- 1Arthritis and Clinical Immunology Program, Oklahoma Medical Research Foundation, Oklahoma City, OK, United States
- 2Department of Microbiology & Immunology, The University of Iowa, Iowa City, IA, United States
- 3Department of Internal Medicine, The University of Iowa, Iowa City, IA, United States
- 4Holden Comprehensive Cancer Center, The University of Iowa, Iowa City, IA, United States
- 5Iowa City VA Medical Center, Iowa City, IA, United States
- 6Department of Medicine and Pathology, Oklahoma University Health Sciences Center, Oklahoma City, OK, United States
Systemic lupus erythematosus (SLE) and other autoimmune diseases are propelled by immune dysregulation and pathogenic, disease-specific autoantibodies. Autoimmunity against the lupus autoantigen Sm is associated with cross-reactivity to Epstein-Barr virus (EBV) nuclear antigen 1 (EBNA-1). Additionally, EBV latent membrane protein-1 (LMP1), initially noted for its oncogenic activity, is an aberrantly active functional mimic of the B cell co-stimulatory molecule CD40. Mice expressing a transgene (Tg) for the mCD40-LMP1 hybrid molecule (containing the cytoplasmic tail of LMP1) have mild autoantibody production and other features of immune dysregulation by 2–3 months of age, but no overt autoimmune disease. This study evaluates whether exposure to the EBV molecular mimic, EBNA-1, stimulates antigen-specific and concurrently-reactive humoral and cellular immunity, as well as lupus-like features. After immunization with EBNA-1, mCD40-LMP1 Tg mice exhibited enhanced, antigen-specific, cellular and humoral responses compared to immunized WT congenic mice. EBNA-1 specific proliferative and inflammatory cytokine responses, including IL-17 and IFN-γ, were significantly increased (p<0.0001) in mCD40-LMP1 Tg mice, as well as antibody responses to amino- and carboxy-domains of EBNA-1. Of particular interest was the ability of mCD40-LMP1 to drive EBNA-1 associated molecular mimicry with the lupus-associated autoantigen, Sm. EBNA-1 immunized mCD40-LMP1 Tg mice exhibited enhanced proliferative and cytokine cellular responses (p<0.0001) to the EBNA-1 homologous epitope PPPGRRP and the Sm B/B’ cross-reactive sequence PPPGMRPP. When immunized with the SLE autoantigen Sm, mCD40-LMP1 Tg mice again exhibited enhanced cellular and humoral immune responses to both Sm and EBNA-1. Cellular immune dysregulation with EBNA-1 immunization in mCD40-LMP1 Tg mice was accompanied by enhanced splenomegaly, increased serum blood urea nitrogen (BUN) and creatinine levels, and elevated anti-dsDNA and antinuclear antibody (ANA) levels (p<0.0001 compared to mCD40 WT mice). However, no evidence of immune-complex glomerulonephritis pathology was noted, suggesting that a combination of EBV and genetic factors may be required to drive lupus-associated renal disease. These data support that the expression of LMP1 in the context of EBNA-1 may interact to increase immune dysregulation that leads to pathogenic, autoantigen-specific lupus inflammation.
Introduction
Systemic lupus erythematosus (SLE) is a chronic autoimmune disease driven by dysregulated cellular and humoral immunity (1–4). Increased immune dysregulation is associated with increased clinical disease activity and flare (5–7) and places patients at risk of permanent end-organ damage, SLE-associated morbidity, and early mortality (8). Such immune dysregulation begins years before clinical disease onset and amplifies through a feed-forward aggregation of altered innate and adaptive immune pathways as patients progress to SLE classification. Concurrent with or following these changes in innate and adaptive immune pathways, pathogenic SLE- associated autoantibody specificities accumulate (9). These autoreactive responses commonly target nuclear antigens such as Ro/SSA, La/SSB, Sm, RNP, and dsDNA (3, 4), the latter two specificities associated with lupus nephritis (10). Despite improved disease management and treatment approaches to suppress and circumvent dysregulated immunity, patients with SLE exhibit persistent and waxing/waning dysregulation of innate and adaptive immune pathways.
Numerous studies over the past two decades have elucidated genetic and genomic contributions to SLE risk and heritability. Despite a twin concordance rate of up to 25% (11) and identification of over 100 lupus associated genetic variants (12, 13), genetics alone explain no more than 50% of SLE risk (14, 15). This supports roles for environmental factors as contributors to SLE etiology (16, 17). Infections, such as Epstein-Barr virus (EBV), are associated with both pediatric (18, 19) and adult (17, 20, 21) SLE. EBV, a member of the herpes virus family, is tropic for B-lymphocytes and promotes cellular dysregulation, including lymphoproliferation (22–24), malignancy (25–27), and autoimmunity (28–30). Compared to unaffected individuals, SLE patients have higher EBV viral loads (31, 32), are more likely to exhibit infection in peripheral blood mononuclear cells (PBMCs) (32, 33) and exhibit aberrant expression of EBV latent genes, including EBV nuclear antigen-1 (EBNA-1) and latent membrane protein-1 (LMP1). These differences in SLE patients may be attributed to immune dysregulation that drives latent protein expression as well as an inability to control viral reactivation (17, 19, 24, 33–37). EBV reactivation is increased in SLE patients, evidenced by increased antibodies to EBNA-1 in conjunction with IgG antibodies against EBV early antigen (EA) and viral capsid antigen (VCA) (17, 38). This viral reactivation is associated with transition to classified SLE (17) as well as clinical disease activity and flare (18, 31).
Both pediatric and adult SLE patients exhibit altered humoral immunity to EBNA-1 (9, 19, 39, 40). EBNA-1 is a structural, molecular mimic with known SLE autoantigens. By eliciting antibodies that structurally cross-react with autoantigens, we and others have shown that EBNA-1 contributes to autoimmunity against Ro/SSA and spliceosomal proteins Sm B, Sm D1, and RNP A (9, 19, 40–42); additional studies by the Spatz laboratory have further found cross-reactivity between EBNA-1 and the SLE-associated autoantigen dsDNA (42–44). Although structural molecular mimicry may be due to random chance, EBNA-1 utilizes and binds to the same nuclear spliceosomal machinery as host cells (45), including Sm and RNP proteins (46), to maintain lytic and latent EBV infection (47). Therefore, functional and structural overlap may drive molecular mimicry between EBNA-1 and SLE-associated autoantigens. Over time with continued cross-reactivity, broken immune tolerance creates a positive feedback loop where autoantibodies mediate cellular damage that releases additional autoantigens, leading to continued immune reactivity, epitope spreading (35, 48), and the accumulation of autoantibody specificities (3, 4, 49) that themselves cross react with EBNA-1 (35). Anti-EBNA-1 antibodies alone may not be enough to break tolerance and drive autoimmunity, as over 90% of individuals have been exposed to EBV and most never develop autoimmunity (50, 51).
Cellular immune dysregulation may facilitate the initial break in tolerance in SLE, as SLE-associated autoantibody specificities associated with EBNA-1 molecular mimicry are detected after evidence of cellular immune dysregulation in pre-clinical SLE (3, 4). Interestingly, EBV encodes proteins that disrupt cellular immune regulation, including LMP1, a functional mimic of CD40. As a costimulatory molecule expressed on antigen-presenting cells, such as B-lymphocytes, dendritic cells, and macrophages, CD40 is vital for B-lymphocyte activation and function and bridges innate and adaptive immunity. Interacting with CD154 on T-lymphocytes (52), CD40 itself triggers B-lymphocyte activation, proliferation, cytokine secretion, and antibody production (52), acts as a co-stimulatory molecule for the B cell receptor (BCR) (53, 54), and amplifies innate signals driven by toll like receptors (TLRs) (55), including TLR7 (56, 57), implicated in SLE pathogenesis (58–60). EBV-encoded LMP1 has been studied in vitro (61–66) and in vivo (62, 67–71) and is a functional mimic of CD40, although it does so in an enhanced and dysregulated manner (Figure 1). Unlike CD40, which requires interaction and trimerization with CD154, the six transmembrane domains of LMP1 are able to self-aggregate in a ligand-independent and uncontrolled manner to drive downstream proximal signaling and subsequent distal functional activities that overlap with CD40, including B-lymphocyte activation, germinal center formation, as well as antibody and cytokine production (71). This ability of LMP1 to spontaneously self-aggregate, without the need for CD154 expressed on T-lymphocytes, may allow for its ability to evade the immune system and contribute to the natural selection of EBV to latently persist within B-lymphocytes (72, 73).
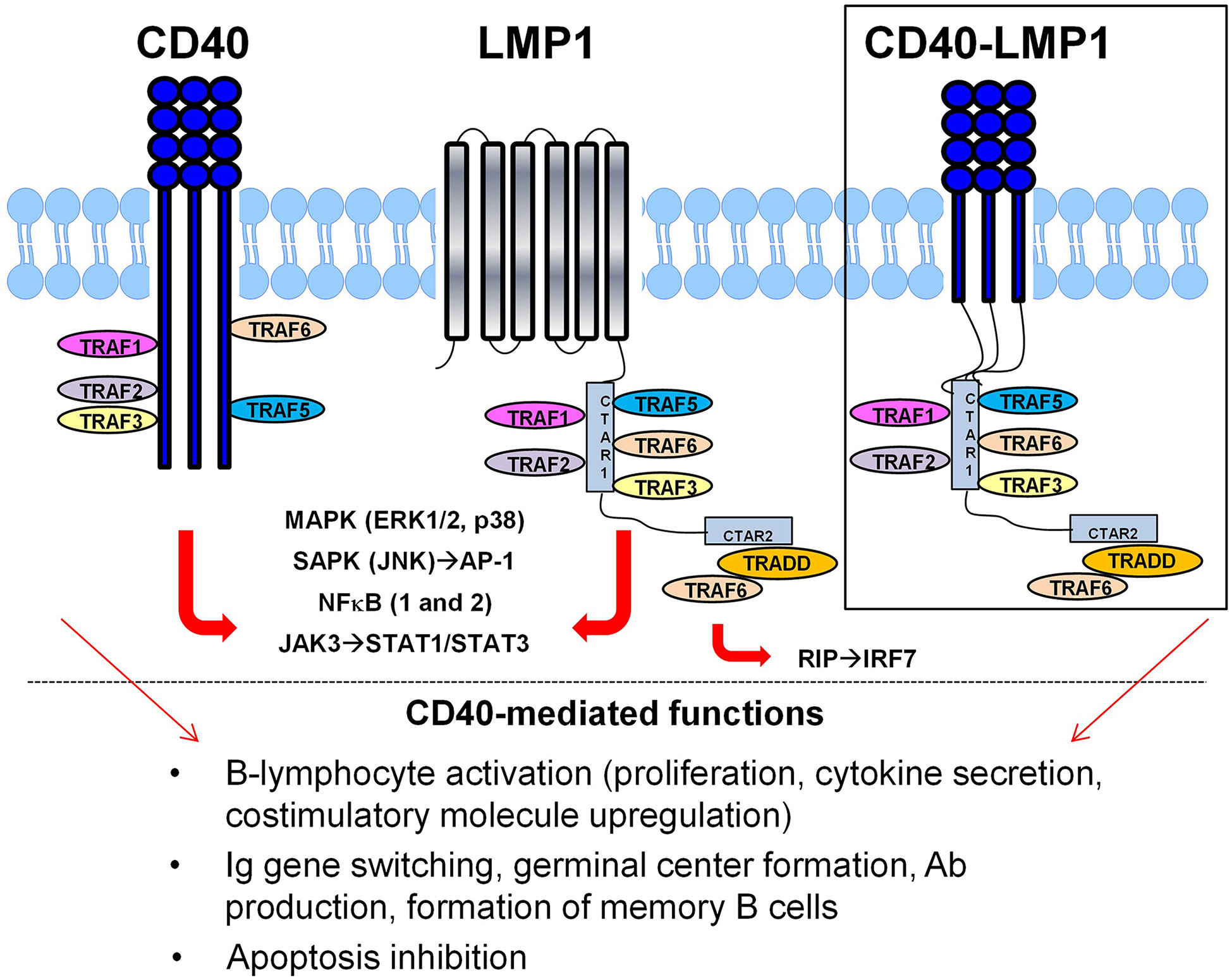
Figure 1 Latent Membrane Protein 1 (LMP1) acts as a viral mimic of the costimulatory molecule CD40. Similar to CD40, LMP1 binds TRAFs through its cytoplasmic domain to mediate proximal signaling/transcriptional regulation and downstream function, including B-lymphocyte activation, antibody production and isotype switching, and apoptosis inhibition. However, LMP1 does this in a dysregulated manner, partially through its ligand-independent, self-aggregating six-transmembrane domains. Replacing the extracellular/transmembrane domain of LMP1 with CD40 demonstrates that the cytoplasmic tail of LMP1 is necessary and sufficient for its enhanced and dysregulated functional mimicry of CD40 (inset).
Like CD40, the cytoplasmic domain of LMP1 does not have enzymatic activity, but instead utilizes TNF-receptor associated factors (TRAFs) to facilitate its signaling and biologic activities. Replacing the LMP1 extracellular/transmembrane domains with those of CD40 (Figure 1, inset) demonstrated that the cytoplasmic tail of LMP1 is necessary and sufficient to mimic CD40 activity and do so in a dysregulated manner (71, 74). LMP1 interacts with TRAFs via two carboxy-terminus activating regions (CTAR), CTAR1 and CTAR2 (66, 68). CTAR1, similar to CD40, contains the TRAF binding motif, PXQXT, to bind TRAF1, TRAF2, TRAF3, TRAF5, and TRAF6 (63, 68). Yet there are key differences in the way LMP1 utilizes TRAFs compared to CD40. CD40 drives B-lymphocyte activation primarily through TRAFs 2 and 6 (75, 76), as well as TRAF1 (77), with TRAF3 acting as an inhibitor (65, 78). In contrast, LMP1 utilizes TRAF3 (63–65) in an activating manner alongside TRAF5 (62), as well as TRAFs 1 and 2 (79, 80). Furthermore, CD40-mediated signaling results in ubiquitination and degradation of TRAFs 2 and 3 to downregulate its signal; this does not occur in LMP1 signaling (63, 74). In addition, LMP1 indirectly binds TRAF6 via TRADD in its CTAR2 domain (81, 82), allowing for additional CD40 signals via IRAK1 (83), as well as IRF7 activation via RIP (84).
These dysregulated, pro-activation differences in utilization of TRAFs by LMP1 have been shown to translate into an autoimmune disease phenotype in vivo. The mCD40-LMP1 transgenic (Tg) mouse model expresses a hybrid molecule with the mouse (m)CD40 extracellular domains and the LMP1 cytoplasmic tail, as described above. The transgene is driven by an MHCII promoter on a C57BL/6 (B6), CD40-deficient background, so that the only CD40 present is mCD40-LMP1. Compared to congenic mCD40 Tg and B6 mice that express full-length, wild-type mCD40 (mCD40 WT mice), mCD40-LMP1 Tg mice exhibit both splenomegaly and lymphadenopathy, with expanded immature/activated B-lymphocyte populations and ectopic germinal center formation. In addition, these mice produce autoantibodies, including anti-dsDNA, and exhibit aberrant cytokine levels, including IL-6 (62, 67, 68, 71). Yet, the mCD40-LMP1 Tg mice are capable of driving T-dependent antibody responses, with normal isotype switching, affinity maturation, and germinal center formation (71).
We have previously demonstrated that in the context of type II collagen, an autoantigen that induces inflammatory arthritis in a murine model of rheumatoid arthritis (85), mCD40-LMP1 Tg mice exhibit accelerated and exacerbated inflammatory arthritis compared to their congenic WT counterparts (70). Ex vivo, these mice exhibit enhanced innate and adaptive cellular immunity in antigenic recall responses, particularly TNF-α, IL-6, and IL-17A, as well as enhanced TNF-α and IL-6 secretion in activated B-lymphocytes. This enhanced cellular immunity is accompanied by an increase in total and collagen-specific antibody production (70), with immune pathway specific isotype switching, suggesting that LMP1 is able to drive enhanced and dysregulated cellular and humoral adaptive immunity.
Because mCD40-LMP1 drives an autoimmunity phenotype that leads to overt pathology in the context of the autoantigen collagen (70), we hypothesized that LMP1 may enhance the onset of autoimmunity in conjunction with molecular mimicry between EBNA-1 and the SLE-associated autoantigen, Sm (86). Therefore, the current study investigates antigen-specific cellular and humoral immune responses to EBNA-1 and its cross-reactive lupus autoantigen, Sm, in the context of mCD40-LMP1-mediated adaptive immunity. Based on our previous epitope mapping studies, this includes reactivity in EBNA-1 and Sm immunized mice to the antigenic epitope, PPPGRRP (EBNA-1) and its homologous, comparable antigenic epitope sequence, PPPGMRPP (Sm) (19, 51, 86, 87). Further, we evaluated these mice for enhanced splenomegaly, the presence of ANA and anti-dsDNA autoantibodies, and altered renal function.
Materials and Methods
Mice
C57BL/6 mice were purchased at 5–8 weeks of age from the National Cancer Institute (Bethesda, MD). Mice transgenic for the molecule mCD40-LMP1 (mCD40-LMP1 Tg) or full length mCD40 (mCD40 Tg), driven by the MHC Class II Eα promoter were transferred from the Bishop Lab (The University of Iowa) to the Oklahoma Medical Research Foundation (OMRF). In addition to B-lymphocytes, EBV can also infect myeloid cells (88, 89), so it is reasonable to express LMP1 on these cell types and B-lymphocytes. Tg mice were maintained on the C57BL/6 CD40-deficient background (B6.129P2-CD40tm1Kik/J from The Jackson Laboratory, Sacramento, CA) at OMRF, as previously described (71). Mice were age- and sex-matched and analyzed at 3–4 months of age. All mice were housed in specific pathogen-free barrier facilities with restricted access, all animal care and housing requirements of the National Institutes of Health Committee on Care and Use of Laboratory Animals were followed, and all procedures were approved by the OMRF Animal Care and Use Committee.
Immunizations
Mice were immunized based on protocols described (86, 90) (Figure 2). Briefly, all mouse strains either remained naïve (n=6 mice/strain) or were immunized with sterile saline (adjuvant control; n= 6 mice/strain), 100 µg EBNA-1 mosaic (n = 8 mice/strain; EBNA-1 antigen with truncated glycine-alanine repeat; BiosPacific, Inc./Bio-techne, Emeryville, CA), or 100 µg Sm antigen (n= 6 mice/strain; Immunovision; Springdale, AR). Saline and immunogens were emulsified 1:1 in either Complete Freund’s Adjuvant (CFA; Sigma-Aldrich/MilliporeSigma, St. Louis, MO) for initial immunization (Day 0), or Incomplete Freund’s Adjuvant (IFA; Sigma-Aldrich/MilliporeSigma) for booster immunizations (Day 10, Day 28). Emulsified adjuvant control (saline) and immunogens were injected in equal portions intraperitoneally (50 µg/100µl) and subcutaneously in alternating flanks (50µg/100µl). Blood samples for sera were collected on Days -3, 10, 28, and 56 relative to initial immunization via tail vein sampling. A subset of EBNA-1 immunized mice completed 10 or 28 days (n = 6 mice/strain) of the protocol (mice groups were staggered so that all of the mice completed the experimental protocol on the same day).
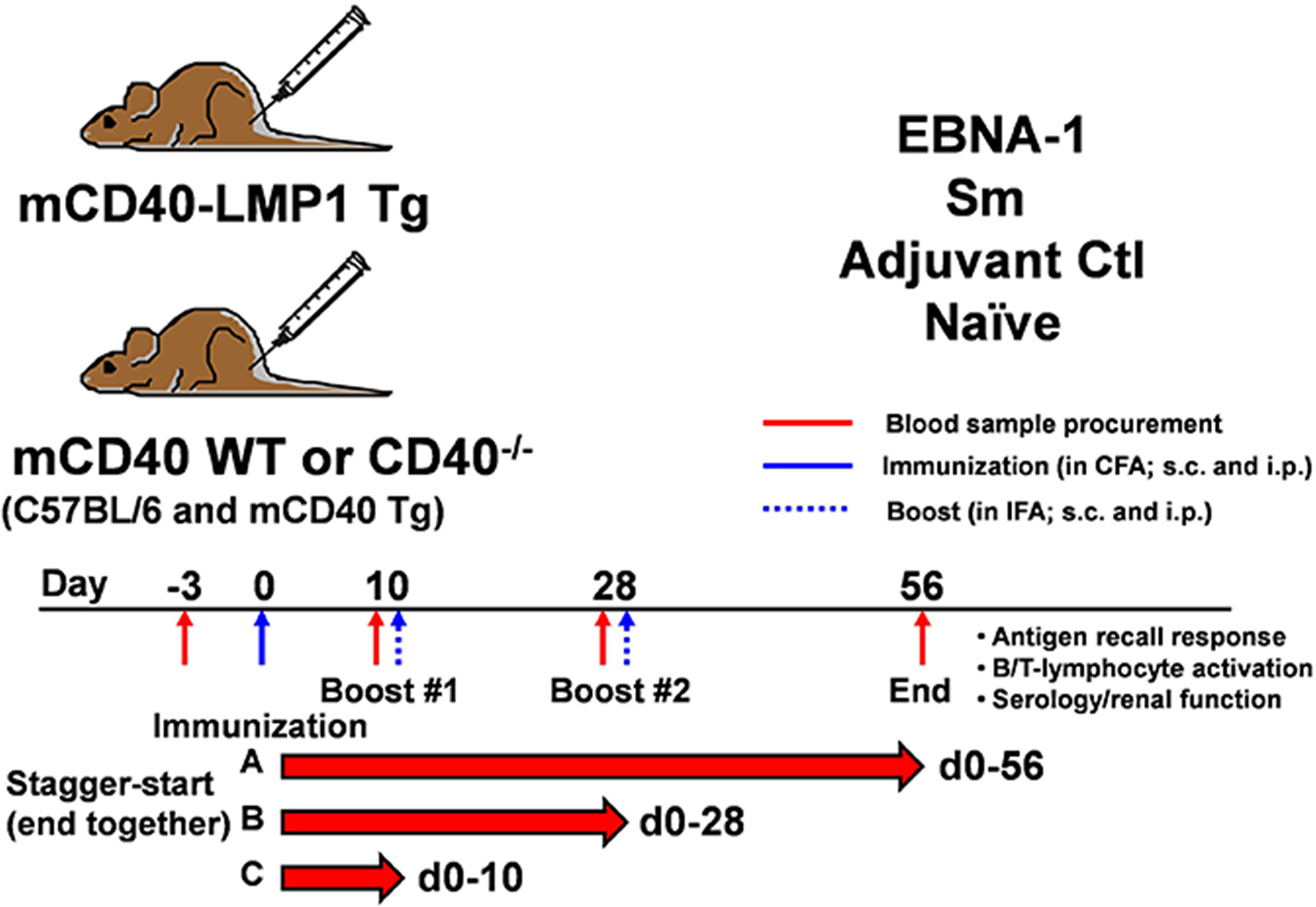
Figure 2 Immunization of mCD40-LMP1 Tg mice with EBNA-1, Sm, or controls to assess antigen-specific immune response and antigen cross-reactivity. As described in Materials and Methods, mCD40-LMP1 Tg, mCD40 WT (C57BL/6 and mCD40 Tg), and mCD40-/- mice remained naïve or were immunized with either saline (adjuvant only), EBNA-1, or Sm on Day 0 in CFA, then received boost injections (in IFA) on Days 10 and Day 28. Mice were euthanized on Day 56, spleen and lymph nodes removed, and cell cultures completed. Blood samples were procured for serum prior to initial immunization (Day -3), and on Days 10, 28, and 56 after initial immunization. Separate groups of EBNA-1 immunized mice completed Days 0–10 or Days 0–28 of the protocol. Each experimental group contained 6–8 mice across two separate experiments.
Lymph Node (LN) Cell Culture
Single cell suspensions (4 x 106/ml) of axillary, mesenteric, and inguinal draining LNs from mice were cultured in RPMI 1640 with 5% heat-inactivated fetal calf serum (FCS; VWR International, Radnor, PA), 10 uM 2-mercaptoethanol (Life Technologies/Thermo Fisher Scientific, Waltham, MA), penicillin and streptomycin. Cells were cultured in medium alone or in the presence of 50 ug/ml EBNA-1 mosaic (EBNA-1 with truncated glycine-alanine rich region), Sm antigen, the EBNA-1 homologous antigenic peptide PPPGRRP, the Sm homologous antigenic peptide PPPGMRPP, or 5 ng/ml PMA + 500 ng/ml ionomycin (positive control; purchased from Sigma-Aldrich/MilliporeSigma). Bulk quantities of the peptides PPPGRRP and PPPGMRPP were constructed on polylysine backbones (MAP™, Applied Biosystems, Foster City, CA) by the University of Oklahoma Health Sciences Center Molecular Biology-Proteomics facility. Antigen specific proliferation was determined in 72 h 96-well cultures by pulsing with 1 µCi/well [3H]TdR (GE Healthcare/Amersham Biosciences) at 48 h, and cpm was determined by liquid scintillation 24 h later. Culture supernatants were collected at optimal culture times for cytokine analysis: 48 h for IL-6, TNF-α, and IL-10, and 72 h for IFN-γ and IL-17A.
Cytokine ELISA
Cytokine concentrations in culture supernatants were determined by ELISA, using cytokine-specific coating and biotinylated detection antibodies diluted per manufacturer’s protocol (eBioscience/Invitrogen/Thermo Fisher Scientific). Streptavidin-HRP (Jackson ImmunoResearch Laboratories, Inc., West Grove, PA) binding to biotinylated detection antibodies was visualized with TMB substrate (KPL/Seracare, Milford, MA) and the reaction was stopped with 0.18 M H2SO4. Plates were read at 450 nm via Emax Plus Reader (Molecular Devices, San Jose, CA). Data were analyzed with SoftMax Pro software (Molecular Devices); unknowns were compared with a standard curve containing at least five to seven dilution points of the relevant recombinant cytokine (eBioscience/Invitrogen/Thermo Fisher Scientific) on each assay plate. In all cases, the coefficient of determination for the standard curve (r2) was ≥0.98. ELISA unknowns were diluted to fall within the range of standard values.
Anti-EBNA-1 and Anti-Sm Serology
Standard solid-phase assays were used to measure the antibody reactivity in mouse sera, as described previously (86). One µg of Sm (Immunovision, Springdale, AR) or EBNA-1 mosaic (BiosPacific) was coated per well in each of 96 polystyrene wells/plate. Mouse sera at a dilution of 1:100 (Sm) or 1:1000 (EBNA-1) were incubated in each well for 3 hrs. After incubation, plates were washed and incubated with anti-mouse alkaline phosphatase-conjugated γ-chain-specific goat IgG (Sigma-Aldrich/MilliporeSigma) at 1/10,000 dilution. Para nitrophenyl phosphate disodium (PNPP, Sigma-Aldrich/MilliporeSigma) was used as a substrate for alkaline phosphatase, and plates were read at 405 nm via Emax Plus reader (Molecular Devices). ELISA tests were considered positive if the optical density (OD) was at least two standard deviations above the naïve/adjuvant control mean.
Solid-Phase Peptide Synthesis and Anti-Peptide Assays
Sequential, overlapping octapeptides from EBNA-1 and Sm BB’ were synthesized at the ends of radiation-derivatized polyethylene pins arranged in a 96-well microtiter plate format, as described previously (19, 51). All unique octapeptides (EBNA-1 aa 1–103 and 288–641; Sm BB’ aa 1-233) were synthesized, while duplicate octapeptides (especially in the glycine–alanine-rich region of EBNA-1 [aa 97–321]) were omitted. Positive control pins were synthesized from a known reactive sequence of the Sm B’ protein (PPPGMRPP) and used with previously characterized reactive (positive) and non-reactive (negative) sera as standards. Sera from mCD40-LMP1 Tg, mCD40 WT, and CD40-deficient mice were tested for binding with the EBNA-1 or Sm BB’ octapeptides by a solid-phase ELISA-based immunoassay, as previously described (19, 51, 86, 91). Briefly, individual solid-phase peptides were incubated with a 1:100 dilution of mouse sera for 2 h at room temperature. Each pin block was washed and incubated with anti-mouse IgG Fc-specific alkaline phosphatase conjugate or with anti-human IgG alkaline phosphatase conjugate for the positive controls (Jackson Immunoresearch Laboratories), overnight at 4°C. Pin blocks were washed, then incubated at 37°C with PNPP substrate until positive control wells had absorbance readings of 1.0 at 405 nm. A well-characterized human positive control serum was used to normalize the results among multiple plates. Reactivity against an octapeptide was considered positive if the absorbance was at least four standard deviations above the naïve/adjuvant control mean.
Autoantibody Detection and Renal Function Tests
Sera were assessed for anti-nuclear antibodies (ANA; Alpha Diagnostic International, San Antonio, TX), anti-dsDNA antibodies (Alpha Diagnostic Int’l), blood urea nitrogen (BUN; Arbor Assays, Ann Arbor, MI), and serum creatinine (Arbor Assays) per manufacturers’ protocols. For ANA and anti-dsDNA assays, sera were measured in duplicate at a 1:100 dilution in a 96-well plate format, and the HRP-coupled secondary Ab was goat anti mouse IgG (H and L). Negative and positive control sera, as well as 5 point calibration curve samples, provided by the manufacturer, were run concurrently with the unknown samples. Sera were diluted 1:10 for BUN assays and 1:30 for creatinine assays, per manufacturers’ protocols. Sera were run in duplicate alongside a 5 (creatinine) or 7 (BUN) point standard curve. All assays were read at 450 nm using an Emax Plus Reader (Molecular Devices). Unknowns were compared with a calibration curve containing five dilution points on each assay plate. In all cases, the coefficient of determination for the standard curve (r2) was ≥0.98.
Statistical Analyses
Analyses were performed with GraphPad version 7.02 Instat software. Student’s paired t-test was used to determine significance between paired groups. One-way ANOVA with Dunnett’s multiple comparison test was used to determine significance between >2 groups. P-value ≤0.05 was considered significant.
Results
Assessment of LMP1 Functional Mimicry in the Context of EBNA-1
We have previously demonstrated that mCD40-LMP1 Tg mice exhibit mild autoimmunity, marked by lymphadenopathy, splenomegaly, enhanced cytokine secretion, and autoantibody production (71). We therefore asked how mCD40-LMP1 would influence antigen-specific inflammatory responses and lupus-like pathogenic features in the context of EBNA-1. Based on our previous studies assessing EBNA-1 humoral immunity in animal models (86, 90), mCD40-LMP1 Tg mice and congenic controls (B6, mCD40Tg, and B6.CD40-deficient mice) were immunized (in CFA) with EBNA-1 or its cross-reactive autoantigen, Sm, and boosted (in IFA) over a 56-day course (Figure 2). Additional mice completed either a 10-, or 28-day EBNA-1 immunization/booster protocol to determine cellular and humoral immune response kinetics. Sera were collected for serology and renal function testing, lymph nodes for assessment of antigen recall responses, and spleens for assessment of splenomegaly and activation capacity of T- and B-lymphocytes.
mCD40-LMP1 Tg Mice Mount Accelerated and Enhanced Cellular Immune Response to EBNA-1 Immunization
To compare the cellular immune response to EBNA-1 and its antigenic epitope PPPGRRP, homologous to a comparable sequence in the lupus autoantigen Sm, draining lymph node cells from EBNA-1-immunized mCD40-LMP1 Tg, congenic WT, or CD40-deficient mice were cultured in the presence or absence of antigen (Figure 3 and Supplementary Figure 1). All three strains of mice were able to mount a proliferative antigen recall response against EBNA-1 56 days after initial EBNA-1 immunization (Figure 3A). However, mCD40-LMP1 mice showed a significantly greater response to EBNA-1 (p<0.01) and PPPGRRP (p<0.001), even after the proliferative response in mCD40-LMP1 mice shifted over time away from an EBNA-1 antigenic response toward PPPGRRP (from 10 to 28 to 56 days post-immunization). Similarly, all strains of mice produced cytokines after PMA/ionomycin stimulation as a positive control, but antigen-specific cytokine secretion was significantly enhanced in mCD40-LMP1 mice, with some unique differences between EBNA-1 and PPPGRRP stimulation (Figures 3B–F). Both EBNA-1 and PPPGRRP stimulated high levels of IL-17 (Figure 3B) and IFN-γ (Figure 3C) in EBNA-1-immunized mCD40-LMP1 mice, where IL-17 and IFN-γ responses increased over time, reaching the maximum levels seen in day 56 WT mice by day 10. The IL-10 response (Figure 3D) was also elevated, though relatively delayed compared to IL-17 and IFN-γ. Interestingly, IL-6 was secreted much more robustly in response to EBNA-1 than to PPPGRRP in mCD40-LMP1 mice, with minimal response to either antigen in CD40 WT mice (Figure 3E). Conversely, TNF-α secretion increased after both EBNA-1 and PPGRRP antigenic stimulation as early as 10 days post-immunization in mCD40-LMP1 mice and by day 56 in mCD40 WT mice, but not in CD40-deficient mice (Figure 3F).
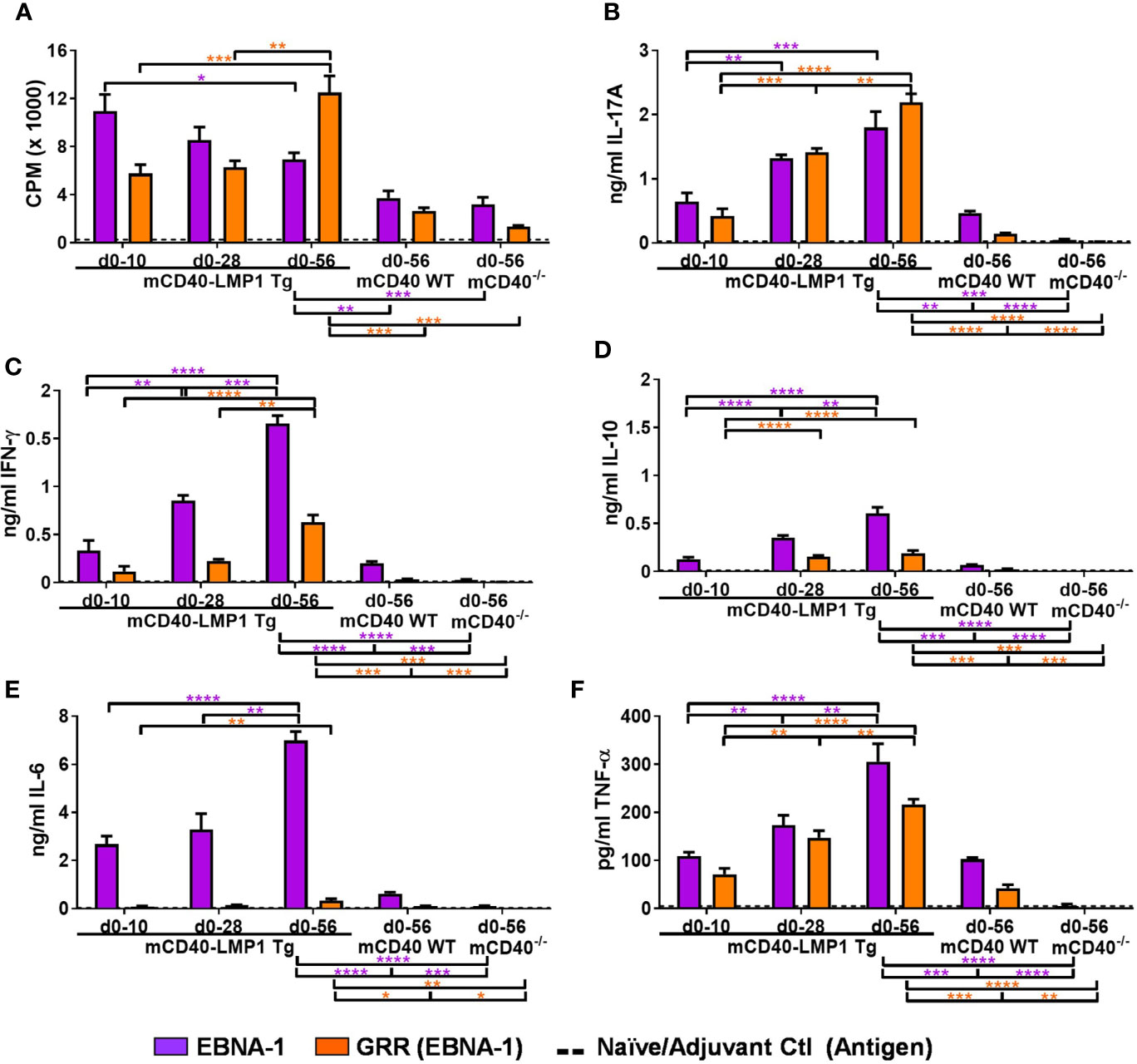
Figure 3 Enhanced antigen recall response to EBNA-1 and homologous region PPPGRRP in mCD40-LMP1 Tg mice. Lymph node cells (4x 106/ml) were cultured in the presence of 50 µg/ml EBNA-1 (mosaic) vs. 50 µg/ml PPPGRRP (GRR; EBNA-1 antigenic epitope). Cell cultures were assessed for proliferation (A) and cell culture supernatant IL-17A (B), IFN-γ (C), IL-10 (D), IL-6 (E), and TNF-α (F). Antigen recall response from mCD40-LMP1 Tg mice was compared to that of CD40 WT mice and CD40-/- mice (Figure 2). Data presented as mean ± SEM. *p ≤ 0.05, **p ≤ 0.01, ***p < 0.001, ****p < 0.0001 one way ANOVA with Dunnett’s multiple comparison test. Significance between experimental groups of mice designated above bar graphs (mCD40-LMP1 mice over time) and below bar graphs (mCD40-LMP1 mice vs. CD40 WT vs. CD40-/- mice); purple = EBNA-1; orange = GRR. A minimal cellular response was exhibited by naïve/adjuvant control mice (dotted line near bottom of y-axis). A minimal cellular response was exhibited by d0-10 and d0-28 mCD40 WT and CD40-deficient mice; only d0-56 data are shown. Antigenic stimulation vs. medium only and PMA/ionomycin is presented in Supplementary Figure 1.
Concurrently Reactive Cellular Immune Response Between EBNA-1 and Sm in EBNA-1 Immunized mCD40-LMP1 Mice
The humoral response to EBNA-1 cross reacts to lupus autoantigens, including, Sm (35, 44, 51). Given the strong antigen-specific cellular immune response in mCD40-LMP1 mice ( (70) and Figure 3/Supplementary Figure 1), we asked if cellular concurrent reactivity occurred between EBNA-1 and Sm in the context of LMP1 (Figure 4 and Supplementary Figure 2). We therefore measured antigen recall responses to Sm and its critical humoral epitope homologous to EBNA-1, PPPGMRPP, in the same mice where EBNA-1 antigenic recall responses were measured in Figure 3. Similar to the EBNA-1 response, mCD40-LMP1 mice had an enhanced cellular immune response to Sm and PPPGMRPP compared to mCD40 WT and CD40-deficient mice, with respect to both proliferation (Figure 4A) and cytokine secretion (Figures 4B–F). In addition to proliferation, Sm and PPPGMRPP antigen stimulation elicited a robust IL-17A response in mCD40-LMP1 mice immunized with EBNA-1 (Figure 4B). Sm and PPGMRPP also stimulated IFN-γ (Figure 4C) and IL-10 (Figure 4D) responses in EBNA-1 immunized mCD40-LMP1 mice, but to a lesser degree than the primary antigen, EBNA-1 (Figures 3C, D). Of note, IL-6 (Figure 4E) showed a response to Sm, but not PPPGMRPP, and TNF-α (Figure 4F) only exhibited PPPGMRPP cellular responses in mCD40-LMP1 mice. The response to Sm in mCD40 WT and CD40-deficient mice immunized with EBNA-1 suggests that these mice do mount a response to EBNA-1, and that concurrent/cross-reactivity of this response may have a CD40-independent component.
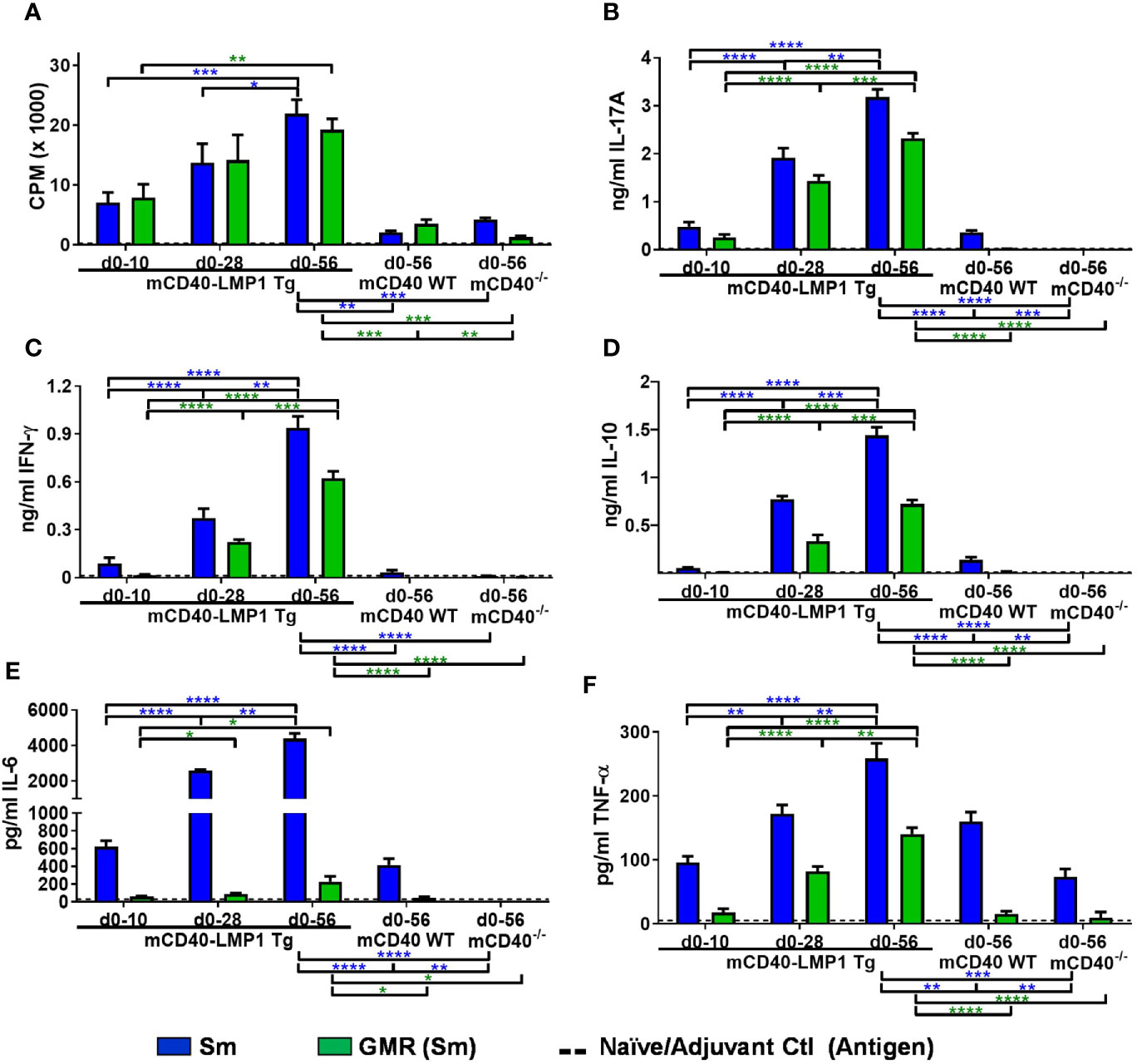
Figure 4 EBNA-1 immunization leads to lupus autoantigen Sm and homologous region PPPGMRPP cellular T cell responses in CD40-LMP1-Tg mice. Lymph node cells (4e6/ml; carried over from Figure 3) were cultured in the presence of 50 µg/ml Sm vs. 50 µg/ml PPPGMRPP (GMR; Sm antigenic epitope). Cell cultures were assessed for proliferation (A) and cell culture supernatant IL-17A (B), IFN-γ (C), IL-10 (D), IL-6 (E), and TNF-α (F). Antigen recall response from mCD40-LMP1 Tg mice was compared to that of CD40 WT mice and CD40-/- mice (Figure 2). Data presented as mean ± SEM. *p ≤ 0.05, **p ≤ 0.01, ***p < 0.001, ****p < 0.0001 one way ANOVA with Dunnett’s multiple comparison test. Significance between experimental groups of mice designated above bar graphs (mCD40-LMP1 mice over time) and below bar graphs (mCD40-LMP1 mice vs. CD40 WT vs. CD40-/- mice); blue = Sm; green = GMR. A minimal cellular response was exhibited by naïve/adjuvant control mice (dotted line near bottom of y-axis). Antigenic stimulation vs. medium only and PMA/ionomycin is presented in Supplementary Figure 2.
Primary and Concurrently Reactive Response After Sm Immunization in mCD40-LMP1 vs. mCD40 WT and mCD40-Deficient Mice
Because EBNA-1 immunization of mCD40-LMP1 mice produced a strong EBNA-1 cellular immune response that concurrently reacted with Sm and its homologous epitope PPPGMRPP (Figures 3, 4), we tested whether Sm immunization of mCD40-LMP1 mice would produce heightened primary (Sm) and concurrently-reactive (EBNA-1) cellular immune responses (Figures 5, 6 and Supplementary Figures 3, 4). Indeed, compared to control mice, mCD40-LMP1 mice exhibited enhanced proliferative (Figure 5A) and cytokine (Figures 5B–F) responses to Sm as the primary antigen, as well against as its antigenic peptide PPPGMRPP, except for a lack of IL-10 after PPPGMRPP stimulation (Figure 5D). Unlike EBNA-1 immunization, Sm immunization did lead to a detectable Sm-specific cellular response in mCD40 WT, and to a lesser extent, mCD40-deficient mice.
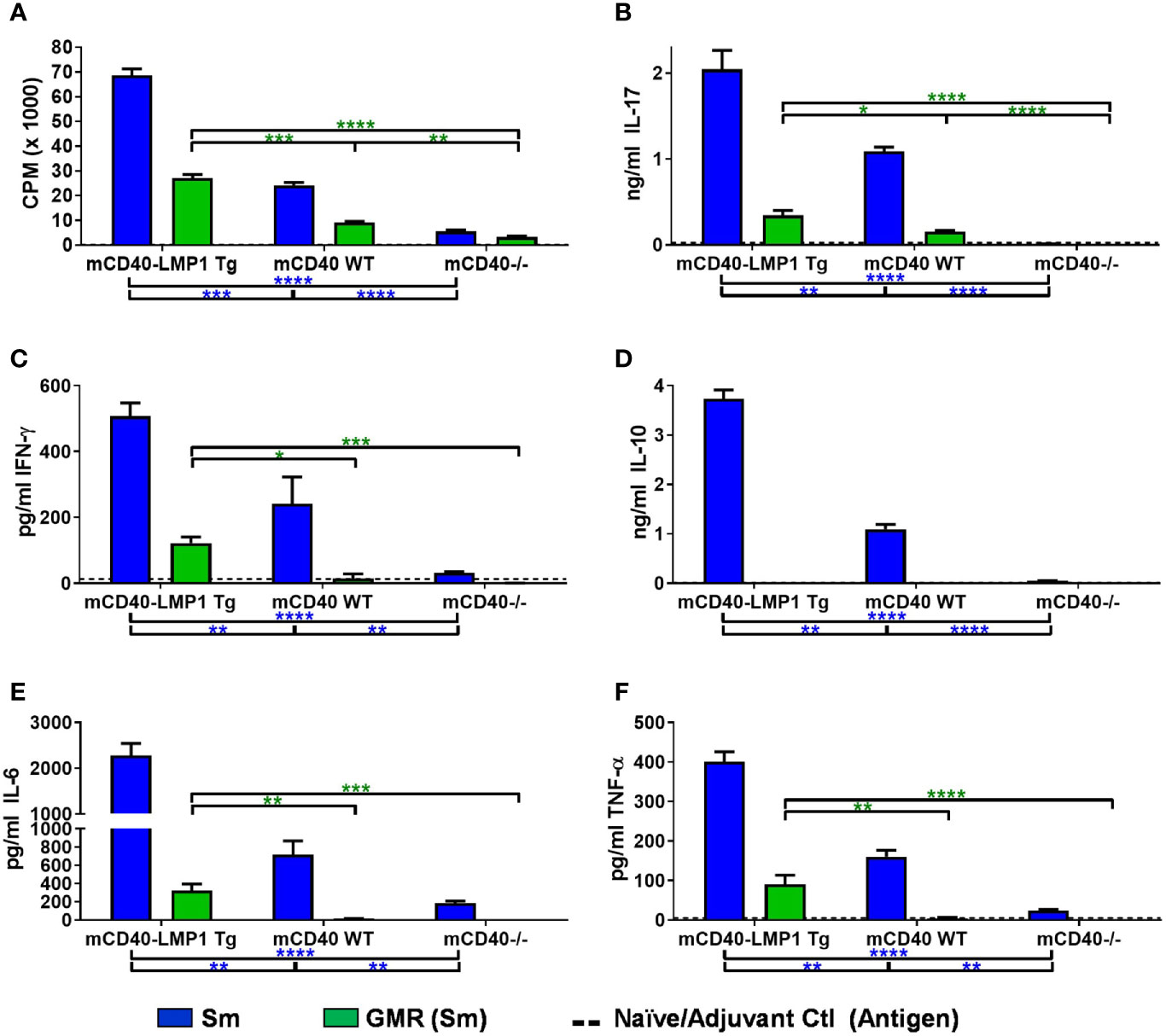
Figure 5 Enhanced antigen recall response to lupus autoantigen Sm and unique reactivity to homologous region PPPGMRPP in mCD40-LMP1 Tg mice. Lymph node cells (4e6/ml) were cultured in the presence of culture medium alone vs. 50 µg/ml Sm, 50 µg/ml PPPGMRPP (GMR; Sm antigenic epitope), and 5 ng/ml PMA/500ng/ml ionomycin. Cell cultures were assessed for proliferation (A) and cell culture supernatant IL-17A (B), IFN-γ (C), IL-10 (D), IL-6 (E), and TNF-α (F). Antigen recall response from mCD40-LMP1 Tg mice was compared to that of CD40 WT mice and CD40-/- mice (Figure 2). Data presented as mean ± SEM. *p ≤ 0.05, **p ≤ 0.01, ***p < 0.001, ****p < 0.0001 one way ANOVA with Dunnett’s multiple comparison test. Significance between experimental groups of mice designated above bar graphs (mCD40-LMP1 mice over time) and below bar graphs (mCD40-LMP1 mice vs. CD40 WT vs. CD40-/- mice); blue = Sm; green = GMR. A minimal cellular response was exhibited by naïve/adjuvant control mice (dotted line near bottom of y-axis). Antigenic stimulation vs. medium only and PMA/ionomycin is presented in Supplementary Figure 3.
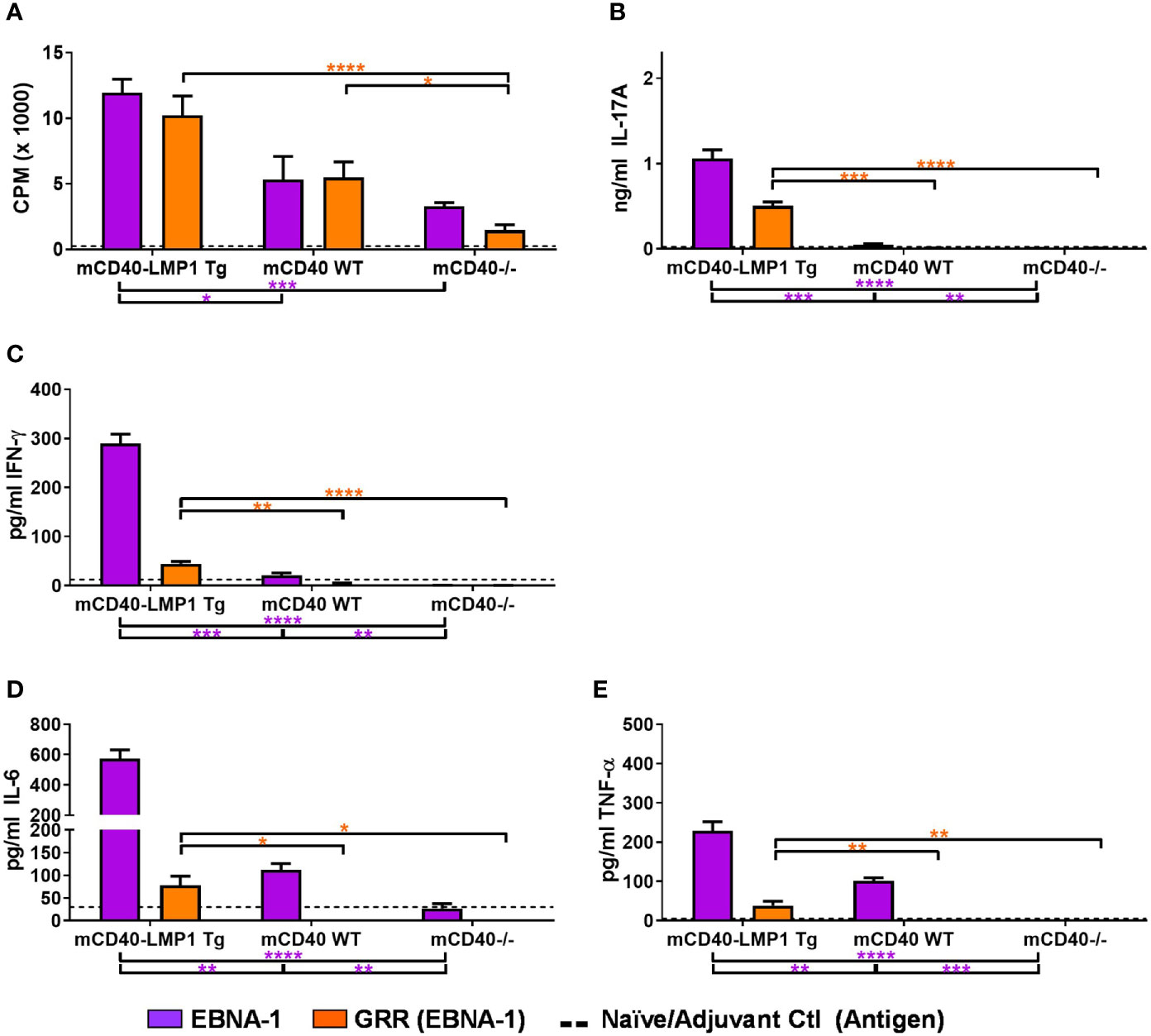
Figure 6 Selective Sm cross-reactivity to EBNA-1 and homologous region PPPGRRP in mCD40-LMP1 Tg mice. Lymph node cells (4e6/ml; carried over from Figure 5) were cultured in the presence of 50 µg/ml EBNA-1 (mosaic) vs.50 µg/ml PPPGRRP (GRR; EBNA-1 antigenic epitope). Cell cultures were assessed for proliferation (A) and cell culture supernatant IL-17A (B), IFN-γ (C), IL-6 (D), and TNF-α (E). Antigen recall response from mCD40-LMP1 Tg mice was compared to that of CD40 WT mice and CD40-/- mice (Figure 2). Data presented as mean ± SEM. *p ≤ 0.05, **p ≤ 0.01, ***p < 0.001, ****p < 0.0001 one way ANOVA with Dunnett’s multiple comparison test. Significance between experimental groups of mice designated above bar graphs (mCD40-LMP1 mice over time) and below bar graphs (mCD40-LMP1 mice vs. CD40 WT vs. CD40-/- mice); purple = EBNA-1; orange = GRR. A minimal cellular response was exhibited by naïve/adjuvant control mice (dotted line near bottom of y-axis). Antigenic stimulation vs. medium only and PMA/ionomycin is presented in Supplementary Figure 4.
Compared to the robust concurrently-reactive Sm response after EBNA-1 immunization (Figures 3, 4), Sm immunization produced a more muted concurrently reactive EBNA-1 response (Figures 5, 6). Nonetheless, mCD40-LMP1 Tg mice did mount a concurrently reactive proliferative and cytokine response to EBNA-1, and to a lesser extent, PPPGRRP, particularly through IL-17 (Figure 6B), IFN-γ (Figure 6C), and TNF-α (Figure 6E). No IL-10 was produced in any Sm-immunized mouse strain in response to EBNA-1 or PPPGRRP (Supplementary Figure 4D). The limited concurrently-reactive EBNA-1 cytokine response in Sm-immunized CD40 WT mice was primarily reflected by readily detectable TNF-α, while mCD40-deficient mice showed no concurrently reactive cytokine response (Figure 6E).
The enhanced, antigen-specific cellular response exhibited in mCD40-LMP1 mice was reflected in an increased presence of activated CD4 T cells before and after EBNA-1 immunization. Further, these mCD40-LMP1 derived T cells were more readily activated by CD3 ± CD28 (Supplementary Figure 5). Although follicular and marginal zone B cells are not different between mCD40-LMP1 and mCD40 WT or CD40-deficient mice, mCD40-LMP1 mice showed enrichment of a CD23lo, CD21/CD35lo immature/activated B cell population, as well as increased proliferative and cytokine responses driven by BCR ± CD154 (CD40L) stimulation (Supplementary Figure 6).
Enhanced Primary and Concurrently Reactive Humoral Immune Responses Between EBNA-1 and Sm in mCD40-LMP1 Tg Mice
Both primary and concurrent, cross-reactive antibody specificities to EBNA-1 and Sm have been observed in SLE (19, 35, 39) and EBNA-1 drives a strong humoral immune response in animal models (42, 44, 86). Therefore, we evaluated whether the anti-EBNA-1 and anti-Sm antibody responses would be enhanced in mCD40-LMP1 mice compared to mCD40 WT or CD40-deficient mice, after EBNA-1 or Sm immunization (Figure 7). Unlike cellular immune responses to either EBNA-1 or Sm, all strains of mice exhibited a readily detectable humoral immune response, although mCD40-deficient mice mounted significantly weaker responses, as expected. Both mCD40-LMP1 Tg and mCD40 WT mice showed a similarly strong, global anti-EBNA-1 antibody response, even as early as 10 days after initial EBNA-1 immunization (Figure 7A). However, mCD40-LMP1 mice mounted an earlier and more robust concurrently-reactive Sm antibody response after EBNA-1 immunization (Figure 7B). After Sm immunization, mCD40-LMP1 mice once again mounted a concurrently-reactive EBNA-1 response (Figure 7C), as well as an enhanced primary response (Sm antigen, Figure 7D) compared to control mice, suggesting that the dysregulated cellular immune response driven by the cytoplasmic tail of LMP1 also extends to humoral immunity.
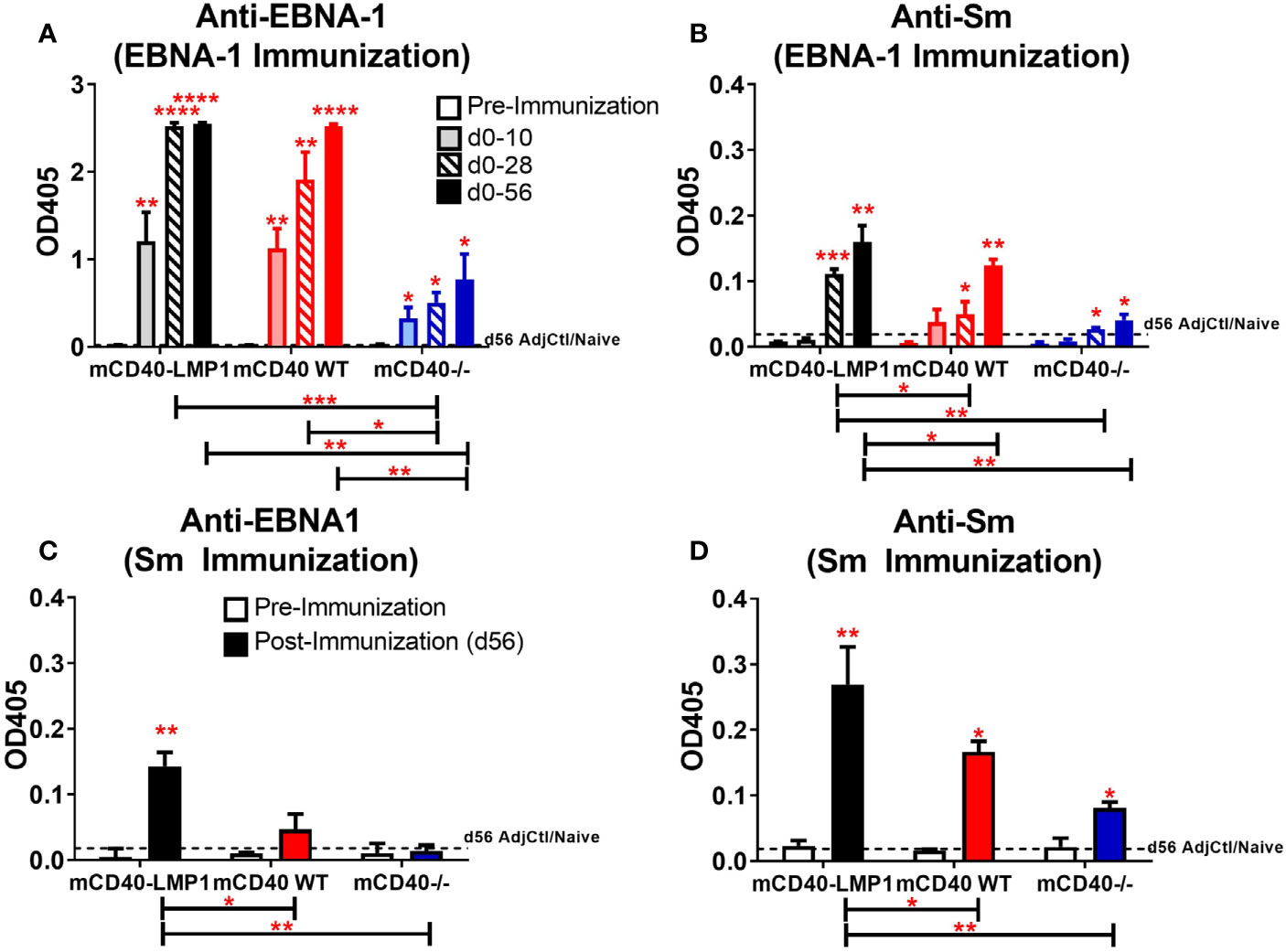
Figure 7 Accelerated humoral reactivity to EBNA-1 and enhanced reactivity/cross-reactivity between EBNA-1 and Sm in mCD40-LMP1 Tg mice. Sera collected at days 10, 28, and 56 (A, B) or day 56 only (C, D) post-immunization with either EBNA-1 (A, B) or Sm (C, D) were assessed for EBNA-1 (A and C, 1:1,000 serum dilution) and Sm (B and D, 1:100 serum dilution). Antibody response from mCD40-LMP1 Tg mice was compared to that of CD40 WT mice and CD40-/- mice (Figure 2). Data presented as mean ± SEM. *p ≤ 0.05, **p ≤ 0.01, ***p < 0.001, ****p < 0.0001 one way ANOVA with Dunnett’s multiple comparison test.
To further characterize the global antibody response to EBNA-1 after EBNA-1 immunization, we mapped the epitope specificity of these responses. Serum reactivity to overlapping octapeptide EBNA-1 epitopes across the EBNA-1 antigen was measured for mCD40-LMP1 Tg, mCD40WT, and mCD40-deficient mice at 10, 28, and 56 days after initial EBNA-1 immunization, compared to adjuvant controls (Figure 8 and Supplementary Figure 7). The patterns of serum interactions across EBNA-1 antigen domains (Figure 8A) showed particular regions of reactivity within the N-terminus (one region displayed in Figure 8B) and C-terminus (one region displayed in Figure 8C) for mCD40-LMP1 Tg mice, mCD40 WT mice, or both. Both mCD40-LMP1 Tg and mCD40 WT mice showed increased responses across the N-terminus (Figure 8D) and C-terminus (Figure 8E) over time (with additional time/EBNA-1 booster immunizations); mCD40-LMP1 mice displayed an enhanced immune response across all time points. Conversely, CD40-deficient mice had a decreasing response over time after initial EBNA-1 immunization, suggesting that an initial CD40-independent antibody response converted to a primarily CD40-dependent response over time.
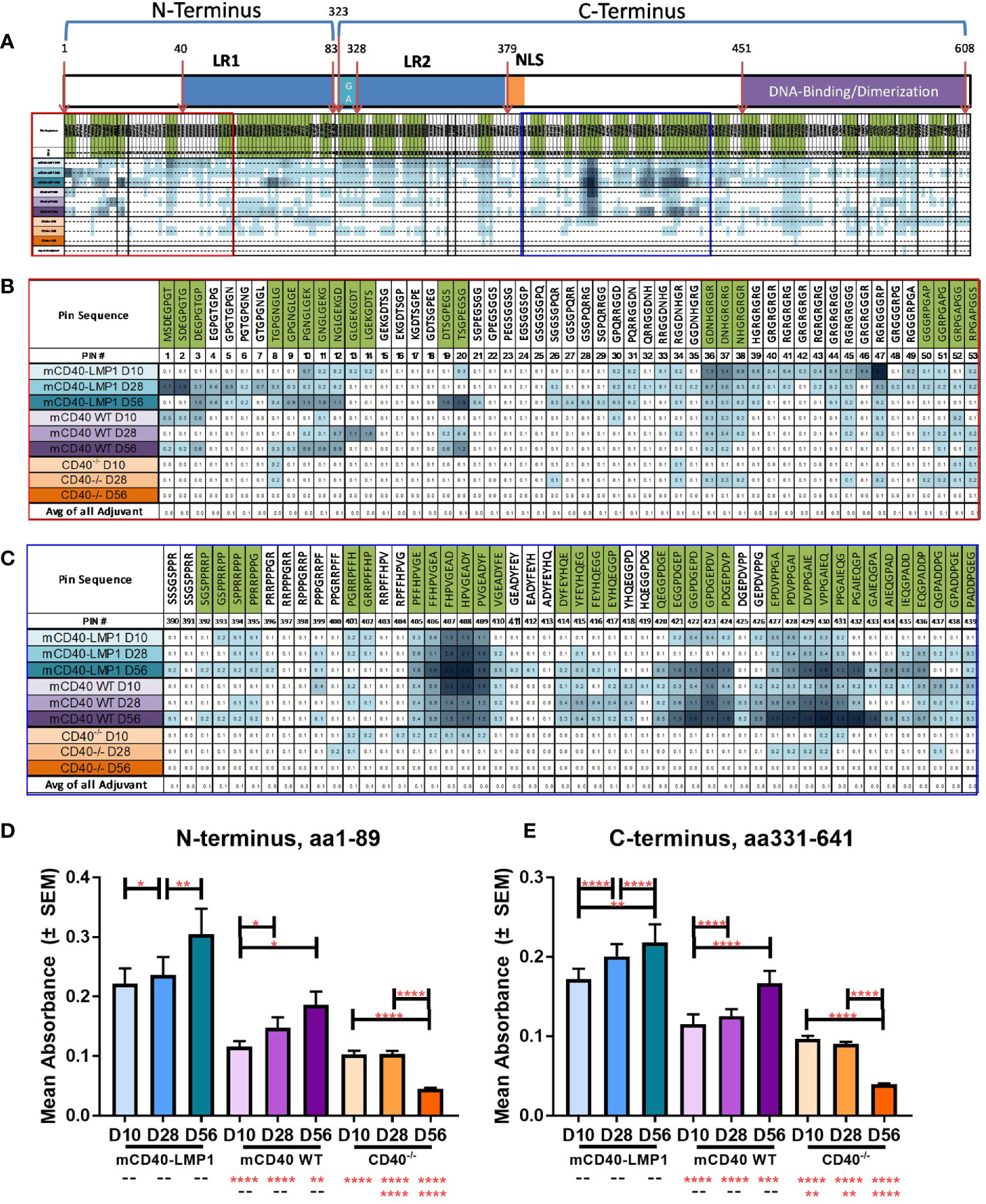
Figure 8 Enhanced EBNA-1 domain-specific humoral response in mCD40-LMP1 Tg mice. EBNA-1 epitope-specific humoral immunity was compared in sera from mCD40-LMP1 Tg mice vs. CD40 WT and CD40-/- mice at 10, 28, and 56 days post-immunization with EBNA-1 (vs. adjuvant/naïve control). EBNA-1 domain map and average reactivity to each EBNA-1 epitope is presented in (A), with green indicating positive epitopes (≥4 SD above adjuvant control). N-terminus area of reactivity from (A) is presented in (B). C-terminus area of reactivity from (A) is presented in (C). Color intensity of each sample block increases with anti-EBNA-1 epitope reactivity (green shaded epitopes are considered positive if ≥4 SD above adjuvant/naïve control). Corresponding epitope mapping histograms are presented in Supplementary Figure 7. Mean ± SEM response to N-terminus (aa1-89, D) and C-terminus (aa331-641, E) are presented. *p ≤ 0.05, **p ≤ 0.01, ***p < 0.001, ****p < 0.0001 one way ANOVA with Dunnett’s multiple comparison test.
Similar serum reactivity to overlapping octapeptide epitopes within the Sm BB’ antigen was measured for mCD40-LMP1 Tg, mCD40WT, and mCD40-deficient mice 56 days after initial Sm immunization, compared to adjuvant controls (Figure 9 and Supplementary Figure 8). Regions of reactivity to Sm BB’ domains (Figure 9A) showed enhanced reactivity in Sm-immunized mice across the Sm1 region in the N-terminus (Figure 9B), with additional reactivity across the C-terminus, including in the PPPGMRPP antigenic region (Figure 9C). Similar to EBNA-1 immunization, immunizing with Sm led to a significantly increased humoral response across both the N-terminus (Figure 9D) and the C-terminus (Figure 9E) in mCD40-LMP1 Tg mice compared to mCD40 WT and CD40-deficient mice; mCD40 WT mice also mounted a significantly greater anti-Sm response across both N- and C-terminal regions compared to CD40-deficient mice. That CD40-deficient mice mounted a small, but measurable response suggests a CD40-independent component to the anti-Sm humoral immune response.
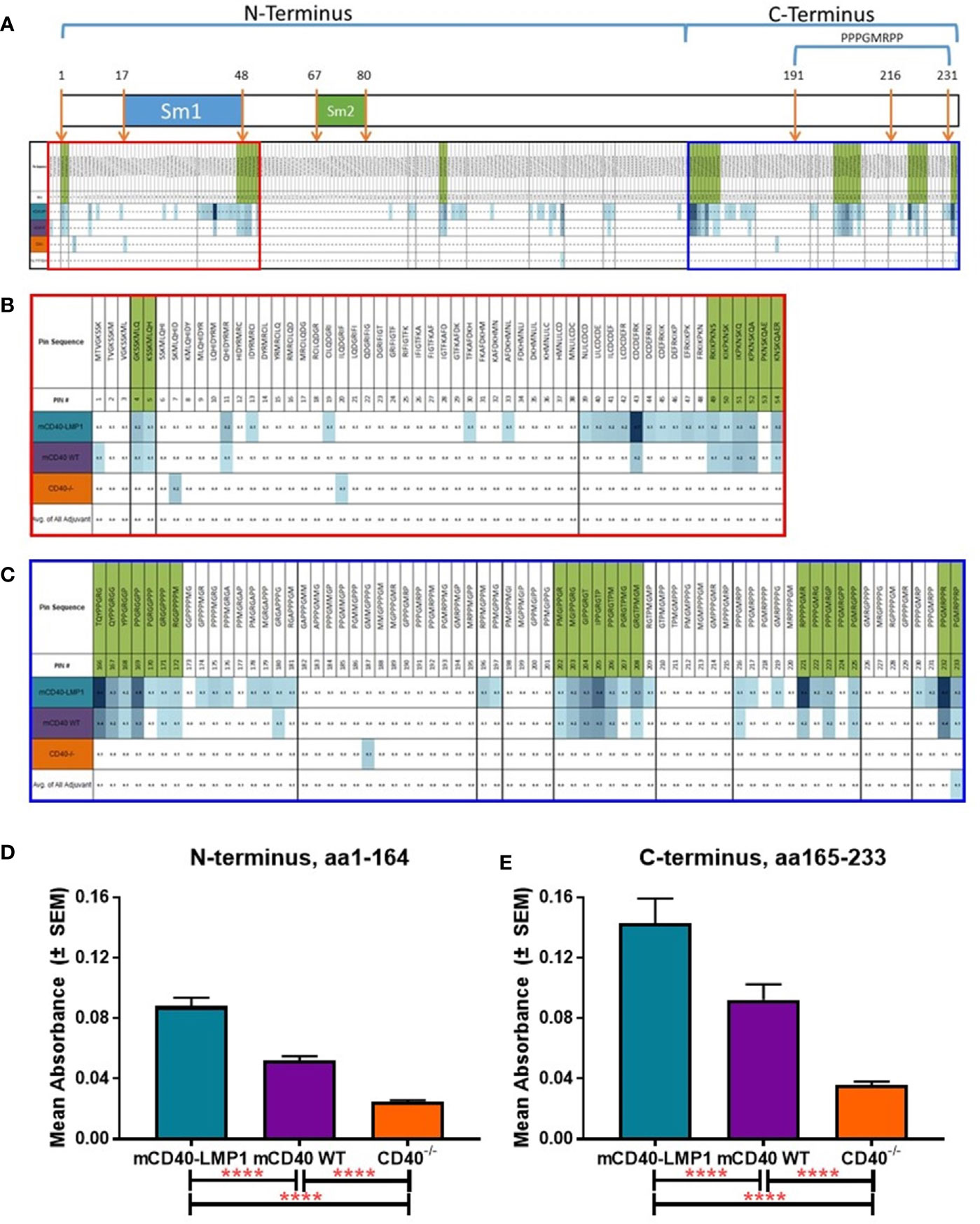
Figure 9 Enhanced Sm BB’ domain-specific humoral response in mCD40-LMP1 Tg mice. Sm BB’ epitope-specific humoral immunity was compared in sera from mCD40-LMP1 Tg mice vs. CD40 WT and CD40-/- mice at 56 days post-immunization with Sm antigen (vs. adjuvant/naïve control). Sm BB’ domain map and average reactivity to each Sm BB’ epitope is presented in (A), with green indicating positive epitopes (≥4 SD above adjuvant control). N-terminus area of reactivity from (A) is presented in (B). C-terminus area of reactivity from (A) is presented in (C). Color intensity of each sample block increases with anti-Sm BB’ epitope reactivity (green shaded epitopes are considered positive if ≥4 SD above adjuvant/naïve control). Corresponding epitope mapping histograms are presented in Supplementary Figure 8. Mean ± SEM response to N-terminus (aa1-164, D) and C-terminus (aa165-233, E) are presented. ****p < 0.0001 one way ANOVA with Dunnett’s multiple comparison test.
Autoimmune Phenotype in mCD40-LMP1 Tg vs. mCD40 WT Mice in Response to EBNA-1 Immunization
Unimmunized mCD40-LMP1 Tg mice show a mild autoimmune phenotype (71) that can be pushed to an inflammatory arthritis phenotype in the context of specific antigen, type II collagen (70), while the congenic mCD40 WT and mCD40-deficient strains are not prone to lupus-like disease. Given the enhanced cellular responses, humoral immunity, and dual-reactivity to lupus autoantigen Sm in mCD40-LMP1 Tg mice immunized with EBNA-1, we assessed the presence of other lupus-like features in this mouse strain (Figure 10). As expected, adjuvant/naïve mCD40-LMP1 Tg mice had enlarged spleens compared to mCD40 WT mice (Figure 10A). EBNA-1 immunization resulted in increased spleen weight in both strains of mice, but to a greater extent in the mCD40-LMP1 Tg mice. With respect to autoantibodies, both mCD40-LMP1 Tg and mCD40 WT mice exhibited increased ANA levels after EBNA-1 immunization, and this was enhanced in the mCD40-LMP1 Tg mice (Figure 10B). In particular, anti-dsDNA autoantibodies were markedly elevated in mCD40-LMP1 Tg mice 56 days after initial EBNA-1 immunization (Figure 10C). Given that anti-dsDNA is associated with lupus nephritis in SLE patients and select lupus nephritis-like mouse models (10), we also assessed serum BUN (Figure 10D) and creatinine (Figure 10E) levels. Both mCD40-LMP1 Tg and mCD40 WT mice showed increases in BUN (Figure 10D) and creatinine (Figure 10E) over time, particularly 56 days after initial EBNA-1 immunization in mCD40-LMP1 mice. However, no overt renal pathology nor areas of inflammatory cell recruitment were observed upon histological examination in either strain (data not shown). These data suggest that while some aspects of lupus-associated autoimmunity seen in mCD40-LMP1 Tg mice, enhanced with EBNA-1 immunization, that this may not be enough to drive classic immune complex glomerulonephritis in the time period assessed.
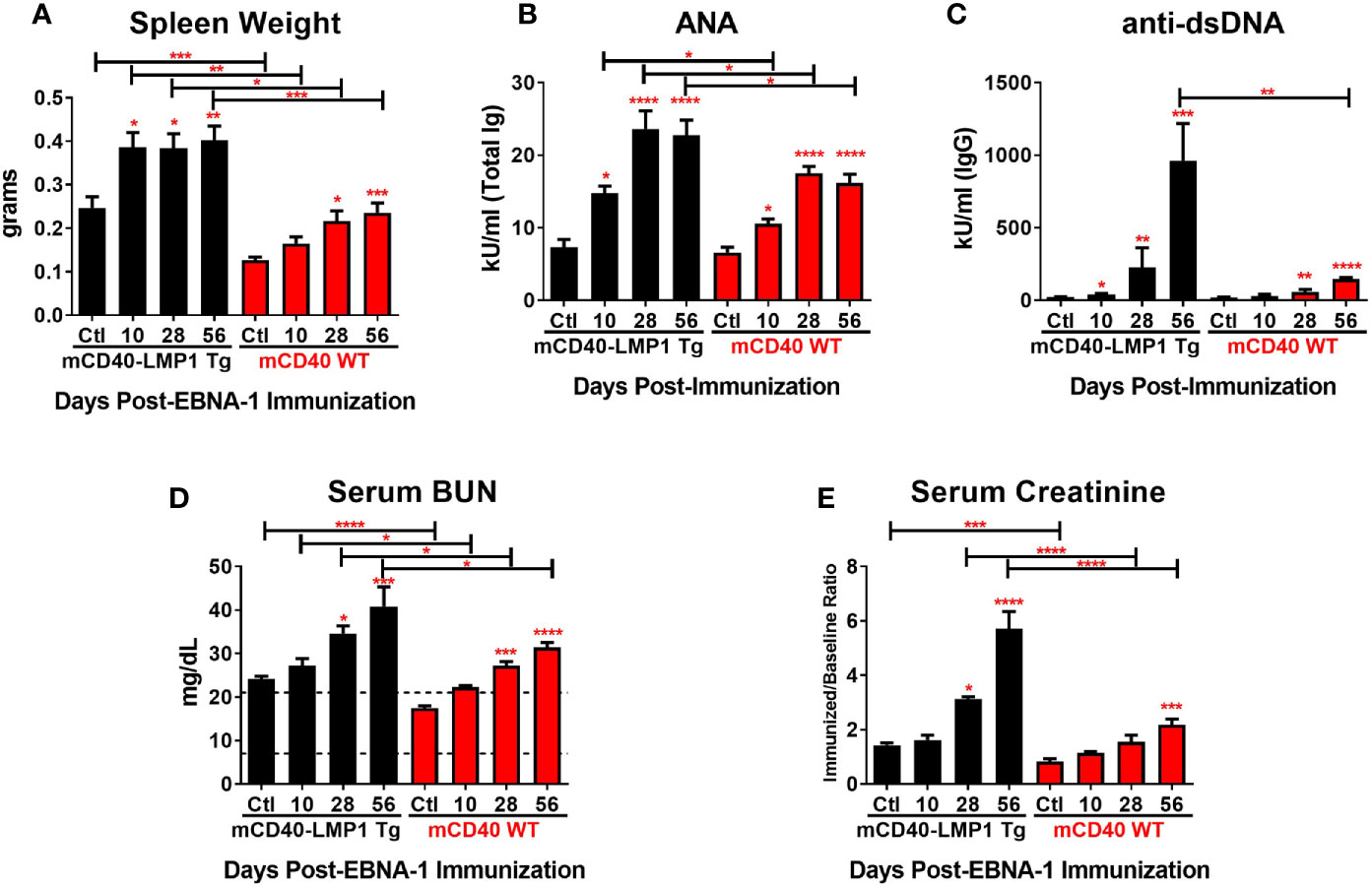
Figure 10 Increased spleen size, autoantibodies, and renal dysfunction in mCD40-LMP1 vs. mCD40 WT over time after EBNA-1 immunization. Mean ± SEM spleen size (A), serum ANA (B), serum anti-dsDNA (C), serum BUN (D), and serum creatinine (E) levels at 10, 28, and 56 days post-EBNA1 immunization (vs. adjuvant/naïve control) in mCD40-LMP1 Tg vs. mCD40 WT mice. *p ≤ 0.05, **p ≤ 0.01, ***p < 0.001, ****p < 0.0001 one way ANOVA with Dunnett’s multiple comparison test.
Discussion
More complete understanding of immune dysregulation in SLE will facilitate proactive interventions with the potential to delay and minimize transition to disease classification, clinical disease flare, and permanent organ damage (5, 92). Despite the ability of a multitude of studies to elucidate genetic risk and highlight immune parameters that may be influenced by genetic variance (12, 13), genetic variation alone incompletely explains lupus pathogenesis. Turning our attention to environmental factors such as EBV with its latent immune mimics has the potential to help us further identify underlying mechanisms of immune dysregulation and opportunities for intervention. The current study expounds on the ability of the EBV-encoded functional immune mimic, LMP1, and molecular mimic, EBNA-1, to dysregulate both cellular and humoral immunity, resulting in reactivity to the SLE-associated autoantigen Sm.
Preclinical SLE is marked by the development of cross-reactive antibodies recognizing both EBNA-1 and autoantigens. Similarly, when immunized with EBNA-1 or Sm, B6 mice expressing WT mCD40 mount both a primary humoral response against the immunizing antigen and a cross-reactive response to Sm or EBNA-1, respectively. In mCD40-LMP1 Tg mice, where the cytoplasmic tail of EBV-encoded CD40 mimic LMP1 drives dysregulated signaling, this response is enhanced, particularly to Sm (primary) and EBNA-1→Sm and Sm→ EBNA-1 concurrent reactivity. Although mCD40 and mCD40-LMP1 mice exhibited a similar response to total EBNA-1, particularly in the C-terminus near the homologous epitope PPPGRRP (aa398-404), mCD40-LMP1 Tg mice had increased reactivity across the N- and C-terminal domains. This was also the case in mCD40-LMP1 Tg mice immunized directly with Sm, with increased reactivity across both N- and C-terminal domains of Sm BB’, particularly in the C-terminus near its homologous epitope PPPGMRPP (aa 191-231). Increased epitope reactivity may allow for enhanced epitope spreading and molecular mimicry/cross-reactivity to lupus associated autoantigens such as Sm. Of note, the modest antibody response to EBNA-1 and Sm in CD40-deficient mice suggests that part of the humoral immune response to these antigens was CD40-independent. The areas of EBNA-1 reactivity were far smaller, but overlapping with mCD40 WT or mCD40-LMP1. A T-independent component of humoral immunity to T-dependent antigens has been demonstrated and likely relies on another TNF-R superfamily member, BLyS/BAFF (93–95).
This study used an immunization protocol designed to induce EBNA-1 humoral immunity in animal models (86), similar to what is observed in SLE patients (19, 39). In addition to an enhanced humoral response, using this immunization strategy in mCD40-LMP1 Tg mice resulted in an enhanced cellular response to EBNA-1/PPPGRRP and Sm/PPPGMRPP, particularly with respect to proliferation, IFN-γ (Th1), and especially IL-17 (Th17) responses, as well as IL-6 and TNF-α. This is not unlike what was observed in the context of type II collagen immunization in the collagen-induced inflammatory arthritis model (70). Of note, the IL-10 response lagged behind other mediators assessed, had less reactivity to the EBNA-1 homologous epitope PPPGRRP, and had no reactivity to the Sm homologous epitope PPPGMRPP. It is possible that the regulatory IL-10 response occurs later than the pro-inflammatory mediator response or that the reactive antigenic region(s) driving an IL-10 response lie(s) outside of the homologous reactive domain for EBNA-1 and Sm. A similar lack of reactivity to the EBNA-1 and Sm homologous domains was also observed with IL-6 secretion. Given that naïve mCD40-LMP1 Tg mice already have increased systemic levels of IL-6 (71), the peptide antigen signal may not be sufficient to drive additional IL-6 production, yet allows for downstream IL-17A secretion. Alternatively, like IL-10, the antigenic region that drives IL-6 production may be outside of the EBNA-1 and Sm homologous domains.
The ability of LMP1 to drive a cellular, concurrently reactive response between EBNA-1 and Sm in vivo suggests a possible route for EBV to contribute to cellular molecular mimicry and immune pathway dysregulation. In the present study, EBNA-1 to Sm dual-reactivity was more robust than Sm to EBNA-1 dual-reactivity, suggesting that EBNA-1 drives concurrently reactive cellular immunity to Sm, and not the reverse. It is not unusual for concurrent, cross-reactive T-lymphocytes to drive immune and autoimmune processes (96). A cellular immune response to EBNA-1 that cross reacts with autoantigen (myelin) has been demonstrated in multiple sclerosis (97–100), despite the common lack of T-lymphocyte control of EBV infection in multiple sclerosis (101, 102) and SLE (103, 104). A cross-reactive cellular immune response to EBNA-1 has yet to be demonstrated in human SLE, but may be best detected during preclinical SLE when cellular immune dysregulation first gives rise to humoral autoimmunity (2–4, 17). Additionally, cross-reactive cellular immunity with EBNA-1 may be apparent during periods of EBV reactivation, when PBMCs are likely to express EBNA-1 and LMP1 (17), especially since LMP1 positive PBMCs coincide directly with immune dysregulation that leads to clinical disease flare (34, 105). Together, our current and previous findings suggest that LMP1 contributes to immune dysregulation that may set the stage for SLE pathogenesis. Sustained and dysregulated cellular immunity driven by LMP1 may allow for a break in tolerance that allows for the production of SLE-associated autoantibodies, including ANA and anti-dsDNA (62, 67, 69, 71). The concurrent expression of EBNA-1 and its role as a molecular mimic may then contribute to accumulation of additional SLE-associated autoantibody specificities, including Sm (9, 19, 35, 39).
Although mCD40-LMP1 Tg mice immunized with EBNA-1 developed ANA and anti-dsDNA autoantibodies, as well as some renal dysfunction with increased BUN and creatinine, no overt renal pathology was noted on histological examination. It is possible that the mCD40-LMP1 Tg mice were just starting to develop nephritis 56 days after initial EBNA-1 immunization and may have developed overt renal pathology if given more time. Alternatively, the B6 strain may be resistant to immune complex glomerulonephritis, thus requiring additional genetic influence even in the context of mCD40-LMP1. Phenotypically, naïve mCD40-LMP1 Tg mice appear similar to B6.Sle2 mice, which exhibit polyclonal antibodies and activated T-cell immunity, but require genes from B6.Sle1 mice to develop overt nephritis (106–108). Crossing mCD40-LMP1 Tg mice with B6.Sle1 mice, but not B6.Sle3 mice, accelerates autoimmunity, including increased cellular immunity, development of anti-dsDNA autoantibodies, and overt renal pathology evidenced by glomerular inflammatory infiltrates (69). Further, LMP1 is expressed in the kidneys of human SLE patients, particularly patients who are positive for anti-Sm autoantibodies (37, 109). This suggests the possibility that enhanced and dysregulated cellular immunity associated with LMP1 functional mimicry (62, 67, 69, 71) may foster anti-Sm and anti-dsDNA autoantibody specificities associated with EBNA-1 molecular mimicry (35, 42–44) to propel some aspects of immune complex-driven lupus nephritis.
We propose that LMP1, in potential conjunction with genetic risk (17, 34, 69, 105), may contribute to immune dysregulation that fosters broken tolerance, enhancing EBNA-1 molecular mimicry and fueling autoantibody production, downstream cellular and tissue damage, and SLE pathogenesis. Although findings in the current study were driven by an mCD40-LMP1 hybrid molecule in the absence of CD40, similar cellular and humoral immune dysregulation has been noted in both in vitro (65) and in vivo (69) mouse studies in the presence of endogenous CD40, as well as in human patients with confirmed LMP1 expression (34, 110–114). Together, these findings suggest a model whereby EBV-encoded latent immune mimics initiate a network of feed-forward loops that contribute to SLE pathogenesis with LMP1 driven immune dysregulation, and EBNA-1 stimulated autoantibody production (Figure 11).
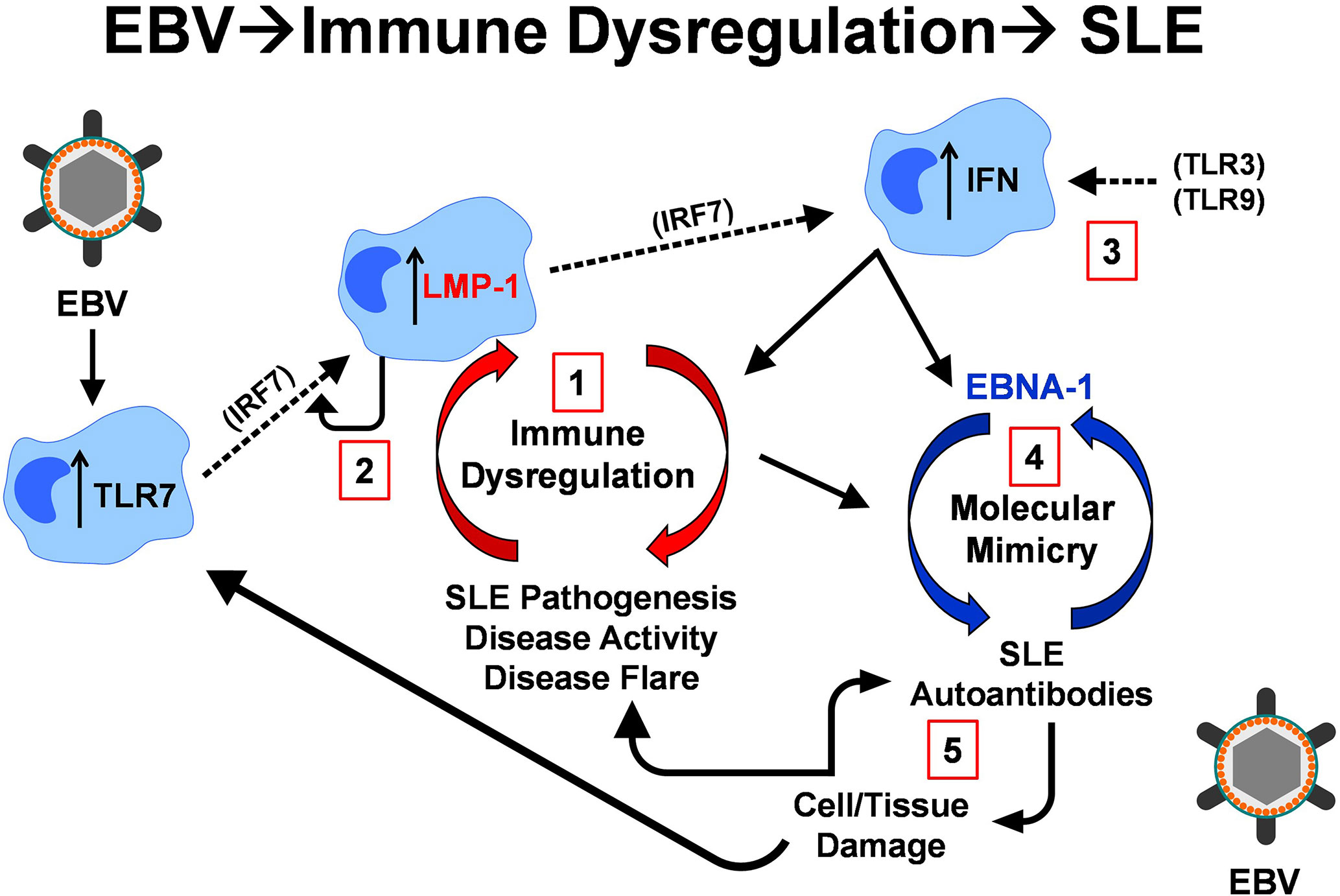
Figure 11 Feed forward model of LMP1 expression, immune dysregulation, and SLE autoimmunity. EBV infection can drive increased TLR7 expression via IRF7, which also stimulates increased LMP1 expression. LMP1, in turn, stimulates IRF7 via RIP to continue this positive autoregulatory loop 1. The very immune dysregulation (innate and adaptive) that LMP1 propels also upregulates LMP1 expression 2. In addition, LMP1 drives interferon (IFN) production via IRF7, in conjunction with other TLRs, including TLR3 and TLR9 3. Type I IFNs (innate response), in addition to the adaptive immune response that LMP1 promotes, can contribute to T cell-mediated antibody/autoantibody production, allowing for the cross-reactive, molecular mimic response between EBNA-1 and lupus autoantigens Ro, Sm, and RNP 4. Autoantibodies, in conjunction with ongoing inflammation, lead to cell and tissue damage, releasing additional lupus-associated autoantigens that interact with TLR7 and continue to drive cellular and humoral autoimmunity 5.
A positive autoregulatory loop that maintains LMP1 expression (Figure 11-1) could perpetuate this cycle. Both SLE-associated genetic polymorphisms (12, 13) and EBV infection can upregulate TLR7 expression via IRF7 (115). TLR7 stimulates LMP1 expression (112), and LMP1, in turn, stimulates IRF7 via RIP, promoting further LMP1 expression. The dysregulation of immune mediators by LMP1 further promotes LMP1 expression (34, 110–112, 116) (Figure 11-2). Of particular interest, the regulatory mediator IL-10, which is upregulated during periods of non-flare in SLE patients (6, 7), promotes LMP1 expression (110), which then has the potential to drive inflammatory immune dysregulation leading to a subsequent period of increased clinical disease activity and flare (34, 105).
LMP1 drives additional forms of immune dysregulation that contribute to SLE disease pathogenesis (3), clinical disease activity and flare (5–7), including type I IFN and Th1-, Th2-, and Th17-type immunity. Type I IFN is produced in response to LMP1-mediated IRF7 stimulation (84), in conjunction with other TLRs, including TLR3 and TLR9 (117–120) (Figure 11-3). Together, type I IFNs (innate response) and the adaptive immune responses enhanced by LMP1 [current study and (70, 113, 114, 116)] can contribute to T cell-mediated antibody/autoantibody production, facilitating cross-reactive responses between molecular mimic EBNA-1 and lupus autoantigens (35) (Figure 11-4). In addition to our findings in the current study, we and others have demonstrated cross-reactivity between EBNA-1 and lupus autoantigens, both in animal models and human SLE patients, including Ro/SSA (19, 39, 40, 86), Sm (19, 39, 44, 121), RNP (39, 51, 86), and dsDNA (39, 40, 42–44). Autoantibodies and ongoing inflammation (122) cause cellular and tissue damage that releases more lupus associated autoantigens, which can interact with TLR7 to further propagate cellular and humoral autoimmunity (112, 117, 123–126) (Figure 11-5).
In addition to cellular expression of LMP1, the LMP1 transmembrane domain enables extracellular expression on vesicles and exosomes (127), where it can be internalized (128), including by dendritic cells (129). This allows for LMP1-induced cellular proliferation and activation (128, 130, 131), as well as antibody production and class-switching in non-infected B cells (132). This would allow for LMP1 expression in cells other than B-lymphocytes [and epithelial cells, which are also tropic for EBV (133)] and drive additional pathogenicity.
Our findings suggest that LMP1 can both promote cellular immune dysregulation and potentiate EBNA-1 humoral immunity and dual-reactivity with the lupus autoantigen Sm. Such dysregulation may be necessary, yet insufficient, to explain SLE pathogenesis. Over 90% of the general population is EBV seropositive (134), yet only a subset of individuals develop SLE or other autoimmune diseases. Indeed, EBNA-1 molecular mimicry and LMP1-mediated immune dysregulation have been noted in patients with mononucleosis (51, 135–137), but this does not lead to autoimmune disease in most patients. It is possible that immune dysregulation fostered by EBV latent mimics provides a break in immune tolerance that creates an opportunity for SLE-associated genetic risk variants to drive SLE pathogenesis (12, 30, 138–140). In a mouse model of lupus-like disease that associates phenotype with genetic risk [B6.Sle1.Sle2.Sle3 mice (107)], we have previously demonstrated that mCD40-LMP1 Tg mice accelerate lupus-like autoimmunity in B6.Sle1, but not B6.Sle3 mice [mCD40-LMP1 Tg mice are phenotypically similar to B6.Sle2 mice (71, 107)], including histologic evidence of glomerulonephritis) (69). Further, SLE patients experiencing heightened clinical disease activity and flare have been shown to exhibit an altered type I IFN gene signature that is associated with LMP1 expression in PBMCs (34). Immune dysregulation that contributes to SLE pathogenesis, clinical disease activity, and organ damage may be further augmented by lifestyle and other environmental factors, including smoking (141–144), UV exposure (145–147), and changes in gut microbiome (148–150). Future studies that further elucidate the relationship in gene-environment interactions, including EBV-encoded latent mimics, have the potential to better define windows of therapeutic opportunity for targeted treatments.
Data Availability Statement
The raw data supporting the conclusions of this article will be made available by the authors, without undue reservation.
Ethics Statement
The animal study was reviewed and approved by Oklahoma Medical Research Foundation Institutional Animal Care & Use Committee (IACUC).
Author Contributions
MM managed mouse colonies, designed and carried out experiments, completed data analysis, and principally wrote manuscript. JA and TG provided technical support. LS and GB provided transgenic mice and Hi5 (WT and mCD154) insect cells, as well as experimental and editorial guidance. JJ provided additional support, as well as experimental and editorial guidance. All authors contributed to the article and approved the submitted version.
Funding
This work was supported by the National Institute of Arthritis and Musculoskeletal and Skin Diseases and the National Institute of General Medical Sciences through the NIH (P30RR031152, UM1AI144292, P30AR073750, and U54GM104938). The contents are solely the responsibility of the authors and do not necessarily represent the official views of the NIH or one of its institutes. This work was also supported by the OMRF Lou C. Kerr Chair in Biomedical Research to JJ.
Conflict of Interest
The authors declare that the research was conducted in the absence of any commercial or financial relationships that could be construed as a potential conflict of interest.
Acknowledgments
The authors wish to thank Rebecka Bourn, Ph.D. for her editorial assistance.
Supplementary Material
The Supplementary Material for this article can be found online at: https://www.frontiersin.org/articles/10.3389/fimmu.2020.606936/full#supplementary-material
References
1. Slight-Webb S, Smith M, Bylinska A, Macwana S, Guthridge C, Lu R, et al. Autoantibody-positive healthy individuals with lower lupus risk display a unique immune endotype. J Allergy Clin Immunol (2020) 146:1419–33. doi: 10.1016/j.jaci.2020.04.047
2. Munroe ME, Young KA, Kamen DL, Guthridge JM, Niewold TB, Costenbader KH, et al. Discerning Risk of Disease Transition in Relatives of Systemic Lupus Erythematosus Patients Utilizing Soluble Mediators and Clinical Features. Arthritis Rheumatol (2017) 69:630–42. doi: 10.1002/art.40004
3. Lu R, Munroe ME, Guthridge JM, Bean KM, Fife DA, Chen H, et al. Dysregulation of Innate and Adaptive Serum Mediators Precedes Systemic Lupus Erythematosus Classification and Improves Prognostic Accuracy of Autoantibodies. J Autoimmun (2016) 74:182–93. doi: 10.1016/j.jaut.2016.06.001
4. Munroe ME, Lu R, Zhao YD, Fife DA, Robertson JM, Guthridge JM, et al. Altered type II interferon precedes autoantibody accrual and elevated type I interferon activity prior to systemic lupus erythematosus classification. Ann Rheum Dis (2016) 75:2014–21. doi: 10.1136/annrheumdis-2015-208140
5. Lu R, Guthridge JM, Chen H, Bourn RL, Kamp S, Munroe ME, et al. Immunologic findings precede rapid lupus flare after transient steroid therapy. Sci Rep (2019) 9:8590. doi: 10.1038/s41598-019-45135-w
6. Munroe ME, Vista ES, Merrill JT, Guthridge JM, Roberts VC, James JA. Pathways of impending disease flare in African-American systemic lupus erythematosus patients. J Autoimmun (2017) 78:70–8. doi: 10.1016/j.jaut.2016.12.005
7. Munroe ME, Vista ES, Guthridge JM, Thompson LF, Merrill JT, James JA. Pro-inflammatory adaptive cytokines and shed tumor necrosis factor receptors are elevated preceding systemic lupus erythematosus disease flare. Arthritis Rheumatol (2014) 66:1888–99. doi: 10.1002/art.38573
8. Bruce IN, O’Keeffe AG, Farewell V, Hanly JG, Manzi S, Su L, et al. Factors associated with damage accrual in patients with systemic lupus erythematosus: results from the Systemic Lupus International Collaborating Clinics (SLICC) Inception Cohort. Ann Rheum Dis (2015) 74:1706–13. doi: 10.1136/annrheumdis-2013-205171
9. McClain MT, Heinlen LD, Dennis GJ, Roebuck J, Harley JB, James JA. Early events in lupus humoral autoimmunity suggest initiation through molecular mimicry. Nat Med (2005) 11:85–9. doi: 10.1038/nm1167
10. Fenton K. The effect of cell death in the initiation of lupus nephritis. Clin Exp Immunol (2015) 179:11–6. doi: 10.1111/cei.12417
11. Ulff-Moller CJ, Svendsen AJ, Viemose LN, Jacobsen S. Concordance of autoimmune disease in a nationwide Danish systemic lupus erythematosus twin cohort. Semin Arthritis Rheum (2018) 47:538–44. doi: 10.1016/j.semarthrit.2017.06.007
12. Reid S, Alexsson A, Frodlund M, Morris D, Sandling JK, Bolin K, et al. High genetic risk score is associated with early disease onset, damage accrual and decreased survival in systemic lupus erythematosus. Ann Rheum Dis (2020) 79:363–9. doi: 10.1136/annrheumdis-2019-216227
13. Langefeld CD, Ainsworth HC, Cunninghame Graham DS, Kelly JA, Comeau ME, Marion MC, et al. Transancestral mapping and genetic load in systemic lupus erythematosus. Nat Commun (2017) 8:16021. doi: 10.1038/ncomms16021
14. Kwon YC, Chun S, Kim K, Mak A. Update on the Genetics of Systemic Lupus Erythematosus: Genome-Wide Association Studies and Beyond. Cells (2019) 8:1180. doi: 10.3390/cells8101180
15. Chen L, Morris DL, Vyse TJ. Genetic advances in systemic lupus erythematosus: an update. Curr Opin Rheumatol (2017) 29:423–33. doi: 10.1097/BOR.0000000000000411
16. Tsokos GC, Lo MS, Costa Reis P, Sullivan KE. New insights into the immunopathogenesis of systemic lupus erythematosus. Nat Rev Rheumatol (2016) 12:716–30. doi: 10.1038/nrrheum.2016.186
17. Jog NR, Young KA, Munroe ME, Harmon MT, Guthridge JM, Kelly JA, et al. Association of Epstein-Barr virus serological reactivation with transitioning to systemic lupus erythematosus in at-risk individuals. Ann Rheum Dis (2019) 78:1235–41. doi: 10.1136/annrheumdis-2019-215361
18. Aygun D, Kuskucu MA, Sahin S, Adrovic A, Barut K, Yildiz M, et al. Epstein-Barr virus, cytomegalovirus and BK polyomavirus burden in juvenile systemic lupus erythematosus: correlation with clinical and laboratory indices of disease activity. Lupus (2020) 29:1263–69 . doi: 10.1177/0961203320940029
19. McClain MT, Poole BD, Bruner BF, Kaufman KM, Harley JB, James JA. An altered immune response to Epstein-Barr nuclear antigen 1 in pediatric systemic lupus erythematosus. Arthritis Rheum (2006) 54:360–8. doi: 10.1002/art.21682
20. Chougule D, Nadkar M, Rajadhyaksha A, Pandit-Shende P, Surve P, Dawkar N, et al. Association of clinical and serological parameters of systemic lupus erythematosus patients with Epstein-Barr virus antibody profile. J Med Virol (2018) 90:559–63. doi: 10.1002/jmv.24904
21. James JA, Robertson JM. Lupus and Epstein-Barr. Curr Opin Rheumatol (2012) 24:383–8. doi: 10.1097/BOR.0b013e3283535801
22. Dugan JP, Coleman CB, Haverkos B. Opportunities to Target the Life Cycle of Epstein-Barr Virus (EBV) in EBV-Associated Lymphoproliferative Disorders. Front Oncol (2019) 9:127. doi: 10.3389/fonc.2019.00127
23. Taylor GS, Long HM, Brooks JM, Rickinson AB, Hislop AD. The immunology of Epstein-Barr virus-induced disease. Annu Rev Immunol (2015) 33:787–821. doi: 10.1146/annurev-immunol-032414-112326
24. Kieser A, Sterz KR. The Latent Membrane Protein 1 (LMP1). Curr Top Microbiol Immunol (2015) 391:119–49. doi: 10.1007/978-3-319-22834-1_4
25. Chatterjee K, Das P, Chattopadhyay NR, Mal S, Choudhuri T. The interplay between Epstein-Bar virus (EBV) with the p53 and its homologs during EBV associated malignancies. Heliyon (2019) 5:e02624. doi: 10.1016/j.heliyon.2019.e02624
26. Klein A, Polliack A, Gafter-Gvili A. Systemic lupus erythematosus and lymphoma: Incidence, pathogenesis and biology. Leuk Res (2018) 75:45–9. doi: 10.1016/j.leukres.2018.11.004
27. Wang LW, Jiang S, Gewurz BE. Epstein-Barr Virus LMP1-Mediated Oncogenicity. J Virol (2017) 91:e01718–6. doi: 10.1128/JVI.01718-16
28. Draborg A, Izarzugaza JM, Houen G. How compelling are the data for Epstein-Barr virus being a trigger for systemic lupus and other autoimmune diseases? Curr Opin Rheumatol (2016) 28:398–404. doi: 10.1097/BOR.0000000000000289
29. Draborg AH, Duus K, Houen G. Epstein-Barr virus in systemic autoimmune diseases. Clin Dev Immunol (2013) 2013:535738. doi: 10.1155/2013/535738
30. Niller HH, Wolf H, Minarovits J. Regulation and dysregulation of Epstein-Barr virus latency: implications for the development of autoimmune diseases. Autoimmunity (2008) 41:298–328. doi: 10.1080/08916930802024772
31. Piroozmand A, Haddad Kashani H, Zamani B. Correlation between Epstein-Barr Virus Infection and Disease Activity of Systemic Lupus Erythematosus: a Cross-Sectional Study. Asian Pac J Cancer Prev (2017) 18:523–7. doi: 10.22034/APJCP.2017.18.2.523
32. Moon UY, Park SJ, Oh ST, Kim WU, Park SH, Lee SH, et al. Patients with systemic lupus erythematosus have abnormally elevated Epstein-Barr virus load in blood. Arthritis Res Ther (2004) 6:R295–302. doi: 10.1186/ar1181
33. Poole BD, Templeton AK, Guthridge JM, Brown EJ, Harley JB, James JA. Aberrant Epstein-Barr viral infection in systemic lupus erythematosus. Autoimmun Rev (2009) 8:337–42. doi: 10.1016/j.autrev.2008.12.008
34. Han L, Zhang Y, Wang Q, Xin M, Yang K, Lei K, et al. Epstein-Barr virus infection and type I interferon signature in patients with systemic lupus erythematosus. Lupus (2018) 27:947–54. doi: 10.1177/0961203317753069
35. Poole BD, Scofield RH, Harley JB, James JA. Epstein-Barr virus and molecular mimicry in systemic lupus erythematosus. Autoimmunity (2006) 39:63–70. doi: 10.1080/08916930500484849
36. Sivachandran N, Wang X, Frappier L. Functions of the Epstein-Barr virus EBNA1 protein in viral reactivation and lytic infection. J Virol (2012) 86:6146–58. doi: 10.1128/JVI.00013-12
37. Ding Y, He X, Liao W, Yi Z, Yang H, Xiang W. The expression of EBV-encoded LMP1 in young patients with lupus nephritis. Int J Clin Exp Med (2015) 8:6073–8.
38. Draborg AH, Jorgensen JM, Muller H, Nielsen CT, Jacobsen S, Iversen LV, et al. Epstein-Barr virus early antigen diffuse (EBV-EA/D)-directed immunoglobulin A antibodies in systemic lupus erythematosus patients. Scand J Rheumatol (2012) 41:280–9. doi: 10.3109/03009742.2012.665944
39. Vista ES, Weisman MH, Ishimori ML, Chen H, Bourn RL, Bruner BF, et al. Strong viral associations with SLE among Filipinos. Lupus Sci Med (2017) 4:e000214. doi: 10.1136/lupus-2017-000214
40. Cuomo L, Cirone M, Di Gregorio AO, Vitillo M, Cattivelli M, Magliocca V, et al. Elevated antinuclear antibodies and altered anti-Epstein-Barr virus immune responses. Virus Res (2015) 195:95–9. doi: 10.1016/j.virusres.2014.09.014
41. Heinlen LD, McClain MT, Ritterhouse LL, Bruner BF, Edgerton CC, Keith MP, et al. 60 kD Ro and nRNP A frequently initiate human lupus autoimmunity. PLoS One (2010) 5:e9599. doi: 10.1371/journal.pone.0009599
42. Yadav P, Tran H, Ebegbe R, Gottlieb P, Wei H, Lewis RH, et al. Antibodies elicited in response to EBNA-1 may cross-react with dsDNA. PLoS One (2011) 6:e14488. doi: 10.1371/journal.pone.0014488
43. Yadav P, Carr MT, Yu R, Mumbey-Wafula A, Spatz LA. Mapping an epitope in EBNA-1 that is recognized by monoclonal antibodies to EBNA-1 that cross-react with dsDNA. Immun Inflamm Dis (2016) 4:362–75. doi: 10.1002/iid3.119
44. Sundar K, Jacques S, Gottlieb P, Villars R, Benito ME, Taylor DK, et al. Expression of the Epstein-Barr virus nuclear antigen-1 (EBNA-1) in the mouse can elicit the production of anti-dsDNA and anti-Sm antibodies. J Autoimmun (2004) 23:127–40. doi: 10.1016/j.jaut.2004.06.001
45. Boudreault S, Armero VES, Scott MS, Perreault JP, Bisaillon M. The Epstein-Barr virus EBNA1 protein modulates the alternative splicing of cellular genes. Virol J (2019) 16:29. doi: 10.1186/s12985-019-1137-5
46. Urlaub H, Raker VA, Kostka S, Luhrmann R. Sm protein-Sm site RNA interactions within the inner ring of the spliceosomal snRNP core structure. EMBO J (2001) 20:187–96. doi: 10.1093/emboj/20.1.187
47. Kang MS, Kieff E. Epstein-Barr virus latent genes. Exp Mol Med (2015) 47:e131. doi: 10.1038/emm.2014.84
48. Munz C, Lunemann JD, Getts MT, Miller SD. Antiviral immune responses: triggers of or triggered by autoimmunity? Nat Rev Immunol (2009) 9:246–58. doi: 10.1038/nri2527
49. Arbuckle MR, McClain MT, Rubertone MV, Scofield RH, Dennis GJ, James JA, et al. Development of autoantibodies before the clinical onset of systemic lupus erythematosus. N Engl J Med (2003) 349:1526–33. doi: 10.1056/NEJMoa021933
50. Dunmire SK, Odumade OA, Porter JL, Reyes-Genere J, Schmeling DO, Bilgic H, et al. Primary EBV infection induces an expression profile distinct from other viruses but similar to hemophagocytic syndromes. PLoS One (2014) 9:e85422. doi: 10.1371/journal.pone.0085422
51. McClain MT, Rapp EC, Harley JB, James JA. Infectious mononucleosis patients temporarily recognize a unique, cross-reactive epitope of Epstein-Barr virus nuclear antigen-1. J Med Virol (2003) 70:253–7. doi: 10.1002/jmv.10385
52. Peters AL, Stunz LL, Bishop GA. CD40 and autoimmunity: the dark side of a great activator. Semin Immunol (2009) 21:293–300. doi: 10.1016/j.smim.2009.05.012
53. Haxhinasto SA, Hostager BS, Bishop GA. Cutting edge: molecular mechanisms of synergy between CD40 and the B cell antigen receptor: role for TNF receptor-associated factor 2 in receptor interaction. J Immunol (2002) 169:1145–9. doi: 10.4049/jimmunol.169.3.1145
54. Haxhinasto SA, Bishop GA. Synergistic B cell activation by CD40 and the B cell antigen receptor: role of B lymphocyte antigen receptor-mediated kinase activation and tumor necrosis factor receptor-associated factor regulation. J Biol Chem (2004) 279:2575–82. doi: 10.1074/jbc.M310628200
55. Buchta CM, Bishop GA. Toll-like receptors and B cells: functions and mechanisms. Immunol Res (2014) 59:12–22. doi: 10.1007/s12026-014-8523-2
56. Peters AL, Plenge RM, Graham RR, Altshuler DM, Moser KL, Gaffney PM, et al. A novel polymorphism of the human CD40 receptor with enhanced function. Blood (2008) 112:1863–71. doi: 10.1182/blood-2008-02-138925
57. Vanden Bush TJ, Bishop GA. TLR7 and CD40 cooperate in IL-6 production via enhanced JNK and AP-1 activation. Eur J Immunol (2008) 38:400–9. doi: 10.1002/eji.200737602
58. Souyris M, Mejia JE, Chaumeil J, Guery JC. Female predisposition to TLR7-driven autoimmunity: gene dosage and the escape from X chromosome inactivation. Semin Immunopathol (2019) 41:153–64. doi: 10.1007/s00281-018-0712-y
59. Celhar T, Magalhaes R, Fairhurst AM. TLR7 and TLR9 in SLE: when sensing self goes wrong. Immunol Res (2012) 53:58–77. doi: 10.1007/s12026-012-8270-1
60. Christensen SR, Shlomchik MJ. Regulation of lupus-related autoantibody production and clinical disease by Toll-like receptors. Semin Immunol (2007) 19:11–23. doi: 10.1016/j.smim.2006.12.005
61. Arcipowski KM, Bishop GA. Roles of the kinase TAK1 in TRAF6-dependent signaling by CD40 and its oncogenic viral mimic, LMP1. PLoS One (2012) 7:e42478. doi: 10.1371/journal.pone.0042478
62. Kraus ZJ, Nakano H, Bishop GA. TRAF5 is a critical mediator of in vitro signals and in vivo functions of LMP1, the viral oncogenic mimic of CD40. Proc Natl Acad Sci U S A (2009) 106:17140–5. doi: 10.1073/pnas.0903786106
63. Graham JP, Moore CR, Bishop GA. Roles of the TRAF2/3 binding site in differential B cell signaling by CD40 and its viral oncogenic mimic, LMP1. J Immunol (2009) 183:2966–73. doi: 10.4049/jimmunol.0900442
64. Wu S, Xie P, Welsh K, Li C, Ni CZ, Zhu X, et al. LMP1 protein from the Epstein-Barr virus is a structural CD40 decoy in B lymphocytes for binding to TRAF3. J Biol Chem (2005) 280:33620–6. doi: 10.1074/jbc.M502511200
65. Xie P, Hostager BS, Bishop GA. Requirement for TRAF3 in signaling by LMP1 but not CD40 in B lymphocytes. J Exp Med (2004) 199:661–71. doi: 10.1084/jem.20031255
66. Xie P, Bishop GA. Roles of TNF receptor-associated factor 3 in signaling to B lymphocytes by carboxyl-terminal activating regions 1 and 2 of the EBV-encoded oncoprotein latent membrane protein 1. J Immunol (2004) 173:5546–55. doi: 10.4049/jimmunol.173.9.5546
67. Arcipowski KM, Stunz LL, Bishop GA. TRAF6 is a critical regulator of LMP1 functions in vivo. Int Immunol (2014) 26:149–58. doi: 10.1093/intimm/dxt052
68. Arcipowski KM, Bishop GA. TRAF binding is required for a distinct subset of in vivo B cell functions of the oncoprotein LMP1. J Immunol (2012) 189:5165–70. doi: 10.4049/jimmunol.1201821
69. Peters AL, Stunz LL, Meyerholz DK, Mohan C, Bishop GA. Latent Membrane Protein 1, the EBV-Encoded Oncogenic Mimic of CD40, Accelerates Autoimmunity in B6.Sle1 Mice. J Immunol (2010) 185:4053–62. doi: 10.4049/jimmunol.0904065
70. Munroe ME, Arbiser JL, Bishop GA. Honokiol, a natural plant product, inhibits inflammatory signals and alleviates inflammatory arthritis. J Immunol (2007) 179:753–63. doi: 10.4049/jimmunol.179.2.753
71. Stunz LL, Busch LK, Munroe ME, Sigmund CD, Tygrett LT, Waldschmidt TJ, et al. Expression of the cytoplasmic tail of LMP1 in mice induces hyperactivation of B lymphocytes and disordered lymphoid architecture. Immunity (2004) 21:255–66. doi: 10.1016/j.immuni.2004.07.008
72. Pratt ZL, Sugden B. How human tumor viruses make use of autophagy. Cells (2012) 1:617–30. doi: 10.3390/cells1030617
73. Pai S, Khanna R. Role of LMP1 in immune control of EBV infection. Semin Cancer Biol (2001) 11:455–60. doi: 10.1006/scbi.2001.0412
74. Brown KD, Hostager BS, Bishop GA. Differential signaling and tumor necrosis factor receptor-associated factor (TRAF) degradation mediated by CD40 and the Epstein-Barr virus oncoprotein latent membrane protein 1 (LMP1). J Exp Med (2001) 193:943–54. doi: 10.1084/jem.193.8.943
75. Rowland SL, Tremblay MM, Ellison JM, Stunz LL, Bishop GA, Hostager BS. A novel mechanism for TNFR-associated factor 6-dependent CD40 signaling. J Immunol (2007) 179:4645–53. doi: 10.4049/jimmunol.179.7.4645
76. Hostager BS, Haxhinasto SA, Rowland SL, Bishop GA. Tumor necrosis factor receptor-associated factor 2 (TRAF2)-deficient B lymphocytes reveal novel roles for TRAF2 in CD40 signaling. J Biol Chem (2003) 278:45382–90. doi: 10.1074/jbc.M306708200
77. Xie P, Hostager BS, Munroe ME, Moore CR, Bishop GA. Cooperation between TNF receptor-associated factors 1 and 2 in CD40 signaling. J Immunol (2006) 176:5388–400. doi: 10.4049/jimmunol.176.9.5388
78. Hostager BS, Bishop GA. Cutting edge: contrasting roles of TNF receptor-associated factor 2 (TRAF2) and TRAF3 in CD40-activated B lymphocyte differentiation. J Immunol (1999) 162:6307–11.
79. Greenfeld H, Takasaki K, Walsh MJ, Ersing I, Bernhardt K, Ma Y, et al. TRAF1 Coordinates Polyubiquitin Signaling to Enhance Epstein-Barr Virus LMP1-Mediated Growth and Survival Pathway Activation. PLoS Pathog (2015) 11:e1004890. doi: 10.1371/journal.ppat.1004890
80. Eliopoulos AG, Waites ER, Blake SM, Davies C, Murray P, Young LS. TRAF1 is a critical regulator of JNK signaling by the TRAF-binding domain of the Epstein-Barr virus-encoded latent infection membrane protein 1 but not CD40. J Virol (2003) 77:1316–28. doi: 10.1128/JVI.77.2.1316-1328.2003
81. Schultheiss U, Puschner S, Kremmer E, Mak TW, Engelmann H, Hammerschmidt W, et al. TRAF6 is a critical mediator of signal transduction by the viral oncogene latent membrane protein 1. EMBO J (2001) 20:5678–91. doi: 10.1093/emboj/20.20.5678
82. Soni V, Cahir-McFarland E, Kieff E. LMP1 TRAFficking activates growth and survival pathways. Adv Exp Med Biol (2007) 597:173–87. doi: 10.1007/978-0-387-70630-6_14
83. Luftig M, Prinarakis E, Yasui T, Tsichritzis T, Cahir-McFarland E, Inoue J, et al. Epstein-Barr virus latent membrane protein 1 activation of NF-kappaB through IRAK1 and TRAF6. Proc Natl Acad Sci U S A (2003) 100:15595–600. doi: 10.1073/pnas.2136756100
84. Song YJ, Izumi KM, Shinners NP, Gewurz BE, Kieff E. IRF7 activation by Epstein-Barr virus latent membrane protein 1 requires localization at activation sites and TRAF6, but not TRAF2 or TRAF3. Proc Natl Acad Sci U S A (2008) 105:18448–53. doi: 10.1073/pnas.0809933105
85. Campbell IK, Hamilton JA, Wicks IP. Collagen-induced arthritis in C57BL/6 (H-2b) mice: new insights into an important disease model of rheumatoid arthritis. Eur J Immunol (2000) 30:1568–75. doi: 10.1002/1521-4141(200006)30:6<1568::AID-IMMU1568>3.0.CO;2-R
86. Poole BD, Gross T, Maier S, Harley JB, James JA. Lupus-like autoantibody development in rabbits and mice after immunization with EBNA-1 fragments. J Autoimmun (2008) 31:362–71. doi: 10.1016/j.jaut.2008.08.007
87. James JA, Harley JB. Linear epitope mapping of an Sm B/B’ polypeptide. J Immunol (1992) 148:2074–9.
88. Graham JP, Arcipowski KM, Bishop GA. Differential B-lymphocyte regulation by CD40 and its viral mimic, latent membrane protein 1. Immunol Rev (2010) 237:226–48. doi: 10.1111/j.1600-065X.2010.00932.x
89. Stunz LL, Bishop GA. Latent membrane protein 1 and the B lymphocyte-a complex relationship. Crit Rev Immunol (2014) 34:177–98. doi: 10.1615/CritRevImmunol.2014010041
90. James JA, Harley JB. A model of peptide-induced lupus autoimmune B cell epitope spreading is strain specific and is not H-2 restricted in mice. J Immunol (1998) 160:502–8.
91. Arbuckle MR, Schilling AR, Harley JB, James JA. A limited lupus anti-spliceosomal response targets a cross-reactive, proline-rich motif. J Autoimmun (1998) 11:431–8. doi: 10.1006/jaut.1998.0227
92. Olsen NJ, James JA, Arriens C, Ishimori ML, Wallace DJ, Kamen DL, et al. Study of Anti-Malarials in Incomplete Lupus Erythematosus (SMILE): study protocol for a randomized controlled trial. Trials (2018) 19:694. doi: 10.1186/s13063-018-3076-7
93. El Shikh ME, El Sayed RM, Szakal AK, Tew JG. T-independent antibody responses to T-dependent antigens: a novel follicular dendritic cell-dependent activity. J Immunol (2009) 182:3482–91. doi: 10.4049/jimmunol.0802317
94. Fagarasan S, Honjo T. T-Independent immune response: new aspects of B cell biology. Science (2000) 290:89–92. doi: 10.1126/science.290.5489.89
95. Schneider P. The role of APRIL and BAFF in lymphocyte activation. Curr Opin Immunol (2005) 17:282–9. doi: 10.1016/j.coi.2005.04.005
96. Petrova G, Ferrante A, Gorski J. Cross-reactivity of T cells and its role in the immune system. Crit Rev Immunol (2012) 32:349–72. doi: 10.1615/CritRevImmunol.v32.i4.50
97. Tschochner M, Leary S, Cooper D, Strautins K, Chopra A, Clark H, et al. Identifying Patient-Specific Epstein-Barr Nuclear Antigen-1 Genetic Variation and Potential Autoreactive Targets Relevant to Multiple Sclerosis Pathogenesis. PLoS One (2016) 11:e0147567. doi: 10.1371/journal.pone.0147567
98. Lossius A, Vartdal F, Holmoy T. Vitamin D sensitive EBNA-1 specific T cells in the cerebrospinal fluid of patients with multiple sclerosis. J Neuroimmunol (2011) 240-241:87–96. doi: 10.1016/j.jneuroim.2011.09.011
99. Lunemann JD, Jelcic I, Roberts S, Lutterotti A, Tackenberg B, Martin R, et al. EBNA1-specific T cells from patients with multiple sclerosis cross react with myelin antigens and co-produce IFN-gamma and IL-2. J Exp Med (2008) 205:1763–73. doi: 10.1084/jem.20072397
100. Lunemann JD, Edwards N, Muraro PA, Hayashi S, Cohen JI, Munz C, et al. Increased frequency and broadened specificity of latent EBV nuclear antigen-1-specific T cells in multiple sclerosis. Brain (2006) 129:1493–506. doi: 10.1093/brain/awl067
101. Pender MP, Csurhes PA, Burrows JM, Burrows SR. Defective T-cell control of Epstein-Barr virus infection in multiple sclerosis. Clin Transl Immunol (2017) 6:e126. doi: 10.1038/cti.2016.87
102. Yea C, Tellier R, Chong P, Westmacott G, Marrie RA, Bar-Or A, et al. Epstein-Barr virus in oral shedding of children with multiple sclerosis. Neurology (2013) 81:1392–9. doi: 10.1212/WNL.0b013e3182a841e4
103. Draborg AH, Jacobsen S, Westergaard M, Mortensen S, Larsen JL, Houen G, et al. Reduced response to Epstein-Barr virus antigens by T-cells in systemic lupus erythematosus patients. Lupus Sci Med (2014) 1:e000015. doi: 10.1136/lupus-2014-000015
104. Kang I, Quan T, Nolasco H, Park SH, Hong MS, Crouch J, et al. Defective control of latent Epstein-Barr virus infection in systemic lupus erythematosus. J Immunol (2004) 172:1287–94. doi: 10.4049/jimmunol.172.2.1287
105. Gross AJ, Hochberg D, Rand WM, Thorley-Lawson DA. EBV and systemic lupus erythematosus: a new perspective. J Immunol (2005) 174:6599–607. doi: 10.4049/jimmunol.174.11.6599
106. Morel L. Genetics of SLE: evidence from mouse models. Nat Rev Rheumatol (2010) 6:348–57. doi: 10.1038/nrrheum.2010.63
107. Morel L, Mohan C, Yu Y, Croker BP, Tian N, Deng A, et al. Functional dissection of systemic lupus erythematosus using congenic mouse strains. J Immunol (1997) 158:6019–28.
108. Morel L, Croker BP, Blenman KR, Mohan C, Huang G, Gilkeson G, et al. Genetic reconstitution of systemic lupus erythematosus immunopathology with polycongenic murine strains. Proc Natl Acad Sci U S A (2000) 97:6670–5. doi: 10.1073/pnas.97.12.6670
109. Yu XX, Yao CW, Tao JL, Yang C, Luo MN, Li SM, et al. The expression of renal Epstein-Barr virus markers in patients with lupus nephritis. Exp Ther Med (2014) 7:1135–40. doi: 10.3892/etm.2014.1578
110. Kis LL, Takahara M, Nagy N, Klein G, Klein E. Cytokine mediated induction of the major Epstein-Barr virus (EBV)-encoded transforming protein, LMP-1. Immunol Lett (2006) 104:83–8. doi: 10.1016/j.imlet.2005.11.003
111. Salamon D, Adori M, Ujvari D, Wu L, Kis LL, Madapura HS, et al. Latency type-dependent modulation of Epstein-Barr virus-encoded latent membrane protein 1 expression by type I interferons in B cells. J Virol (2012) 86:4701–7. doi: 10.1128/JVI.06829-11
112. Valente RM, Ehlers E, Xu D, Ahmad H, Steadman A, Blasnitz L, et al. Toll-like receptor 7 stimulates the expression of Epstein-Barr virus latent membrane protein 1. PLoS One (2012) 7:e43317. doi: 10.1371/journal.pone.0043317
113. Xu D, Brumm K, Zhang L. The latent membrane protein 1 of Epstein-Barr virus (EBV) primes EBV latency cells for type I interferon production. J Biol Chem (2006) 281:9163–9. doi: 10.1074/jbc.M511884200
114. Chuang HC, Lay JD, Hsieh WC, Wang HC, Chang Y, Chuang SE, et al. Epstein-Barr virus LMP1 inhibits the expression of SAP gene and upregulates Th1 cytokines in the pathogenesis of hemophagocytic syndrome. Blood (2005) 106:3090–6. doi: 10.1182/blood-2005-04-1406
115. Martin HJ, Lee JM, Walls D, Hayward SD. Manipulation of the toll-like receptor 7 signaling pathway by Epstein-Barr virus. J Virol (2007) 81:9748–58. doi: 10.1128/JVI.01122-07
116. Chen H, Hutt-Fletcher L, Cao L, Hayward SD. A positive autoregulatory loop of LMP1 expression and STAT activation in epithelial cells latently infected with Epstein-Barr virus. J Virol (2003) 77:4139–48. doi: 10.1128/JVI.77.7.4139-4148.2003
117. Soni C, Wong EB, Domeier PP, Khan TN, Satoh T, Akira S, et al. B cell-intrinsic TLR7 signaling is essential for the development of spontaneous germinal centers. J Immunol (2014) 193:4400–14. doi: 10.4049/jimmunol.1401720
118. Patole PS, Grone HJ, Segerer S, Ciubar R, Belemezova E, Henger A, et al. Viral double-stranded RNA aggravates lupus nephritis through Toll-like receptor 3 on glomerular mesangial cells and antigen-presenting cells. J Am Soc Nephrol JASN (2005) 16:1326–38. doi: 10.1681/ASN.2004100820
119. Chauhan SK, Singh VV, Rai R, Rai M, Rai G. Distinct autoantibody profiles in systemic lupus erythematosus patients are selectively associated with TLR7 and TLR9 upregulation. J Clin Immunol (2013) 33:954–64. doi: 10.1007/s10875-013-9887-0
120. Savarese E, Chae OW, Trowitzsch S, Weber G, Kastner B, Akira S, et al. U1 small nuclear ribonucleoprotein immune complexes induce type I interferon in plasmacytoid dendritic cells through TLR7. Blood (2006) 107:3229–34. doi: 10.1182/blood-2005-07-2650
121. Esen BA, Yilmaz G, Uzun S, Ozdamar M, Aksozek A, Kamali S, et al. Serologic response to Epstein-Barr virus antigens in patients with systemic lupus erythematosus: a controlled study. Rheumatol Int (2012) 32:79–83. doi: 10.1007/s00296-010-1573-4
122. Zharkova O, Celhar T, Cravens PD, Satterthwaite AB, Fairhurst AM, Davis LS. Pathways leading to an immunological disease: systemic lupus erythematosus. Rheumatol (Oxford) (2017) 56:i55–66. doi: 10.1093/rheumatology/kew427
123. Chodisetti SB, Fike AJ, Domeier PP, Singh H, Choi NM, Corradetti C, et al. Type II but Not Type I IFN Signaling Is Indispensable for TLR7-Promoted Development of Autoreactive B Cells and Systemic Autoimmunity. J Immunol (2020) 204:796–809. doi: 10.4049/jimmunol.1901175
124. Zumaquero E, Stone SL, Scharer CD, Jenks SA, Nellore A, Mousseau B, et al. IFNgamma induces epigenetic programming of human T-bet(hi) B cells and promotes TLR7/8 and IL-21 induced differentiation. Elife (2019) 8:e41641. doi: 10.7554/eLife.41641
125. Giltiay NV, Chappell CP, Sun X, Kolhatkar N, Teal TH, Wiedeman AE, et al. Overexpression of TLR7 promotes cell-intrinsic expansion and autoantibody production by transitional T1 B cells. J Exp Med (2013) 210:2773–89. doi: 10.1084/jem.20122798
126. Hwang SH, Lee H, Yamamoto M, Jones LA, Dayalan J, Hopkins R, et al. B cell TLR7 expression drives anti-RNA autoantibody production and exacerbates disease in systemic lupus erythematosus-prone mice. J Immunol (2012) 189:5786–96. doi: 10.4049/jimmunol.1202195
127. Nkosi D, Howell LA, Cheerathodi MR, Hurwitz SN, Tremblay DC, Liu X, et al. Transmembrane Domains Mediate Intra- and Extracellular Trafficking of Epstein-Barr Virus Latent Membrane Protein 1. J Virol (2018) 92:e00280-18. doi: 10.1128/JVI.00280-18
128. Nanbo A, Kawanishi E, Yoshida R, Yoshiyama H. Exosomes derived from Epstein-Barr virus-infected cells are internalized via caveola-dependent endocytosis and promote phenotypic modulation in target cells. J Virol (2013) 87:10334–47. doi: 10.1128/JVI.01310-13
129. Uccini S, Al-Jadiry MF, Pepe G, Pasquini A, Alsaadawi AR, Al-Hadad SA, et al. Follicular dendritic cells display microvesicle-associated LMP1 in reactive germinal centers of EBV+ classic Hodgkin lymphoma. Virchows Arch (2019) 475:175–80. doi: 10.1007/s00428-019-02605-w
130. Zhang Z, Yu X, Zhou Z, Li B, Peng J, Wu X, et al. LMP1-positive extracellular vesicles promote radioresistance in nasopharyngeal carcinoma cells through P38 MAPK signaling. Cancer Med (2019) 8:6082–94. doi: 10.1002/cam4.2506
131. Nkosi D, Sun L, Duke LC, Patel N, Surapaneni SK, Singh M, et al. Epstein-Barr Virus LMP1 Promotes Syntenin-1- and Hrs-Induced Extracellular Vesicle Formation for Its Own Secretion To Increase Cell Proliferation and Migration. mBio (2020) 11:e00589-20. doi: 10.1128/mBio.00589-20
132. Gutzeit C, Nagy N, Gentile M, Lyberg K, Gumz J, Vallhov H, et al. Exosomes derived from Burkitt’s lymphoma cell lines induce proliferation, differentiation, and class-switch recombination in B cells. J Immunol (2014) 192:5852–62. doi: 10.4049/jimmunol.1302068
133. Hannigan A, Wilson JB. Evaluation of LMP1 of Epstein-Barr virus as a therapeutic target by its inhibition. Mol Cancer (2010) 9:184. doi: 10.1186/1476-4598-9-184
134. Dunmire SK, Hogquist KA, Balfour HH. Infectious Mononucleosis. Curr Top Microbiol Immunol (2015) 390:211–40. doi: 10.1007/978-3-319-22822-8_9
135. Marshall NA, Culligan DJ, Johnston PW, Millar C, Barker RN, Vickers MA. CD4(+) T-cell responses to Epstein-Barr virus (EBV) latent membrane protein 1 in infectious mononucleosis and EBV-associated non-Hodgkin lymphoma: Th1 in active disease but Tr1 in remission. Br J Haematol (2007) 139:81–9. doi: 10.1111/j.1365-2141.2007.06765.x
136. Kwok H, Chan KW, Chan KH, Chiang AK. Distribution, persistence and interchange of Epstein-Barr virus strains among PBMC, plasma and saliva of primary infection subjects. PLoS One (2015) 10:e0120710. doi: 10.1371/journal.pone.0120710
137. Nakayama T, Hieshima K, Nagakubo D, Sato E, Nakayama M, Kawa K, et al. Selective induction of Th2-attracting chemokines CCL17 and CCL22 in human B cells by latent membrane protein 1 of Epstein-Barr virus. J Virol (2004) 78:1665–74. doi: 10.1128/JVI.78.4.1665-1674.2004
138. Li ZX, Zeng S, Wu HX, Zhou Y. The risk of systemic lupus erythematosus associated with Epstein-Barr virus infection: a systematic review and meta-analysis. Clin Exp Med (2019) 19:23–36. doi: 10.1007/s10238-018-0535-0
139. Niller HH, Wolf H, Ay E, Minarovits J. Epigenetic dysregulation of epstein-barr virus latency and development of autoimmune disease. Adv Exp Med Biol (2011) 711:82–102. doi: 10.1007/978-1-4419-8216-2_7
140. Pan Q, Chen J, Guo L, Lu X, Liao S, Zhao C, et al. Mechanistic insights into environmental and genetic risk factors for systemic lupus erythematosus. Am J Transl Res (2019) 11:1241–54.
141. Asanuma Y, Chung CP, Oeser A, Shintani A, Stanley E, Raggi P, et al. Increased concentration of proatherogenic inflammatory cytokines in systemic lupus erythematosus: relationship to cardiovascular risk factors. J Rheumatol (2006) 33:539–45.
142. Hahn J, Leatherwood C, Malspeis S, Liu X, Lu B, Roberts AL, et al. Associations between Smoking and Systemic Lupus Erythematosus (SLE)-Related Cytokines and Chemokines among US Female Nurses. Arthritis Care Res (2020). doi: 10.1002/acr.24370
143. Freemer MM, . King, Jr TE, Criswell LA. Association of smoking with dsDNA autoantibody production in systemic lupus erythematosus. Ann Rheum Dis (2006) 65:581–4. doi: 10.1136/ard.2005.039438
144. Parisis D, Bernier C, Chasset F, Arnaud L. Impact of tobacco smoking upon disease risk, activity and therapeutic response in systemic lupus erythematosus: A systematic review and meta-analysis. Autoimmun Rev (2019) 18:102393. doi: 10.1016/j.autrev.2019.102393
145. Wolf SJ, Estadt SN, Theros J, Moore T, Ellis J, Liu J, et al. Ultraviolet light induces increased T cell activation in lupus-prone mice via type I IFN-dependent inhibition of T regulatory cells. J Autoimmun (2019) 103:102291. doi: 10.1016/j.jaut.2019.06.002
146. Pentony P, Duquenne L, Dutton K, Mankia K, Gul H, Vital E, et al. The initiation of autoimmunity at epithelial surfaces: a focus on rheumatoid arthritis and systemic lupus erythematosus. Discov Med (2017) 24:191–200.
147. Neubert E, Bach KM, Busse J, Bogeski I, Schon MP, Kruss S, et al. Blue and Long-Wave Ultraviolet Light Induce in vitro Neutrophil Extracellular Trap (NET) Formation. Front Immunol (2019) 10:2428. doi: 10.3389/fimmu.2019.02428
148. Johnson BM, Gaudreau MC, Gudi R, Brown R, Gilkeson G, Vasu C. Gut microbiota differently contributes to intestinal immune phenotype and systemic autoimmune progression in female and male lupus-prone mice. J Autoimmun (2020) 108:102420. doi: 10.1016/j.jaut.2020.102420
149. Ma Y, Xu X, Li M, Cai J, Wei Q, Niu H. Gut microbiota promote the inflammatory response in the pathogenesis of systemic lupus erythematosus. Mol Med (2019) 25:35. doi: 10.1186/s10020-019-0102-5
Keywords: autoimmunity, systemic lupus erythematosus, Epstein-Barr virus, molecular mimicry, functional mimicry, EBNA-1, LMP1, mouse
Citation: Munroe ME, Anderson JR, Gross TF, Stunz LL, Bishop GA and James JA (2021) Epstein-Barr Functional Mimicry: Pathogenicity of Oncogenic Latent Membrane Protein-1 in Systemic Lupus Erythematosus and Autoimmunity. Front. Immunol. 11:606936. doi: 10.3389/fimmu.2020.606936
Received: 16 September 2020; Accepted: 21 December 2020;
Published: 03 February 2021.
Edited by:
Amr Sawalha, University of Pittsburgh, United StatesReviewed by:
Gunnar Houen, Statens Serum Institut (SSI), DenmarkMarta E. Alarcon-Riquelme, Junta de Andalucía de Genómica e Investigación Oncológica (GENYO), Spain
Copyright © 2021 Munroe, Anderson, Gross, Stunz, Bishop and James. This is an open-access article distributed under the terms of the Creative Commons Attribution License (CC BY). The use, distribution or reproduction in other forums is permitted, provided the original author(s) and the copyright owner(s) are credited and that the original publication in this journal is cited, in accordance with accepted academic practice. No use, distribution or reproduction is permitted which does not comply with these terms.
*Correspondence: Melissa E. Munroe, bWVsaXNzYS1tdW5yb2VAb21yZi5vcmc=