- Department of Pathology and Molecular Medicine, McMaster Immunology Research Centre (MIRC), McMaster University, Hamilton ON, Canada
While type 2 immunity has been conventionally viewed as beneficial against helminths, venoms, and poisons, and harmful in allergy, contemporary research has uncovered its critical role in the maintenance of homeostasis. The initiation of a type 2 immune response involves an intricate crosstalk between structural and immune cells. Structural cells react to physical and chemical tissue perturbations by secreting alarmins, which signal the innate immune system to restore homeostasis. This pathway acts autonomously in the context of sterile injury and in the presence of foreign antigen initiates an adaptive Th2 response that is beneficial in the context of venoms, toxins, and helminths, but not food allergens. The investigation of the triggers and mechanisms underlying food allergic sensitization in humans is elusive because sensitization is a silent process. Therefore, the central construct driving food allergy modeling is based on introducing perturbations of tissue homeostasis along with an allergen which will result in an immunological and clinical phenotype that is consistent with that observed in humans. The collective evidence from multiple models has revealed the pre-eminent role of innate cells and molecules in the elicitation of allergic sensitization. We posit that, with the expanding use of technologies capable of producing formidable datasets, models of food allergy will continue to have an indispensable role to delineate mechanisms and establish causal relationships.
Introduction
Our understanding of the underlying pathogenesis of food allergy and its attendant applications has been largely attained through experimental modeling. In fact, modeling, which has been instrumental to scientific progress in general, is an inescapable approach to decipher complex problems such as a disease. Here, we discuss a broad perspective of type 2 immunity, highlighting its role in homeostasis. Then, we provide a succinct ideology of modeling and examine strategies for food allergy modeling. Lastly, we advance our view on why food allergy modeling will remain an indispensable discovery tool, especially with the continuing development of technologies that facilitate high parameter analyses.
Pathways of Innate-Driven Type 2 Immunity
Historically, type 2 immunity was conceived as a balancing system for type 1 inflammatory responses and whose primary purpose was to provide defense against helminths (1). Indeed, the symptomatology elicited by type 2 responses, including itching, tearing, intestinal cramps, wheezing, swelling, and, in extreme instances, anaphylaxis, is geared toward containment or expulsion of helminths, poisons, and toxins and are, therefore, beneficial for the host. Antithetically, type 2 immunity promotes harmful responses against food allergens.
Research over the last decade, largely in experimental models, has revolutionized this view ushering in a much richer perspective of both the functions and the engineering of type 2 immunity. With respect to the former, important roles for the cells and molecules of type 2 immunity have been uncovered in a wide range of processes, from tissue repair and metabolism to sensing temperature and chemical imbalances (2). Regarding the engineering, it is clear that the initiation and execution of a type 2 immune response entails an intricate crosstalk between structural and immune cells. Collectively, this research has positioned type 2 immunity as a guardian of homeostasis.
Considerable evidence from multiple contexts has revealed a generic type 2 pathway mediated by innate immune cells and molecules that regulates homeostasis. Structural cells at mucosal sites and the skin, prominently epithelial and endothelial cells, react to tissue perturbations or physiological changes by secreting alarmins, such as IL-33, IL-25, and TSLP (3, 4). These alarmins act on cognate receptors on ILC2s which expand and enact a variety of reparative functions primarily through the secretion of IL-5, IL-13, and amphiregulin (5–7). IL-5 facilitates the accumulation of eosinophils at the tissue site which generally impact homeostasis through their cytokines and granule products (8–12). ILC2s, IL-13, and amphiregulin induce a plethora of developmental and reparative functions. These include but are not limited to promotion of postnatal lung alveolarization (13, 14), stimulating epithelial repair and extracellular matrix production to repair wounds (15–19), regulating muscle cell metabolism and the clearance of necrotic debris during muscular injury and exercise (9, 20, 21), and regulating energy metabolism and the differentiation of beige adipose tissue to increase caloric expenditure and generate heat (22–26). To date, components of this pathway have been implicated in homeostatic maintenance in the skin (18, 19), muscles (9, 20), intestines (27, 28), nervous system (29, 30), liver (8, 31, 32), biliary tract (15), lungs (16), kidney (11), adipose tissue (33), among others. Emergent roles in the initiation and amplification of type 2 immunity are being described for other innate immune cells, such as mast cells and basophils, which produce alarmins and potently secrete other cytokines in some contexts (34, 35). Notably, virtually no adaptive immune involvement has been reported in type 2-mediated homeostasis and, in many cases, occurs in mice without an adaptive immune system.
Adaptive type 2 immunity is also activated in the context of significant tissue perturbations. The distinctive rule of engagement is the presence of foreign antigens in the tissue microenvironment. For example, the life cycle of many helminths involves perforating epithelial barriers and implanting into tissues. Most venoms are proteases which cleave proteins critical for tissue integrity and cause necrotic cell death. Some aeroallergens, like house dust mite, have inherent proteolytic activity (36). It follows that type 2 adaptive immunity may have evolved to respond to foreign antigens that are conspicuously present in the local microenvironment of tissue injury. In these alarmin-rich contexts, epithelial cell-derived cytokines directly act through cognate receptors on dendritic cells to condition the expression of the costimulatory molecule OX40L and downregulate IL-12 expression (37, 38). These dendritic cells also capture local antigen and migrate to secondary lymphoid organs where they engage naïve CD4+ T cells. OX40L is critical to induce IL-4 secretion from CD4+ T cells, which is thought to act in an autocrine manner to upregulate STAT6 and GATA3 resulting in Th2 differentiation (39–41). Some T follicular helper cells acquire a Th2-like phenotype required for the differentiation of IgE-secreting cells (42). Adaptive type 2 immunity is beneficial for the host in the aforementioned contexts; IgE is reported to provide resistance to venoms (43) and Th2 cells and IgE facilitate expeditious clearance of parasites (44–46).
We posit that the type 2 innate and adaptive responses are both instigated by the same triggers, perturbations of homeostasis, differing only by the presence or absence of antigen. When tissue homeostasis is disrupted, alarmins signal the innate immune system to initiate reparative functions to regain homeostasis Figure 1A). Simultaneously, some of these alarmins condition nearby dendritic cells, which are actively scanning the microenvironment for foreign antigens. Capture of antigens which colocalize with tissue damage are loaded into MHC II and elicit an adaptive type 2 immune response. If a foreign antigen is encountered that signals through pattern recognition receptors (like a bacteria or virus), type 1 immunity is engaged for pathogen control (Figure 1B). When no foreign antigens are encountered, DCs internalize and present only self-antigens which usually will not result in adaptive immunity as self-reactive T cells are eliminated or constrained by central and peripheral tolerance (Figure 1A). Type 2 immunity is intuitive for helminths, which cause damage when invading tissues and shed antigenic material that is internalized by DCs, thus mounting Th2 and IgE responses (Figure 1C) (45). It follows similarly for toxins and some aeroallergens, which cause damage through proteolytic activity and are small enough to be taken up by DCs (Figure 1D). However, it falls short when rationalizing immune responses against food allergens, which are highly diverse in structure, the vast majority of which are not immunogenic, and, enigmatically, which are essential for survival.
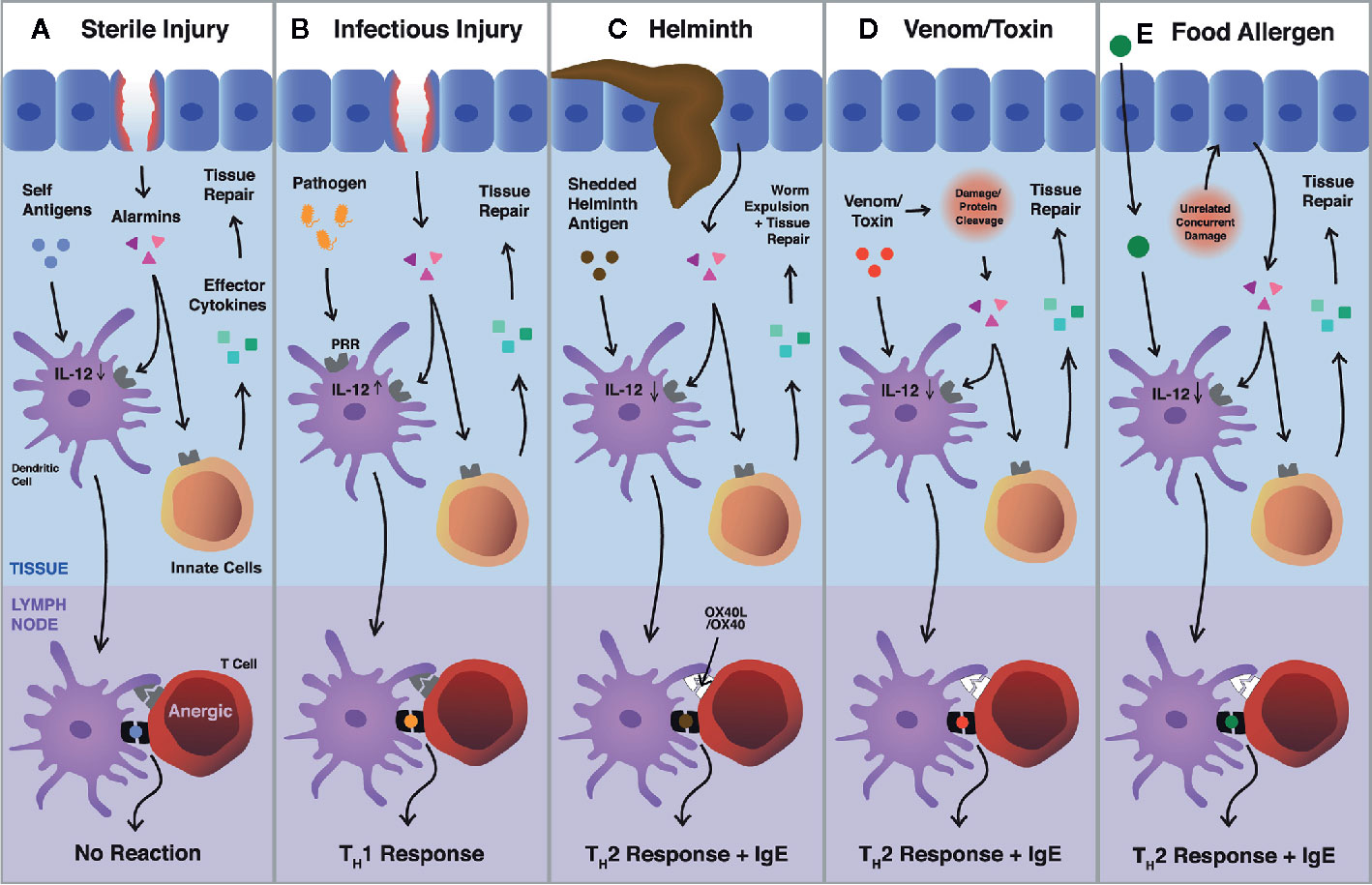
Figure 1 Pathways of Type 2 immune activation via tissue perturbations. (A–D) Epithelial cells release alarmins (e.g., IL-33, IL-25, and TSLP) in response to tissue damage. Alarmins signal through cognate receptors on innate immune cells, resulting in proliferation and the release of effector cytokines (e.g., IL-5, IL-13, and amphiregulin). These cytokines directly influence tissue repair (e.g., stimulating re-epithelization and extracellular matrix production) or cause the recruitment of other cells (e.g., eosinophils) which aid in tissue repair. Simultaneously, local dendritic cells uptake tissue antigens and respond to alarmin signaling by travelling to the draining lymph nodes and inducing Th2 cell differentiation through OX40-OX40L. (A) In the context of sterile injury, only self-antigens are uptaken by dendritic cells and are presented to T cells, which have been limited by central and peripheral tolerance. (B) When infectious agents are present, they signal through pattern recognition receptors, upregulating IL-12 in dendritic cells, and initiating Th1 immunity for host defence. (C, D) Helminths and venoms, canonical examples of Th2 responses, drive tissue damage by invading tissues or through cleaving proteins, respectively. Antigens that colocalize with damage are presented to T cells and initiate Th2 responses. (E) It remains unclear how Th2 immunity is initiated against food allergens. Mouse models require the use of adjuvants (e.g., cholera toxin or tape stripping) to perturb homeostasis, suggesting that food allergic responses are initiated in the presence of damage that is unrelated to the food allergen itself.
Ideology of Disease Modeling
Complexity has driven scientists from all branches to develop models, these understood as the functional simplification of an intractable reality. The development of a scientific model is based on the prevailing interpretation of the available information at a given time. Therefore, models are not static but evolve as we enhance our knowledge of a process. A biomedical model is a surrogate for a human being, or a human biological system. Its primary purpose is to understand how the human body works, from genotype to phenotype. Within biomedical models, animal models provide precise genetic and experimental control and are, thus, critical to establish causality. Understanding is not gained by observation but by manipulation, physically, genetically, pharmacologically or immunologically, in ways that would be unreasonable or even unethical to do in a human.
Animal models are critical for investigating disease mechanisms. Diseases are exceptionally intricate, rarely involving a single phenotypic abnormality and often affecting multiple organ systems. A case in point is the multiple phenotypes observed in allergic disease. This presents a conundrum: on the one hand, deciphering this complexity in a human being is an indomitable task; on the other, animal models are a simplification of this reality and can only provide approximations. Consequently, the expectation that findings from a single model may wholly explain a heterogeneous disease is unrealistic. The implication is that multiple models asking multiple questions are necessary to provide a comprehensive view of a disease process, food allergy in this case. Animal models are extensively used as well to interrogate the biological impact of novel therapeutics. These studies along with other experimental approaches are critical to narrow the decision of which therapeutics should progress to large scale studies in humans. In other words, knowing what not-to-do helps immensely with the decision of what-to-do.
Perspective on Food Allergy Modeling
Food allergy is typically diagnosed following a clinical reaction in infants or toddlers upon first known exposure (47). However, a prior event must have taken place to trigger allergic sensitization and, ultimately, production of food-specific IgE. As sensitization in humans is a silent process neither its initiation nor its evolution can be interrogated. Moreover, there appears to be no unifying genetic or environmental exposures, highlighting the heterogenous nature of food allergy. Accordingly, a diversity of animal models—primarily mouse models—have provided a tangible, ethical, and indispensable approach to decipher the cellular and molecular mechanisms underlying allergic sensitization.
Unlike some aeroallergens, toxins, and animal poisons, the vast majority of food antigens are not inherently immunogenic. As a consequence, most humans and experimental animals develop oral tolerance, an active state of non-reactivity, upon first exposure to food antigens (48, 49). In fact, the LEAP study demonstrated that over 98% of infants with atopic comorbidities (eczema and/or egg allergy) receiving their first exposure to peanut before 11 months of age develop oral tolerance (50). Similar to a type 2 homeostatic response, the tolerogenic response to food antigens [reviewed in citations (51, 52)] fundamentally involves DCs. However, acquisition of oral tolerance imperatively lacks the key molecules and the majority of cells that trigger Th2 polarization. It follows that a concomitant subversion of homeostasis is required to prime the immune system to mount a Th2-dominant response against food antigens (Figure 1E). In mouse modeling, this subversion is generally achieved through the use of adjuvants—substances which aid in eliciting robust immune responses (53). Cholera toxin (CT), staphylococcal enterotoxin B (SEB), and aluminum hydroxide (alum), are three of the most widely used biological or chemical adjuvants that facilitate a Th2-dominant response in mice (54–58). Damaged skin, due to physical disruption of epidermal barriers (i.e., epicutaneous sensitization), drives sensitization to topically applied allergen (59–63). A second approach to modeling food allergies, also a subversion of homeostasis, involves reducing the threshold of reactivity via genetic mutations. For example, Il4raF709 mice which contain a loss-of-function mutation in the Il4ra immunotyrosine inhibitory motif, display a lower sensitization threshold upon food allergen exposure (64, 65). Thus, the use of adjuvanted models has played a key role in overcoming the elusiveness of allergic sensitization in humans.
Basic discoveries that discriminate tolerant (homeostatic) versus pathogenic (allergic) responses to foods have been described using models in which mice are sensitized with a single or repeated administration of CT along with a food antigen (56, 66–68). CT is classified as an AB5 toxin; this class of toxins are produced by many enterotropic bacteria, such as Escherichia coli and Bordetella pertussis (69). Oral administration of CT enhances CD103+ CD11c+ DC migration to the mesenteric lymph nodes as well as expression of MHC II and costimulatory molecules (e.g., CD86) (70–72). These effects per se do not rationalize the elicitation of dominant Th2 priming. However, studies investigating the gene expression profile of mesenteric lymph node DCs in CT-immunized mice showed an upregulation of OX40L (70). Antibody-mediated blockade and genetic knockouts demonstrated a critical role of OX40L to promote Th2 skewing by enabling the initial burst of IL-4 from CD4 T cells (28, 41, 70). Further studies exposed the involvement of the IL-33/ST2 innate axis, where IL-33-signaling facilitates OX40L upregulation (41). Intriguingly, use of an ILC2 depletion strategy revealed a dispensable role of ILC2s in oral sensitization to foods, despite a dominant presence in intestinal tissues and a well-defined role in type 2 homeostatic processes (41).
A relationship between skin atopic abnormalities and sensitization to food allergens has become clear through large-scale longitudinal studies (73, 74). A primary example of this are loss-of-function mutations in the filaggrin gene (Flg) that correlate with peanut allergy in humans (75). To elucidate the immunological mechanisms of this relationship, models of epicutaneous sensitization have been employed. Sensitization is typically achieved through tape stripping, which removes the outermost epidermal layer, causing skin inflammation (63). These models have been referred to as “adjuvant-free”, which is misleading as tape stripping provides the same function as an adjuvant—induction of an immune response via an external input (76–78). Animal models have revealed an essential role of epithelial cells pertaining to barrier function and alarmin production during epicutaneous allergen exposure. In mice, disturbed production of filaggrin, a protein involved in epidermal barrier function, facilitated allergic sensitization to allergen applied topically (79). Damaged epithelial cells are an important source of alarmins such as IL-33, TSLP, and uric acid which, as reviewed earlier, enable DC activation and subsequent CD4 T cell priming (80–81). Tape-strip models have also revealed a role of ILC2s which, in response to IL-25 and IL-33 secretion from tape-stripped skin, proliferate in intestinal tissues to drive IL-4-dependent mast cell expansion (82).
In addition to elucidating the innate immunological events underlying allergic sensitization, animal modeling has enabled discoveries pertaining to allergic reactions. Alarmins involved in sensitization also participate in the effector phase. IL-33 interacts directly with mast cells to potentiate mast cell degranulation (84). Moreover, FcεRI-mediated mast cell activation stimulates production of IL-25, which is hypothesized to further drive the Th2 phenotype (85). Mouse models have elucidated the vasoactive mediators that facilitate anaphylaxis. In humans a correlation between anaphylactic severity and serum platelet activating factor (PAF) levels, but not histamine levels, was reported (86). Use of mouse models determined that blockade of histamine receptors (H1 and H2) was insufficient at preventing anaphylaxis, but that concurrent treatment with antihistamines and a PAF-receptor antagonist could significantly reduce anaphylactic severity (87).
In mouse models, allergen challenges vary greatly in dose and route (intraperitoneal, oral, intradermal, and intravenous) (56, 58, 88, 89). Routes of challenge differ in the clinical phenotype that is induced. For example, oral challenges tend to result in diarrhea and, sometimes, a mild drop in core body temperature. Intraperitoneal challenges, on the other hand, facilitate a much more robust drop in core body temperature and other severe systemic reactions (e.g., seizures). The severity of clinical reactivity may, in part, be dependent on antigen availability and mast cell density at the site of allergen administration (90). As well, IgG has also been shown to mediate anaphylaxis in food allergy mouse models, although an equivalent IgG-mediated food-induced anaphylactic pathway in humans remains contentious (63, 91, 92). In all instances—independent of allergen administration route—modeling allergen challenge reveals downstream functions of allergen-specific immunoglobulins (e.g., the events following allergen cross-linking of mast cell-bound IgE). Thus, the selection of the route of allergen challenge should be guided by the research question, where intraperitoneal and intravenous challenges are best suited to assess systemic anaphylaxis, oral challenge to evaluate gut-local effects (e.g., allergic diarrhea, goblet cell hyperplasia), and intradermal challenge to measure localized vascular permeability. Use of anti-FcγRII/III blocking antibodies may also be employed to further discriminate between IgE- and IgG-mediated allergen reactivity (91).
The use of animal models of food allergy for translational research has been scrutinized due to the necessity of “artificial/experimental sensitization” (i.e., requiring eccentric interventions). The validity of animal models for translational research can, however, be evaluated by three criteria: 1) face validity, 2) predictive validity, and 3) target validity (93, 94). Face validity evaluates the similarity of disease biology and symptomology between humans and the animal model (93). The biological processes of food allergy at large are conserved between mice and humans. For example, the immune response is dominantly Th2 polarized, IL-33 and uric acid are elevated compared to non-allergic controls, and clinical reactivity is IgE-mediated (84, 92, 95). Many of the clinical signs of an allergic reaction are also alike including itching, diarrhea, local inflammation, and systemic shock (76, 96). Predictive validity compares the effectiveness of interventions in humans and in the relevant animal model (93). Due to the small number of interventions tried in human food allergy, evaluation of predictive validity is limited, but oral immunotherapy provides a key example. Oral immunotherapy in both mice and humans can increase the threshold of reactivity (i.e., desensitization) to food allergens, although this desensitized state is, just as it is in the majority of humans, unsustained (68, 97). This limited assessment of predictive validity will be greatly improved come the initiation of clinical trials testing novel biotherapeutics (e.g., anti-IL-4Rα). Lastly, target validity establishes that a particular target (e.g., a cytokine) has the same function in humans and the disease model (93). Many of the molecules and cells involved in human food allergy provide similar actions in animal models. For instance, IL-4 promotes Th2 polarization and IgE class-switch in both mice and human food allergy (95). In contrast, the role of IgG in human food-induced anaphylaxis remains contentious and, therefore, mouse models would provide low target validity for investigation of IgG-targeted interventions. In summary, despite not knowing the “adjuvants” at play in human food allergy, adjuvant-based experimental models have revealed cellular and molecular signatures that exist in humans. Moreover, regardless of experimental model, it is clear that an innate program drives allergic sensitization.
Concluding Remarks
Over the last few decades, there have been fundamental advances in our understanding of allergic disease, including food allergy. These advances have led to a dramatic re-conceptualization of the functions of type 2 immunity and the critical role of the innate system in programming these functions. We must recognize, however, that the current state of knowledge is vastly incomplete. For example, how gut dysbiosis stresses local tissue environments and impacts primary and secondary immune responses to foods is an emerging area already providing novel insights (98, 99). We surmise that experimental models will continue providing seminal discoveries on the machinery (cells and molecules) and the engineering (operating mechanisms) that underlie allergy. Indeed, the ability to overexpress or repress individual genes in animals, either permanently or on-demand, pervasively or in specific cell types, unlocks unique opportunities to decipher both the pathogenesis and the trajectory of the allergic diathesis. Lastly, experimental modeling remains an unparalleled sieve to guide what should be investigated in humans.
The ever-increasing application of technologies that deliver formidable datasets will help to delineate complex inter- and intracellular pathways, thus enlightening the astounding phenotypic diversity and plasticity of the allergic response. Mass cytometry has dramatically expanded upon flow cytometry in the analysis of protein expression at the single cell level and is drastically reshaping histology allowing for the measurement of very many markers on a single section. Bulk and single cell RNA sequencing is rapidly expanding in read depth, resulting in greater appreciation of the genetic signatures and subpopulations that define disease states. Constant advances in bioinformatics and machine learning continue to unveil new ways of mining these massive datasets for pathways and cell differentiation trajectories. There is no doubt that the next generation of large data analysis is already being conceived and developed beyond the gaze of immunologists. The logic of applying these analyses to human samples is clear. However, applying these technologies to animal research will remain imperative in at least two areas that are not currently possible in humans: 1) To address questions that require manipulation (genotype and exposure), not only observation, to understand how the system reacts and 2) To efficiently deconvolute the temporal and spatial evolution of a given perturbation or process; particularly those that are obscured by the complex exposure history of humans. Furthermore, emerging discoveries from these datasets in both humans and models will have to be validated through mechanistic studies in experimental models using the long-established immunological toolkit to decipher causal relationships.
Author Contributions
KB, JK, AP, and MJ wrote and edited the manuscript. All authors contributed to the article and approved the submitted version.
Funding
This work was supported by funds from Food Allergy Canada, Walter and Maria Schroeder Foundation, Michael Zych Family, and Canadian Asthma, Allergy, and Immunology Foundation (CAAIF). KB holds a Canada Graduate Scholarship. AP holds The Eva Eugenia Lillian Cope Scholarship.
Conflict of Interest
The authors declare that the research was conducted in the absence of any commercial or financial relationships that could be construed as a potential conflict of interest.
Acknowledgments
We kindly thank C. Spadafora for her illustrations.
References
1. Mosmann TR, Coffman RL. TH1 and TH2 Cells: Different Patterns of Lymphokine Secretion Lead to Different Functional Properties. Annu Rev Immunol (1989) 7:145–73. doi: 10.1146/annurev.iy.07.040189.001045
2. Lloyd CM, Snelgrove RJ. Type 2 immunity: Expanding our view. Sci Immunol (2018) 3:1604. doi: 10.1126/sciimmunol.aat1604
3. Molofsky AB, Savage AK, Locksley RM. Interleukin-33 in Tissue Homeostasis, Injury, and Inflammation. Immunity (2015) 42:1005–19. doi: 10.1016/j.immuni.2015.06.006
4. Yang D, Han Z, Oppenheim JJ. Alarmins and immunity. Immunol Rev (2017) 280:41–56. doi: 10.1111/imr.12577
5. Neill DR, Wong SH, Bellosi A, Flynn RJ, Daly M, Langford TKA, et al. Nuocytes represent a new innate effector leukocyte that mediates type-2 immunity. Nature (2010) 464:1367–70. doi: 10.1038/nature08900
6. Moro K, Yamada T, Tanabe M, Takeuchi T, Ikawa T, Kawamoto H, et al. Innate production of TH 2 cytokines by adipose tissue-associated c-Kit+ Sca-1+ lymphoid cells. Nature (2010) 463:540–4. doi: 10.1038/nature08636
7. Price AE, Liang HE, Sullivan BM, Reinhardt RL, Eisley CJ, Erle DJ, et al. Systemically dispersed innate IL-13-expressing cells in type 2 immunity. Proc Natl Acad Sci U S A (2010) 107:11489–94. doi: 10.1073/pnas.1003988107
8. Goh YPS, Henderson NC, Heredia JE, Eagle AR, Odegaard JI, Lehwald N, et al. Eosinophils secrete IL-4 to facilitate liver regeneration. Proc Natl Acad Sci U S A (2013) 110:9914–9. doi: 10.1073/pnas.1304046110
9. Heredia JE, Mukundan L, Chen FM, Mueller AA, Deo RC, Locksley RM, et al. Type 2 innate signals stimulate fibro/adipogenic progenitors to facilitate muscle regeneration. Cell (2013) 153:376–88. doi: 10.1016/j.cell.2013.02.053
10. Nussbaum JC, Van Dyken SJ, Von Moltke J, Cheng LE, Mohapatra A, Molofsky AB, et al. Type 2 innate lymphoid cells control eosinophil homeostasis. Nature (2013) 502:245–8. doi: 10.1038/nature12526
11. Riedel JH, Becker M, Kopp K, Düster M, Brix SR, Meyer-Schwesinger C, et al. IL-33-Mediated expansion of type 2 innate lymphoid cells protects from progressive glomerulosclerosis. J Am Soc Nephrol (2017) 28:2068–80. doi: 10.1681/ASN.2016080877
12. Weller PF, Spencer LA. Functions of tissue-resident eosinophils. Nat Rev Immunol (2017) 17:746–60. doi: 10.1038/nri.2017.95
13. Loering S, Cameron GJM, Starkey MR, Hansbro PM. Lung development and emerging roles for type 2 immunity. J Pathol (2019) 247:686–96. doi: 10.1002/path.5211
14. de Kleer IM, Kool M, de Bruijn MJW, Willart M, van Moorleghem J, Schuijs MJ, et al. Perinatal Activation of the Interleukin-33 Pathway Promotes Type 2 Immunity in the Developing Lung. Immunity (2016) 45:1285–98. doi: 10.1016/j.immuni.2016.10.031
15. Li J, Razumilava N, Gores GJ, Walters S, Mizuochi T, Mourya R, et al. Biliary repair and carcinogenesis are mediated by IL-33-dependent cholangiocyte proliferation. J Clin Invest (2014) 124:3241–51. doi: 10.1172/JCI73742
16. Monticelli LA, Sonnenberg GF, Abt MC, Alenghat T, Ziegler CGK, Doering TA, et al. Innate lymphoid cells promote lung-tissue homeostasis after infection with influenza virus. Nat Immunol (2011) 12:1045–54. doi: 10.1038/ni.2131
17. Yin H, Li X, Hu S, Liu T, Yuan B, Gu H, et al. IL-33 accelerates cutaneous wound healing involved in upregulation of alternatively activated macrophages. Mol Immunol (2013) 56:347–53. doi: 10.1016/j.molimm.2013.05.225
18. Rankin AL, Mumm JB, Murphy E, Turner S, Yu N, McClanahan TK, et al. IL-33 Induces IL-13–Dependent Cutaneous Fibrosis. J Immunol (2010) 184:1526–35. doi: 10.4049/jimmunol.0903306
19. Rak GD, Osborne LC, Siracusa MC, Kim BS, Wang K, Bayat A, et al. IL-33-Dependent Group 2 Innate Lymphoid Cells Promote Cutaneous Wound Healing. J Invest Dermatol (2016) 136:487–96. doi: 10.1038/JID.2015.406
20. Knudsen NH, Stanya KJ, Hyde AL, Chalom MM, Alexander RK, Liou YH, et al. Interleukin-13 drives metabolic conditioning of muscle to endurance exercise. Sci (80- ) (2020) 368:eaat3987. doi: 10.1126/science.aat3987
21. Kastenschmidt J. A role for group 2 innate lymphoid cells in muscular dystrophy. J Immunol (2019) 202:1350. doi: 10.3390/ijms21041350
22. Hams E, Locksley RM, McKenzie ANJ, Fallon PG. Cutting Edge: IL-25 Elicits Innate Lymphoid Type 2 and Type II NKT Cells That Regulate Obesity in Mice. J Immunol (2013) 191:5349–53. doi: 10.4049/jimmunol.1301176
23. Stanya KJ, Jacobi D, Liu S, Bhargava P, Dai L, Gangl MR, et al. Direct control of hepatic glucose production by interleukin-13 in mice. J Clin Invest (2013) 123:261–71. doi: 10.1172/JCI64941
24. Brestoff JR, Kim BS, Saenz SA, Stine RR, Monticelli LA, Sonnenberg GF, et al. Group 2 innate lymphoid cells promote beiging of white adipose tissue and limit obesity. Nature (2015) 519:242–6. doi: 10.1038/nature14115
25. Lee MW, Odegaard JI, Mukundan L, Qiu Y, Molofsky AB, Nussbaum JC, et al. Activated type 2 innate lymphoid cells regulate beige fat biogenesis. Cell (2015) 160:74–87. doi: 10.1016/j.cell.2014.12.011
26. Qiu Y, Nguyen KD, Odegaard JI, Cui X, Tian X, Locksley RM, et al. Eosinophils and type 2 cytokine signaling in macrophages orchestrate development of functional beige fat. Cell (2014) 157:1292–308. doi: 10.1016/j.cell.2014.03.066
27. Waddell A, Vallance JE, Moore PD, Hummel AT, Wu D, Shanmukhappa SK, et al. IL-33 Signaling Protects from Murine Oxazolone Colitis by Supporting Intestinal Epithelial Function. Inflammation Bowel Dis (2015) 21:2737–46. doi: 10.1097/MIB.0000000000000532
28. Monticelli LA, Osborne LC, Noti M, Tran SV, Zaiss DMW, Artis D. IL-33 promotes an innate immune pathway of intestinal tissue protection dependent on amphiregulin-EGFR interactions. Proc Natl Acad Sci U S A (2015) 112:10762–7. doi: 10.1073/pnas.1509070112
29. Gadani SP, Smirnov I, Smith AT, Overall CC, Kipnis J. Characterization of meningeal type 2 innate lymphocytes and their response to CNS injury. J Exp Med (2017) 214:285–96. doi: 10.1084/jem.20161982
30. Gadani SP, Walsh JT, Smirnov I, Zheng J, Kipnis J. The Glia-Derived Alarmin IL-33 Orchestrates the Immune Response and Promotes Recovery following CNS Injury. Neuron (2015) 85:703–9. doi: 10.1016/j.neuron.2015.01.013
31. Steinmann S, Schoedsack M, Heinrich F, Breda PC, Ochel A, Tiegs G, et al. Hepatic ILC2 activity is regulated by liver inflammation-induced cytokines and effector CD4+ T cells. Sci Rep (2020) 10:1–13. doi: 10.1038/s41598-020-57985-w
32. Gieseck RL, Ramalingam TR, Hart KM, Vannella KM, Cantu DA, Lu WY, et al. Interleukin-13 Activates Distinct Cellular Pathways Leading to Ductular Reaction, Steatosis, and Fibrosis. Immunity (2016) 45:145–58. doi: 10.1016/j.immuni.2016.06.009
33. Bénézech C, Jackson-Jones LH. ILC2 Orchestration of Local Immune Function in Adipose Tissue. Front Immunol (2019) 10:171. doi: 10.3389/fimmu.2019.00171
34. Sokol CL, Medzhitov R. Emerging functions of basophils in protective and allergic immune responses. Mucosal Immunol (2010) 3:129–37. doi: 10.1038/mi.2009.137
35. Krystel-Whittemore M, Dileepan KN, Wood JG. Mast cell: A multi-functional master cell. Front Immunol (2016) 6:1–12. doi: 10.3389/fimmu.2015.00620
36. Chevigné A, Jacquet A. Emerging roles of the protease allergen Der p 1 in house dust mite–induced airway inflammation. J Allergy Clin Immunol (2018) 142:398–400. doi: 10.1016/j.jaci.2018.05.027
37. Hussaarts L, Yazdanbakhsh M, Guigas B. Priming Dendritic Cells for Th2 Polarization: Lessons Learned from Helminths and Implications for Metabolic Disorders. Front Immunol (2014) 5:499. doi: 10.3389/fimmu.2014.00499
38. Chu DK, Llop-Guevara A, Walker TD, Flader K, Goncharova S, Boudreau JE, et al. IL-33, but not thymic stromal lymphopoietin or IL-25, is central to mite and peanut allergic sensitization. J Allergy Clin Immunol (2013) 131:187–200.e1–8. doi: 10.1016/j.jaci.2012.08.002
39. Liu Z, Liu Q, Hamed H, Anthony RM, Foster A, Finkelman FD, et al. IL-2 and Autocrine IL-4 Drive the In Vivo Development of Antigen-Specific Th2 T Cells Elicited by Nematode Parasites. J Immunol (2005) 174:2242–9. doi: 10.4049/jimmunol.174.4.2242
40. Noben-Trauth N, Hu-Li J, Paul WE. IL-4 secreted from individual naive CD4+ T cells acts in an autocrine manner to induce Th2 differentiation. Eur J Immunol (2002) 32:1428–33. doi: 10.1002/1521-4141(200205)32:5<1428::AID-IMMU1428>3.0.CO;2-0
41. Chu DK, Mohammed-Ali Z, Jiménez-Saiz R, Walker TD, Goncharova S, Llop-Guevara A, et al. T helper cell IL-4 drives intestinal Th2 priming to oral peanut antigen, under the control of OX40L and independent of innate-like lymphocytes. Mucosal Immunol (2014) 7:1395–404. doi: 10.1038/mi.2014.29
42. Gowthaman U, Chen JS, Zhang B, Flynn WF, Lu Y, Song W, et al. Identification of a T follicular helper cell subset that drives anaphylactic IgE. Sci (80- ) (2019) 365:eaaw6433. doi: 10.1126/science.aaw6433
43. Marichal T, Starkl P, Reber LL, Kalesnikoff J, Oettgen HC, Tsai M, et al. A Beneficial Role for Immunoglobulin E in Host Defense against Honeybee Venom. Immunity (2013) 39:963–75. doi: 10.1016/j.immuni.2013.10.005
44. Gurish MF, Bryce PJ, Tao H, Kisselgof AB, Thornton EM, Miller HR, et al. IgE Enhances Parasite Clearance and Regulates Mast Cell Responses in Mice Infected with Trichinella spiralis. J Immunol (2004) 172:1139–45. doi: 10.4049/jimmunol.172.2.1139
45. White RR, Artavanis-Tsakonas K. How helminths use excretory secretory fractions to modulate dendritic cells. Virulence (2012) 3:668–77. doi: 10.4161/viru.22832
46. King CL, Xianli J, Malhotra I, Liu S, Mahmoud AA, Oettgen HC. Mice with a targeted deletion of the IgE gene have increased worm burdens and reduced granulomatous inflammation following primary infection with Schistosoma mansoni. J Immunol (1997) 158:294–300.
47. Savage J, Johns CB. Food allergy: epidemiology and natural history. Immunol Allergy Clin North Am (2015) 35:45–59. doi: 10.1016/j.iac.2014.09.004
48. Husby S, Mestecky J, Moldoveanu Z, Holland S, Elson CO. Oral tolerance in humans. T cell but not B cell tolerance after antigen feeding. J Immunol (1994) 152:4663–70.
49. Friedman A, Weiner HL. Induction of anergy or active suppression following oral tolerance is determined by antigen dosage. Proc Natl Acad Sci U S A (1994) 91:6688–92. doi: 10.1073/pnas.91.14.6688
50. Du Toit G, Roberts G, Sayre PH, Bahnson HT, Radulovic S, Santos AF, et al. Randomized Trial of Peanut Consumption in Infants at Risk for Peanut Allergy. N Engl J Med (2015) 372:803–13. doi: 10.1056/NEJMoa1414850
51. Pabst O, Mowat AM. Oral tolerance to food protein. Mucosal Immunol (2012) 5:232–9. doi: 10.1038/mi.2012.4
52. Satitsuksanoa P, Jansen K, Głobińska A, van de Veen W, Akdis M. Regulatory Immune Mechanisms in Tolerance to Food Allergy. Front Immunol (2018) 9:2939. doi: 10.3389/fimmu.2018.02939
53. Awate S, Babiuk LA, Mutwiri G. Mechanisms of action of adjuvants. Front Immunol (2013) 4:114:114. doi: 10.3389/fimmu.2013.00114
54. Snider DP, Marshall JS, Perdue MH, Liang H. Production of IgE antibody and allergic sensitization of intestinal and peripheral tissues after oral immunization with protein Ag and cholera toxin. J Immunol (1994) 153:647–57.
55. Marinaro M, Staats HF, Hiroi T, Jackson RJ, Coste M, Boyaka PN, et al. Mucosal adjuvant effect of cholera toxin in mice results from induction of T helper 2 (Th2) cells and IL-4. J Immunol (1995) 155:4621–9.
56. Sun J, Arias K, Alvarez D, Fattouh R, Walker T, Goncharova S, et al. Impact of CD40 Ligand, B Cells, and Mast Cells in Peanut-Induced Anaphylactic Responses. J Immunol (2007) 179:6696–703. doi: 10.4049/jimmunol.179.10.6696
57. Ganeshan K, Neilsen CV, Hadsaitong A, Schleimer RP, Luo X, Bryce PJ. Impairing oral tolerance promotes allergy and anaphylaxis: A new murine food allergy model. J Allergy Clin Immunol (2009) 123:231. doi: 10.1016/J.JACI.2008.10.011
58. Brandt EB, Strait RT, Hershko D, Wang Q, Muntel EE, Scribner TA, et al. Mast cells are required for experimental oral allergen–induced diarrhea. J Clin Invest (2003) 112:1666. doi: 10.1172/JCI19785
59. Wang LF, Lin JY, Hsieh KH, Lin RH. Epicutaneous exposure of protein antigen induces a predominant Th2-like response with high IgE production in mice. J Immunol (1996) 156:4077–82.
60. Hsieh K-Y, Tsai C-C, Herbert Wu CH, Lin R-H. Epicutaneous Exposure to Protein Antigen and Food Allergy. Clin Exp Allergy (2003) 33:1067–75. doi: 10.1046/J.1365-2222.2003.01724.X
61. Tordesillas L, Goswami R, Benedé S, Grishina G, Dunkin D, Järvinen KM, et al. Skin exposure promotes a Th2-dependent sensitization to peanut allergens. J Clin Invest (2014) 124:4965. doi: 10.1172/JCI75660
62. Noti M, Kim BS, Siracusa MC, Rak GD, Kubo M, Moghaddam AE, et al. Exposure to food allergens through inflamed skin promotes intestinal food allergy through the thymic stromal lymphopoietin-basophil axis. J Allergy Clin Immunol (2014) 133:1390–9, 1399.e1–6. doi: 10.1016/j.jaci.2014.01.021
63. Jiménez-Saiz R, Ellenbogen Y, Koenig J, Gordon ME, Walker TD, Rosace D, et al. IgG1 + B cell immunity predates IgE responses in epicutaneous sensitization to foods. Allergy (2018) 74:165–75. doi: 10.1111/all.13481
64. Tachdjian R, Al Khatib S, Schwinglshackl A, Kim HS, Chen A, Blasioli J, et al. In vivo regulation of the allergic response by the IL-4 receptor alpha chain immunoreceptor tyrosine-based inhibitory motif. J Allergy Clin Immunol (2010) 125:1128–36. doi: 10.1016/j.jaci.2010.01.054
65. Burton OT, Noval Rivas M, Zhou JS, Logsdon SL, Darling AR, Koleoglou KJ, et al. Immunoglobulin E signal inhibition during allergen ingestion leads to reversal of established food allergy and induction of regulatory T cells. Immunity (2014) 41:141–51. doi: 10.1016/J.IMMUNI.2014.05.017
66. Zhang B, Liu E, Gertie JA, Joseph J, Xu L, Pinker EY, et al. Divergent T follicular helper cell requirement for IgA and IgE production to peanut during allergic sensitization. Sci Immunol (2020) 5:eaay2754. doi: 10.1126/sciimmunol.aay2754
67. Li XM, Serebrisky D, Lee SY, Huang CK, Bardina L, Schofield BH, et al. A murine model of peanut anaphylaxis: T- and B-cell responses to a major peanut allergen mimic human responses. J Allergy Clin Immunol (2000) 106:150–8. doi: 10.1067/mai.2000.107395
68. Leonard SA, Martos G, Wang W, Nowak-Węgrzyn A, Berin MC. Oral immunotherapy induces local protective mechanisms in the gastrointestinal mucosa. J Allergy Clin Immunol (2012) 129:1579–87.e1. doi: 10.1016/j.jaci.2012.04.009
69. Beddoe T, Paton AW, Le Nours J, Rossjohn J, Paton JC. Structure, biological functions and applications of the AB5 toxins. Trends Biochem Sci (2010) 35:411–8. doi: 10.1016/j.tibs.2010.02.003
70. Blázquez AB, Berin MC, Viney JL. Gastrointestinal dendritic cells promote Th2 skewing via OX40L. J Immunol (2008) 180:4441–50. doi: 10.4049/jimmunol.180.7.4441
71. Fahlén-Yrlid L, Gustafsson T, Westlund J, Holmberg A, Strömbeck A, Blomquist M, et al. CD11c(high )dendritic cells are essential for activation of CD4+ T cells and generation of specific antibodies following mucosal immunization. J Immunol (2009) 183:5032–41. doi: 10.4049/jimmunol.0803992
72. Anjuère F, Luci C, Lebens M, Rousseau D, Hervouet C, Milon G, et al. In vivo adjuvant-induced mobilization and maturation of gut dendritic cells after oral administration of cholera toxin. J Immunol (2004) 173:5103–11. doi: 10.4049/jimmunol.173.8.5103
73. Lack G, Fox D, Northstone K, Golding J. Factors Associated with the Development of Peanut Allergy in Childhood. N Engl J Med (2003) 348:977–85. doi: 10.1056/NEJMoa013536
74. Hill DJ, Hosking CS. Food allergy and atopic dermatitis in infancy: an epidemiologic study. Pediatr Allergy Immunol (2004) 15:421–7. doi: 10.1111/j.1399-3038.2004.00178.x
75. Brown SJ, Asai Y, Cordell HJ, Campbell LE, Zhao Y, Liao H, et al. Loss-of-function variants in the filaggrin gene are a significant risk factor for peanut allergy. J Allergy Clin Immunol (2011) 127:661–7. doi: 10.1016/j.jaci.2011.01.031
76. Berin MC, Mayer L. Immunophysiology of experimental food allergy. Mucosal Immunol (2009) 2:24–32. doi: 10.1038/mi.2008.72
77. Kanagaratham C, Sallis BF, Fiebiger E. Experimental Models for Studying Food Allergy. Cell Mol Gastroenterol Hepatol (2018) 6:356–69. doi: 10.1016/J.JCMGH.2018.05.010
78. Tordesillas L, Mondoulet L, Blazquez A, Benhamou P, Sampson HA, Berin MC. Epicutaneous Immunotherapy Induces Gastrointestinal LAP + Regulatory T Cells and Prevents Food-Induced Anaphylaxis. J Allergy Clin Immunol (2017) 139:189–201. doi: 10.1016/J.JACI.2016.03.057
79. Fallon PG, Sasaki T, Sandilands A, Campbell LE, Saunders SP, Mangan NE, et al. A homozygous frameshift mutation in the murine filaggrin gene facilitates enhanced percutaneous allergen priming. Nat Genet (2009) 41:602. doi: 10.1038/NG.358
80. Bruhs A, Proksch E, Schwarz T, Schwarz A. Disruption of the Epidermal Barrier Induces Regulatory T Cells via IL-33 in Mice. J Invest Dermatol (2018) 138:570–9. doi: 10.1016/J.JID.2017.09.032
81. Oyoshi MK, Larson RP, Ziegler SF, Geha RS. Mechanical injury polarizes skin dendritic cells to elicit a Th2 response by inducing cutaneous TSLP expression. J Allergy Clin Immunol (2010) 126:976. doi: 10.1016/J.JACI.2010.08.041
82. Kong J, Chalcraft K, Mandur TS, Jimenez-Saiz R, Walker TD, Goncharova S, et al. Comprehensive metabolomics identifies the alarmin uric acid as a critical signal for the induction of peanut allergy. Allergy (2015) 70:495–505. doi: 10.1111/all.12579
83. Leyva-Castillo J-M, Galand C, Kam C, Burton O, Gurish M, Musser MA, et al. Mechanical Skin Injury Promotes Food Anaphylaxis by Driving Intestinal Mast Cell Expansion. Immunity (2019) 50:1262–75.e4. doi: 10.1016/J.IMMUNI.2019.03.023
84. Galand C, Leyva-Castillo JM, Yoon J, Han A, Lee MS, McKenzie ANJ, et al. IL-33 promotes food anaphylaxis in epicutaneously sensitized mice by targeting mast cells. J Allergy Clin Immunol (2016) 138:1356–66. doi: 10.1016/j.jaci.2016.03.056
85. Ikeda K, Nakajima H, Suzuki K, Kagami S, Hirose K, Suto A, et al. Mast cells produce interleukin-25 upon Fc epsilon RI-mediated activation. Blood (2003) 101:3594–6. doi: 10.1182/blood-2002-09-2817
86. Vadas P, Gold M, Perelman B, Liss GM, Lack G, Blyth T, et al. Platelet-Activating Factor, PAF Acetylhydrolase, and Severe Anaphylaxis. N Engl J Med (2008) 358:28–35. doi: 10.1056/NEJMoa070030
87. Arias K, Baig M, Colangelo M, Chu D, Walker T, Goncharova S, et al. Concurrent blockade of platelet-activating factor and histamine prevents life-threatening peanut-induced anaphylactic reactions. J Allergy Clin Immunol (2009) 124:307–14, 314.e1–2. doi: 10.1016/j.jaci.2009.03.012
88. He J-S, Subramaniam S, Narang V, Srinivasan K, Saunders SP, Carbajo D, et al. IgG1 memory B cells keep the memory of IgE responses. Nat Commun (2017) 8:641. doi: 10.1038/s41467-017-00723-0
89. Oettgen HC, Martin TR, Wynshaw-Boris A, Deng C, Drazen JM, Leder P. Active anaphylaxis in IgE-deficient mice. Nature (1994) 370:367–70. doi: 10.1038/370367a0
90. Hogan SP, Wang YH, Strait R, Finkelman FD. Food-induced anaphylaxis: Mast cells as modulators of anaphylactic severity. Semin Immunopathol (2012) 34:643–53. doi: 10.1007/s00281-012-0320-1
91. Arias K, Chu DK, Flader K, Botelho F, Walker TD, Arias N, et al. Distinct immune effector pathways contribute to the full expression of peanut-induced anaphylactic reactions in mice. J Allergy Clin Immunol (2011) 127:1552–61.e1. doi: 10.1016/j.jaci.2011.03.044
92. Finkelman FD, Simons FER, Frew AJ, Ansotegui IJ, Bochner BS, Golden DBK, et al. Anaphylaxis: lessons from mouse models. J Allergy Clin Immunol (2007) 120:506–25. doi: 10.1016/j.jaci.2007.07.033
93. Denayer T, Stöhr T, Van Roy M. Animal models in translational medicine: Validation and prediction. New Horizons Transl Med (2014) 2:5–11. doi: 10.1016/J.NHTM.2014.08.001
94. Ferreira GS, Veening-Griffioen DH, Boon WPC, Moors EHM, Gispen-de Wied CC, Schellekens H, et al. A standardised framework to identify optimal animal models for efficacy assessment in drug development. PloS One (2019) 14:e0218014. doi: 10.1371/journal.pone.0218014
95. Bruton K, Spill P, Vohra S, Baribeau O, Manzoor S, Gadkar S, et al. Interrupting reactivation of immunological memory reprograms allergy and averts anaphylaxis. bioRxiv (2020). doi: 10.1101/2020.03.04.975706. 2020.03.04.975706.
96. Tordesillas L, Berin MC, Sampson HA. Immunology of Food Allergy. Immunity (2017) 47:32–50. doi: 10.1016/j.immuni.2017.07.004
97. Chinthrajah RS, Purington N, Andorf S, Long A, O’Laughlin KL, Lyu SC, et al. Sustained outcomes in oral immunotherapy for peanut allergy (POISED study): a large, randomised, double-blind, placebo-controlled, phase 2 study. Lancet (London England) (2019) 394:1437–49. doi: 10.1016/S0140-6736(19)31793-3
98. Plunkett CH, Nagler CR. The Influence of the Microbiome on Allergic Sensitization to Food. J Immunol (2017) 198:581–9. doi: 10.4049/jimmunol.1601266
Keywords: food allergy, animal modeling, type 2 immunity, Th2, homeostasis
Citation: Bruton K, Koenig JFE, Phelps A and Jordana M (2020) Perturbations to Homeostasis in Experimental Models Revealed Innate Pathways Driving Food Allergy. Front. Immunol. 11:603272. doi: 10.3389/fimmu.2020.603272
Received: 06 September 2020; Accepted: 10 November 2020;
Published: 10 December 2020.
Edited by:
Simon Patrick Hogan, University of Michigan, United StatesReviewed by:
Hans Oettgen, Boston Children's Hospital and Harvard Medical School, United StatesMark C. Siracusa, Rutgers Biomedical and Health Sciences, United States
Copyright © 2020 Bruton, Koenig, Phelps and Jordana. This is an open-access article distributed under the terms of the Creative Commons Attribution License (CC BY). The use, distribution or reproduction in other forums is permitted, provided the original author(s) and the copyright owner(s) are credited and that the original publication in this journal is cited, in accordance with accepted academic practice. No use, distribution or reproduction is permitted which does not comply with these terms.
*Correspondence: Manel Jordana, am9yZGFuYW1AbWNtYXN0ZXIuY2E=