets during the first 21 days of life (C). Sugar metabolites and tryptophan derivatives (i.e., indoles) present in the distal gut suggest that neonatal diet interactions with the host-microbiota impact the intestinal metabolism which can be associated with the altered serum metabolites from both diets (D). Diet- microbial interactions reflected in the excretion of mono- and oligosaccharides (i.e., 1,5-anhydroglucitol and raffinose, respectively) in the urine of HM-group compared to sugar alcohols (i.e., threitol) and cholesterol abundance in the urine of MF-group. This model suggests that both HM and MF can impact the host-microbial and the host-intermediate metabolism resulting in a different metabolic profile prior to weaning.
The raw metabolite data are available online as Supplementary Table 6. Further inquiries can be directed to the corresponding author.
The animal study was reviewed and approved by University of Arkansas For Medical Sciences.
LY - conceived the study. FR and LY - conducted data anlyses and interpretation, and wrote the manuscript. KM and AB—conducted the study. KW—statistical analysis of the data, AE—input on data analysis, SA and LB—edited the manuscript. All authors contributed to the article and approved the submitted version.
The project is funded by USDA-ARS Project 6026-51000-012-05S and 6026-51000-012-06S, and LY is also supported by NIH 1R21AI146521.
The authors declare that the article was conducted in the absence of any commercial or financial relationships that could be construed as a potential conflict of interest.
The authors of this paper would like to thank the vivarium personnel Matt Ferguson, Jessica Besancon, Mallory Jayroe, Bobby Fay and Trae Pittman for their assistance with the piglet studies.
The Supplementary Material for this article can be found online at: https://www.frontiersin.org/articles/10.3389/fimmu.2020.607609/full#supplementary-material
Supplementary Figure 1 | Two-dimensional scores plot of partial square discriminant analysis (PLS-DA) model showing the distribution of the luminal contents used as quality control pools in the metabolomic analysis. PLS-DA scores (i.e., individual samples) for components 1 and 2 are displayed. Gray circle shadow represents the 95% confidence region. Red circles indicate the individual luminal content samples.
Supplementary Table 1 | Prior to metabolome data statistical analysis at PND 51, metabolite abundance in cecum, proximal colon, distal colon, and rectum contents were assessed by permutational multivariate ANOVA (PERMANOVA) including Diet (human milk or milk formula), group (immunization vs control), and their interactions (Diet:group).
Supplementary Table 2 | Average abundances (quantifier ion [quantion] intensities) of metabolites significantly altered by diet at postnatal day (PND) 21 (n=8-11/group) across the cecum, proximal colon, distal colon, and rectum contents of piglets fed with human milk (HM) or milk formula (MF) through PND 21.
Supplementary Table 3 | Average abundances (quantifier ion [quantion] intensities) of metabolites significantly altered by diet at postnatal day (PND) 51 (n=9–15/group) across the cecum, proximal colon, distal colon, and rectum contents of piglets fed with human milk (HM) or milk formula (MF) through PND 21.
Supplementary Table 4 | Average abundances (quantifier ion [quantion] intensities) of serum metabolites significantly altered by diet at postnatal day (PND) 21 (n=25/group) and PND 51 (n=15/group) of piglets fed with human milk (HM) or milk formula (MF) through PND 21.
Supplementary Table 5 | Average abundances (quantion peak intensities) of urinary metabolites significantly altered by diet at postnatal day (PND) 21 (n=25/group) and PND 51 (n=15/group), in piglets fed with human milk (HM) or milk formula (MF) through PND 21.
1. Hanson LA, Korotkova M. The role of breastfeeding in prevention of neonatal infection. Semin Neonatol (2002) 7(4):275–81. doi: 10.1053/siny.2002.0124
2. Hanson LA, Korotkova M, Telemo E. Breast-feeding, infant formulas, and the immune system. Ann Allergy Asthma Immunol (2003) 90(6 Suppl 3):59–63. doi: 10.1016/S1081-1206(10)61662-6
3. Hanson LA. Session 1: Feeding and infant development breast-feeding and immune function. Proc Nutr Soc (2007) 66(3):384–96. doi: 10.1017/S0029665107005654
4. Andersson Y, Hammarstrom ML, Lonnerdal B, Graverholt G, Hernell O. Formula feeding skews immune cell composition toward adaptive immunity compared to breastfeeding. J Immunol (2009) 183(7):4322–8. doi: 10.4049/jimmunol.0900829
5. Belderbos ME, Houben ML, van Bleek GM, Schuijff L, van Uden NO, Bloemen-Carlier EM, et al. Breastfeeding modulates neonatal innate immune responses: a prospective birth cohort study. Pediatr Allergy Immunol (2012) 23(1):65–74. doi: 10.1111/j.1399-3038.2011.01230.x
6. Backhed F, Roswall J, Peng Y, Feng Q, Jia H, Kovatcheva-Datchary P, et al. Dynamics and Stabilization of the Human Gut Microbiome during the First Year of Life. Cell Host Microbe (2015) 17(6):852. doi: 10.1016/j.chom.2015.05.012
7. Lonnerdal B, Kvistgaard AS, Peerson JM, Donovan SM, Peng YM. Growth, Nutrition, and Cytokine Response of Breast-fed Infants and Infants Fed Formula With Added Bovine Osteopontin. J Pediatr Gastroenterol Nutr (2016) 62(4):650–7. doi: 10.1097/MPG.0000000000001005
8. Rossen LM, Simon AE, Herrick KA. Types of Infant Formulas Consumed in the United States. Clin Pediatr (Phila) (2016) 55(3):278–85. doi: 10.1177/0009922815591881
9. Bezirtzoglou E, Tsiotsias A, Welling GW. Microbiota profile in feces of breast- and formula-fed newborns by using fluorescence in situ hybridization (FISH). Anaerobe (2011) 17(6):478–82. doi: 10.1016/j.anaerobe.2011.03.009
10. Davis EC, Wang M, Donovan SM. The role of early life nutrition in the establishment of gastrointestinal microbial composition and function. Gut Microbes (2017) 8(2):143–71. doi: 10.1080/19490976.2016.1278104
11. He X, Parenti M, Grip T, Domellof M, Lonnerdal B, Hernell O, et al. Metabolic phenotype of breast-fed infants, and infants fed standard formula or bovine MFGM supplemented formula: a randomized controlled trial. Sci Rep (2019) 9(1):339. doi: 10.1038/s41598-019-48858-y
12. He X, Parenti M, Grip T, Lönnerdal B, Timby N, Domellöf M, et al. Fecal microbiome and metabolome of infants fed bovine MFGM supplemented formula or standard formula with breast-fed infants as reference: a randomized controlled trial. Sci Rep (2019) 9(1):11589–9. doi: 10.1038/s41598-019-47953-4
13. Slupsky CM, He X, Hernell O, Andersson Y, Rudolph C, Lönnerdal B, et al. Postprandial metabolic response of breast-fed infants and infants fed lactose-free vs regular infant formula: A randomized controlled trial. Sci Rep (2017) 7(1):3640–0. doi: 10.1038/s41598-017-03975-4
14. Wang A, Diana A, Rahmannia S, Gibson R, Houghton L, Slupsky C. Differences in the Fecal Metabolome and Microbiome Between Exclusive and Partial Breastfed Infants are More Pronounced at 2 Months Compared to 5 Months. Curr Dev Nutr (2020) 4(Supplement_2):1097–7. doi: 10.1093/cdn/nzaa054_169
15. He X, Sotelo-Orozco J, Rudolph C, Lönnerdal B, Slupsky CM. The Role of Protein and Free Amino Acids on Intake, Metabolism, and Gut Microbiome: A Comparison Between Breast-Fed and Formula-Fed Rhesus Monkey Infants. Front Pediatr (2019) 7:563. doi: 10.3389/fped.2019.00563
16. O’Sullivan A, He X, McNiven EMS, Haggarty NW, Lönnerdal B, Slupsky CM. Early Diet Impacts Infant Rhesus Gut Microbiome, Immunity, and Metabolism. J Proteome Res (2013) 12(6):2833–45. doi: 10.1021/pr4001702
17. Brink LR, Matazel K, Piccolo BD, Bowlin AK, Chintapalli SV, Shankar K, et al. Neonatal Diet Impacts Bioregional Microbiota Composition in Piglets Fed Human Breast Milk or Infant Formula. J Nutr (2019) 149(12):2236–46. doi: 10.1093/jn/nxz170
18. Elolimy AA, Washam C, Byrum S, Chen C, Dawson H, Bowlin AK, et al. Formula Diet Alters the Ileal Metagenome and Transcriptome at Weaning and during the Postweaning Period in a Porcine Model. mSystems (2020) 5(4):e00457–20. doi: 10.1128/mSystems.00457-20
19. Miklavcic JJ, Badger TM, Bowlin AK, Matazel KS, Cleves MA, LeRoith T, et al. Human Breast-Milk Feeding Enhances the Humoral and Cell-Mediated Immune Response in Neonatal Piglets. J Nutr (2018) 148(11):1860–70. doi: 10.1093/jn/nxy170
20. Piccolo BD, Mercer KE, Bhattacharyya S, Bowlin AK, Saraf MK, Pack L, et al. Early Postnatal Diets Affect the Bioregional Small Intestine Microbiome and Ileal Metabolome in Neonatal Pigs. J Nutr (2017) 147(8):1499–509. doi: 10.3945/jn.117.252767
21. Monaco MH, Kim DH, Gurung RB, Donovan SM. Evaluation of 6’-Sialyllactose Sodium Salt Supplementation to Formula on Growth and Clinical Parameters in Neonatal Piglets. Nutrients (2020) 12(4):1030. doi: 10.3390/nu12041030
22. Reznikov EA, Comstock SS, Yi C, Contractor N, Donovan SM. Dietary bovine lactoferrin increases intestinal cell proliferation in neonatal piglets. J Nutr (2014) 144(9):1401–8. doi: 10.3945/jn.114.196568
23. Saraf MK, Piccolo BD, Bowlin AK, Mercer KE, LeRoith T, Chintapalli SV, et al. Formula diet driven microbiota shifts tryptophan metabolism from serotonin to tryptamine in neonatal porcine colon. Microbiome (2017) 5(1):77. doi: 10.1186/s40168-017-0297-z
24. Babawale EA, Jones PJ, Mercer KE, Lin H, Yeruva L, Bar Yoseph F, et al. Modulating Sterol Concentrations in Infant Formula Influences Cholesterol Absorption and Synthesis in the Neonatal Piglet. Nutrients (2018) 10(12):1848. doi: 10.3390/nu10121848
25. Boudry G, Morise A, Seve B, Le Huërou-Luron I. Effect of milk formula protein content on intestinal barrier function in a porcine model of LBW neonates. Pediatr Res (2011) 69(1):4–9. doi: 10.1203/PDR.0b013e3181fc9d13
26. Puiman P, Stoll B. Animal models to study neonatal nutrition in humans. Curr Opin Clin Nutr Metab Care (2008) 11(5):601–6. doi: 10.1097/MCO.0b013e32830b5b15
27. Council NR. Nutrient Requirements of Swine: Eleventh Revised Edition. Washington, DC: The National Academies Press (2012). p. 420.
28. Fiehn O, Wohlgemuth G, Scholz M, Kind T, Lee DY, Lu Y, et al. Quality control for plant metabolomics: reporting MSI-compliant studies. Plant J (2008) 53(4):691–704. doi: 10.1111/j.1365-313X.2007.03387.x
29. Fiehn O. Metabolomics by Gas Chromatography-Mass Spectrometry: Combined Targeted and Untargeted Profiling. Curr Protoc Mol Biol (2016) 114:30.4.1–30.4.32. doi: 10.1002/0471142727.mb3004s114
30. Heller SR, McNaught A, Pletnev I, Stein S, Tchekhovskoi D. InChI, the IUPAC International Chemical Identifier. J Cheminform (2015) 7:23–3. doi: 10.1186/s13321-015-0068-4
31. Bolton EE, Wang Y, Thiessen PA, Bryant SH. Chapter 12 - PubChem: Integrated Platform of Small Molecules and Biological Activities. In: Wheeler RA, Spellmeyer DC, editors. Annual Reports in Computational Chemistry. Elsevier Science (2008). p. 217–41. doi: 10.1016/S1574-1400(08)00012-1
32. Chong J, Soufan O, Li C, Caraus I, Li S, Bourque G, et al. MetaboAnalyst 4.0: towards more transparent and integrative metabolomics analysis. Nucleic Acids Res (2018) 46(W1):W486–94. doi: 10.1093/nar/gky310
33. Xia J, Wishart DS. Web-based inference of biological patterns, functions and pathways from metabolomic data using MetaboAnalyst. Nat Protoc (2011) 6(6):743–60. doi: 10.1038/nprot.2011.319
34. Elolimy A, Alharthi A, Zeineldin M, Parys C, Helmbrecht A, Loor JJ. Supply of Methionine During Late-Pregnancy Alters Fecal Microbiota and Metabolome in Neonatal Dairy Calves Without Changes in Daily Feed Intake. Front Microbiol (2019) 10:2159. doi: 10.3389/fmicb.2019.02159
35. Jain A, Li XH, Chen WN. An untargeted fecal and urine metabolomics analysis of the interplay between the gut microbiome, diet and human metabolism in Indian and Chinese adults. Sci Rep (2019) 9(1):9191. doi: 10.1038/s41598-019-45640-y
36. Evenepoel P, Claus D, Geypens B, Hiele M, Geboes K, Rutgeerts P, et al. Amount and fate of egg protein escaping assimilation in the small intestine of humans. Am J Physiol (1999) 277(5):G935–43. doi: 10.1152/ajpgi.1999.277.5.G935
37. Gibson JA, Sladen GE, Dawson AM. Protein absorption and ammonia production: the effects of dietary protein and removal of the colon. Br J Nutr (1976) 35(1):61–5. doi: 10.1079/BJN19760009
38. Macfarlane GT, Allison C, Gibson SA, Cummings JH. Contribution of the microflora to proteolysis in the human large intestine. J Appl Bacteriol (1988) 64(1):37–46. doi: 10.1111/j.1365-2672.1988.tb02427.x
39. Davila AM, Blachier F, Gotteland M, Andriamihaja M, Benetti PH, Sanz Y, et al. Re-print of “Intestinal luminal nitrogen metabolism: role of the gut microbiota and consequences for the host”. Pharmacol Res (2013) 69(1):114–26. doi: 10.1016/j.phrs.2013.01.003
40. Neis EPJG, Dejong CHC, Rensen SS. The role of microbial amino acid metabolism in host metabolism. Nutrients (2015) 7(4):2930–46. doi: 10.3390/nu7042930
41. Brink LR, Mercer KE, Piccolo BD, Chintapalli SV, Elolimy A, Bowlin AK, et al. Neonatal diet alters fecal microbiota and metabolome profiles at different ages in infants fed breast milk or formula. Am J Clin Nutr (2020) 111(6):1190–202. doi: 10.1093/ajcn/nqaa076
42. Venkatesh M, Mukherjee S, Wang H, Li H, Sun K, Benechet AP, et al. Symbiotic bacterial metabolites regulate gastrointestinal barrier function via the xenobiotic sensor PXR and Toll-like receptor 4. Immunity (2014) 41(2):296–310. doi: 10.1016/j.immuni.2014.06.014
43. Zelante T, Iannitti RG, Cunha C, De Luca A, Giovannini G, Pieraccini G, et al. Tryptophan catabolites from microbiota engage aryl hydrocarbon receptor and balance mucosal reactivity via interleukin-22. Immunity (2013) 39(2):372–85. doi: 10.1016/j.immuni.2013.08.003
44. Koletzko B, Rodriguez-Palmero M, Demmelmair H, Fidler N, Jensen R, Sauerwald T. Physiological aspects of human milk lipids. Early Hum Dev (2001) 65 Suppl:S3–S18. doi: 10.1016/S0378-3782(01)00204-3
45. Mendonça MA, Araújo WMC, Borgo LA, Alencar E. Lipid profile of different infant formulas for infants. PLoS One (2017) 12(6):e0177812. doi: 10.1371/journal.pone.0177812
46. Abedi E, Sahari MA. Long-chain polyunsaturated fatty acid sources and evaluation of their nutritional and functional properties. Food Sci Nutr (2014) 2(5):443–63. doi: 10.1002/fsn3.121
47. Wood JD, Enser M, Fisher AV, Nute GR, Richardson RI, Sheard PR. Manipulating meat quality and composition. Proc Nutr Soc (1999) 58(2):363–70. doi: 10.1017/S0029665199000488
48. Rangel-Huerta OD, Aguilera CM, Mesa MD, Gil A. Omega-3 long-chain polyunsaturated fatty acids supplementation on inflammatory biomakers: a systematic review of randomised clinical trials. Br J Nutr (2012) 107 Suppl 2:S159–70. doi: 10.1017/S0007114512001559
49. Whiting CV, Bland PW, Tarlton JF. Dietary N-3 Polyunsaturated Fatty Acids Reduce Disease and Colonic Proinflammatory Cytokines in a Mouse Model of Colitis. Inflamm Bowel Dis (2005) 11(4):340–9. doi: 10.1097/01.MIB.0000164016.98913.7c
50. Hassan A, Ibrahim A, Mbodji K, Coëffier M, Ziegler F, Bounoure F, et al. An α-Linolenic Acid-Rich Formula Reduces Oxidative Stress and Inflammation by Regulating NF-κB in Rats with TNBS-Induced Colitis. J Nutr (2010) 140(10):1714–21. doi: 10.3945/jn.109.119768
51. Marion-Letellier R, Butler M, Dechelotte P, Playford RJ, Ghosh S. Comparison of cytokine modulation by natural peroxisome proliferator-activated receptor gamma ligands with synthetic ligands in intestinal-like Caco-2 cells and human dendritic cells–potential for dietary modulation of peroxisome proliferator-activated receptor gamma in intestinal inflammation. Am J Clin Nutr (2008) 87(4):939–48. doi: 10.1093/ajcn/87.4.939
52. Lauridsen C. Effects of dietary fatty acids on gut health and function of pigs pre- and post-weaning. J Anim Sci (2020) 98(4):skaa086. doi: 10.1093/jas/skaa086
53. McAfee JM, Kattesh HG, Lindemann MD, Voy BH, Kojima CJ, Burdick Sanchez NC, et al. Effect of omega-3 polyunsaturated fatty acid (n-3 PUFA) supplementation to lactating sows on growth and indicators of stress in the postweaned pig1,2. J Anim Sci (2019) 97(11):4453–63. doi: 10.1093/jas/skz300
54. Plaza-Zamora J, Sabater-Molina M, Rodriguez-Palmero M, Rivero M, Bosch V, Nadal JM, et al. Polyamines in human breast milk for preterm and term infants. Br J Nutr (2013) 110(3):524–8. doi: 10.1017/S0007114512005284
55. Loser C, Eisel A, Harms D, Folsch UR. Dietary polyamines are essential luminal growth factors for small intestinal and colonic mucosal growth and development. Gut (1999) 44(1):12–6. doi: 10.1136/gut.44.1.12
56. Wang JY. Polyamines regulate expression of E-cadherin and play an important role in control of intestinal epithelial barrier function. Inflammopharmacology (2005) 13(1-3):91–101. doi: 10.1163/156856005774423890
57. Dandrifosse G, Peulen O, El Khefif N, Deloyer P, Dandrifosse AC, Grandfils C. Are milk polyamines preventive agents against food allergy? Proc Nutr Soc (2000) 59(1):81–6. doi: 10.1017/S0029665100000100
58. Atiya Ali M, Strandvik B, Sabel KG, Palme Kilander C, Strömberg R, Yngve A. Polyamine levels in breast milk are associated with mothers’ dietary intake and are higher in preterm than full-term human milk and formulas. J Hum Nutr Diet (2014) 27(5):459–67. doi: 10.1111/jhn.12156
59. Li G, Ding H, Yu X, Meng Y, Li J, Guo Q, et al. Spermidine Suppresses Inflammatory DC Function by Activating the FOXO3 Pathway and Counteracts Autoimmunity. iScience (2020) 23(1):100807. doi: 10.1016/j.isci.2019.100807
60. Ossa JC, Yáñez D, Valenzuela R, Gallardo P, Lucero Y, Farfán MJ. Intestinal Inflammation in Chilean Infants Fed With Bovine Formula vs. Breast Milk and Its Association With Their Gut Microbiota. Front Cell Infect Microbiol (2018) 8:190. doi: 10.3389/fcimb.2018.00190
61. Zhang H, Alsaleh G, Feltham J, Sun Y, Napolitano G, Riffelmacher T, et al. Polyamines Control eIF5A Hypusination, TFEB Translation, and Autophagy to Reverse B Cell Senescence. Mol Cell (2019) 76(1):110–25.e9. doi: 10.1016/j.molcel.2019.08.005
62. Beaudry M, Dufour R, Marcoux S. Relation between infant feeding and infections during the first six months of life. J Pediatr (1995) 126(2):191–7. doi: 10.1016/S0022-3476(95)70544-9
63. Beaudry M, Dufour R, Marcoux S. [Breast feeding and protection against infection in industrialized countries]. Arch Pediatr (1996) 3 Suppl 1:126s–7s. doi: 10.1016/0929-693X(96)86014-3
64. Hahn-Zoric M, Fulconis F, Minoli I, Moro G, Carlsson B, Böttiger M, et al. Antibody responses to parenteral and oral vaccines are impaired by conventional and low protein formulas as compared to breast-feeding. Acta Paediatr Scand (1990) 79(12):1137–42. doi: 10.1111/j.1651-2227.1990.tb11401.x
65. Gómez-Gallego C, Collado MC, Ilo T, Jaakkola UM, Bernal MJ, Periago MJ, et al. Infant formula supplemented with polyamines alters the intestinal microbiota in neonatal BALB/cOlaHsd mice. J Nutr Biochem (2012) 23(11):1508–13. doi: 10.1016/j.jnutbio.2011.10.003
66. Delplanque B, Gibson R, Koletzko B, Lapillonne A, Strandvik B. Lipid Quality in Infant Nutrition: Current Knowledge and Future Opportunities. J Pediatr Gastroenterol Nutr (2015) 61(1):8–17. doi: 10.1097/MPG.0000000000000818
67. Prosser CG, Svetashev VI, Vyssotski MV, Lowry DJ. Composition and distribution of fatty acids in triglycerides from goat infant formulas with milk fat. J Dairy Sci (2010) 93(7):2857–62. doi: 10.3168/jds.2009-2946
68. Kruit J-K, Groen AK, van Berkel TJ, Kuipers F. Emerging roles of the intestine in control of cholesterol metabolism. World J Gastroenterol (2006) 12(40):6429–39. doi: 10.3748/wjg.v12.i40.6429
69. Santosa S, Varady KA, AbuMweis S, Jones PJ. Physiological and therapeutic factors affecting cholesterol metabolism: does a reciprocal relationship between cholesterol absorption and synthesis really exist? Life Sci (2007) 80(6):505–14. doi: 10.1016/j.lfs.2006.10.006
70. Bayley TM, Alasmi M, Thorkelson T, Krug-Wispe S, Jones PJ, Bulani JL, et al. Influence of formula versus breast milk on cholesterol synthesis rates in four-month-old infants. Pediatr Res (1998) 44(1):60–7. doi: 10.1203/00006450-199807000-00010
71. Wong WW, Hachey DL, Insull W, Opekun AR, Klein PD. Effect of dietary cholesterol on cholesterol synthesis in breast-fed and formula-fed infants. J Lipid Res (1993) 34(8):1403–11.
72. Jones PJ, Hrboticky N, Hahn P, Innis SM. Comparison of breast-feeding and formula feeding on intestinal and hepatic cholesterol metabolism in neonatal pigs. Am J Clin Nutr (1990) 51(6):979–84. doi: 10.1093/ajcn/51.6.979
73. Mercer KE, Bhattacharyya S, Diaz-Rubio ME, Piccolo BD, Pack LM, Sharma N, et al. Infant Formula Feeding Increases Hepatic Cholesterol 7alpha Hydroxylase (CYP7A1) Expression and Fecal Bile Acid Loss in Neonatal Piglets. J Nutr (2018) 148(5):702–11. doi: 10.1093/jn/nxy038
74. Chiang JYL, Ferrell JM. Bile Acid Biology, Pathophysiology, and Therapeutics. Clin Liver Dis (Hoboken) (2020) 15(3):91–4. doi: 10.1002/cld.861
75. Foley MH, O’Flaherty S, Barrangou R, Theriot CM. Bile salt hydrolases: Gatekeepers of bile acid metabolism and host-microbiome crosstalk in the gastrointestinal tract. PLoS Pathog (2019) 15(3):e1007581. doi: 10.1371/journal.ppat.1007581
76. Ridlon JM, Kang DJ, Hylemon PB. Bile salt biotransformations by human intestinal bacteria. J Lipid Res (2006) 47(2):241–59. doi: 10.1194/jlr.R500013-JLR200
77. Urdaneta V, Casadesus J. Interactions between Bacteria and Bile Salts in the Gastrointestinal and Hepatobiliary Tracts. Front Med (Lausanne) (2017) 4:163. doi: 10.3389/fmed.2017.00163
78. Xu M, Cen M, Shen Y, Zhu Y, Cheng F, Tang L, et al. Deoxycholic Acid-Induced Gut Dysbiosis Disrupts Bile Acid Enterohepatic Circulation and Promotes Intestinal Inflammation. Dig Dis Sci (2020) 10:1007. doi: 10.1007/s10620-020-06208-3
79. Blacher E, Levy M, Tatirovsky E, Elinav E. Microbiome-Modulated Metabolites at the Interface of Host Immunity. J Immunol (2017) 198(2):572–80. doi: 10.4049/jimmunol.1601247
80. Chuang CK, Lin SP, Lee HC, Wang TJ, Shih YS, Huang FY, et al. Free amino acids in full-term and pre-term human milk and infant formula. J Pediatr Gastroenterol Nutr (2005) 40(4):496–500. doi: 10.1097/01.MPG.0000150407.30058.47
81. Zhang Z, Adelman AS, Rai D, Boettcher J, Lőnnerdal B. Amino acid profiles in term and preterm human milk through lactation: a systematic review. Nutrients (2013) 5(12):4800–21. doi: 10.3390/nu5124800
82. Koletzko B. Glutamate Supply and Metabolism in Infants. Ann Nutr Metab (2018) 73 Suppl 5:29–35. doi: 10.1159/000494780
83. Agostoni C, Carratu B, Boniglia C, Riva E, Sanzini E. Free amino acid content in standard infant formulas: comparison with human milk. J Am Coll Nutr (2000) 19(4):434–8. doi: 10.1080/07315724.2000.10718943
84. Agostoni C, Carratù B, Boniglia C, Lammardo AM, Riva E, Sanzini E. Free glutamine and glutamic acid increase in human milk through a three-month lactation period. J Pediatr Gastroenterol Nutr (2000) 31(5):508–12. doi: 10.1097/00005176-200011000-00011
85. Windmueller HG. Glutamine utilization by the small intestine. Adv Enzymol Relat Areas Mol Biol (1982) 53:201–37. doi: 10.1002/9780470122983.ch6
86. Hernell O, Lönnerdal B. Nutritional evaluation of protein hydrolysate formulas in healthy term infants: plasma amino acids, hematology, and trace elements. Am J Clin Nutr (2003) 78(2):296–301. doi: 10.1093/ajcn/78.2.296
87. Kirchberg FF, Harder U, Weber M, Grote V, Demmelmair H, Peissner W, et al. Dietary protein intake affects amino acid and acylcarnitine metabolism in infants aged 6 months. J Clin Endocrinol Metab (2015) 100(1):149–58. doi: 10.1210/jc.2014-3157
88. Socha P, Grote V, Gruszfeld D, Janas R, Demmelmair H, Closa-Monasterolo R, et al. Milk protein intake, the metabolic-endocrine response, and growth in infancy: data from a randomized clinical trial. Am J Clin Nutr (2011) 94(6 Suppl):1776S–84S. doi: 10.3945/ajcn.110.000596
89. Huisman M, van Beusekom CM, Lanting CI, Nijeboer HJ, Muskiet FA, Boersma ER. Triglycerides, fatty acids, sterols, mono- and disaccharides and sugar alcohols in human milk and current types of infant formula milk. Eur J Clin Nutr (1996) 50(4):255–60.
Keywords: human milk, infant formula, neonates, metabolism, host-microbiota
Citation: Rosa F, Matazel KS, Bowlin AK, Williams KD, Elolimy AA, Adams SH, Bode L and Yeruva L (2020) Neonatal Diet Impacts the Large Intestine Luminal Metabolome at Weaning and Post-Weaning in Piglets Fed Formula or Human Milk. Front. Immunol. 11:607609. doi: 10.3389/fimmu.2020.607609
Received: 17 September 2020; Accepted: 05 November 2020;
Published: 07 December 2020.
Edited by:
Xin Zhao, McGill University, CanadaReviewed by:
Wayne Young, AgResearch Ltd, New ZealandCopyright © 2020 Rosa, Matazel, Bowlin, Williams, Elolimy, Adams, Bode and Yeruva. This is an open-access article distributed under the terms of the Creative Commons Attribution License (CC BY). The use, distribution or reproduction in other forums is permitted, provided the original author(s) and the copyright owner(s) are credited and that the original publication in this journal is cited, in accordance with accepted academic practice. No use, distribution or reproduction is permitted which does not comply with these terms.
*Correspondence: Laxmi Yeruva, dmx5ZXJ1dmFAdWFtcy5lZHU=
Disclaimer: All claims expressed in this article are solely those of the authors and do not necessarily represent those of their affiliated organizations, or those of the publisher, the editors and the reviewers. Any product that may be evaluated in this article or claim that may be made by its manufacturer is not guaranteed or endorsed by the publisher.
Research integrity at Frontiers
Learn more about the work of our research integrity team to safeguard the quality of each article we publish.