- 1Centro de Investigação em Biodiversidade e Recursos Genéticos (CIBIO-UP), Centro de Investigação em Biodiversidade e Recursos Genéticos, InBIO, Laboratório Associado, Universidade do Porto, Vairão, Portugal
- 2Departamento de Biologia, Faculdade de Ciências, Universidade do Porto, Porto, Portugal
- 3Departments of Medicine, Microbiology, and Biochemistry & Molecular Genetics and the Comprehensive Cancer Center, University of Alabama at Birmingham, Birmingham, AL, United States
- 4CITS - Centro de Investigação em Tecnologias de Saúde, Cooperativa de Ensino Superior Politécnico e Universitário, CRL (CESPU), Gandra, Portugal
Fc receptor-like (FCRL) molecules comprise a large family of receptors, homologous to the receptors for the Fc portion of immunoglobulins (FCR). Within this family, an unusual gene known to exist in mice, rats and dogs, termed FCRLS, encodes a chimeric protein with both Ig-like FCRL and type B scavenger-receptor cysteine-rich (SRCR)-like domains. In mice, FCRLS is located next to the CD5L and KIRREL1 genes. Here, we show that the curious FCRLS gene is actually present across major mammalian groups, but its annotation is generally incorrect or absent. Anchored on mouse FCRLS and FCRL2 genomic sequence alignments, phylogenetic analyses demonstrated that many mammalian sequences currently annotated as FCRL2 cluster with FCRLS, supported by a conserved genetic synteny among organisms. This analysis shows that FCRLS is present in Rodentia, some Carnivora (Canidae and Ursidae), Chiroptera, Arctiodactyla, Proboscidae, and some Primata. Thus, the FCRLS most likely originated in a eutherian mammal ancestor since it is not present in Monotremata or Marsupialia. FCRLS has a peculiar distribution pattern across mammalian lineages, being present in some species, but absent in others from the same family, as in carnivores for example. The most parsimonious hypothesis to explain this FCRLS evolution is that it was convergently lost in several independent mammalian lineages. Analyses of branch-specific nucleotide evolutionary rates, show that FCRL2 and FCRLS have similar ranges of rates across mammals, suggesting that both genes have crucial, but separate functions in the immune system. Bayesian estimates of evolutionary rates for FCRLS in mammalian lineages revealed that carnivores display the highest mutation rate after rodents. Additionally, positive diversifying selection was detected for both FCRL2 and FCRLS. Our results show that the presence of the FCRLS gene is older and more widespread across mammals than previously thought and appears to be functional, being under positive selection. Its precise physiologic role should thus be investigated.
Introduction
Members of the classical Fc receptor (FCR) family that bind IgG and IgE belong to the greater immunoglobulin superfamily and are responsible for preserving the complex balance between cellular and humoral immunity in higher vertebrates (1, 2). Mammals possess different classes of FCRs, which correlate with their genetic, molecular, and structural diversity as well as ligand affinity. Accordingly, the number of isotype-specific FCRs has increased throughout vertebrate evolution most likely in parallel with the expansion of Ig-isotypes (1, 3). The FCRL molecule family is related to an ancient multigene family that is suspected to be linked by a common ancestor to the classical FCRs. The relationship between the FCRs and FCRLs is evident in their genetic organization, similar composition and structure of encoded extracellular domains, and the use of cytoplasmic tyrosine-based signaling elements (4, 5). In addition to their structural similarities, in primates, such as macaques, orangutans, chimpanzees and humans, all of the classical FCRs and FCRL are located on chromosome 1, implicating their evolution from a common ancestor (1).
The FCRL family comprises genes encoding type I transmembrane proteins with various Ig-like extracellular domains, multiple isoforms and allelic variations, preferential B lineage expression, and autonomous or dual signaling properties, with most displaying immunoreceptor tyrosine-based activating (ITAM) and/or inhibitory (ITIM) motifs in their cytoplasmic tails (1, 2, 4). For example, FCRL2 encodes a transmembrane protein with four extracellular Ig-like domains and a cytoplasmic tail with ITIM and ITAM-like sequences that is expressed by memory B cells (6). Despite having a conserved sequence motif in one Ig domain subtype that suggests Ig-binding capability, these receptors do not appear to possess the same binding properties as the classical FCR (4). Instead, many of the ligands for these molecules have remained elusive (7). However, recent discoveries have demonstrated the ability of FCRL3 to bind secretory IgA (8), FCRL4 to bind IgA, FCRL5 to bind IgG, and FCRL6 to interact with MHC class II molecules (9–11). Eight different FCRL genes have been identified in mammalian genomes including FCRL1–6, FCRLA and FCRLB (5, 12). However, not all eight FCRL genes are simultaneously present in every species. From a functional standpoint, FCRL molecules appear to primarily dampen immune responses (1). This hypothesis is supported by an overrepresentation of ITIMs in their cytoplasmic tails and numerous studies that have shown an association between autoimmune disorders and genetic variation in FCRL genes, more specifically the involvement of FCRL3 polymorphisms in autoimmunity (1, 7, 13–16).
In contrast to the abundance of FCRL genes present in the human, canine, and elephant genomes, only five orthologues were identified in mice: FCRL1 and FCRL5 on chromosome 3 and FCRL6, FCRLA and FCRLB on chromosome 1 (1). Together with these genes, an atypical gene, known as FCRLS, was identified, which does not exist in humans, but is also present in the rat and dog genomes (1, 3). This gene encodes a mosaic protein that lacks a transmembrane-encoding segment and includes an exon encoding a type B scavenger receptor cysteine rich (type B-SRCR) domain. These features make FCRLS unique as an Ig-like/SRCR-B chimeric protein (3, 17). Scavenger receptors possess various biological functions, forming a structurally diverse and large family of proteins. Some of these SRCR proteins localize on the cell surface as transmembrane molecules and may bind multiple ligands that aid in the removal of non-self or altered-self molecules through adhesion, endocytosis, phagocytosis, and signal transduction (18, 19). Scavenger receptors are mainly expressed by myeloid cells, and Brown and Goldstein (20) were the first to report their activity in macrophages, when investigating the formation of lipid-laden macrophages in atherosclerotic plaques (18, 21). The FCRLS gene in mice is also highly expressed by microglial cells in non-hematopoietic organs. In fact, a recent gene expression and proteomic analysis identified FCRLS as a differentially expressed gene (DEG), that can discriminate central nervous system (CNS)-derived microglial cells from peripheral monocytes/macrophages (22–24). Validation of FCRLS as a translated protein that is involved in CNS-related pathology (glioma) further indicates its value as a distinguishing marker of monocyte populations and supports the premise that this gene has functional relevance in the immune system (22).
Proteins harboring scavenger receptor cysteine-rich (SRCR) motifs, more recently classified as class I scavenger receptors, constitute a group of scavenger receptors that engage in the development of the immune system, and regulate innate and adaptive immune responses (18, 25). Members of the SRCR typically possess one or multiple repeats of a cysteine-rich extracellular domain and can be membrane-bound or secreted (25). A member of this class is the CD5L, a soluble glycoprotein, also known as apoptosis inhibitor expressed by macrophages (AIM) due to its first identified function (26, 27). This protein is involved in a wide range of biological functions, and circulates in serum at relatively high concentrations. The main cellular source of CD5L is tissue macrophages and it has been implicated in various models of inflammatory diseases, such as systemic lupus erythematosus (26–28). CD5L possesses three scavenger domains very similar to the one present in FCRLS (the SRCR-like domain of FCRLS shows 62% amino acid identity with mouse AIM/CD5L) (17). Given that FCRLS is generally found immediately adjacent to the CD5L gene, has the opposite orientation of FCRL1 and FCRL5, and appears to have a chimeric genetic structure that encodes a soluble protein, it is likely that this gene has resulted from an ancient recombination event involving a member of the FCRL family and CD5L (4). A common feature preserved among the FCR and FCRLs is a split signal peptide encoded by two exons, with the second exon consistently constituting 21 bp, which gives rise to the second half of the leader sequence (2, 29–31). This characteristic 21 bp second exon is also present in FCRLS. While the CD5L gene also possesses a split signal peptide, in contrast to the FCR/FCRL genes and FCRLS, the second half of the signal peptide is encoded by a 35 bp exon (26). The similarities in the protein domains of FCRLS, FCRLs and CD5L support the hypothesis that recombination events may be responsible for the genetic makeup and origin of FCRLS (Figure 1).
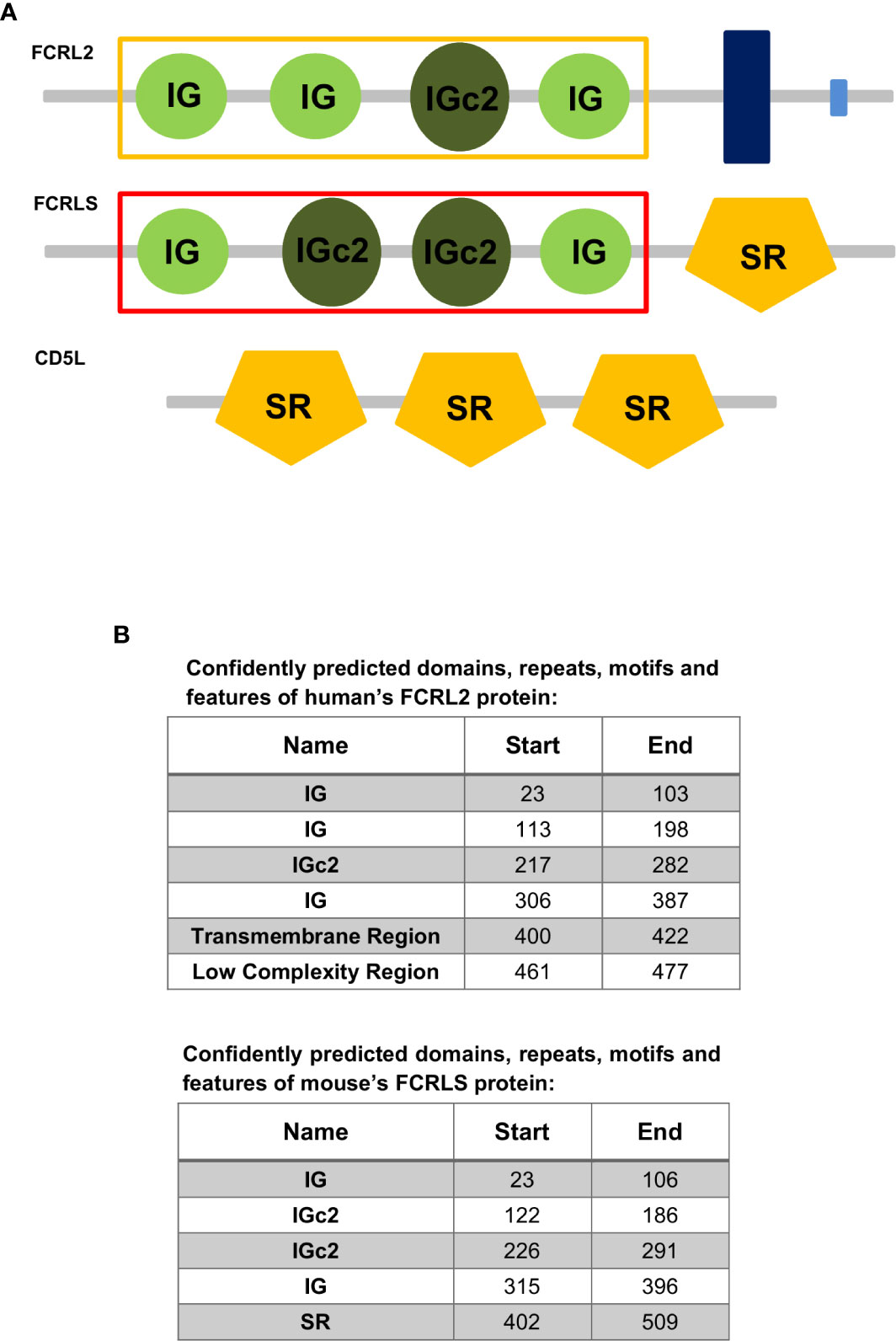
Figure 1 (A) Representation of the domain organization of the Fc receptor-like protein 2 (FCRL2) of Homo sapiens and the Fc receptor-like S, scavenger receptor (FCRLS) of Mus musculus and the CD5 molecule like (CD5L) of Homo sapiens. The yellow and red rectangles surrounding the IG and IGc2 domains signalize the domains used to construct the ML tree. IG, Immunoglobulin domain; IGc2, Immunoglobulin C-2 Type domain; SR, Scavenger receptor Cys-rich (SRCR) domain; Dark Blue Rectangle, Transmembrane region; Light Blue Rectangle, Low complexity region. (B) Confidently predicted domains, repeats, motifs and features of Fc receptor-like protein 2 (FCRL2) of Homo sapiens and the Fc receptor-like S, scavenger receptor (FCRLS) of Mus musculus (http://smart.embl-heidelberg.de/).
The FCRL family presents high genetic and structural diversity and a peculiar evolutionary history that requires further clarification. Here, we applied bioinformatics tools to gain a more comprehensive view of the distribution of these genes in a group of selected mammalian genomes. This analysis demonstrates that the FCRLS gene is actually present in most of major mammalian families and is either incorrectly annotated as FCRL2-like in most organisms or simply not annotated. These findings provide important new insights into the evolution of FCRLS.
Methods
Sequences
Publicly available gene sequences for mammalian genomes annotated as FCRL2, FCRL2-like or FCRLS were obtained through BLASTn (Standard Nucleotide BLAST) searches, using the Mus musculus, Rattus norvegicus, and Canis lupus familiaris FCRLS gene sequence and the Homo sapiens FCRL2 gene sequence as query. Searches were conducted in the NCBI’s GenBank (http://www.ncbi.nlm.nih.gov/genbank/) and Ensembl (https://www.ensembl.org/index.html) genome databases (32). Sequences that contained premature stop codons and/or frameshifting insertions or deletions were discarded from the analysis. The obtained sequences were aligned using ClustalW (33) in BioEdit (34) software and the resulting alignment was modified manually as needed as to respect reading frame correctness and exon boundaries. The resulting alignments are available as Supplementary Material (Supplementary Data 1). Subsequently, the identified gene sequences synteny was analyzed to ensure that all sequences were in fact orthologous genes. In total, we analyzed 81 sequences of 74 species, including representatives of Rodentia, Primata, Carnivora, Chiroptera, Artiodactyla, Lagomorpha, and Proboscidea (the respective accession numbers are given in Supplementary Table 1).
Phylogenetic Analysis
The phylogenetic relationships of the FCRL2 and FCRLS genes were analyzed in a Maximum Likelihood (ML) framework, using the GTR+G+I model of nucleotide substitution, in MEGA version X 10.1.6 software (35). Node support was estimated using 1000 bootstrap replicates of ML trees. FCRL3 sequences of five mammals’ were used to root the tree.
Genomic Synteny Analysis
To determine relative syntenic positions among genomes, gene loci were identified from the NCBI (https://www.ncbi.nlm.nih.gov/gene/) and Ensembl (https://www.ensembl.org/index.html) databases and the sizes of the genes, including the distance between the neighbouring genes, were calculated and used to construct a map in scale of the loci (support data given in Supplementary Table 2) using the approach described in (1, 36). For each species, we used the transcriptional variant with the same exons, when present, or the only sequence available.
Nucleotide Evolutionary Rates
The identified FCRLS and FCRL2 genes were separated into two distinct alignments, which were used to infer evolutionary rates with the Bayesian method implemented in BEAST v1.10.4 (37), under a strict molecular clock and under an uncorrelated lognormal relaxed clock (38). This relaxed clock model estimates the evolutionary rate for each branch of the tree, thus accounting for the possibility of extreme rate heterogeneity, while the strict model clock assumes constancy of evolutionary rates across branches. The analyses were calibrated setting normally distributed priors for the time of the most recent common ancestor, retrieved from the TimeTree database (39), of seven monophyletic clades for FCRLS (Boreotheria, 96 million years ago (Mya); Scrotifera, 79 Mya; Primatomorpha, 76 Mya; Rodentia, 73 Mya; Yangochiroptera, 53 Mya; Caniformia, 46 Mya; and Bovidae, 24.6 Mya) and eight monophyletic clades for FCRL2 (Boreotheria, 96 million Mya; Euarchonta, 82 Mya; Ferae, 75 Mya; Primata, 73.8 Mya; Caniformia, 46 Mya; Simiiformes, 43.2 Mya; Feliformia, 40 Mya; and Lemuroidea, 38 Mya), and a standard deviation of 2. Posterior probabilities were determined using Yule tree priors and GTR+G nucleotide substitution models. Independent runs of 10,000,000 generations were performed, and convergence was assessed using Tracer v1.7 (40). Final estimates were based on the combined results of three replicate runs, discarding the first 10% as burn-in. Posterior trees were summarized using TreeAnnotator v1.10.4, included in the BEAST v1.10.4 package. Marginal likelihood estimation (MLE), using the path sampling approach, available in BEAST, was performed to calculate Bayes Factors and determine which clock model (strict clock or uncorrelated lognormal relaxed clock) best fitted the data.
Codon-Based Analyses of Positive Diversifying Selection
To determine whether both genes, FCRLS and FCRL2, evolved under similar evolutionary regimes, we compared the rate per-site of nonsynonymous substitution (dN) to the rate per-site of synonymous substitutions (dS) for each gene separately in a maximum likelihood (ML) framework using three different methods. As each of the methods employs unique algorithms, and hence has advantages and drawbacks, we only considered those codons identified by a minimum of two of the ML methods as being positively selected codons (PSC) (41–43). Using MEGA version X 10.1.6 software (35), a Neighbor-joining (NJ) tree was obtained for each gene dataset to be used as the working topology, with the p-distance substitution model and the complete deletion option to handle gaps and missing data. Generally, the topology of the used trees reflected the accepted topology for mammals.
Two alternative models were compared in CODEML (PAMLX) (44, 45), one allowing codons to evolve under positive selection (dN/dS>1), M8, and one that does not (dN/dS ≤ 1), M7. The analyses were performed with the F3x4 model of codon frequencies and were run twice to guarantee congruous results. The models were compared using a likelihood ratio test (LRT) with 2 degrees of freedom (46, 47). For the M8 model, codons under positive selection were determined using a Bayes Empirical Bayes approach (BEB) (48) and considering a posterior probability of >90%.
We then used two methods for detecting positive selection accessible on the DataMonkey web server (49): the Random Effect Likelihood model (REL) and the Mixed Effects Model of Evolution (MEME). Both methods of analysis were run three times to ensure consistent results. The best fitting nucleotide substitution model was first determined by the automatic model selection tool available on the server.
To verify that recombination was not providing a false assumption of positive selection (50–53), we used the GARD method from the DataMonkey web server (49) to screen the datasets. The results did not show evidence of recombination.
Results
By screening 74 mammalian genomes, we identified multiple species where two annotated copies of the FCRL2 gene, that differed both in genome location and orientation, were apparently present. By aligning these two gene copies, differences among the genomic sequences suggested that the two FCRL2 gene copies were, in fact, two distinct genes. To test this hypothesis, we performed gene alignments, constructed phylogenetic trees, and performed evolutionary rate variation analyses.
Phylogenetic and Evolutionary Analysis
In this study, 81 genomic sequences for FCRLS-related genes, identified through BLASTn, were phylogenetically analyzed using a Maximum-Likelihood method (ML) and the GTR+G+I model of nucleotide substitution.
The obtained ML phylogenetic tree clearly supports two distinct groups, one clustering FCRL2 genes (n=43) and a second encompassing the rodent FCRLS genes (n=38) supported by bootstrap values of 99 and 100, respectively (Figure 2). Considering that the scavenger portion of the FCRLS and transmembrane region of FCRL2 sequences could not be properly aligned, we constructed the ML phylogenetic tree using only the Ig-like domains of FCRL2 and FCRLS.
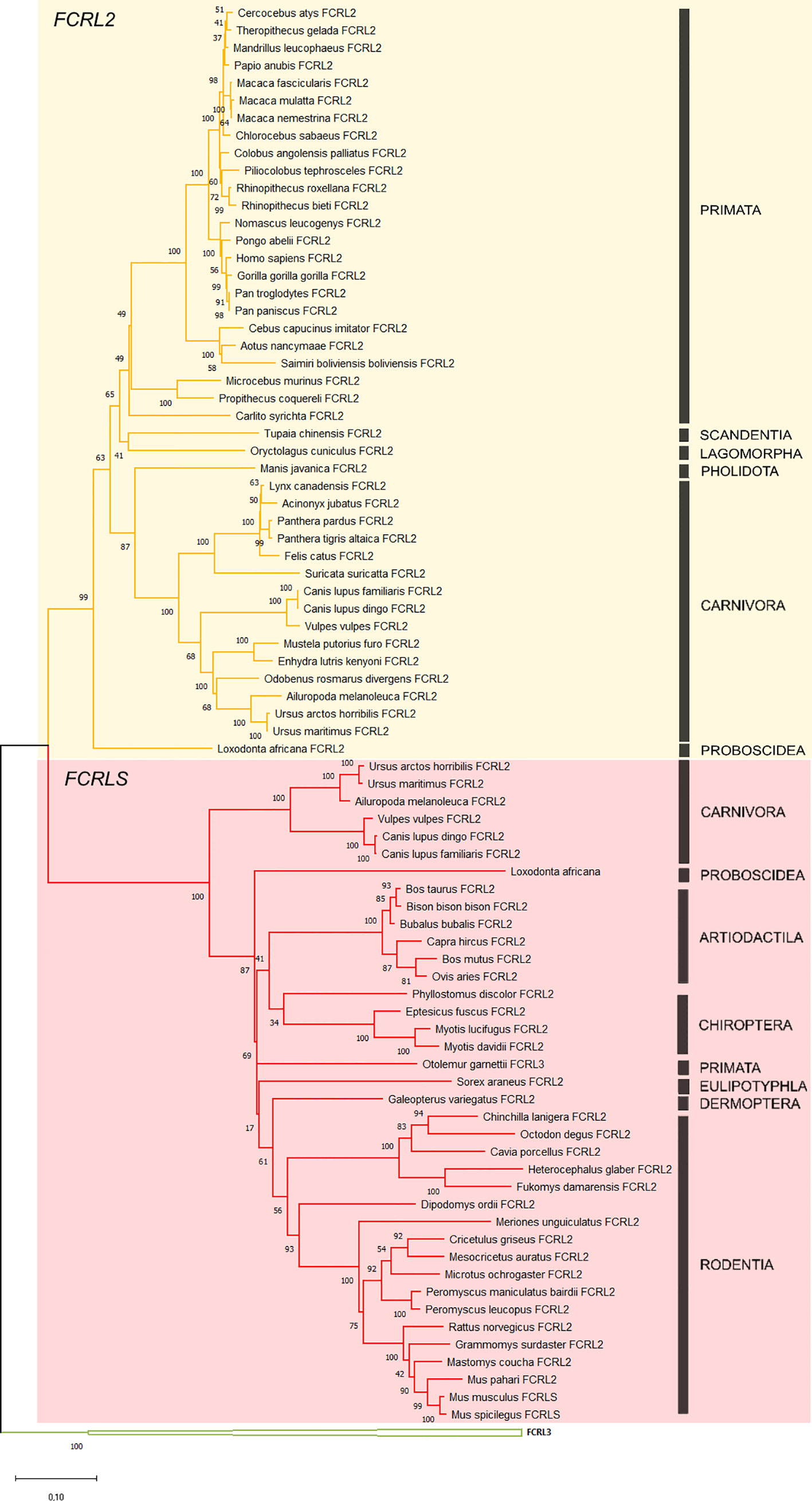
Figure 2 Phylogenetic tree of the FCRL2 and FCRLS genes. Maximum likelihood (ML) method and the GTR+G+I model of nucleotide substitution were used to assemble the phylogenetic tree. Some groups were collapsed for simplification. The tree is color-coded: in yellow are the FCRL2 branches (n=43), in red the FCRLS branches (n=38) and in green the FCRL3 branches that were used to root the tree. Bootstrap values are indicated to the left of each node. Black bars indicate mammalian orders.
The genes found to cluster with the rodent FCRLS (hence referred to as FCRLS) were mostly annotated as Fc Receptor-like 2 or Fc Receptor-like protein 2, but in Otolemur garnettii, FCRLS was actually annotated as Fc receptor-like protein 3. A deeper analysis of each gene sequence and its predicted encoded protein domains, revealed a resemblance between these genes and FCRL2, including shared similar Ig-like domains (Figure 1), which may explain their misannotation. The major difference in this cluster resides in the type B-SRCR scavenger portion of the genes, which is conserved in FCRLS sequences throughout species (76% and 68% of nucleotide and amino acid similarity, respectively)
With this analysis, we have identified the presence of FCRLS in Proboscidea, Arctiodactyla, Carnivora, Chiroptera, Primata, Dermoptera, and Eulipotyphla, confirming its presence in ancestral species such as Loxodonta africana. No significant blasts were obtained for the FCRLS gene in the Monotremata and Marsupialia genomes suggesting that this gene is not present in these groups. Interestingly, the FCRLS gene seems to be absent in several independent lineages. In Primata this gene is present in Otolemur garnettii, a Lorisiform, but is absent among most other primates. In Carnivora, this gene is present in Ursidae and Canidae families, but is absent in Felidae, Mustelidae, Odobenidae, and Herpestidae. We were also unable to find FCRLS for Oryctolagus cuniculus, the European rabbit, which is a Lagomorph, the sister group to Rodentia. Additionally, we searched for FCRLS remains in the scaffolds containing FCRLS flanking genes, KIRREL1 and CD5L, for the species for which we obtained no significant blasts. Overall, the coverage for these scaffolds is very good ranging 7x to 411x. In addition to this unusual evolutionary pattern, the carnivore FCRLS genes appear to cluster in a well-supported group (bootstrap value=93, Figure 2) at a basal position relative to other mammals.
Genomic Synteny Analysis
Of the 74 mammalian genomes analyzed here, 38 harbour the FCRLS gene (Figure 2). For most, the FCRLS gene is located between KIRREL1 and CD5L and is in the opposite orientation of the FCRL gene locus, revealing a shared synteny (Figure 3). Given that FCRLS generally shares the same orientation as KIRREL1, it is possible that these genes may have undergone an ancient translocation event. However, there are exceptions. For example, in Canidae, this gene has the same orientation as most mammals; yet, instead of being flanked by KIRREL1, FCRLS is located between CAPN8 and CD5L, both of which have the same orientation as the FCRL genes. The other exception observed is in Chiroptera, where the CD5L gene is lacking and there is generally a very limited repertoire of FCRL genes. When present, the FCRL genes typically have the same orientation as FCRLS and KIRREL1.
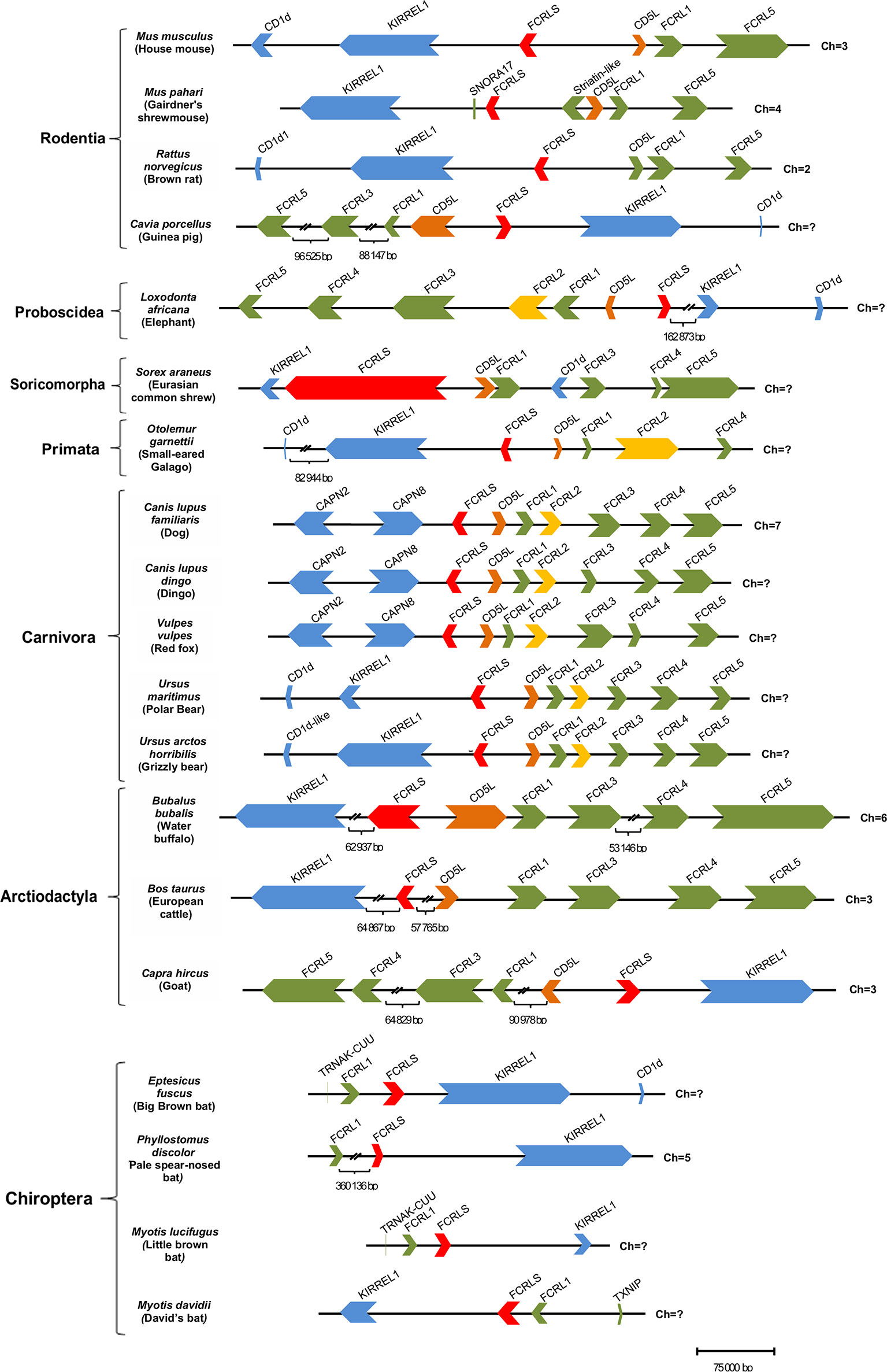
Figure 3 Structure of the FCRL gene family locus among a panel of mammalian genomes. Each horizontal line corresponds to the chromosome on which the FCRL and flanking genes are located. Genes are color-coded: FCRL genes are shown in green except FCRL2 that is in yellow, FCRLS is in red, CD5L is in orange, flanking genes on the opposite side of the FCRL cluster are in blue, and flanking genes next to the FCRL cluster are also depicted in green.
Further analysis of the genomic synteny, revealed that the Mus musculus FCRLS gene is composed of 8 exons, but the Rattus norvegicus FCRLS gene is composed of 7 exons. It also became clear that the FCRLS gene has the same approximate genetic length in most species with two exceptions. The first example is Sorex araneus (scaffold coverage of 120X) in which FCRLS, despite having the same number of exons as M. musculus, has large introns that yield the most expansive FCRLS genetic length among mammals. The second is Bubalus bubalis (scaffold coverage 62X) in which FCRLS is comprised of 10 exons, two more than in M. musculus, and has a large first intron.
Evolutionary Rates
The Bayes Factors (BF) showed decisive support against strict clock models in both FCRL2 and FCRLS (log10(BF) = 40 and 76 respectively), suggesting evolutionary rate variation across lineages. The mean nucleotide evolutionary rate estimated across all tree branches was slightly higher for FCRLS than for FCRL2 (2.22 x 10-3 and 1.90 x 10-3 substitutions/site/My, respectively) but with an overlap of the 95% high posterior distribution (HPD) intervals (2.02 x 10-3 – 2.42 x 10-3, and 1.73 x 10-3 – 2.06 x 10-3 substitutions/site/My for FCRLS and FCRL2 respectively). In FCRLS, higher rates were estimated for some Rodentia (e.g. 4.08 x 10-3 substitutions/site/My), Carnivora (4.29 x 10-3 substitutions/site/My) and Chiroptera (e.g. 3.43 x 10-3 substitutions/site/My) (Figure 4). Contrastingly, Artiodactyla showed the smaller evolutionary rates. In FCRL2, branches with high rates were estimated in Primata (e.g. 4.80 x 10-3 substitutions/site/My or 3.43 x 10-3 substitutions/site/My) (Figure 4).
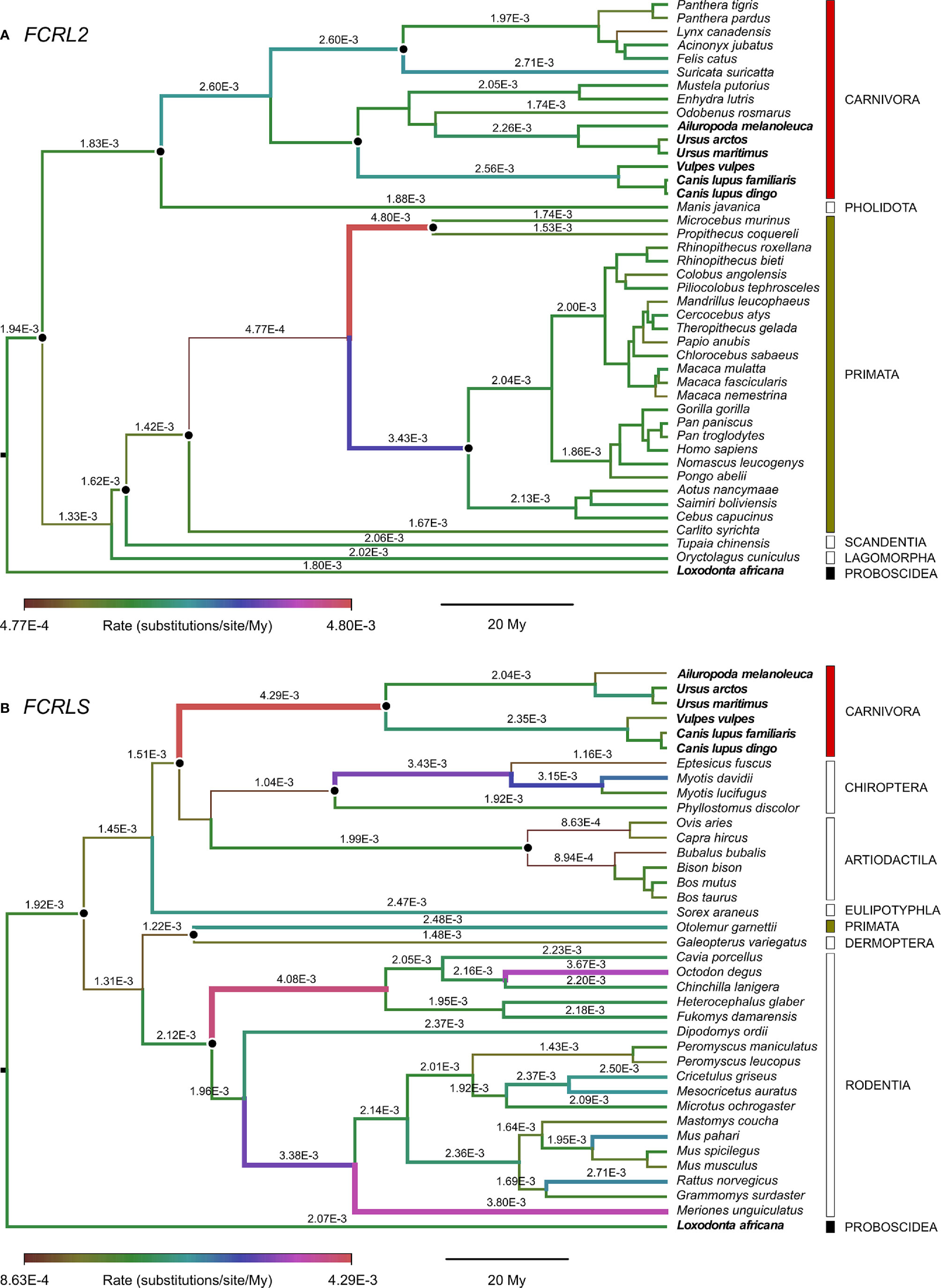
Figure 4 Branch-specific evolutionary rates (units of substitutions/site/My) for (A) FCRL2 (n=43) and (B) FCRLS (n=38) inferred using an uncorrelated lognormal relaxed clock implemented in Beast v1.10.4 and plotted with FigTree. The median of the high posterior density distribution is shown above branches; some rates were omitted for convenience of display. The thickness and colour of branches vary according to the inferred rate, and black dots indicate the calibrated nodes. Red, green and black bars indicate mammalian orders represented in both FCRL2 and FCRLS analyses, while empty bars depict orders with data from one of the genes. Bold species names indicate those present in both FCRL2 and FCRLS analyses.
Analyses of Positive Diversifying Selection
Through the comparison of the CODEML contrasting models, we found evidence of positive selection acting for both FCRL2 and FCRLS, with the model allowing sites to evolve under positive selection (M8) showing a significantly better fit than the model that did not (M7) for both genes (Table 1).
Comparing the sites identified by each of the three used methods, 26 PSCs were identified for FCRL2 and 12 PSCs were identified for FCRLS (Table 1). Positive selection seems to act with greater incidence in the first Ig domain of FCRL2, 19 of the 26 identified PSCs locate to this domain (Table 1). As for FCRLS, positive selection acts essentially in the first and last protein domains with 5 PSCs locating to the first Ig domain and 5 PSCs locating to the scavenger domain.
To understand the biological significance of the detected PSC, functional studies are required in order to establish the residues responsible for the interaction with ligands and to determine the three-dimensional structure of both proteins.
Discussion
From the phylogenetic results obtained, it is clear that what is commonly annotated as a second FCRL2 copy is actually FCRLS, a gene previously described only in the rodent and canine genomes (Figures 2 and 3). Our analyses of evolutionary variation rates along with the phylogenies of FCRL2 and FCRLS show a similar pattern of rate variation, strongly suggesting that like FCRL2, FCRLS is also a functional gene. The highest evolutionary rates for FCRLS were observed in rodent branches; however, our analyses also suggest that FCRLS is a fast-evolving gene in Carnivores. On the contrary, the lowest rates were found in Artiodactyla (Figure 4). These results suggest that distinct selective evolutionary pressures on FCRLS are at play and have governed the evolution of this gene among different groups of mammals, as is supported by the positive diversifying selection analysis (Table 1). Through these analyses, we uncovered strong positive selection to be acting for both genes, which further supports our hypothesis that both have crucial roles in immunity. Both genes display PSCs in the first Ig domain, which leads us to believe that this domain is particularly important in the function or conformation of the protein, but more studies will have to be conducted. For FCRLS, we also found PSCs in the scavenger domain that, once more, support our hypothesis that this domain is crucial for the protein function.
The synteny of the FCRLS locus is conserved across mammalian lineages, though some lineages show changes in this locus. This is especially the case in Chiroptera, which have lost most FCRL genes but retain FCRLS in the opposite orientation when compared to most mammals. Furthermore, most species retained either the FCRL2 gene or the FCRLS gene, with very few exceptions where both genes are present (Figure 5). It is unlikely that the loss of one of the genes could have eventually resulted from a functional redundancy of the two genes as FCRL2 and FCRLS display very distinct expression patterns. FCRL2 is known to be expressed as a type I transmembrane protein on human memory B cells (6), having significant expression the lymph node, spleen and appendix of human tissues (https://www.ncbi.nlm.nih.gov/gene/79368/?report=expression). FCRLS appears to encode a soluble protein that is expressed by microglial cells in mice (4, 22, 24) and has significant transcript expression in adult mouse tissues including mammary gland, subcutaneous fat pad, cerebral cortex, frontal lobe and bladder (https://www.ncbi.nlm.nih.gov/gene/80891/?report=expression). The expression in mouse brain tissues has been confirmed at the protein level (22, 24), leading us to believe that FCRLS plays an important role in the immune responses of the brain. Thus, these disparate protein structures and cellular distributions imply distinctly different functions for FCRL2 and FCRLS. In organisms lacking the FCRLS gene, its function could possibly be ensured by other scavenger receptors rather than the FCRL2 gene.
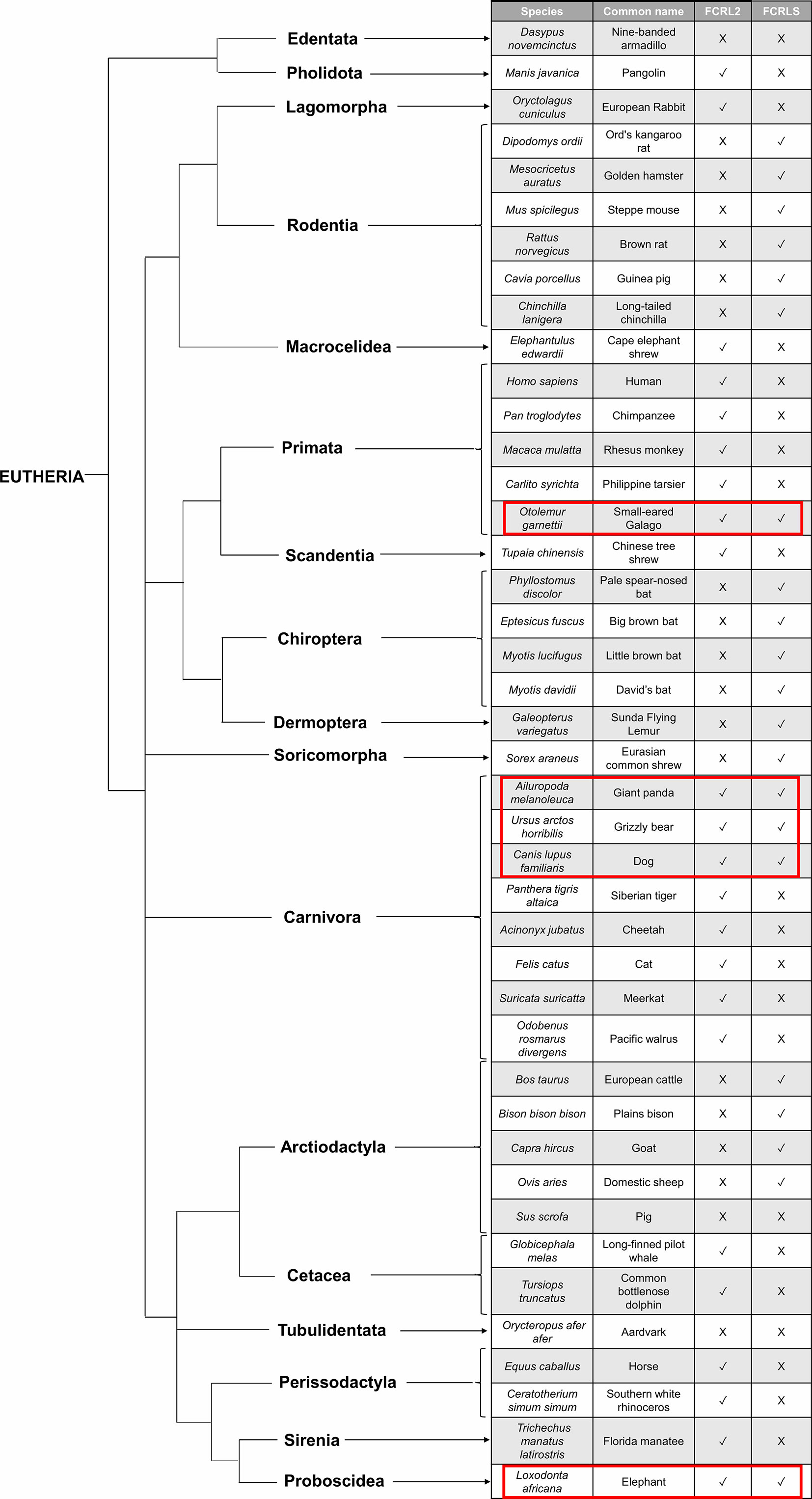
Figure 5 Species tree illustrating the evolutionary pattern of FCRLS and FCRL2. The red rectangles surround the species that retain the FCRL2 and the FCRLS gene.
The evolutionary scenario that seems to accommodate all of our observations is that FCRLS originated by recombination between a FCRL gene and a gene with a scavenger domain, in an eutherian mammal ancestor. The FCRLS gene is not present in Monotremata or Marsupialia, but is present in an ancient African mammal, the elephant (Loxodonta africana), indicating that the FCRLS gene appeared before the eutherian mammal diversification. The most accepted hypothesis for the emergence of FCRLS is that CD5L was involved in the recombination that originated the FCRLS gene (4), but we were unable to prove that, since our BLAST analysis of the scavenger portion of the FCRLS did not produce a significant match with a CD5L gene (divergence higher than 30%). This could be due to the accumulation of structural and conformational changes of this domain in order to make the FCRLS protein more efficient. Alternatively, the recombination donor gene with the scavenger domain was lost during the recombination process.
Interestingly, and despite having identified the FCRLS gene for representatives of most mammalian orders, this gene is not present in several independent lineages. In Primata this gene is present in Otolemur garnettii, a Lorisiform, but is absent among most other primates. This suggests that the gene was present in the Primata ancestral but was lost in the Lemuriformes (Lorisiformes sister group) at circa 60 million years ago and also in the Haplorrhini at circa 74 million years ago (39, 54). For Carnivora, this gene is present in Ursidae and Canidae families but is absent in Felidae, Mustelidae, Odobenidae, and Herpestidae, suggesting FCRLS was lost independently in these lineages. We were also unable to find FCRLS for Oryctolagus cuniculus, the European rabbit, which is a Lagomorph, the sister group to Rodentia that were separated at 65 million years ago (39). The most parsimonious hypothesis to accommodate this unusual evolutionary pattern is that the FCRLS emerged in an eutherian mammal ancestor and was then convergently lost in several independent mammalian lineages. An alternative scenario would involve the independent appearance of the FCRLS at least 3 times during mammalian evolution, which seems highly unlikely given the conserved gene structure and synteny observed among mammals.
At this point, it is unclear whether the loss reflected unnecessary redundant function or was instead accompanied by functional replacement from other genes. Regardless, the identification of FCRLS in many mammalian families’ hints at an important immunological function for this receptor, especially given its identification as a microglial DEG marker, which suggests an essential role in neurological immune responses.
Conclusion
The results obtained in this work show that the FCRLS gene was wrongly annotated as FCRL2 in the majority of the screened mammalian genomes. The FCRLS is most likely the result of a recombination between an FCRL gene and a scavenger domain gene. FCRLS is older than previously thought and it was already present before the Eutherian mammal’s radiation. It is present at least in Rodentia, some Carnivora (Canidae and Ursidae), Chiroptera, Arctiodactyla, Proboscidae and in a primitive primate, Otolemur garnettii. The FCRLS gene distribution pattern across all mammals is very unusual with the loss of this gene at different time points in three main mammalian groups: Primata, Carnivora and Lagomorpha. The variation in evolutionary rates and the identified PSCs across mammalian lineages and species suggests that distinct selective pressures governed the evolution of this gene, compatible with a functional role in immunity, which requires clarification. The FCRLS molecule has a SRCR-type B domain that may be indicative of its scavenger functions and properties. The broad distribution of this gene with indication of positive selection pressure opens new research avenues that should be explored by expression and functional studies.
Data Availability Statement
The original contributions presented in the study are included in the article/Supplementary Material. Further inquiries can be directed to the corresponding author.
Author Contributions
MM analyzed the data and wrote the manuscript. AP and JM-F analyzed and discussed the data. RD discussed the data. PE conceived the study. All authors contributed to the article and approved the submitted version.
Funding
This work was funded by national funds through FCT—Foundation for Science and Technology—under the project PTDC/BIA-OUT/29667/2017. FCT also supported the post-doctoral fellowships of AP (ref. SFRH/BPD/117451/2016), the FCT Investigator grant of PE (IF/00376/2015) and the Scientific Stimulus contract of JM-F (CEECIND/00372/2018). RD was funded in part by NIH/NIAID award AI110553.
Conflict of Interest
The authors declare that the research was conducted in the absence of any commercial or financial relationships that could be construed as a potential conflict of interest.
Supplementary Material
The Supplementary Material for this article can be found online at: https://www.frontiersin.org/articles/10.3389/fimmu.2020.590280/full#supplementary-material
References
1. Akula S, Mohammadamin S, Hellman L. Fc Receptors for Immunoglobulins and Their Appearance during Vertebrate Evolution. PLoS One (2014) 9(5):e96903. doi: 10.1371/journal.pone.0096903
2. Davis RS, Dennis G Jr, Odom MR, Gibson AW, Kimberly RP, Burrows PD, Cooper MD. Fc receptor homologs: newest members of a remarkably diverse Fc receptor gene family. Immunol Rev (2002) 190:123–36. doi: 10.1034/j.1600-065x.2002.19009.x
3. Fayngerts SA, Najakshin AM, Taranin AV. Species-specific evolution of the FcR family in endothermic vertebrates. Immunogenetics (2007) 59(6):493–506. doi: 10.1007/s00251-007-0208-8
4. Davis RS. Fc receptor-like molecules. Annu Rev Immunol (2007) 25:525–60. doi: 10.1146/annurev.immunol.25.022106.141541
5. Ehrhardt GR, Cooper MD. Immunoregulatory roles for fc receptor-like molecules. Curr Topics Microbiol Immunol (2011) 350:89–104. doi: 10.1007/82_2010_88
6. Jackson TA, Haga CL, Ehrhardt GR, Davis RS, Cooper MD. FcR-like 2 Inhibition of B cell receptor-mediated activation of B cells. J Immunol (Baltimore Md: 1950) (2010) 185(12):7405–12. doi: 10.4049/jimmunol.1002305
7. Tsubata T. Role of inhibitory BCR co-receptors in immunity. Infect Disord-Drug Targets (Formerly Curr Drug Targ-Infect Disord) (2012) 12(3):181–90. doi: 10.2174/187152612800564455
8. Agarwal S, Kraus Z, Dement-Brown J, Alabi O, Starost K, Tolnay M. Human Fc Receptor-like 3 Inhibits Regulatory T Cell Function and Binds Secretory IgA. Cell Rep (2020) 30(5):1292–99.e3. doi: 10.1016/j.celrep.2019.12.099
9. Wilson TJ, Fuchs A, Colonna M. Cutting edge: human FcRL4 and FcRL5 are receptors for IgA and IgG. J Immunol (Baltimore Md 1950) (2012) 188(10):4741–5. doi: 10.4049/jimmunol.1102651
10. Franco A, Damdinsuren B, Ise T, Dement-Brown J, Li H, Nagata S, et al. Human Fc receptor-like 5 binds intact IgG via mechanisms distinct from those of Fc receptors. J Immunol (Baltimore Md: 1950) (2013) 190(11):5739–46. doi: 10.4049/jimmunol.1202860
11. Schreeder DM, Cannon JP, Wu J, Li R, Shakhmatov MA, Davis RS. Cutting edge: FcR-like 6 is an MHC class II receptor. J Immunol (Baltimore Md 1950) (2010) 185(1):23–7. doi: 10.4049/jimmunol.1000832
12. Rivera J, Fierro NA, Olivera A, Suzuki R. New insights on mast cell activation via the high affinity receptor for IgE. Adv Immunol (2008) 98:85–120. doi: 10.1016/S0065-2776(08)00403-3
13. Li X, Gibson AW, Kimberly RP. Human FcR polymorphism and disease. Curr Topics Microbiol Immunol (2014) 382:275–302. doi: 10.1007/978-3-319-07911-0_13
14. Chistiakov DA, Chistiakov AP. Is FCRL3 a new general autoimmunity gene? Hum Immunol (2007) 68(5):375–83. doi: 10.1016/j.humimm.2007.01.013
15. Gibson AW, Li FJ, Wu J, Edberg JC, Su K, Cafardi J, et al. The FCRL3-169CT promoter single-nucleotide polymorphism, which is associated with systemic lupus erythematosus in a Japanese population, predicts expression of receptor protein on CD19+ B cells. Arthritis Rheum (2009) 60(11):3510–2. doi: 10.1002/art.24915
16. Chu X, Pan CM, Zhao SX, Liang J, Gao GQ, Zhang XM, et al. A genome-wide association study identifies two new risk loci for Graves’ disease. Nat Genet (2011) 43(9):897. doi: 10.1038/ng.898
17. Guselnikov SV, Ershova SA, Mechetina LV, Najakshin AM, Volkova OY, Alabyev BY, et al. A family of highly diverse human and mouse genes structurally links leukocyte FcR, gp42 and PECAM-1. Immunogenetics (2002) 54(2):87–95. doi: 10.1007/s00251-002-0436-x
18. PrabhuDas MR, Baldwin CL, Bollyky PL, Bowdish D, Drickamer K, Febbraio M, et al. A Consensus Definitive Classification of Scavenger Receptors and Their Roles in Health and Disease. J Immunol (Baltimore Md 1950) (2017) 198(10):3775–89. doi: 10.4049/jimmunol.1700373
19. Kunjathoor VV, Febbraio M, Podrez EA, Moore KJ, Andersson L, Koehn S, et al. Scavenger receptors class A-I/II and CD36 are the principal receptors responsible for the uptake of modified low density lipoprotein leading to lipid loading in macrophages. J Biol Chem (2002) 277(51):49982–8. doi: 10.1074/jbc.M209649200
20. Goldstein JL, Ho YK, Basu SK, Brown MS. Binding site on macrophages that mediates uptake and degradation of acetylated low density lipoprotein, producing massive cholesterol deposition. Proc Natl Acad Sci U S A (1979) 76(1):333–7. doi: 10.1073/pnas.76.1.333
21. Peiser L, Gordon S. The function of scavenger receptors expressed by macrophages and their role in the regulation of inflammation. Microbes Infect (2001) 3(2):149–59. doi: 10.1016/S1286-4579(00)01362-9
22. Haage V, Semtner M, Vidal RO, Hernandez DP, Pong WW, Chen Z, et al. Comprehensive gene expression meta-analysis identifies signature genes that distinguish microglia from peripheral monocytes/macrophages in health and glioma. Acta Neuropathol Commun (2019) 7:20. doi: 10.1186/s40478-019-0665-y
23. Stratoulias V, Venero JL, Tremblay MÈ., Joseph B. Microglial subtypes: diversity within the microglial community. EMBO J (2019) 38(17):e101997. doi: 10.15252/embj.2019101997
24. Hammond TR, Dufort C, Dissing-Olesen L, Giera S, Young A, Wysoker A, et al. Single-Cell RNA Sequencing of Microglia throughout the Mouse Lifespan and in the Injured Brain Reveals Complex Cell-State Changes. Immunity (2019) 50(1):253–71.e6. doi: 10.1016/j.immuni.2018.11.004
25. Sarrias MR, Padilla O, Monreal Y, Carrascal M, Abian J, Vives J, et al. Biochemical characterization of recombinant and circulating human Spα. Tissue Antigens (2004) 63(4):335–44. doi: 10.1111/j.0001-2815.2004.00193.x
26. Miyazaki T, Hirokami Y, Matsuhashi N, Takatsuka H, Naito M. Increased susceptibility of thymocytes to apoptosis in mice lacking AIM, a novel murine macrophage-derived soluble factor belonging to the scavenger receptor cysteine-rich domain superfamily. J Exp Med (1999) 189(2):413–22. doi: 10.1084/jem.189.2.413
27. Lai X, Xiang Y, Zou L, Li Y, Zhang L. Elevation of serum CD5L concentration is correlated with disease activity in patients with systemic lupus erythematosus. Int Immunopharmacol (2018) 63:311–6. doi: 10.1016/j.intimp.2018.07.022
28. Sanjurjo L, Aran G, Téllez É., Amézaga N, Armengol C, López D, et al. CD5L Promotes M2 Macrophage Polarization through Autophagy-Mediated Upregulation of ID3. Front Immunol (2018) 9:480. doi: 10.3389/fimmu.2018.00480
29. Qiu WQ, De Bruin D, Brownstein BH, Pearse R, Ravetch JV. Organization of the human and mouse low-affinity Fc gamma R genes: duplication and recombination. Science (1990) 248(4956):732–5. doi: 10.1126/science.2139735
30. Kulczycki A jr, Webber J, Soares HA, Onken MD, Thompson JA, Chaplin DD, et al. Genomic organization of mouse Fc gamma receptor genes. Proc Natl Acad Sci U S A (1990) 87(7):2856–60. doi: 10.1073/pnas.87.7.2856
31. Van de Winkel JG, Ernst LK, Anderson CL, Chiu IM. Gene organization of the human high affinity receptor for IgG, Fc gamma RI (CD64). Characterization and evidence for a second gene. J Biol Chem (1991) 266(20):13449–55. doi: 10.1016/S0021-9258(18)98860-5
32. Pinheiro A, Águeda-Pinto A, Melo-Ferreira J, Neves F, Abrantes J, Esteves PJ. Analysis of substitution rates showed that TLR5 is evolving at different rates among mammalian groups. BMC Evolutionary Biol (2019) 19(1):1–9. doi: 10.1186/s12862-019-1547-4
33. Thompson JD, Higgins DG, Gibson TJ. CLUSTAL W: improving the sensitivity of progressive multiple sequence alignment through sequence weighting, position-specific gap penalties and weight matrix choice. Nucleic Acids Res (1994) 22(22):4673–80. doi: 10.1093/nar/22.22.4673
34. Hall TA. BioEdit: a user-friendly biological sequence alignment editor and analysis program for Windows 95/98/NT. In Nucleic acids symposium series (Vol. 41, No. 41, pp. 95-98). London: Information Retrieval Ltd. (1999, January) p. c1979–2000.
35. Kumar S, Stecher G, Li M, Knyaz C, Tamura K. MEGA X: Molecular Evolutionary Genetics Analysis across Computing Platforms. Mol Biol Evol (2018) 35(6):1547–9. doi: 10.1093/molbev/msy096
36. de Sousa-Pereira P, Abrantes J, Pinheiro A, Colaço B, Vitorino R, Esteves PJ. Evolution of C, D and S-type cystatins in mammals: an extensive gene duplication in primates. PLoS One (2014) 9(10):e109050. doi: 10.1371/journal.pone.0109050
37. Drummond AJ, Rambaut A. BEAST: Bayesian evolutionary analysis by sampling trees. BMC Evolutionary Biol (2007) 7:214. doi: 10.1186/1471-2148-7-214
38. Drummond AJ, Ho SY, Phillips MJ, Rambaut A. Relaxed phylogenetics and dating with confidence. PLoS Biol (2006) 4(5):e88. doi: 10.1371/journal.pbio.0040088
39. Kumar S, Stecher G, Suleski M, Hedges SB. TimeTree: A Resource for Timelines, Timetrees, and Divergence Times. Mol Biol Evol (2017) 34(7):1812–9. doi: 10.1093/molbev/msx116
40. Rambaut A, Drummond AJ, Xie D, Baele G, Suchard MA. Posterior Summarization in Bayesian Phylogenetics Using Tracer 1.7. Syst Biol (2018) 67(5):901–4. doi: 10.1093/sysbio/syy032
41. Pinheiro A, Woof JM, Abi-Rached L, Parham P, Esteves PJ. Computational analyses of an evolutionary arms race between mammalian immunity mediated by immunoglobulin A and its subversion by bacterial pathogens. PLoS One (2013) 8(9):e73934. doi: 10.1371/journal.pone.0073934
42. Pinheiro A, Woof JM, Almeida T, Abrantes J, Alves PC, Gortázar C, et al. Leporid immunoglobulin G shows evidence of strong selective pressure on the hinge and CH3 domains. Open Biol (2014) 4(9):140088. doi: 10.1098/rsob.140088
43. Wlasiuk G, Nachman MW. Adaptation and constraint at Toll-like receptors in primates. Mol Biol Evol (2010) 27(9):2172–86. doi: 10.1093/molbev/msq104
44. Yang Z. PAML: a program package for phylogenetic analysis by maximum likelihood. Comput Appl Biosci (1997) 13(5):555–6. doi: 10.1093/bioinformatics/13.5.555
45. Yang Z. PAML 4: phylogenetic analysis by maximum likelihood. Mol Biol Evol (2007) 24(8):1586–91. doi: 10.1093/molbev/msm088
46. Nielsen R, Yang Z. Likelihood models for detecting positively selected amino acid sites and applications to the HIV-1 envelope gene. Genetics (1998) 148(3):929–36.
47. Yang Z, Swanson WJ, Vacquier VD. Maximum-likelihood analysis of molecular adaptation in abalone sperm lysin reveals variable selective pressures among lineages and sites. Mol Biol Evol (2000) 17(10):1446–55. doi: 10.1093/oxfordjournals.molbev.a026245
48. Yang Z, Wong WS, Nielsen R. Bayes empirical bayes inference of amino acid sites under positive selection. Mol Biol Evol (2005) 22(4):1107–18. doi: 10.1093/molbev/msi097
49. Pond SL, Frost SD. Datamonkey: rapid detection of selective pressure on individual sites of codon alignments. Bioinf (Oxford England) (2005) 21(10):2531–3. doi: 10.1093/bioinformatics/bti320
50. Anisimova M, Nielsen R, Yang Z. Effect of recombination on the accuracy of the likelihood method for detecting positive selection at amino acid sites. Genetics (2003) 164(3):1229–36.
51. Scheffler K, Martin DP. Cathal Seoighe, Robust inference of positive selection from recombining coding sequences. Bioinformatics (2006) Volume 22:2493–9. doi: 10.1093/bioinformatics/btl427
52. Shriner D, Nickle DC, Jensen MA, Mullins J II. Potential impact of recombination on sitewise approaches for detecting positive natural selection. Genet Res (2003) 81(2):115–21. doi: 10.1017/s0016672303006128
53. Kosakovsky Pond SL, Posada D, Gravenor MB, Woelk CH, Frost SD. Automated phylogenetic detection of recombination using a genetic algorithm. Mol Biol Evol (2006) 23(10):1891–901. doi: 10.1093/molbev/msl051
Keywords: FCR, FCRL, FCRLS, scavenger receptors, CD5L, evolution
Citation: Matos MC, Pinheiro A, Melo-Ferreira J, Davis RS and Esteves PJ (2021) Evolution of Fc Receptor-Like Scavenger in Mammals. Front. Immunol. 11:590280. doi: 10.3389/fimmu.2020.590280
Received: 31 July 2020; Accepted: 31 December 2020;
Published: 23 February 2021.
Edited by:
Jun Li, Lake Superior State University, United StatesReviewed by:
Federico Guillermo Hoffmann, Mississippi State University, United StatesJohn Anthony Hammond, Pirbright Institute, United Kingdom
Copyright © 2021 Matos, Pinheiro, Melo-Ferreira, Davis and Esteves. This is an open-access article distributed under the terms of the Creative Commons Attribution License (CC BY). The use, distribution or reproduction in other forums is permitted, provided the original author(s) and the copyright owner(s) are credited and that the original publication in this journal is cited, in accordance with accepted academic practice. No use, distribution or reproduction is permitted which does not comply with these terms.
*Correspondence: Pedro José Esteves, cGplc3RldmVzQGNpYmlvLnVwLnB0