- Department of Oral Biology, College of Dentistry, University of Florida, Gainesville, FL, United States
There is mounting evidence that members of the human microbiome are highly associated with a wide variety of cancer types. Among oral cancers, oral squamous cell carcinoma (OSCC) is the most prevalent and most commonly studied, and it is the most common malignancy of the head and neck worldwide. However, there is a void regarding the role that the oral microbiome may play in OSCC. Previous studies have not consistently found a characteristic oral microbiome composition associated with OSCC. Although a direct causality has not been proven, individual members of the oral microbiome are capable of promoting various tumorigenic functions related to cancer development. Two prominent oral pathogens, Porphyromonas gingivalis, and Fusobacterium nucleatum can promote tumor progression in mice. P. gingivalis infection has been associated with oro-digestive cancer, increased oral cancer invasion, and proliferation of oral cancer stem cells. The microbiome can influence the evolution of the disease by directly interacting with the human body and significantly altering the response and toxicity to various forms of cancer therapy. Recent studies have shown an association of certain phylogenetic groups with the immunotherapy treatment outcomes of certain tumors. On the other side of the coin, recently it has been a resurgence in interest on the potential use of bacteria to cure cancer. These kinds of treatments were used in the late nineteenth and early twentieth centuries as the first line of defense against cancer in some hospitals but later displaced by other types of treatments such as radiotherapy. Currently, organisms such as Salmonella typhimurium and Clostridium spp. have been used for targeted strategies as potential vectors to treat cancer. In this review, we briefly summarize our current knowledge of the role of the oral microbiome, focusing on its bacterial fraction, in cancer in general and in OSCC more precisely, and a brief description of the potential use of bacteria to target tumors.
The Human Microbiome and Cancer. An Overview
About 30 trillion bacterial cells are living in or on every human. That is around one bacterium for each cell in the human body (1). These microorganisms are on the whole known as the microbiome. Since the completion of the Human Microbiome Project (2), we have witnessed an increased interest in the role that the human microbiome plays in human health, many studies have linked changes in microbial communities to systemic conditions such as allergies, diabetes, inflammatory bowel disease, and atherosclerosis (3–7). Among the systemic conditions influenced by the microbiome, cancer has not been an exception. We have learned that chronic infections contribute to carcinogenesis, with approximately 13% of the global cancer burden being directly attributable to infectious agents (8).
Many viruses promote cancer through well-described genetic mechanisms. Around 10–15% of human cancers worldwide are caused by seven human viruses, which include Epstein-Bar Virus (EBV), Hepatitis B Virus (HBV), Human T-lymphotropic virus-I (HTLV-I), Human papillomaviruses (HPV), Hepatitis C virus (HCV), Kaposi’s sarcoma herpesvirus (KSHV) and Merkel cell polyomavirus (MCV) (9). However, the first evidence that bacteria were directly involved in cancer development did not come until the 1980s with the work of Marshall and Warren (10). When they presented their results, entrenched was the belief that lifestyle caused ulcers that it was difficult for them to convince the scientific world of Helicobacter pylori’s role in gastric cancer (Figure 1). To provide even more conclusive evidence, in 1985, Marshall deliberately infected himself with the bacterium and established his stomach illness. Since then, it has been firmly proven by many researchers worldwide that H. pylori cause more than 90% of duodenal ulcers and up to 80% of gastric ulcers, and has been classified as a class I carcinogen by the World Health Organization due to its ability to promote stomach cancer after chronic infection (11–13). Disease-promoting and cancer-promoting effects of pathogens often depend on virulence factors. In H. pylori, strains expressing the virulence factors cytotoxin associated gene A (CagA) or vacuolating cytotoxin A (VacA), exemplify the role of virulence factors by increasing inflammation, and cancer rates (14).
An emerging concept in cancer biology implicates the microbiome as an influential environmental factor modulating the carcinogenic process. The idea that inflammation promotes carcinogenesis was first postulated more than 150 years ago by the German pathologist Virchow (15). The link between chronic inflammation and cancer is now well established (16–18). This association has recently experienced a renewed interest with the recognition that members of the human microbiome can be responsible for the chronic inflammation observed in a wide variety of cancers (19). Increasing evidence shows the association of changes in the human microbiome with certain types of cancer (20–22). Studies in germ-free animals have revealed evidence for tumor-promoting effects of the microbiome in spontaneous, genetically-induced, and carcinogen-induced cancers in various organs (23).
There is strong epidemiological evidence that other bacterial species are associated with cancer development, most likely induced by creating a pro-inflammatory micro-environment (24) or suppressing the immune response (25). Among the species of bacteria that have been directly linked to the development of cancer is Salmonella enterica subsp. enterica sv. Typhi (S. Typhi) and gallbladder cancer (26–28), Streptococcus bovis and colon cancer (29–31), and Chlamydia pneumoniae with lung cancer (32–36). The most persuasive epidemiological evidence of bacterial oncogenic potential, aside from H. pylori, concerns S. Typhi. However, the evidence for S. bovis and C. pneumoniae and Fusobacterium nucleatum is less conclusive, a meta-analysis on the association of those organisms with increased risk of cancer have shown either different or weak associations (33, 37–42).
Although gallbladder carcinoma (GBC) is rare in western countries, there is a high incidence in countries with endemic S. Typhi infections such as South America and parts of Africa and Asia, particularly India and Pakistan (43). The first epidemiological association was found by Welton et al. in 1979. In that paper, they analyzed 471 deceased typhoid carriers, registered by the New York City Health Department between 1922 and 1975, and matched with 942 controls for sex, age at death, year of death, the borough the carrier died, and where they were born. The results show that chronic typhoid carriers die of hepatobiliary cancer six times more often than the matched controls (44). Two more recent meta-analyses confirmed these initial results. In the first study by Koshiol et al., they performed a case-control and a meta-analysis of more than 1,000 GBC cases, and in both cases, they found a positive association between S. Typhi and GBC (45). In the second meta-analysis by Nagaraja and Eslick, they selected 17 studies for their analysis, most of them from India and China. The highest incidence of gallbladder cancer (GBC) occurs in India, contributing to about 10% of the global GBC cases (46). When Nagaraja and Eslick performed a subgroup analysis according to region, they found a significant association between S. Typhi carrier state and carcinoma of the gallbladder based on detection methods of S. Typhi antibody levels and culture (47). A possible mechanism has been proposed that explains the link between gallbladder carcinoma and infection by S. Typhi. In predispose mice, organoids and tissue culture with mutations in TP53 (tumor suppressor gene), and enhanced c-MYC (oncogenes) expression translocated bacterial effector molecules SopE, SopE2, SopB (Salmonella outer proteins), and SptP (Salmonella protein tyrosine phosphatase), from the Salmonella pathogenicity island 2 (SPI-2), activate the protein kinase B (Akt), or MAPK inhibitors, prevented mouse embryonic fibroblast transformation (48).
The previous examples refer to the link between specific organisms and carcinogenesis; however, microbes that trigger transformation events in host cells are rare. It has been demonstrated that in some cases, the tumorigenic process is not the result of the activities of a specific organism but rather the result of an instability in the composition of the bacterial communities or dysbiosis, often associated with inflammatory disorders such as colitis or periodontal disease. In mouse models, it has been shown that a dysbiotic community can lead to the development of colorectal cancer (49, 50).
The shift from a eubiotic community, with low cancer risk, to a dysbiotic community, with increased cancer risk, is the result of changes in environmental conditions and associated with metabolic responses in the host that modulate the progression of cancer (51). Dysbiosis of the oral microbiome could influence cancer outcomes by different mechanisms. Two common mechanisms that could have severe implications in the development of the disease are chronic inflammation and the synthesis of metabolites that could induce mutations (52).
The Oral Microbiome and Cancer
There were reports of a correlation between periodontitis and leukemia as early as the late 1940s early 1950s (53, 54). Since those pioneer studies, there is mounting evidence of the correlation between periodontal disease and various cancers. Several meta-analyses have confirmed the suspicion that periodontal disease should be considered as a risk factor in several types of cancers. In fact in a meta-analysis by Corbella et al., they found that a statistically significant association was found for all cancers studied, both combined and individually (digestive tract, pancreatic, prostate, breast, corpus uteri, lung, hematological, esophageal/oropharyngeal and Non-Hodgkin lymphoma) (55). Never smokers population with periodontal disease has a higher risk of developing hematopoietic and lymphatic cancers (55, 56). Pancreatic, lung, and colorectal cancers also show a positive correlation with periodontal disease (55, 57–60). In the case of breast cancer, the evidence shows a more modest positive association between periodontal disease and breast cancer (61). Finally, the most expected correlation would be with oral cancers, and indeed there is a clear positive correlation between periodontal disease and oral cancers (55, 59, 60). Edentulism has been positively correlated with pancreatic cancer (58) but not within colorectal cancer (62). Interestingly, in the study where authors did not find associations of edentulism and colorectal cancer (CRC), they also found no correlation between edentulism and periodontitis (62). One of these meta-analyses looked at not only a correlation between periodontal disease and cancer but also correlations with particular organisms (60). The results of that meta-analysis indicated that periodontal bacterial infection increased cancer incidence and was associated with poor overall survival, disease-free survival, and cancer-specific survival. Subgroup analysis indicated that the risk of cancer was associated with Porphyromonas gingivalis and Prevotella intermedia infection but not Tannerella forsythia, Treponema denticola, Aggregatibacter actinomycetemcomitans, and F. nucleatum infection (60).
In the case of pancreatic cancer, a potential mechanism has been proposed (57). The innate immune response to pathogenic bacteria that results in inflammation also has been linked with pancreatic carcinogenesis. Lipopolysaccharide, a component in the outer membrane of Gram-negative bacteria, such as, P. gingivalis, triggers an innate immune response that involves recognition by Toll-like receptor 4 (TLR4), which stimulates both myeloid differentiation primary response 88 (MyD88) dependent and MyD88-independent pathways that then activate the nuclear factor κB pathway and results in the release of pro-inflammatory cytokines. Interestingly, TLRs seem to have a role in pancreatic cancer development, and TLR4 is explicitly highly expressed in human pancreatic cancer but not a healthy pancreas. In the murine models, the TLR4/MyD88 pathway can trigger protection from pancreatic cancer development or acting to promote inflammation and pancreatic cancer development (63). LPS was shown to drive pancreatic carcinogenesis, as was the blockade of the MyD88-dependent pathway (via a dendritic cell-mediated deviation to TH2), whereas blockade of TLR4 (via TRIF, TIR-domain-containing adapter-inducing interferon-β) and blockade of the MyD88 independent (via TRIF) were protective against pancreatic cancer (63). Although more empirical evidence is needed, similar mechanisms implicating inflammatory response of the innate immune system may be implicated in other types of cancer.
Oral Microorganisms and Cancers Outside the Oral Cavity
One characteristic of the oral microbiome that distinguishes from other body sites is that many oral microorganisms that are considered commensals in the oral cavity are commonly associated with various cancers in distant organs (Table 1). The associations of oral organisms and distant cancers occur in two categories. The first appears in certain types of cancer where no oral microbes are directly involved in the tumor’s pathogenesis, but there is a consistent change in the composition of the oral microbiome associated with cancer, thus with the potential use of those changes as biomarkers of cancer (71, 86). The second kind of association is the association of organisms involved in tumorigenesis, such as F. nucleatum association with CRC (88, 89, 100).
Dysbiosis of the oral microbiome has been associated with a wide variety of cancers (Table 1). There are three types of associations between microbiome and disease that have been described as potential biomarkers in cancer: increase or decrease in numbers of individual organisms, use of models of several organisms as predictors and assessing their performance, and finally changes in diversity indexes that give an overall picture of the behavior of the community.
As examples of specific organisms as biomarkers, an increase in F. nucleatum has been linked to CRC (88), and P. gingivalis (69) and Fusobacterium spp. (70) have been linked to pancreatic cancer. Additional examples of links of specific bacteria and oral organisms are presented in Table 1. Another approach in the search for biomarkers of cancer is the use of complex models of several organisms that maximize the area under the Receiver Operating Characteristics (ROC) curve or Area Under Curve (AUC). ROC shows how well a model can discriminate or separate the cases and controls, and AUC has a value between 0.50 and 1.0, indicating no discrimination and perfect discrimination, respectively (101). Thus, and AUC of 0.9 would indicate that the probability of discriminating a case vs. control is 90%. In Table 1, we show three examples of combined taxons to distinguish controls vs. cancer samples: a three taxon model of Lautropia, Streptococcus and an unspecified genus of the order Bacteroidales, with AUC =0.94, has been associated with esophageal cancer (66), a two taxon model, Streptococcus mitis and Neisseria elongata with an AUC of 0.90, associated with pancreatic cancer (71) and a bacterial cluster associated with gastric cancer with AUC of 0.76 (96). Finally, another approach to the use of biomarkers in differentiating case vs. control samples is using changes in biodiversity indicators as a proxy of changes in the microbiome that could be linked to the status of the sample, independently of what organisms are present or absent. We have also presented examples of this approach in Table 1. For instance, Chen et al. observed an overall decrease of microbial diversity in the saliva of esophageal cancer patients (67), while an overall increase in diversity in saliva and plaque from gastric cancer patients was observed by Sun et al. (95).
Except for CRC, where Fusobacterium spp. are ubiquitous, in general, there is little overlapping in the biomarkers identified in the different studies (Table 1), probably due to the different type of samples and methods of analysis used to identify the composition of the community.
Pancreatic cancer has been one of the most widely studied types of cancer in seeking association between changes in the oral microbiome and disease (68–76). Although the communities vary depending on the study, Porphyromonas and Fusobacterium genera were associated with cancer in most studies (Table 1). Likewise, in the case of lung cancer, the genus Veillonella appeared associated with the majority of studies (Table 1).
CRC has been an exception in that Fusobacterium spp. have been consistently associated with this type of cancer, both as a biomarker in the oral cavity (91) and more importantly, in stool and biopsies from tumors samples (21, 84, 87–90, 92, 93), indicating a possible direct effect on the progression of the disease. A more direct indication of the clinical relevance of F. nucleatum in CRC has been presented in a recent paper where the authors show that in mice with colorectal tumors, oral or intravenous administration of dextran nanoparticles covalently linked to azide-modified phages that inhibit the growth of F. nucleatum significantly augments the efficiency of first-line chemotherapy treatments of CRC (102). For all these reasons, most focus has been placed on studying the role of F. nucleatum in CRC. Using arbitrarily primed PCR (AP-PCR) to identify isolates at the strain level, Komiya et al. demonstrated that the strains present in CRC samples were identical to strains isolated from the saliva of CRC patients, supporting the oral origin of F. nucleatum in the intestine of CRC patients (103).
In 2012 Kostic et al., using genomic analysis, identified Fusobacterium sequences as enriched in colon carcinomas; they did not prove a causal relationship between Fusobacterium and colorectal cancer was the first indication of its potential importance in CRC (21). One year later, Kostic et al., using the mouse model of intestinal tumorigenesis Apc(Min/+), showed that F. nucleatum was capable of increasing tumor multiplicity and selectively recruit tumor-infiltrating myeloid cells, which can promote tumor progression. Tumors from Apc(Min/+) mice exposed to F. nucleatum exhibit a pro-inflammatory expression signature shared with human fusobacteria-positive colorectal carcinomas. F. nucleatum generates a pro-inflammatory microenvironment conducive to colorectal neoplasia progression by recruiting tumor-infiltrating immune cells (87). This same year Rubinstein et al. proposed a possible mechanism by which F. nucleatum induces tumorigenesis (104). F. nucleatum encodes several adhesins for interspecies interactions, but so far, only one, FadA (adhesion protein FadA), has been identified as binding to host cells (105). FadA is not only an adhesin but also an invasin required for binding and invasion of both healthy and cancerous host cells and binds to cell-junction molecules, the cadherins (105). In Rubinstein et al. model, FadA binds to E-cadherin activating β-catenin signaling (104). The activation of β-catenin signaling in colorectal cancer is mediated via a TLR4/P-PAK1 (p21-activated kinases) cascade (106). Loss of E-cadherin-mediated-adhesion characterizes benign lesions’ transition to invasive, metastatic cancer (107, 108).
In a more recent article, Rubinstein et al. expanded their model to include Annexin A1, a previously unrecognized modulator of Wnt/β-catenin signaling, which is a crucial component through which F. nucleatum exerts its stimulatory effect. Annexin A1 is expressed explicitly in proliferating colorectal cancer cells and involved in the activation of Cyclin D1 (109). Over-expression of cyclin D1 has been linked to the development and progression of cancer (110). Based on their results, Rubinstein et al. proposed a “two-hit” model to explain how F. nucleatum acts in CRC. Somatic mutations cause the first “hit”, and the second “hit” is caused by F. nucleatum, exacerbating cancer progression on those cells that suffered the initial mutations (109).
The Microbiome and Oral Cancer
Head and neck cancer was the seventh most common cancer worldwide in 2018 (890,000 new cases and 450,000 deaths) (111). Oral squamous cell carcinomas (OSCC), the most frequent malignancies in the oral cavity, represented 2% of all cancers worldwide (354,864 cases and 177,384 deaths) (111). In the United States alone, it is expected that a total of 52,260 new cases of oral and pharynx cancer will occur in the year 2020 (112). The financial cost of treating oral and oropharyngeal cancer may be the highest of all cancers in the United States (113). Additionally, an essential factor in OSCC mortality is the high level of recurrence after treatment. Several studies, with many cases, have shown that the overall recurrence rate was approximately 30% (114–116). Recurrence rate in OSCC is high. In the first 36 months, the recurrence rates range from 70 to 92% of cases (114, 117–119). Interestingly the observed 5-year survival after recurrence varies depending on the moment in which recurrence occurs. If recurrence occurs within the 18 months after treatment, the rate of survival ranges between 20.5 to 27.55%, but if recurrence occurs afterward, the rate of survival increases to 38.1% to 42.3% (114, 120).
Despite advances in our knowledge of the causes and risk factors associated with OSCC, survival rates for oral cancers, including OSCC have not improved substantially in the last forty years, emphasizing that new means of early detection and treatment are urgently needed.
Viruses have long been associated with the risk of developing OSCC. The etiological role of human papillomavirus (HPV) in OSCC has been widely researched for more than three decades. Several meta-analyses have demonstrated that infection by HPV increments the risk of OSCC by up to 3-fold (121, 122). The prevalence of HPV among OSCC patients is around 25% (121, 123, 124); however, there is a geographical component with the highest HPV prevalence in Africa and Asia, notably among Chinese studies from provinces with high OSCC incidence rates (123). Other viruses have been identified in OSCC samples, as individual infections or in co-infection with HPV. Still, their role in the disease is unclear (124–127). Despite its importance, HPV-unrelated OSCC cases account for the vast majority of oral cancer cases. Other environmental factors should play an essential role in OSCC development and progression; among them, the microbiome’s role has just begun to be considered a risk factor.
As mentioned previously, periodontitis has been linked to various types of cancers, including esophagus/oropharyngeal cancers. Table 2 presents some of the studies that linked bacteria’s presence to cancer of the oral cavity and pharynx. Several studies have found that the risk of developing OSCC may increase with periodontal disease (55, 142, 143), signaling a possible role of inflammation caused by the microbiome with oral cancer. Periodontitis is a typical example of an infectious disease causing chronic inflammation in the oral cavity (144, 145). Thus several systematic reviews of the literature showed that periodontal disease increases the risk of oral cancer even after adjusting for significant risk factors (146, 147).
Moreover, expression of pro-inflammatory cytokines in periodontal disease such as IL-1 and TNF-α has been linked to microbial triggered carcinogenesis (19). In a study comparing the microbiome of gingival squamous cell carcinoma (GSCC) with periodontitis microbiome, members of the genera Fusobacterium, Peptostreptococcus, and Prevotella were more abundant in cancerous, periodontal tissues. In contrast, saliva or soft mucosa harbored more periodontal health-related bacteria (148).
Most studies on the role of the human microbiome on cancer have focused on describing microbial communities present in specific samples or the immunological response of the host to the bacterial challenge. The oral microbiome has been proposed as a diagnostic indicator of oral cancer; however, as in other types of cancer, the search for possible biomarkers of oral cancer and, most specifically, OSCC has not produced conclusive results (52). Different organisms have been shown to increase in OSCC samples, as a few examples: Capnocytophaga gingivalis, Prevotella melaninogenica, and S. mitis (131); F. nucleatum (149); Pseudomonas aeruginosa (150); Campylobacter concisus, Prevotella salivae, Prevotella loeschii, and Fusobacterium oral taxon 204 (151); genera Fusobacterium, Dialister, Peptostreptococcus, Filifactor, Peptococcus, Catonella, and Parvimonas (136); and Prevotella oris, Neisseria flava, Neisseria flavescens/subflava, F. nucleatum ss polymorphum, Aggregatibacter segnis, and Fusobacterium periodonticum (152).
Yang et al. studied the progression of the microbiome during cancer’s progression from the early to the late stage and found a significant increase of Fusobacteria. At the species level, they found that F. periodonticum, Parvimonas micra, Streptococcus constellatus, Haemophilus influenza, and Filifactor alocis were associated with OSCC, and they progressively increased in abundance from stage 1 to 4 (80).
Direct evidence of the role of the microbiome in OSCC was presented by Stashenko et al. were using a germ-free mouse model a 4-nitroquinoline-1 oxide (4NQO)-induced carcinogenesis the authors observed a significant increase on the number of tumors and their size when the mice were inoculated with two different microbiomes vs. inoculated controls. The microbiomes used came from the tongue of a healthy mouse and the other from the tumor lesion of a diseased mouse, and Pasteurella was the dominant genus in both groups (153).
The carcinogenic potential of periodontal pathogens has also been described in several studies. P. gingivalis, one of the essential periodontal pathogens, increase the invasiveness of oral cancer cells and resistance to chemotherapeutic agents (154–156). Using a 4NQO-induced mouse model of oral cancer, the inoculation of P. gingivalis promoted tumor progression by invading precancerous lesions and recruiting the myeloid-derived suppressor cells by expressing chemokines such as C-C motif ligand 2 (CCL2) and chemokine (C-X-C motif) ligand 2 (CXCL2), and cytokines such as IL-6 and IL-8 (157). P. gingivalis also induces expression of the ZEB1 transcription factor, which controls the epithelial-mesenchymal transition. Interestingly, the up-regulation of ZEB1 appears to be controlled by FimA, a major virulence factor involved in adhesion and cellular invasion (158). The infection of P. gingivalis increases the expression of mesenchymal markers, including vimentin and matrix metalloproteinase MMP-9 (132, 158, 159).
Utilizing a murine model of periodontitis-associated oral tumorigenesis, Binder Gallimidi et al. showed that chronic bacterial co-infection of P. gingivalis and F. nucleatum promotes OSCC via direct interaction with oral epithelial cells through Toll-like receptors, with an increase in expression of TLR2 in OSCC cells and IL-6 in both cells and the mouse model (160). A detailed review of all the studies where P. gingivalis has been associated with the development of OSCC has been recently published by Lafuente Ibáñez de Mendoza et al. (161).
F. nucleatum in esophageal cancer tissues has been associated with shorter survival, suggesting a prognostic biomarker’s potential role. F. nucleatum might also contribute to aggressive tumor behavior through the activation of chemokines, such as chemokine (C-C motif) ligand 20 (CCL20) (162). Epithelial-mesenchymal transition (EMT) is the process by which epithelial cells acquire a mesenchymal-like phenotype. It has been proposed that EMT is responsible for compromising epithelial barrier function in the pathogenesis of several diseases, including OSCC. F. nucleatum infection of oral cell lines triggers EMT (163, 164). F. nucleatum triggers EMT via lncRNA/MIR4435-2HG/miR-296-5p/Akt2/SNAI1 signaling pathway and up-regulates mesenchymal markers, including N-cadherin, Vimentin, and snail family transcription repressor 1 (SNAI1) (164). The fact that infection by periodontal pathogens generates EMT features introduces the possibility that this process may be involved in the loss of epithelial integrity during periodontitis and may promote predisposition to malignant transformation through the EMT.
The metatranscriptome has recently begun to be used to analyze community-wide gene expression in the human microbiome (165–168). Measuring bacterial gene expression in the wild has been challenging. The half-life of mRNA is short, and mRNA in bacteria and archaea usually comprises only a small fraction of total RNA. Therefore large samples are needed to study expression, but obtaining such samples is not always possible. Additionally, working with non-model organisms presents the challenge of lacking most of the bioinformatic tools readily available for model organisms.
Using a metatranscriptome analysis of OSCC samples, Yost et al. found that Fusobacteria showed a statistically significantly higher number of transcripts at tumor sites, indicating a higher activity of this group of organisms in cancer. Moreover, when looking at tumor signatures of the oral microbiome, metabolic activities such as iron ion transport, tryptophanase activity, peptidase activities, and superoxide dismutase were over-represented in tumor samples when compared to the healthy controls (169).
Effect of the Microbiome in the Outcomes of Cancer Therapy
Despite its clinical importance, the human microbiome’s effect on malignancy treatment is merely starting to be investigated. While organisms assume significant activities in keeping up human well-being, they are likewise engaged with the turn of events that lead to tumor development. There is now proof indicating that the microbiome can impact patient reactions to cancer treatment. The microbiome has been implicated in modulating cancer therapy’s efficacy and toxicity, including chemotherapy and immunotherapy (170, 171). Moreover, preclinical data suggest that the microbiome modulation could become a novel strategy for improving the efficacy of immune-based therapies for cancer (172).
The gut microbiota has the potential to affect the ability of cancer therapy. The microbiota, when affected by dysbiosis, can profoundly influence both cancer pathogenesis and its therapeutic outcome. In particular, the regulation of such a therapeutic outcome is firmly linked with the gut microbiota’s capacity to process anti-cancer compounds and modify the host’s immune response. These two effects combined may clarify the patient’s microbiome composition’s substantial participation in affecting the efficiency of both immunotherapy and chemotherapy.
The oral microbiome’s role in the outcome of cancer treatments has not yet been evaluated. Only in two studies, oral organisms were identified as influencing the outcome of immunotherapies. The first of these studies showed that F. nucleatum, along with Bacteroides fragilis and Escherichia coli improved the survival of adoptive cell therapy (ACT) treated patients, probably by increasing cytokine production and T cell infiltration (173). The second study found that Lactobacillus fermentum attenuated the immune response in patients treated with CpG-oligonucleotides, which are short synthetic single-stranded DNA molecules containing unmethylated CpG dinucleotides, acting as agonists of Toll-like receptor 9 (TLR9), and leading to strong immunostimulatory effects (174).
Effects On Cancer Chemotherapy
A recent survey of in situ bacterial effects on frequently used chemotherapeutics suggests the profound influence of distinct bacteria species on the anti-tumor effect. Heshiki et al. examined the influence of the intestinal microbiome on treatment effects (175) in a heterogeneous cohort that comprised eight diverse malignancy types to recognize organisms with a collective effect on the immune response. It is revealed through human gut metagenomic examination that responder patients had inherently higher microbial diversity arrangements than non-responders. Moreover, by assessing the gut microbiome’s job without precedent for a heterogeneous patient cohort with different kinds of malignant growth and anti-cancer medicines, Heshiki et al. found a worldwide microbiome signature that is autonomous of disease type and heterogeneity (175). Explicit species, Bacteroides xylanisolvens, and Bacteroides ovatus were decidedly connected with treatment results. Oral gavage of these responder microbes fundamentally expanded the adequacy of chemotherapy (erlotinib) and actuated the declaration of CXCL9 and IFN-γ in a murine lung cancer model. Also, oral gavage of explicit gut microbiome substantially expanded the impact of chemotherapy in mice, decreasing the tumor volume by 46% contrasted with the control.
This information recommends an anticipated effect of the microbiome’s explicit constituents on tumor development and disease treatment results with suggestions for both visualization and treatment. Curiously, the microbiome can bolster the resistant framework in the battle against malignancy. For instance, cyclophosphamide (a medication used to treat leukemia and lymphomas) was found to impact the organisms living in the gut. These gut organisms reacted by advancing the production of resistant cells, which appears to improve cyclophosphamide adequacy (176). Among patients with hematologic malignancies, specific bacterial taxa are connected with the effectiveness of allogeneic hematopoietic stem cell transplantation (allo-HSCT) and reduced hazard for graft-versus-host disease (GVHD) succeeding treatment (177, 178). Besides, holding expanded measures of microorganisms having a place with the variety of Blautia was related to diminished GVHD lethality in this cohort and was affirmed in another independent cohort of 51 patients from a similar organization (177).
Effects On Cancer Radiotherapy
Ionizing radiation therapy (RTX) was received by cancer patients that are genotoxic for tumor cells and might be curative for restricted cancers. The great worldview in radiation biology accepted that the cellular nucleus was the main objective of radiation and DNA harm was incited by direct testimony of vitality or creation of reactive oxygen species (ROS) through a radiation-induced separation of intracellular water molecules. Ionizing radiation, however, additionally incites non-targeted impact on non-irradiated cells, such as genomic unpredictability, systemic radio-adaptive retorts, inflammatory and immune reactivity, and bystander effect on nearby cells. Observer and foundational impacts are auxiliary to DNA harm and are intervened by the interruption of gap intersection proteins engaged with cell-cell interactions and by the arrival of extracellular intermediaries, together with cytokines, exosomes, ROS and nitric oxide (NO). Along these lines, comparably to the tissue harm related to contamination by microorganisms, radiations initiate the arrival of damage-associated molecular pattern (DAMP) pressure signals (179). The impacts of radiation are perplexing. It initiates both immunostimulant and immunosuppressive reactions and might be deficient in enacting a defensive anti-cancer invulnerable reaction (180). It very well may be speculated that the gut microbiome likewise satisfies a job in the immunostimulatory impacts of RTX. The viewed fluctuations in microbiome arrangement at epithelial surfaces in patients and mice rewarded with RTX have been proposed to contribute to the pathogenesis of bone marrow failure, colitis, looseness of the bowels, oral mucositis and enteritis (181). RTX persuades apoptosis in the breach of the intestinal barrier, intestinal crypts, and modifications in the microbiome conformation (182).
Studies have shown that the intestinal microbiome has a significant effect on total body irradiation. Fewer endothelial cells of the intestinal mucosa are derived through irradiation into apoptosis and prompt less lymphocyte invasion in germ-free mice than in conventional mice (183). This finding shows that gut commensals can assume a harmful role in protecting the enteric harmfulness of total body irradiation (TBI) in germ-free mice.
Effects On Cancer Immunotherapy
Ongoing examinations have featured the significance and possible effect of organisms on ailment recuperation of immunotherapy. Strikingly, the microbiome can bolster the safe framework in the battle against malignant growth (176). Microorganisms have been appeared to advance cancer growth improvement by instigating inflammation. This provocative reaction can, likewise, beneficially affect malignancy treatment. A few treatments, such as CpG-oligonucleotide immunotherapy, rely on inflammation (174).
In an examination performed by Iida et al., it was seen that mice treated with anti-toxins did not react to platinum chemotherapy or CpG-oligonucleotide insusceptible treatment contrasted with mice with flawless gut microorganisms of subcutaneous tumors. These outcomes recommend that gut microbiome improves the impacts of treatments that are subject to aggravation. Desirable reactions to cancer therapy require an unblemished commensal microbiome that intervenes in its belongings by regulating myeloid-inferred cell capacities in the tumor microenvironment. These discoveries underscore the significance of the microbiome in the outcomes of sickness treatment (174).
In most patients treated with traditional cancer therapies, tumors become resistant to therapy, and the chances of tumor recurrence are high (184). Immunotherapy approaches have shown potential in treating hematopoietic (185, 186) and solid cancers (187–189). However, the efficacy of immunotherapy is still limited by the variability of the immune response in different patients and the different susceptibility of tumor types (190, 191). The emerging knowledge of the ability of the gut microbiome to modulate the response to immunotherapy offers new possibilities to improve its efficacy by targeting the microbiome.
Utilization of Check Point Inhibitors (ICIs) has revolutionized cancer treatment across multiple cancer types and has gotten the first since forever FDA endorsement of a tumor agonistic agent in tumors with microsatellite instability (MSI) (192). The most generally utilized ICIs are monoclonal antibodies that focus on the customized cell demise protein (PD-1), its ligand (PD-L1), or the cytotoxic T-lymphocyte antigen 4 protein (CTLA-4). Table 3 shows the microbiome in malignant growth patients dealt with immune checkpoint inhibitors (ICIs). It was proposed by Sivan (197) that Bifidobacterium, a particular taxon of microbial commensals, armed anti-tumor resistance and raised the viability of PD-L1 blocking treatment. They additionally recommended that microbiome could modify the anti-tumor invulnerability as well as the reaction to PD-L1 inhibitors. Routy et al. investigated the relationship of dysbiosis with epithelial tumors to comprehend whether synchronous utilization of anti-infection agents creates essential protection from ICIs in mice and patients. Their outcomes indicated that the anti-tumor impact was undermined in antibiotic (ATB) treatment group, with progression-free survival (PFS) and overall survival (OS), being fundamentally shorter contrasted with that of the control group, showing that ATB could be utilized as a prescient marker for estimating ICIs obstruction. Additionally, utilizing the shotgun sequencing for quantitative metagenomics of the fecal example, Enterococcus hirae and Akkermansia muciniphila were demonstrated to be altogether plentiful in patients with best clinical reaction to ICIs (PFS > 3 months) (196).

Table 3 Characteristics of the microbiome in cancer patients treated with immune check point inhibitors (ICIs).
Naidoo et al. established a connection between the gut microbiome and remedial results after the clinical examination of Chinese patients with cutting edge non-little cell lung carcinoma treated with PD-1 ICIs treatments. As indicated by their outcomes, reacting patients held greater assorted variety and stable arrangement of the natural gut microbiome during treatment and had drawn-out PFS altogether. In detail, Prevotella copri, Bifidobacterium longum, and Alistipes putredinis were improved in responders, though Ruminococcus spp. was found mainly in non-reacting patients. As expected, in the periphery blood of responding patients, a more noteworthy recurrence of natural killer cell and memory CD8+ T cell subgroups was seen (198).
A consortium of 11 bacterial strains was isolated by Tanoue et al. from healthy human donor feces that can thrive in the intestine, increasing interferon-γ-producing CD8 T cells. The colonization of mice improved immune checkpoint inhibitors’ therapeutic effectiveness with these 11-strain mixtures in syngeneic tumor models. All these strains act together in a way that is subject to significant histocompatibility (MHC) class Ia molecules and CD103+ dendritic cells. Primarily the 11 strains represent rare, low-abundance components of the human microbiome, and subsequently, have incredible potential as extensively powerful biotherapeutics (199).
Then again, immunotherapy viability has all the earmarks of being intensely impacted by gut microbiome confirmation. Oral organization of probiotics, for example, A. muciniphila (196) and Bifidobacterium species (197) or fecal microbiota transplantation (FMT) (200) from treatment-responsive patients, considerably upgraded the PD1-based immunotherapy and canceled tumor outgrowth, robotically through the enlarged dendritic cell and T cell reaction (197). Even though these examinations are not utilizing colorectal cancer (CRC) models, seeing how gut microbiome adjusts strong reaction might be necessary to encourage positive remedial results in CRC patients getting immunotherapy, or even to conquer opposition-held by non-responders. To our best information, no clinical preliminaries assessing gut microbiome control and treatment viability are distributed right now (201). A couple of clinical preliminaries are started and now at the selecting stage (Table 4) in light of Fong et al. (201). It stays dark whether these preclinical discoveries can be effectively meant clinical application.
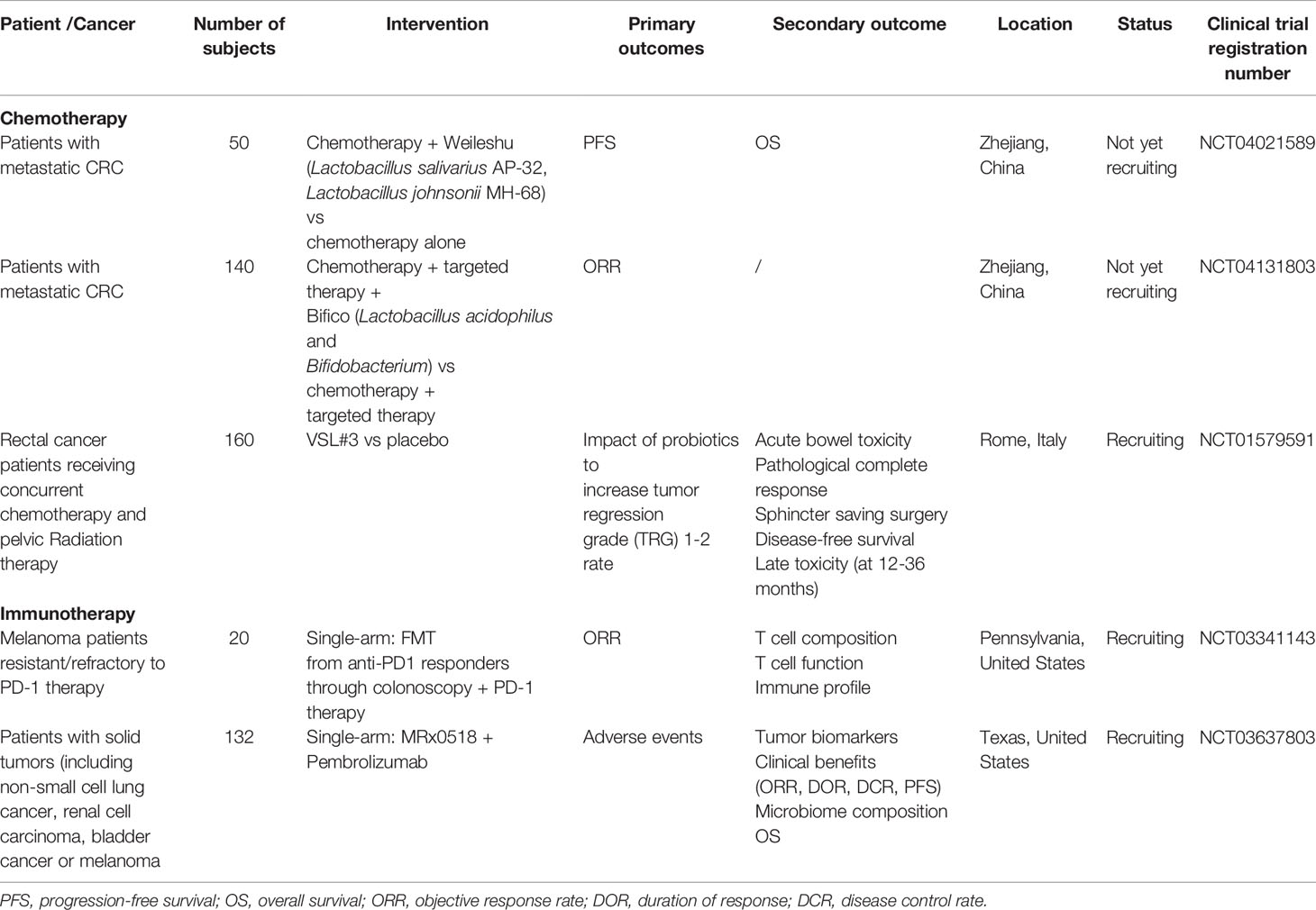
Table 4 Ongoing clinical trials of gut microbiota modulation in potentiating efficacy of anticancer therapies.
The microbiome, aside from the balance of adequacy, may likewise foresee the susceptibility to immunotherapy-associated unfavorable events. While ICIs have given way to overawed immunological resilience to tumors, the danger of auto insusceptibility in healthy tissues is a critical restriction in their utilization. In patients on anti-CTLA-4, the immune-related adverse events (irAEs) happen all the more generally contrasted with those taking anti-PD-1/PD-L1, and when these mediators are used in amalgamation, the frequency of irAEs appears to upsurge consequently (198). The microbiome has been related to the danger of creating immune-related noxiousness. The abundance of species according to study by Dubin et al. (202) among Bacteroidetes phylum, explicitly Barnesiellaceae, Bacteroidaceae, and Rikenellaceae, was related with resistance to colitis in patients with metastatic melanoma dealt with ipilimumab (n=34), though reduced recognition of hereditary pathways engaged with polyamine transport and vitamin B amalgamation in the gut related with an expanded danger of colitis (202).
Moreover, Chaput et al. showed that baseline gut microbiota is a good predictor of clinical response and colitis in metastatic melanoma patients treated with ipilimumab. Twenty-six patients with metastatic melanoma treated with ipilimumab were enrolled in the study. Fecal microbiota composition was assessed at baseline and before each ipilimumab infusion. In their results, baseline gut microbiota enriched with Faecalibacterium and other Firmicutes was associated with beneficial clinical response compared with patients whose baseline microbiota was driven by Bacteroides (194).
The significant reason for repeat and poor prognosis is the treatment failure in colorectal cancer patients, resistance to malignancy medicines has been connected to the nearness of explicit sorts of microbes in the gut. In colorectal cancer patients, scientists observed that resistance to drugs correlated with an expansion in F. nucleatum in the gut. The bacterium appeared to square passing (apoptosis) of the malignant growth cells and trigger autophagy, an endurance device for the disease cells. Yu et al. explored the commitment of gut microbiome to chemoresistance in patients with colorectal malignant growth (203). In colorectal cancer tissues in patients with relapse post-chemotherapy, the F. nucleatum was found in abundance and was related to patient clinicopathological qualities. They additionally showed that F. nucleatum elevated colorectal malignant growth protection from chemotherapy. F. nucleatum, mechanistically, targets TLR4 and MYD88 inborn immune signaling and specific microRNAs to enact the autophagy pathway and modify colorectal malignant growth chemotherapeutic reaction. To control colorectal cancer chemoresistance mechanistically, clinically, and biologically, the F. nucleatum arranges an atomic system of the Toll-like receptor, autophagy, and micro-RNAs. Estimating and focusing on F. nucleatum and its related pathway will yield critical understanding into clinical administration and may enhance colorectal malignant patient outcomes (203).
Albeit a progression of examinations has confirmed the effect of the microbiome on disease treatment, there still lies a lot of vagueness and deficiency in these investigations. The initial test experienced is the inadequate comprehension of the rare microbial species engaged with a better strong reaction.
The Potential of Microbiome in Cancer Therapy
The worldwide malignant growth trouble has risen drastically, making it a critical need to create novel treatments and anticipate which treatment will offer the most advantage to a disease quiet. The use of microorganisms to treat tumors is nothing new. German physician W Busch probably treated the first patient with cancer to be purposefully infected with bacteria in 1868 (204). He induced a bacterial infection in a woman with an inoperable sarcoma by first cauterizing the tumor and then placing her into bedding previously occupied by a patient with “erysipelas” (Streptococcus pyogenes). Busch reported that within a week, the primary tumor and the lymph nodes in the neck had shrunk in size. Unfortunately, the patient died a few days after the infection had begun. Later, in 1883 Friedrich Fehleisen, a German surgeon had identified S. pyogenes as the cause of “erysipelas” and had begun treating patients with cancer with the living cultures of the bacteria with success (205). Almost simultaneously in the USA, William B. Coley was performing similar experiments. In 1981 he injected Streptococcus into a patient with inoperable cancer (206). The infection caused by the bacterium has the side effect of shrinking the malignant tumor, and this was one of the first examples of immunotherapy. Given the risks of using live organisms, Coley developed a mixture of dead bacteria to treat his patients. They were known as Coley’s toxins. He then devoted most of his life as head of the Bone Tumor Service at Memorial Hospital in New York, treating more than 1000 cancer patients with bacteria or bacterial products (207). However, his studies, the first using immunotherapy, were forgotten for several reasons. First, the use of microorganism(s) to treat human cancers provided a short term benefit, but eventually, tumors recurred; second, the discovery of radioactivity and its use to treat tumors in the early 1930s and second the advent of chemotherapy in the 1940s relegated the use of immunotherapies on a second plane.
In recent years, there has been a renewed interest in using bacteria-mediated tumor therapy (204, 208, 209). Although widely used for treating many tumors, chemotherapy causes many off-target effects, such as significant damage to healthy tissues. In contrast, biological generally exert target-specific effects and are relatively safer for human use. Moreover, bacterial-based immunotherapies can penetrate solid tumors and inexpensive. Upon systemic administration, various types of non-pathogenic obligate anaerobes and facultative anaerobes have been shown to infiltrate and selectively replicate within solid tumors (204, 209).
A significant step in the development of bacterial therapeutics is identifying potential species and strains with minimal pathogenicity to the host, and that can replicate precisely in the tumor hypoxic microenvironment. Several genera of bacteria, including Clostridium, Bifidobacterium, E. coli, and attenuated Salmonella, have been used in bacteria-mediated tumor therapy (204, 208–210).
The genera Clostridium and Salmonella are probable the best-studied as vectors in bacteria-mediated tumor therapy. In 1947, it was first shown that direct injection of spores of Clostridium histolyticus into a transplantable mouse sarcoma caused oncolysis and tumor regression; however, mice died soon after (211). In 1964 Moese and Moese injected a non-pathogenic isolate of Clostridium butyricum intravenously and observed the tumor’s disappearance, but again the survival of the mice was non-permanent (212). The ability of Clostridium to grow in hypoxic areas with necrosis gives them an advantage to target tumors. With the development of genetic systems to work with Clostridium, this bacterium has attracted renewed interest as a potential vector to treat solid tumors. Thus, a genetically engineered C. acetobutylicum expressing and secreting the E. coli cytosine deaminase (CDase) has been used against rhabdomyosarcoma-bearing WAG/Rij rats and show that the enzyme was produced in tumors in vivo (213).
Numerous S. typhimurium mutant strains have been studied from the perspective of cancer treatment. One of the significant advantages of using S. typhimurium is that there is a well-developed genetic system that allows for all kinds of genetic manipulations of this organism. Many different immunomodulators have been cloned into S. typhimurium to be expressed at the tumor site. These include cytotoxic proteins, to kill tumor cells directly, and tumor-associated antigens to increase the immune response in the site of the tumor, and prodrug enzymes that modify a substrate to convert it into a toxic product (214, 215).
So far, the only strain of S. typhimurium going through a phase I clinical trial is strain VNP20009, which contains deletions in the msbB and purl genes, to attenuate virulence and avoid septic shock (216).
A more recent approach has been combining bacteria-mediated cancer therapies with other kinds of therapies. For example, a photo-thermal agent, such as melanin-like poly-dopamine (pDA), was coated with VNP20009 targeted to hypoxic and necrotic tumor areas. A mouse model of the tumor was irradiated with a near-infrared laser, which achieved tumor targeting and tumor elimination without relapse or metastasis (217).
Although no reports on the treatment of OSCC have been published, the potential of these techniques to treat oral tumors is enormous. OSCC are solid tumors, and they are easily accessible to be treated at the site, which makes the ideal type of cancer treated with bacterial-mediated tumor therapies.
The Future of Microbiome in Cancer Therapy
We anticipate that the human microbiome will bit by bit play an undeniably conspicuous role in cancer treatment. As of now, the system of the microbiome’s impacts in cancer treatment is not surely known; be that as it may, some clinical pilot studies will assist with uncovering the capability of the microbiome in tumor advancement and malignancy treatment. The advancement of these clinical preliminaries will expel impediments for utilizing the microbiome to improve and help treatment utilizing ICIs. The microbiome, at first, can lessen complexities during malignant growth treatment. Right now, the most widely recognized noxious reaction when utilizing ICIs is related to colitis. The reason for the ailment is uncertain. Curiously, the lactic acid bacterium Lactobacillus reuteri can wipe out ICIs related to colitis and improve weight reduction and irritation (218). Second, the intestinal microbiome upgrades the nourishing assimilation limit of patients with cancer and improves their anti-tumor capacity. The rise of tumor micro-ecological immune nutrition has additionally prepared for the improvement of the microbiome as implement in disease immunotherapy. Third, microbiome research is relied upon to prompt the structure of immunization against tumors. An ongoing microbial-based malignancy immunization has demonstrated its utility. This malignant growth antibody forestalls the development of squamous cell carcinoma communicating epidermal growth factor receptor (EGFR) vIII and instigates EGFR vIII-explicit cell insusceptibility (219).
Current research is thrilling for investigation against tumor resistance and signifies a discovery in the structure of the microbiome. For cancer immunotherapy, FMT is predictable to be the most critical immediate bio optimization tool. FMT is a mainstream innovation that has been utilized clinically to treat repetitive Clostridium difficile contaminations (220).
Finally, recent studies support the hypothesis that periodontal inflammation exacerbates gut inflammation in vivo by translocation of oral pathobionts to the gut, activating the inflammasome in colonic mononuclear phagocytes resulting in inflammation (221, 222). Additionally, periodontitis results in the generation of reactive Th17 cells against oral bacteria. These reactive Th17 cells are exhibit gut tropism and migrate to the inflamed gut. When in the gut, Th17 cells of oral origin can be activated by translocated oral pathobionts and cause the development of colitis, but gut-resident microbes do not activate them. We do not know if gut inflammation could have a similar effect on the oral cavity’s inflammatory environment, as a result of which the severity of head and neck cancer could increase. Studies of the gut microbiome’s effect on the immune response to head and neck cancer are lacking but maybe worth pursuing.
Author Contributions
All authors contributed equally to the writing of the review. All authors contributed to the article and approved the submitted version.
Funding
National Institute of Dental and Craniofacial Research. Grant DE021553.
Conflict of Interest
The authors declare that the research was conducted in the absence of any commercial or financial relationships that could be construed as a potential conflict of interest.
References
1. Sender R, Fuchs S, Milo R. Revised Estimates for the Number of Human and Bacteria Cells in the Body. PLoS Biol (2016) 14:1–14. doi: 10.1371/journal.pbio.1002533
2. Group TNHW, Peterson J, Garges S, Giovanni M, McInnes P, Wang L, et al. The NIH Human Microbiome Project. Genome Res (2009) 19:2317–23. doi: 10.1101/gr.096651.109
3. Fukasawa A, Kurita-Ochiai T, Hashizume T, Kobayashi R, Akimoto Y, Yamamoto M. Porphyromonas gingivalis accelerates atherosclerosis in C57BL/6 mice fed a high-fat diet. Immunopharmacol Immunotoxicol (2012) 34:470–6. doi: 10.3109/08923973.2011.627866
4. Gavin PG, Mullaney JA, Loo D, Cao K-AL, Gottlieb PA, Hill MM, et al. Intestinal Metaproteomics Reveals Host-Microbiota Interactions in Subjects at Risk for Type 1 Diabetes. Diabetes Care (2018) 41:2178–86. doi: 10.2337/dc18-0777
5. Ling Z, Li Z, Liu X, Cheng Y, Luo Y, Tong X, et al. Altered Fecal Microbiota Composition Associated with Food Allergy in Infants. Appl Environ Microbiol (2014) 80:2546–54. doi: 10.1128/AEM.00003-14
6. Sartor RB, Mazmanian SK. Intestinal Microbes in Inflammatory Bowel Diseases. Am J Gastroenterol Suppl (2012) 1:15–21. doi: 10.1038/ajgsup.2012.4
7. Tamboli CP, Neut C, Desreumaux P, Colombel JF. Dysbiosis in inflammatory bowel disease. Gut (2004) 53:1–4. doi: 10.1136/gut.53.1.1
8. de Martel C, Georges D, Bray F, Ferlay J, Clifford GM. Global burden of cancer attributable to infections in 2018: a worldwide incidence analysis. Lancet Glob Health (2020) 8:e180–90. doi: 10.1016/S2214-109X(19)30488-7
9. Moore PS, Chang YH. Why do viruses cause cancer? Highlights of the first century of human tumour virology. Nat Rev Cancer (2010) 10:878–89. doi: 10.1038/nrc2961
10. Marshall Barry J, Warren JR, Blincow Elizabeth D, Phillips M, Goodwin CS, Murray R, et al. Prospective Double-Blind Trial of Duodenal Ulcer Relapse After Eradication of Campylobacter Pylori. Lancet (1988) 332:1437–42. doi: 10.1016/S0140-6736(88)90929-4
11. Amieva M, Peek RM. Pathobiology of Helicobacter pylori-induced Gastric Cancer. Gastroenterology (2015) 150:64–78. doi: 10.1053/j.gastro.2015.09.004
12. Parsonnet J. Helicobacter pylori and gastric cancer. Gastroenterol Clin North Am (1993) 22:89–104.
13. Wang F, Meng W, Wang B, Qiao L. Helicobacter pylori-induced gastric inflammation and gastric cancer. Cancer Lett (2014) 345:196–202. doi: 10.1016/j.canlet.2013.08.016
14. Fox JG, Wang TC. Inflammation, atrophy, and gastric cancer. J Clin Invest (2007) 117:60–9. doi: 10.1172/JCI30111
15. Virchow R, Virchow R. Die krankhaften Geschwülste: dreissig Vorlesungen, gehalten während des Wintersemesters 1862-1863 an der Universität zu Berlin. Berlin: Verlag von August Hirschwald (1863).
16. Bhatelia K, Singh K, Singh R. TLRs: linking inflammation and breast cancer. Cell Signal (2014) 26:2350–7. doi: 10.1016/j.cellsig.2014.07.035
17. Cutolo M, Paolino S, Pizzorni C. Possible contribution of chronic inflammation in the induction of cancer in rheumatic diseases. Clin Exp Rheumatol (2014) 32:839–47.
18. Wang D, DuBois RN. Immunosuppression associated with chronic inflammation in the tumor microenvironment. Carcinogenesis (2015) 36:1085–93. doi: 10.1093/carcin/bgv123
19. Kipanyula MJ, Seke Etet PF, Vecchio L, Farahna M, Nukenine EN, Nwabo Kamdje AH. Signaling pathways bridging microbial-triggered inflammation and cancer. Cell Signal (2013) 25:403–16. doi: 10.1016/j.cellsig.2012.10.014
20. Chang AH, Parsonnet J. Role of Bacteria in Oncogenesis. Clin Microbiol Rev (2010) 23:837–57. doi: 10.1128/CMR.00012-10
21. Kostic AD, Gevers D, Pedamallu CS, Michaud M, Duke F, Earl AM, et al. Genomic analysis identifies association of Fusobacterium with colorectal carcinoma. Genome Res (2012) 22:292–8. doi: 10.1101/gr.126573.111
22. Whitmore SE, Lamont RJ. Oral Bacteria and Cancer. PLoS Pathog (2014) 10:e1003933. doi: 10.1371/journal.ppat.1003933
23. Schwabe RF, Jobin C. The microbiome and cancer. Nat Rev Cancer (2013) 13:800–12. doi: 10.1038/nrc3610
24. Arthur JC, Gharaibeh RZ, Mühlbauer M, Perez-Chanona E, Uronis JM, McCafferty J, et al. Microbial genomic analysis reveals the essential role of inflammation in bacteria-induced colorectal cancer. Nat Commun (2014) 5:4724. doi: 10.1038/ncomms5724
25. Ohadian Moghadam S, Momeni SA. Human microbiome and prostate cancer development: current insights into the prevention and treatment. Front Med (2020) 14(3):1–22. doi: 10.1007/s11684-019-0731-7
26. Dutta U, Garg PK, Kumar R, Tandon RK. Typhoid carriers among patients with gallstones are at increased risk for carcinoma of the gallbladder. Am J Gastroenterol (2000) 95:784–7. doi: 10.1111/j.1572-0241.2000.01860.x
27. Lax AJ, Thomas W. How bacteria could cause cancer: one step at a time. Trends Microbiol (2002) 10:293–9. doi: 10.1016/s0966-842x(02)02360-0
28. Vaishnavi C, Kochhar R, Singh G, Kumar S, Singh S, Singh K. Epidemiology of typhoid carriers among blood donors and patients with biliary, gastrointestinal and other related diseases. Microbiol Immunol (2005) 49:107–12. doi: 10.1111/j.1348-0421.2005.tb03709.x
29. Ellmerich S, Schöller M, Duranton B, Gossé F, Galluser M, Klein JP, et al. Promotion of intestinal carcinogenesis by Streptococcus bovis. Carcinogenesis (2000) 21:753–6. doi: 10.1093/carcin/21.4.753
30. Gold JS, Bayar S, Salem RR. Association of Streptococcus bovis bacteremia with colonic neoplasia and extracolonic malignancy. Arch Surg (2004) 139:760–5. doi: 10.1001/archsurg.139.7.760
31. Zarkin BA, Lillemoe KD, Cameron JL, Effron PN, Magnuson TH, Pitt HA. The triad of Streptococcus bovis bacteremia, colonic pathology, and liver disease. Ann Surg (1990) 211:786–791; discussion 791-792. doi: 10.1097/00000658-199006000-00019
32. Anttila T, Koskela P, Leinonen M, Laukkanen P, Hakulinen T, Lehtinen M, et al. Chlamydia pneumoniae infection and the risk of female early-onset lung cancer. Int J Cancer (2003) 107:681–2. doi: 10.1002/ijc.11353
33. Hua-Feng X, Yue-Ming W, Hong L, Junyi D. A meta-analysis of the association between Chlamydia pneumoniae infection and lung cancer risk. Indian J Cancer (2015) 52(Suppl 2):e112–115. doi: 10.4103/0019-509X.172506
34. Khan S, Imran A, Khan AA, Abul Kalam M, Alshamsan A. Systems Biology Approaches for the Prediction of Possible Role of Chlamydia pneumoniae Proteins in the Etiology of Lung Cancer. PLoS One (2016) 11:e0148530. doi: 10.1371/journal.pone.0148530
35. Koyi H, Brandén E, Gnarpe J, Gnarpe H, Steen B. An association between chronic infection with Chlamydia pneumoniae and lung cancer. A prospective 2-year study. APMIS (2001) 109:572–80. doi: 10.1034/j.1600-0463.2001.d01-177.x
36. Littman AJ, White E, Jackson LA, Thornquist MD, Gaydos CA, Goodman GE, et al. Chlamydia pneumoniae infection and risk of lung cancer. Cancer Epidemiol Biomarkers Prev (2004) 13:1624–30. doi: 10.1016/S1047-2797(03)00148-0
37. Boleij A, van Gelder MMHJ, Swinkels DW, Tjalsma H. Clinical Importance of Streptococcus gallolyticus infection among colorectal cancer patients: systematic review and meta-analysis. Clin Infect Dis (2011) 53:870–8. doi: 10.1093/cid/cir609
38. Bronzato JD, Bomfim RA, Edwards DH, Crouch D, Hector MP, Gomes BPFA. Detection of Fusobacterium in oral and head and neck cancer samples: A systematic review and meta-analysis. Arch Oral Biol (2020) 112:104669. doi: 10.1016/j.archoralbio.2020.104669
39. Krishnan S, Eslick GD. Streptococcus bovis infection and colorectal neoplasia: a meta-analysis. Colorectal Dis (2014) 16:672–80. doi: 10.1111/codi.12662
40. Peng B-J, Cao C-Y, Li W, Zhou Y-J, Zhang Y, Nie Y-Q, et al. Diagnostic Performance of Intestinal Fusobacterium nucleatum in Colorectal Cancer: A Meta-Analysis. Chin Med J (2018) 131:1349–56. doi: 10.4103/0366-6999.232814
41. Repass J, Reproducibility Project: Cancer Biology, Iorns E, Denis A, Williams SR, Perfito N, et al. Replication Study: Fusobacterium nucleatum infection is prevalent in human colorectal carcinoma. Elife (2018) 7:1–12. doi: 10.7554/eLife.25801
42. Zhan P, Suo L, Qian Q, Shen X, Qiu L-X, Yu L, et al. Chlamydia pneumoniae infection and lung cancer risk: a meta-analysis. Eur J Cancer (2011) 47:742–7. doi: 10.1016/j.ejca.2010.11.003
43. Hundal R, Shaffer EA. Gallbladder cancer: epidemiology and outcome. Clin Epidemiol (2014) 6:99–109. doi: 10.2147/CLEP.S37357
44. Welton JC, Marr JS, Friedman SM. Association between hepatobiliary cancer and typhoid carrier state. Lancet (1979) 1:791–4. doi: 10.1016/s0140-6736(79)91315-1
45. Koshiol J, Wozniak A, Cook P, Adaniel C, Acevedo J, Azócar L, et al. Salmonella enterica serovar Typhi and gallbladder cancer: a case-control study and meta-analysis. Cancer Med (2016) 5:3310–235. doi: 10.1002/cam4.915
46. Dutta U, Bush N, Kalsi D, Popli P, Kapoor VK. Epidemiology of gallbladder cancer in India. Chin Clin Oncol (2019) 8:33. doi: 10.21037/cco.2019.08.03
47. Nagaraja V, Eslick GD. Systematic review with meta-analysis: the relationship between chronic Salmonella typhi carrier status and gall-bladder cancer. Aliment Pharmacol Ther (2014) 39:745–50. doi: 10.1111/apt.12655
48. Scanu T, Spaapen RM, Bakker JM, Pratap CB, Wu L, Hofland I, et al. Salmonella Manipulation of Host Signaling Pathways Provokes Cellular Transformation Associated with Gallbladder Carcinoma. Cell Host Microbe (2015) 17:763–74. doi: 10.1016/j.chom.2015.05.002
49. Couturier-Maillard A, Secher T, Rehman A, Normand S, De Arcangelis A, Haesler R, et al. NOD2-mediated dysbiosis predisposes mice to transmissible colitis and colorectal cancer. J Clin Invest (2013) 123:700–11. doi: 10.1172/JCI62236
50. Zackular JP, Baxter NT, Iverson KD, Sadler WD, Petrosino JF, Chen GY, et al. The gut microbiome modulates colon tumorigenesis. MBio (2013) 4:e00692–00613. doi: 10.1128/mBio.00692-13
51. Sheflin AM, Whitney AK, Weir TL. Cancer-Promoting Effects of Microbial Dysbiosis. Curr Oncol Rep (2014) 16:406. doi: 10.1007/s11912-014-0406-0
52. La Rosa GRM, Gattuso G, Pedullà E, Rapisarda E, Nicolosi D, Salmeri M. Association of oral dysbiosis with oral cancer development. Oncol Lett (2020) 19:3045–58. doi: 10.3892/ol.2020.11441
53. Cook TJ. Blood dyscrasias as related to periodontal disease; with special reference to leukemia. J Periodontol (1947) 18:159–65. doi: 10.1902/jop.1947.18.4.159
54. Wentz FM. Oral Manifestations of the Blood Diseases. J Am Dental Assoc (1952) 44:698–701. doi: 10.14219/jada.archive.1952.0114
55. Corbella S, Veronesi P, Galimberti V, Weinstein R, Del Fabbro M, Francetti L. Is periodontitis a risk indicator for cancer? A meta-analysis. PLoS One (2018) 13:e0195683. doi: 10.1371/journal.pone.0195683
56. Wu Y, Shi X, Li Y, Shi X, Gu Y, Qian Q, et al. Hematopoietic and lymphatic cancers in patients with periodontitis: a systematic review and meta-analysis. Med Oral Patol Oral Cir Bucal (2020) 25:e21–8. doi: 10.4317/medoral.23166
57. Bracci PM. Oral Health and the Oral Microbiome in Pancreatic Cancer: An Overview of Epidemiological Studies. Cancer J (2017) 23:310–4. doi: 10.1097/PPO.0000000000000287
58. Maisonneuve P, Amar S, Lowenfels AB. Periodontal disease, edentulism, and pancreatic cancer: a meta-analysis. Ann Oncol (2017) 28:985–95. doi: 10.1093/annonc/mdx019
59. Michaud DS, Fu Z, Shi J, Chung M. Periodontal Disease, Tooth Loss, and Cancer Risk. Epidemiol Rev (2017) 39:49–58. doi: 10.1093/epirev/mxx006
60. Xiao L, Zhang Q, Peng Y, Wang D, Liu Y. The effect of periodontal bacteria infection on incidence and prognosis of cancer: A systematic review and meta-analysis. Medicine (2020) 99:e19698. doi: 10.1097/MD.0000000000019698
61. Shi T, Min M, Sun C, Zhang Y, Liang M, Sun Y. Periodontal disease and susceptibility to breast cancer: A meta-analysis of observational studies. J Clin Periodontol (2018) 45:1025–33. doi: 10.1111/jcpe.12982
62. Ren HG, Luu HN, Cai H, Xiang YB, Steinwandel M, Gao YT, et al. Oral health and risk of colorectal cancer: results from three cohort studies and a meta-analysis. Ann Oncol (2016) 27:1329–36. doi: 10.1093/annonc/mdw172
63. Ochi A, Nguyen AH, Bedrosian AS, Mushlin HM, Zarbakhsh S, Barilla R, et al. MyD88 inhibition amplifies dendritic cell capacity to promote pancreatic carcinogenesis via Th2 cells. J Exp Med (2012) 209:1671–87. doi: 10.1084/jem.20111706
64. Peters BA, Wu J, Pei Z, Yang L, Purdue MP, Freedman ND, et al. Oral microbiome composition reflects prospective risk for esophageal cancers. Cancer Res (2017) 77(23):6777–87.
65. Narikiyo M, Tanabe C, Yamada Y, Igaki H, Tachimori Y, Kato H, et al. Frequent and preferential infection of Treponema denticola, Streptococcus mitis, and Streptococcus anginosus in esophageal cancers. Cancer Sci (2004) 95(7):569–74.
66. Snider EJ, Compres G, Freedberg DE, Giddins MJ, Khiabanian H, Lightdale CJ, et al. Barrett’s esophagus is associated with a distinct oral microbiome. Clin Transl Gastroenterol (2018) 9:135. doi: 10.1038/s41424-018-0005-8
67. Chen X, Winckler B, Lu M, Cheng H, Yuan Z, Yang Y, et al. Oral Microbiota and Risk for Esophageal Squamous Cell Carcinoma in a High-Risk Area of China. PLoS One (2015) 10:e0143603. doi: 10.1371/journal.pone.0143603
68. Fan X, Alekseyenko AV, Wu J, Peters BA, Jacobs EJ, Gapstur SM, et al. Human oral microbiome and prospective risk for pancreatic cancer: a population-based nested case-control study. Gut (2018) 67:120–7. doi: 10.1136/gutjnl-2016-312580
69. Michaud DS, Izard J, Wilhelm-Benartzi CS, You D-H, Grote VA, Tjønneland A, et al. Plasma antibodies to oral bacteria and risk of pancreatic cancer in a large European prospective cohort study. Gut (2013) 62:1764–70. doi: 10.1136/gutjnl-2012-303006
70. Mitsuhashi K, Nosho K, Sukawa Y, Matsunaga Y, Ito M, Kurihara H, et al. Association of Fusobacterium species in pancreatic cancer tissues with molecular features and prognosis. Oncotarget (2015) 6:7209–20. doi: 10.18632/oncotarget.3109
71. Farrell JJ, Zhang L, Zhou H, Chia D, Elashoff D, Akin D, et al. Variations of oral microbiota are associated with pancreatic diseases including pancreatic cancer. Gut (2012) 61:582–8. doi: 10.1136/gutjnl-2011-300784
72. Torres PJ, Fletcher EM, Gibbons SM, Bouvet M, Doran KS, Kelley ST. Characterization of the salivary microbiome in patients with pancreatic cancer. PeerJ (2015) 3:e1373. doi: 10.7717/peerj.1373
73. Vogtmann E, Han Y, Caporaso JG, Bokulich N, Mohamadkhani A, Moayyedkazemi A, et al. Oral microbial community composition is associated with pancreatic cancer: A case-control study in Iran. Cancer Med (2020) 9:797–806. doi: 10.1002/cam4.2660
74. Del Castillo E, Meier R, Chung M, Koestler DC, Chen T, Paster BJ, et al. The Microbiomes of Pancreatic and Duodenum Tissue Overlap and Are Highly Subject Specific but Differ between Pancreatic Cancer and Noncancer Subjects. Cancer Epidemiol Biomarkers Prev (2019) 28:370–83. doi: 10.1158/1055-9965.EPI-18-0542
75. Olson SH, Satagopan J, Xu Y, Ling L, Leong S, Orlow I, et al. The oral microbiota in patients with pancreatic cancer, patients with IPMNs, and controls: a pilot study. Cancer Causes Control (2017) 28:959–69. doi: 10.1007/s10552-017-0933-8
76. Lu H, Ren Z, Li A, Li J, Xu S, Zhang H, et al. Tongue coating microbiome data distinguish patients with pancreatic head cancer from healthy controls. J Oral Microbiol (2019) 11:1563409. doi: 10.1080/20002297.2018.1563409
77. Lu H, Ren Z, Li A, Zhang H, Jiang J, Xu S, et al. Deep sequencing reveals microbiota dysbiosis of tongue coat in patients with liver carcinoma. Sci Rep (2016) 6:33142.
78. Yan X, Yang M, Liu J, Gao R, Hu J, Li J, et al. Discovery and validation of potential bacterial biomarkers for lung cancer. Am J Cancer Res (2015) 5(10):3111.
79. Tsay J-CJ, Wu BG, Badri MH, Clemente JC, Shen N, Meyn P, et al. Airway microbiota is associated with upregulation of the PI3K pathway in lung cancer. Am J respiratory Crit Care Med (2018) 198(9):1188–98.
80. Yang C-Y, Yeh Y-M, Yu H-Y, Chin C-Y, Hsu C-W, Liu H, et al. Oral Microbiota Community Dynamics Associated With Oral Squamous Cell Carcinoma Staging. Front Microbiol (2018) 9:862. doi: 10.3389/fmicb.2018.00862
81. Zhang W, Luo J, Dong X, Zhao S, Hao Y, Peng C, et al. Salivary microbial dysbiosis is associated with systemic inflammatory markers and predicted oral metabolites in non-small cell lung cancer patients. J Cancer (2019) 10(7):1651.
82. Yang Y, Zheng W, Cai Q-Y, Shrubsole MJ, Pei Z, Brucker R, et al. Cigarette smoking and oral microbiota in low-income and African-American populations. J Epidemiol Community Health (2019) 73(12):1108–15.
83. Ahn J, Sinha R, Pei Z, Dominianni C, Wu J, Shi J, et al. Human gut microbiome and risk for colorectal cancer. J Natl Cancer Institute (2013) 105(24):1907–11.
84. Tahara T, Yamamoto E, Suzuki H, Maruyama R, Chung W, Garriga J, et al. Fusobacterium in colonic flora and molecular features of colorectal carcinoma. Cancer Res (2014) 74:1311–8. doi: 10.1158/0008-5472.CAN-13-1865
85. Kato I, Vasquez AA, Moyerbrailean G, Land S, Sun J, Lin H-S, et al. Oral microbiome and history of smoking and colorectal cancer. J epidemiological Res (2016) 2(2):92.
86. Flemer B, Warren RD, Barrett MP, Cisek K, Das A, Jeffery IB, et al. The oral microbiota in colorectal cancer is distinctive and predictive. Gut (2018) 67:1454–63. doi: 10.1136/gutjnl-2017-314814
87. Kostic AD, Chun E, Robertson L, Glickman JN, Gallini CA, Michaud M, et al. Fusobacterium nucleatum potentiates intestinal tumorigenesis and modulates the tumor-immune microenvironment. Cell Host Microbe (2013) 14:207–15. doi: 10.1016/j.chom.2013.07.007
88. Flanagan L, Schmid J, Ebert M, Soucek P, Kunicka T, Liska V, et al. Fusobacterium nucleatum associates with stages of colorectal neoplasia development, colorectal cancer and disease outcome. Eur J Clin Microbiol Infect Dis (2014) 33:1381–90. doi: 10.1007/s10096-014-2081-3
89. Castellarin M, Warren RL, Freeman JD, Dreolini L, Krzywinski M, Strauss J, et al. Fusobacterium nucleatum infection is prevalent in human colorectal carcinoma. Genome Res (2012) 22:299–306. doi: 10.1101/gr.126516.111
90. Gao Z, Guo B, Gao R, Zhu Q, Qin H. Microbiota disbiosis is associated with colorectal cancer. Front Microbiol (2015) 6:20:1–9. doi: 10.3389/fmicb.2015.00020
91. Kageyama S, Takeshita T, Takeuchi K, Asakawa M, Matsumi R, Furuta M, et al. Characteristics of the Salivary Microbiota in Patients With Various Digestive Tract Cancers. Front Microbiol (2019) 10:1780. doi: 10.3389/fmicb.2019.01780
92. Drewes JL, White JR, Dejea CM, Fathi P, Iyadorai T, Vadivelu J, et al. High-resolution bacterial 16S rRNA gene profile meta-analysis and biofilm status reveal common colorectal cancer consortia. NPJ Biofilms Microbiomes (2017) 3:34. doi: 10.1038/s41522-017-0040-3
93. Thomas AM, Manghi P, Asnicar F, Pasolli E, Armanini F, Zolfo M, et al. Metagenomic analysis of colorectal cancer datasets identifies cross-cohort microbial diagnostic signatures and a link with choline degradation. Nat Med (2019) 25:667–78. doi: 10.1038/s41591-019-0405-7
94. Hu J, Han S, Chen Y, Ji Z. Variations of tongue coating microbiota in patients with gastric cancer. BioMed Res Int (2015) 2015.
95. Sun J-H, Li X-L, Yin J, Li Y-H, Hou B-X, Zhang Z. A screening method for gastric cancer by oral microbiome detection. Oncol Rep (2018) 39:2217–24. doi: 10.3892/or.2018.6286
96. Wu J, Xu S, Xiang C, Cao Q, Li Q, Huang J, et al. Tongue Coating Microbiota Community and Risk Effect on Gastric Cancer. J Cancer (2018) 9:4039–48. doi: 10.7150/jca.25280
97. Wang H, Altemus J, Niazi F, Green H, Calhoun BC, Sturgis C, et al. Breast tissue, oral and urinary microbiomes in breast cancer. Oncotarget (2017) 8(50):88122.
98. Hieken TJ, Chen J, Hoskin TL, Walther-Antonio M, Johnson S, Ramaker S, et al. The microbiome of aseptically collected human breast tissue in benign and malignant disease. Sci Rep (2016) 6:30751.
99. Byrd V, Getz T, Padmanabhan R, Arora H, Eng C. The microbiome in PTEN hamartoma tumor syndrome. Endocrine-related Cancer (2018) 25(3):233–43.
100. Shang F-M, Liu H-L. Fusobacterium nucleatum and colorectal cancer: A review. World J Gastrointest Oncol (2018) 10:71–81. doi: 10.4251/wjgo.v10.i3.71
101. Cook NR. Methods for Evaluating Novel Biomarkers - a New Paradigm. Int J Clin Pract (2010) 64:1723–7. doi: 10.1111/j.1742-1241.2010.02469.x
102. Zheng D-W, Dong X, Pan P, Chen K-W, Fan J-X, Cheng S-X, et al. Phage-guided modulation of the gut microbiota of mouse models of colorectal cancer augments their responses to chemotherapy. Nat BioMed Eng (2019) 3:717–28. doi: 10.1038/s41551-019-0423-2
103. Komiya Y, Shimomura Y, Higurashi T, Sugi Y, Arimoto J, Umezawa S, et al. Patients with colorectal cancer have identical strains of Fusobacterium nucleatum in their colorectal cancer and oral cavity. Gut (2019) 68:1335–7. doi: 10.1136/gutjnl-2018-316661
104. Rubinstein MR, Wang X, Liu W, Hao Y, Cai G, Han YW. Fusobacterium nucleatum promotes colorectal carcinogenesis by modulating E-cadherin/β-catenin signaling via its FadA adhesin. Cell Host Microbe (2013) 14:195–206. doi: 10.1016/j.chom.2013.07.012
105. Han YW. Fusobacterium nucleatum: a commensal-turned pathogen. Curr Opin Microbiol (2015) 23C:141–7. doi: 10.1016/j.mib.2014.11.013
106. Chen Y, Peng Y, Yu J, Chen T, Wu Y, Shi L, et al. Invasive Fusobacterium nucleatum activates beta-catenin signaling in colorectal cancer via a TLR4/P-PAK1 cascade. Oncotarget (2017) 8:31802–14. doi: 10.18632/oncotarget.15992
107. Nelson WJ, Nusse R. Convergence of Wnt, β-Catenin, and Cadherin Pathways. Science (2004) 303:1483–7. doi: 10.1126/science.1094291
108. Tian X, Liu Z, Niu B, Zhang J, Tan TK, Lee SR, et al. E-Cadherin/β-Catenin Complex and the Epithelial Barrier. J BioMed Biotechnol (2011) 2011:1–7. doi: 10.1155/2011/567305
109. Rubinstein MR, Baik JE, Lagana SM, Han RP, Raab WJ, Sahoo D, et al. Fusobacterium nucleatum promotes colorectal cancer by inducing Wnt/β-catenin modulator Annexin A1. EMBO Rep (2019) 20:1–17. doi: 10.15252/embr.201847638
110. Alao JP. The regulation of cyclin D1 degradation: roles in cancer development and the potential for therapeutic invention. Mol Cancer (2007) 6:24. doi: 10.1186/1476-4598-6-24
111. Bray F, Ferlay J, Soerjomataram I, Siegel RL, Torre LA, Jemal A. Global cancer statistics 2018: GLOBOCAN estimates of incidence and mortality worldwide for 36 cancers in 185 countries. CA Cancer J Clin (2018) 68:394–424. doi: 10.3322/caac.21492
112. Siegel RL, Miller KD, Jemal A. Cancer statistics, 2020. CA: A Cancer J Clinicians (2020) 70:7–30. doi: 10.3322/caac.21590
113. Huber MA, Tantiwongkosi B. Oral and oropharyngeal cancer. Med Clin North Am (2014) 98:1299–321. doi: 10.1016/j.mcna.2014.08.005
114. Mücke T, Wagenpfeil S, Kesting MR, Hölzle F, Wolff K-D. Recurrence interval affects survival after local relapse of oral cancer. Oral Oncol (2009) 45:687–91. doi: 10.1016/j.oraloncology.2008.10.011
115. Rogers SN, Brown JS, Woolgar JA, Lowe D, Magennis P, Shaw RJ, et al. Survival following primary surgery for oral cancer. Oral Oncol (2009) 45:201–11. doi: 10.1016/j.oraloncology.2008.05.008
116. Wang B, Zhang S, Yue K, Wang X-D. The recurrence and survival of oral squamous cell carcinoma: a report of 275 cases. Chin J Cancer (2013) 32:614–8. doi: 10.5732/cjc.012.10219
117. Boysen M, Lövdal O, Tausjö J, Winther F. The value of follow-up in patients treated for squamous cell carcinoma of the head and neck. Eur J Cancer (1992) 28:426–30. doi: 10.1016/S0959-8049(05)80068-1
118. Braakhuis BJM, Tabor MP, Leemans CR, van der Waal I, Snow GB, Brakenhoff RH. Second primary tumors and field cancerization in oral and oropharyngeal cancer: molecular techniques provide new insights and definitions. Head Neck (2002) 24:198–206. doi: 10.1002/hed.10042
119. Schwartz GJ, Mehta RH, Wenig BL, Shaligram C, Portugal LG. Salvage treatment for recurrent squamous cell carcinoma of the oral cavity. Head Neck (2000) 22:34–41. doi: 10.1002/(SICI)1097-0347(200001)22:1<34::AID-HED6>3.0.CO;2-3
120. Liu S-A, Wong Y-K, Lin J-C, Poon C-K, Tung K-C, Tsai W-C. Impact of recurrence interval on survival of oral cavity squamous cell carcinoma patients after local relapse. Otolaryngol Head Neck Surg (2007) 136:112–8. doi: 10.1016/j.otohns.2006.07.002
121. Hardefeldt HA, Cox MR, Eslick GD. Association between human papillomavirus (HPV) and oesophageal squamous cell carcinoma: a meta-analysis. Epidemiol Infect (2014) 142:1119–37. doi: 10.1017/S0950268814000016
122. Liyanage SS, Rahman B, Ridda I, Newall AT, Tabrizi SN, Garland SM, et al. The aetiological role of human papillomavirus in oesophageal squamous cell carcinoma: a meta-analysis. PLoS One (2013) 8:e69238. doi: 10.1371/journal.pone.0069238
123. Petrick JL, Wyss AB, Butler AM, Cummings C, Sun X, Poole C, et al. Prevalence of human papillomavirus among oesophageal squamous cell carcinoma cases: systematic review and meta-analysis. Br J Cancer (2014) 110:2369–77. doi: 10.1038/bjc.2014.96
124. Syrjänen K, Syrjänen S. Detection of human papillomavirus in esophageal papillomas: systematic review and meta-analysis. APMIS (2013) 121:363–74. doi: 10.1111/apm.12003
125. Carpén T, Syrjänen S, Jouhi L, Randen-Brady R, Haglund C, Mäkitie A, et al. Epstein-Barr virus (EBV) and polyomaviruses are detectable in oropharyngeal cancer and EBV may have prognostic impact. Cancer Immunol Immunother (2020) 69:1615–26. doi: 10.1007/s00262-020-02570-3
126. Drop B, Strycharz-Dudziak M, Kliszczewska E, Polz-Dacewicz M. Coinfection with Epstein-Barr Virus (EBV), Human Papilloma Virus (HPV) and Polyoma BK Virus (BKPyV) in Laryngeal, Oropharyngeal and Oral Cavity Cancer. Int J Mol Sci (2017) 18: 1–13. doi: 10.3390/ijms18122752
127. Jalouli J, Jalouli MM, Sapkota D, Ibrahim SO, Larsson P-A, Sand L. Human papilloma virus, herpes simplex virus and epstein barr virus in oral squamous cell carcinoma from eight different countries. Anticancer Res (2012) 32:571–80.
128. Guerrero-Preston R, Godoy-Vitorino F, Jedlicka A, Rodríguez-Hilario A, González H, Bondy J, et al. 16S rRNA amplicon sequencing identifies microbiota associated with oral cancer, human papilloma virus infection and surgical treatment. Oncotarget (2016) 7(32):51320.
129. Tateda M, Shiga K, Saijo S, Sone M, Hori T, Yokoyama J, et al. Streptococcus anginosus in head and neck squamous cell carcinoma: implication in carcinogenesis. Int J Mol Med (2000) 6(6):699–1402.
130. Sasaki M, Yamaura C, Ohara-Nemoto Y, Tajika S, Kodama Y, Ohya T, et al. Streptococcus anginosus infection in oral cancer and its infection route. Oral Dis (2005) 11(3):151–6.
131. Mager DL, Haffajee AD, Devlin PM, Norris CM, Posner MR, Goodson JM. The salivary microbiota as a diagnostic indicator of oral cancer: a descriptive, non-randomized study of cancer-free and oral squamous cell carcinoma subjects. J Trans Med (2005) 3:27. doi: 10.1186/1479-5876-3-27
132. Lee J, Roberts JS, Atanasova KR, Chowdhury N, Han K, Yilmaz Ö. Human Primary Epithelial Cells Acquire an Epithelial-Mesenchymal-Transition Phenotype during Long-Term Infection by the Oral Opportunistic Pathogen, Porphyromonas gingivalis. Front Cell Infect Microbiol (2017) 7:493. doi: 10.3389/fcimb.2017.00493
133. Pushalkar S, Ji X, Li Y, Estilo C, Yegnanarayana R, Singh B, et al. Comparison of oral microbiota in tumor and non-tumor tissues of patients with oral squamous cell carcinoma. BMC Microbiol (2012) 12(1):1–15.
134. Wang H, Funchain P, Bebek G, Altemus J, Zhang H, Niazi F, et al. Microbiomic differences in tumor and paired-normal tissue in head and neck squamous cell carcinomas. Genome Med (2017) 9(1):1–10.
135. Zhang Z, Yang J, Feng Q, Chen B, Li M, Liang C, et al. Compositional and functional analysis of the microbiome in tissue and saliva of oral squamous cell carcinoma. Front Microbiol (2019) 10:1439.
136. Zhao H, Chu M, Huang Z, Yang X, Ran S, Hu B, et al. Variations in oral microbiota associated with oral cancer. Sci Rep (2017) 7:11773. doi: 10.1038/s41598-017-11779-9
137. Hooper SJ, Crean SJ, Lewis MA, Spratt DA, Wade WG, Wilson MJ. Viable bacteria present within oral squamous cell carcinoma tissue. J Clin Microbiol (2006) 44(5):1719–25.
138. Katz J, Onate MD, Pauley KM, Bhattacharyya I, Cha S. Presence of Porphyromonas gingivalis in gingival squamous cell carcinoma. Int J Oral Sci (2011) 3(4):209–15.
139. Sakamoto H, Naito H, Ohta Y, Tanakna R, Maeda N, Sasaki J, et al. Isolation of bacteria from cervical lymph nodes in patients with oral cancer. Arch Oral Biol (1999) 44(10):789–93.
140. Nagy K, Sonkodi I, Szöke I, Nagy E, Newman H. The microflora associated with human oral carcinomas. Oral Oncol (1998) 34(4):304–8.
141. Amer A, Galvin S, Healy CM, Moran GP. The microbiome of potentially malignant oral leukoplakia exhibits enrichment for Fusobacterium, Leptotrichia, Campylobacter, and Rothia species. Front Microbiol (2017) 8:2391.
142. Galvão-Moreira LV, da Cruz MCFN. Oral microbiome, periodontitis and risk of head and neck cancer. Oral Oncol (2016) 53:17–9. doi: 10.1016/j.oraloncology.2015.11.013
143. Karmakar S, Kar A, Thakur S, Rao VUS. Periodontitis and oral Cancer-A striking link. Oral Oncol (2020) 106:104630. doi: 10.1016/j.oraloncology.2020.104630
144. Hajishengallis G. Immunomicrobial pathogenesis of periodontitis: keystones, pathobionts, and host response. Trends Immunol (2014) 35:3–11. doi: 10.1016/j.it.2013.09.001
145. Hajishengallis G. Periodontitis: from microbial immune subversion to systemic inflammation. Nat Rev Immunol (2015) 15:30–44. doi: 10.1038/nri3785
146. Fitzpatrick SG, Katz J. The association between periodontal disease and cancer: a review of the literature. J Dent (2010) 38:83–95. doi: 10.1016/j.jdent.2009.10.007
147. Javed F, Warnakulasuriya S. Is there a relationship between periodontal disease and oral cancer? A systematic review of currently available evidence. Crit Rev Oncol Hematol (2015) 97:197–205. doi: 10.1016/j.critrevonc.2015.08.018
148. Li Y, Tan X, Zhao X, Xu Z, Dai W, Duan W, et al. Composition and function of oral microbiota between gingival squamous cell carcinoma and periodontitis. Oral Oncol (2020) 107:104710. doi: 10.1016/j.oraloncology.2020.104710
149. Su S-C, Chang L-C, Huang H-D, Peng C-Y, Chuang C-Y, Chen Y-T, et al. Oral microbial dysbiosis and its performance in predicting oral cancer. Carcinogenesis (2020) 1–9. doi: 10.1093/carcin/bgaa062
150. Kakabadze MZ, Paresishvili T, Karalashvili L, Chakhunashvili D, Kakabadze Z. Oral microbiota and oral cancer: Review. Oncol Rev (2020) 14:476. doi: 10.4081/oncol.2020.476
151. Perera M, Al-Hebshi NN, Perera I, Ipe D, Ulett GC, Speicher DJ, et al. Inflammatory Bacteriome and Oral Squamous Cell Carcinoma. J Dent Res (2018) 97:725–32. doi: 10.1177/0022034518767118
152. Al-Hebshi NN, Nasher AT, Idris AM, Chen T. Robust species taxonomy assignment algorithm for 16S rRNA NGS reads: application to oral carcinoma samples. J Oral Microbiol (2015) 7:28934. doi: 10.3402/jom.v7.28934
153. Stashenko P, Yost S, Choi Y, Danciu T, Chen T, Yoganathan S, et al. The Oral Mouse Microbiome Promotes Tumorigenesis in Oral Squamous Cell Carcinoma. mSystems (2019) 4:e00323–19. doi: 10.1128/mSystems.00323-19
154. Geng F, Liu J, Guo Y, Li C, Wang H, Wang H, et al. Persistent Exposure to Porphyromonas gingivalis Promotes Proliferative and Invasion Capabilities, and Tumorigenic Properties of Human Immortalized Oral Epithelial Cells. Front Cell Infect Microbiol (2017) 7:57. doi: 10.3389/fcimb.2017.00057
155. Ha NH, Woo BH, Kim DJ, Ha ES, Choi JI, Kim SJ, et al. Prolonged and repetitive exposure to Porphyromonas gingivalis increases aggressiveness of oral cancer cells by promoting acquisition of cancer stem cell properties. Tumour Biol (2015) 36:9947–60. doi: 10.1007/s13277-015-3764-9
156. Ha NH, Park DG, Woo BH, Kim DJ, Choi JI, Park BS, et al. Porphyromonas gingivalis increases the invasiveness of oral cancer cells by upregulating IL-8 and MMPs. Cytokine (2016) 86:64–72. doi: 10.1016/j.cyto.2016.07.013
157. Wen L, Mu W, Lu H, Wang X, Fang J, Jia Y, et al. Porphyromonas gingivalis Promotes Oral Squamous Cell Carcinoma Progression in an Immune Microenvironment. J Dental Res (2020) 99(6):666–675. doi: 10.1177/0022034520909312
158. Sztukowska MN, Ojo A, Ahmed S, Carenbauer AL, Wang Q, Shumway B, et al. Porphyromonas gingivalis initiates a mesenchymal-like transition through ZEB1 in gingival epithelial cells. Cell Microbiol (2015) 18(6):844–58. doi: 10.1111/cmi.12554
159. Inaba H, Sugita H, Kuboniwa M, Iwai S, Hamada M, Noda T, et al. Porphyromonas gingivalis promotes invasion of oral squamous cell carcinoma through induction of proMMP9 and its activation. Cell Microbiol (2014) 16:131–45. doi: 10.1111/cmi.12211
160. Binder Gallimidi A, Fischman S, Revach B, Bulvik R, Maliutina A, Rubinstein AM, et al. Periodontal pathogens Porphyromonas gingivalis and Fusobacterium nucleatum promote tumor progression in an oral-specific chemical carcinogenesis model. Oncotarget (2015) 6:22613–23. doi: 10.18632/oncotarget.4209
161. Lafuente Ibáñez de Mendoza I, Maritxalar Mendia X, García de la Fuente AM, Quindós Andrés G, Aguirre Urizar JM. Role of Porphyromonas gingivalis in oral squamous cell carcinoma development: A systematic review. J Periodont Res (2020) 55:13–22. doi: 10.1111/jre.12691
162. Yamamura K, Baba Y, Nakagawa S, Mima K, Miyake K, Nakamura K, et al. Human Microbiome Fusobacterium Nucleatum in Esophageal Cancer Tissue Is Associated with Prognosis. Clin Cancer Res (2016) 22:5574–81. doi: 10.1158/1078-0432.CCR-16-1786
163. Abdulkareem AA, Shelton RM, Landini G, Cooper PR, Milward MR. Potential role of periodontal pathogens in compromising epithelial barrier function by inducing epithelial-mesenchymal transition. J Periodont Res (2018) 53:565–74. doi: 10.1111/jre.12546
164. Zhang S, Li C, Liu J, Geng F, Shi X, Li Q, et al. Fusobacterium nucleatum promotes epithelial-mesenchymal transiton through regulation of the lncRNA MIR4435-2HG/miR-296-5p/Akt2/SNAI1 signaling pathway. FEBS J (2020) 287(18):4032–47. doi: 10.1111/febs.15233
165. Booijink CCGM, Boekhorst J, Zoetendal EG, Smidt H, Kleerebezem M, de Vos WM. Metatranscriptome Analysis of the Human Fecal Microbiota Reveals Subject-Specific Expression Profiles, with Genes Encoding Proteins Involved in Carbohydrate Metabolism Being Dominantly Expressed. Appl Environ Microbiol (2010) 76:5533–40. doi: 10.1128/AEM.00502-10
166. Duran-Pinedo AE, Chen T, Teles R, Starr JR, Wang X, Krishnan K, et al. Community-wide transcriptome of the oral microbiome in subjects with and without periodontitis. ISME J (2014) 8:1659–72. doi: 10.1038/ismej.2014.23
167. Jorth P, Turner KH, Gumus P, Nizam N, Buduneli N, Whiteley M. Metatranscriptomics of the Human Oral Microbiome during Health and Disease. mBio (2014) 5:e01012–14. doi: 10.1128/mBio.01012-14
168. Yost S, Duran-Pinedo AE, Teles R, Krishnan K, Frias-Lopez J. Functional signatures of oral dysbiosis during periodontitis progression revealed by microbial metatranscriptome analysis. Genome Med (2015) 7:27. doi: 10.1186/s13073-015-0153-3
169. Yost S, Stashenko P, Choi Y, Kukuruzinska M, Genco CA, Salama A, et al. Increased virulence of the oral microbiome in oral squamous cell carcinoma revealed by metatranscriptome analyses. Int J Oral Sci (2018) 10:32. doi: 10.1038/s41368-018-0037-7
170. Ma W, Mao Q, Xia W, Dong G, Yu C, Jiang F. Gut Microbiota Shapes the Efficiency of Cancer Therapy. Front Microbiol (2019) 10:1050. doi: 10.3389/fmicb.2019.01050
171. Panebianco C, Andriulli A, Pazienza V. Pharmacomicrobiomics: exploiting the drug-microbiota interactions in anticancer therapies. Microbiome (2018) 6:92. doi: 10.1186/s40168-018-0483-7
172. Fessler J, Matson V, Gajewski TF. Exploring the emerging role of the microbiome in cancer immunotherapy. J Immunother Cancer (2019) 7:108. doi: 10.1186/s40425-019-0574-4
173. Cremonesi E, Governa V, Garzon JFG, Mele V, Amicarella F, Muraro MG, et al. Gut microbiota modulate T cell trafficking into human colorectal cancer. Gut (2018) 67:1984–94. doi: 10.1136/gutjnl-2016-313498
174. Iida N, Dzutsev A, Stewart CA, Smith L, Bouladoux N, Weingarten RA, et al. Commensal bacteria control cancer response to therapy by modulating the tumor microenvironment. Science (2013) 342:967–70. doi: 10.1126/science.1240527
175. Heshiki Y, Vazquez-Uribe R, Li J, Ni Y, Quainoo S, Imamovic L, et al. Predictable modulation of cancer treatment outcomes by the gut microbiota. Microbiome (2020) 8:28. doi: 10.1186/s40168-020-00811-2
176. Viaud S, Saccheri F, Mignot G, Yamazaki T, Daillère R, Hannani D, et al. The Intestinal Microbiota Modulates the Anticancer Immune Effects of Cyclophosphamide. Science (2013) 342:971–6. doi: 10.1126/science.1240537
177. Jenq RR, Taur Y, Devlin SM, Ponce DM, Goldberg JD, Ahr KF, et al. Intestinal Blautia Is Associated with Reduced Death from Graft-versus-Host Disease. Biol Blood Marrow Transplant (2015) 21:1373–83. doi: 10.1016/j.bbmt.2015.04.016
178. Peled JU, Devlin SM, Staffas A, Lumish M, Khanin R, Littmann ER, et al. Intestinal Microbiota and Relapse After Hematopoietic-Cell Transplantation. J Clin Oncol (2017) 35:1650–9. doi: 10.1200/JCO.2016.70.3348
179. Roy S, Trinchieri G. Microbiota: a key orchestrator of cancer therapy. Nat Rev Cancer (2017) 17:271–85. doi: 10.1038/nrc.2017.13
180. Kroemer G, Galluzzi L, Kepp O, Zitvogel L. Immunogenic cell death in cancer therapy. Annu Rev Immunol (2013) 31:51–72. doi: 10.1146/annurev-immunol-032712-100008
181. Touchefeu Y, Montassier E, Nieman K, Gastinne T, Potel G, Bruley des Varannes S, et al. Systematic review: the role of the gut microbiota in chemotherapy- or radiation-induced gastrointestinal mucositis - current evidence and potential clinical applications. Aliment Pharmacol Ther (2014) 40:409–21. doi: 10.1111/apt.12878
182. Barker HE, Paget JTE, Khan AA, Harrington KJ. The tumour microenvironment after radiotherapy: mechanisms of resistance and recurrence. Nat Rev Cancer (2015) 15:409–25. doi: 10.1038/nrc3958
183. Crawford PA, Gordon JI. Microbial regulation of intestinal radiosensitivity. Proc Natl Acad Sci U S A (2005) 102:13254–9. doi: 10.1073/pnas.0504830102
184. Holohan C, Van Schaeybroeck S, Longley DB, Johnston PG. Cancer drug resistance: an evolving paradigm. Nat Rev Cancer (2013) 13:714–26. doi: 10.1038/nrc3599
185. Burt RK, Balabanov R, Burman J, Sharrack B, Snowden JA, Oliveira MC, et al. Effect of Nonmyeloablative Hematopoietic Stem Cell Transplantation vs Continued Disease-Modifying Therapy on Disease Progression in Patients With Relapsing-Remitting Multiple Sclerosis: A Randomized Clinical Trial. JAMA (2019) 321:165–74. doi: 10.1001/jama.2018.18743
186. Nilsson MS, Hallner A, Brune M, Nilsson S, Thorén FB, Martner A, et al. Complete remission after the first cycle of induction chemotherapy determines the clinical efficacy of relapse-preventive immunotherapy in acute myeloid leukaemia. Br J Haematol (2020) 188:e49–53. doi: 10.1111/bjh.16320
187. Bruna R, Benedetti F, Boccomini C, Patti C, Barbui AM, Pulsoni A, et al. Prolonged survival in the absence of disease-recurrence in advanced-stage follicular lymphoma following chemo-immunotherapy: 13-year update of the prospective, multicenter randomized GITMO-IIL trial. Haematologica (2019) 104:2241–8. doi: 10.3324/haematol.2018.209932
188. Haddad R, Concha-Benavente F, Blumenschein G, Fayette J, Guigay J, Colevas AD, et al. Nivolumab treatment beyond RECIST-defined progression in recurrent or metastatic squamous cell carcinoma of the head and neck in CheckMate 141: A subgroup analysis of a randomized phase 3 clinical trial. Cancer (2019) 125:3208–18. doi: 10.1002/cncr.32190
189. Paz-Ares L, Dvorkin M, Chen Y, Reinmuth N, Hotta K, Trukhin D, et al. Durvalumab plus platinum-etoposide versus platinum-etoposide in first-line treatment of extensive-stage small-cell lung cancer (CASPIAN): a randomised, controlled, open-label, phase 3 trial. Lancet (2019) 394:1929–39. doi: 10.1016/S0140-6736(19)32222-6
190. Nixon AB, Schalper KA, Jacobs I, Potluri S, Wang I-M, Fleener C. Peripheral immune-based biomarkers in cancer immunotherapy: can we realize their predictive potential? J Immunother Cancer (2019) 7:325. doi: 10.1186/s40425-019-0799-2
191. Wilky BA. Immune checkpoint inhibitors: The linchpins of modern immunotherapy. Immunol Rev (2019) 290:6–23. doi: 10.1111/imr.12766
192. Marcus L, Lemery SJ, Keegan P, Pazdur R. FDA Approval Summary: Pembrolizumab for the Treatment of Microsatellite Instability-High Solid Tumors. Clin Cancer Res (2019) 25:3753–8. doi: 10.1158/1078-0432.CCR-18-4070
193. Vétizou M, Pitt JM, Daillère R, Lepage P, Waldschmitt N, Flament C, et al. Anticancer immunotherapy by CTLA-4 blockade relies on the gut microbiota. Science (2015) 350(6264):1079–84.
194. Chaput N, Lepage P, Coutzac C, Soularue E, Le Roux K, Monot C, et al. Baseline gut microbiota predicts clinical response and colitis in metastatic melanoma patients treated with ipilimumab. Ann Oncol (2017) 28:1368–79. doi: 10.1093/annonc/mdx108
197. Sivan A, Corrales L, Hubert N, Williams JB, Aquino-Michaels K, Earley ZM, et al. Commensal Bifidobacterium promotes antitumor immunity and facilitates anti-PD-L1 efficacy. Science (2015) 350:1084–9. doi: 10.1126/science.aac4255
195. Matson V, Fessler J, Bao R, Chongsuwat T, Zha Y, Alegre M-L, et al. The commensal microbiome is associated with anti–PD-1 efficacy in metastatic melanoma patients. Science (2018) 359(6371):104–8.
196. Routy B, Le Chatelier E, Derosa L, Duong CPM, Alou MT, Daillère R, et al. Gut microbiome influences efficacy of PD-1-based immunotherapy against epithelial tumors. Science (2018) 359:91–7. doi: 10.1126/science.aan3706
198. Naidoo J, Page DB, Li BT, Connell LC, Schindler K, Lacouture ME, et al. Toxicities of the anti-PD-1 and anti-PD-L1 immune checkpoint antibodies. Ann Oncol (2015) 26:2375–91. doi: 10.1093/annonc/mdv383
199. Tanoue T, Morita S, Plichta DR, Skelly AN, Suda W, Sugiura Y, et al. A defined commensal consortium elicits CD8 T cells and anti-cancer immunity. Nature (2019) 565:600–5. doi: 10.1038/s41586-019-0878-z
200. Gopalakrishnan V, Helmink BA, Spencer CN, Reuben A, Wargo JA. The influence of the gut microbiome on cancer, immunity, and cancer immunotherapy. Cancer Cell (2018) 33:570–80. doi: 10.1016/j.ccell.2018.03.015
201. Fong W, Li Q, Yu J. Gut microbiota modulation: a novel strategy for prevention and treatment of colorectal cancer. Oncogene (2020) 39:4925–43. doi: 10.1038/s41388-020-1341-1
202. Dubin K, Callahan MK, Ren B, Khanin R, Viale A, Ling L, et al. Intestinal microbiome analyses identify melanoma patients at risk for checkpoint-blockade-induced colitis. Nat Commun (2016) 7:10391. doi: 10.1038/ncomms10391
203. Yu T, Guo F, Yu Y, Sun T, Ma D, Han J, et al. Fusobacterium nucleatum Promotes Chemoresistance to Colorectal Cancer by Modulating Autophagy. Cell (2017) 170:548–563.e16. doi: 10.1016/j.cell.2017.07.008
204. Pawelek JM, Low KB, Bermudes D. Bacteria as tumour-targeting vectors. Lancet Oncol (2003) 4:548–56. doi: 10.1016/s1470-2045(03)01194-x
206. Coley WB II. Contribution to the Knowledge of Sarcoma. Ann Surg (1891) 14:199–220. doi: 10.1097/00000658-189112000-00015
207. McCarthy EF. The Toxins of William B. Coley and the Treatment of Bone and Soft-Tissue Sarcomas. Iowa Orthop J (2006) 26:154–8.
208. Mengesha A, Dubois L, Chiu RK, Paesmans K, Wouters BG, Lambin P, et al. Potential and limitations of bacterial-mediated cancer therapy. Front Biosci (2007) 12:3880–91. doi: 10.2741/2357
209. Nallar SC, Xu D-Q, Kalvakolanu DV. Bacteria and genetically modified bacteria as cancer therapeutics: Current advances and challenges. Cytokine (2017) 89:160–72. doi: 10.1016/j.cyto.2016.01.002
210. Yazawa K, Fujimori M, Amano J, Kano Y, Taniguchi S. Bifidobacterium longum as a delivery system for cancer gene therapy: selective localization and growth in hypoxic tumors. Cancer Gene Ther (2000) 7:269–74. doi: 10.1038/sj.cgt.7700122
211. Parker RC, Plummer HC. Effect of histolyticus infection and toxin on transplantable mouse tumors. Proc Soc Exp Biol Med (1947) 66:461–7. doi: 10.3181/00379727-66-16124
212. Moese JR, Moese G. Oncolysis by clostridia. I. Activity of clostridium butyricum (m-55) and other nonpathogenic clostridia against the ehrlich carcinoma. Cancer Res (1964) 24:212–6.
213. Theys J, Landuyt W, Nuyts S, Van Mellaert L, van Oosterom A, Lambin P, et al. Specific targeting of cytosine deaminase to solid tumors by engineered Clostridium acetobutylicum. Cancer Gene Ther (2001) 8:294–7. doi: 10.1038/sj.cgt.7700303
214. Guo Y, Chen Y, Liu X, Min J-J, Tan W, Zheng JH. Targeted cancer immunotherapy with genetically engineered oncolytic Salmonella typhimurium. Cancer Lett (2020) 469:102–10. doi: 10.1016/j.canlet.2019.10.033
215. Liang K, Liu Q, Li P, Luo H, Wang H, Kong Q. Genetically engineered Salmonella Typhimurium: Recent advances in cancer therapy. Cancer Lett (2019) 448:168–81. doi: 10.1016/j.canlet.2019.01.037
216. Cunningham C, Nemunaitis J. A phase I trial of genetically modified Salmonella typhimurium expressing cytosine deaminase (TAPET-CD, VNP20029) administered by intratumoral injection in combination with 5-fluorocytosine for patients with advanced or metastatic cancer. Protocol no: CL-017. Version: April 9, 2001. Human Gene Therap (2001) 12(2):1594–6. doi: 10.1371/journal.pone.0209153
217. Chen W, Wang Y, Qin M, Zhang X, Zhang Z, Sun X, et al. Bacteria-Driven Hypoxia Targeting for Combined Biotherapy and Photothermal Therapy. ACS Nano (2018) 12:5995–6005. doi: 10.1021/acsnano.8b02235
218. Dai Z, Zhang J, Wu Q, Fang H, Shi C, Li Z, et al. Intestinal microbiota: a new force in cancer immunotherapy. Cell Commun Signal (2020) 18:90. doi: 10.1186/s12964-020-00599-6
219. Zebertavage L, Bambina S, Shugart J, Alice A, Zens KD, Lauer P, et al. A microbial-based cancer vaccine for induction of EGFRvIII-specific CD8+ T cells and anti-tumor immunity. PLoS One (2019) 14:e0209153. doi: 10.1371/journal.pone.0209153
220. D’Haens GR, Jobin C. Fecal Microbial Transplantation for Diseases Beyond Recurrent Clostridium Difficile Infection. Gastroenterology (2019) 157:624–36. doi: 10.1053/j.gastro.2019.04.053
221. Kitamoto S, Nagao-Kitamoto H, Hein R, Schmidt TM, Kamada N. The Bacterial Connection between the Oral Cavity and the Gut Diseases. J Dent Res (2020) 99:1021–9. doi: 10.1177/0022034520924633
Keywords: oral microbiome, cancer, oral squamous cell carcinoma, Fusobacterium nucleatum, Porphyromonas gingivalis, immunotherapy, bacteria-mediated tumor therapy
Citation: Irfan M, Delgado RZR and Frias-Lopez J (2020) The Oral Microbiome and Cancer. Front. Immunol. 11:591088. doi: 10.3389/fimmu.2020.591088
Received: 03 August 2020; Accepted: 29 September 2020;
Published: 23 October 2020.
Edited by:
Marcelo Freire, J. Craig Venter Institute (La Jolla), United StatesReviewed by:
Mohammad Samiul Alam, United States Food and Drug Administration, United StatesYu L. Lei, University of Michigan, United States
Copyright © 2020 Irfan, Delgado and Frias-Lopez. This is an open-access article distributed under the terms of the Creative Commons Attribution License (CC BY). The use, distribution or reproduction in other forums is permitted, provided the original author(s) and the copyright owner(s) are credited and that the original publication in this journal is cited, in accordance with accepted academic practice. No use, distribution or reproduction is permitted which does not comply with these terms.
*Correspondence: Jorge Frias-Lopez, amZyaWFzLWxvcGV6QGRlbnRhbC51ZmwuZWR1