- 1State Key Laboratory of Fine Chemicals, Dalian University of Technology, Dalian, China
- 2School of Chemical Engineering, Dalian University of Technology, Dalian, China
- 3National Engineering Research Center of Immunological Products, Department of Microbiology and Biochemical Pharmacy, College of Pharmacy and Laboratory Medicine, Third Military Medical University, Chongqing, China
- 4NCPC Genetech Biotechnology Co., Ltd., Shijiazhuang, China
- 5Basic Research Department, Shanghai Zerun Biotechnology Co., Ltd., Shanghai, China
Vaccine development utilizing various platforms is one of the strategies that has been proposed to address the coronavirus disease 2019 (COVID-19) pandemic. Adjuvants are critical components of both subunit and certain inactivated vaccines because they induce specific immune responses that are more robust and long-lasting. A review of the history of coronavirus vaccine development demonstrates that only a few adjuvants, including aluminum salts, emulsions, and TLR agonists, have been formulated for the severe acute respiratory syndrome-associated coronavirus (SARS-CoV), Middle East respiratory syndrome-related coronavirus (MERS-CoV), and currently the SARS-CoV-2 vaccines in experimental and pre-clinical studies. However, there is still a lack of evidence regarding the effects of the adjuvants tested in coronavirus vaccines. This paper presents an overview of adjuvants that have been formulated in reported coronavirus vaccine studies, which should assist with the design and selection of adjuvants with optimal efficacy and safety profiles for COVID-19 vaccines.
Introduction
Coronaviruses (CoVs) are single-stranded RNA viruses characterized by club-like spikes that can potentially cause severe respiratory disease in humans (1, 2). The outbreak of severe acute respiratory syndrome (SARS) caused by the SARS-CoV resulted in more than 8000 confirmed infections, with an overall case fatality rate of 10% in 2002 (3). The Middle East respiratory syndrome (MERS)-CoV continues to cause deaths with increasing geographical distribution and a 34.4% case fatality rate, according to the World Health Organization (WHO) (4). Most recently, the coronavirus disease 2019 (COVID-19) caused by SARS-CoV-2 has spread globally, with over 33 million confirmed cases as of October 2020 (5). Considering the challenges to global health systems and the far-reaching consequences on the world economy, there is an urgent need to develop effective and safe vaccines that can be quickly deployed on a global scale (2, 6).
Vaccine candidates are currently under development using different platforms, such as inactivated vaccines, recombinant protein vaccines, live-attenuated vaccines, viral vector (adenovirus) vaccines, DNA vaccines, and mRNA vaccines (2, 6, 7). Adenovirus-vector could induce potent immunological responses due to the presence of viral proteins and stimulation of innate immunity sensors, e.g., toll-like receptors (8). Nucleic-acid vaccines, e.g., DNA and mRNA vaccines, encode the virus’s spike protein, intrinsically could engage innate immunity that instructs induction of immune protection (9). However, these platforms haven’t been used in licensed human vaccines before. In other platforms, subunit or inactivated antigens were used, but these antigens lack the immunological profiles that mediate the enhanced adaptive immunity. Thus, in these CoV vaccines, they require the addition of adjuvants for directing the types and magnitude of immune responses (10). In previously reported exploratory and pre-clinical CoV vaccine studies, adjuvants such as aluminum salts, emulsions, and toll-like receptor (TLR) agonists, have been used in vaccine formulations for studies with various animal models (Table 1). The adjuvants AS03, MF59, and CpG 1018 have already been used in licensed vaccines (28) and have been committed by GlaxoSmithKline, Seqirus, and Dynavax to be available for COVID-19 vaccine development (29). When combined with subunit and specific inactivated antigens (30, 31), adjuvants with various characteristics elicit distinctive immunological profiles with regard to the direction, duration, and strength of immune responses. Thus far, there are at least 40 candidate vaccines in clinical trials and 149 vaccines in preclinical evaluation, of which 67 subunit and 15 inactivated COVID-19 vaccines have being developed (32). Among these adjuvants, alum have been formulated with S protein or RBD to induce neutralizing antibody production (17, 18), which has suggested to be associated with protection against SARS-CoV-2 (15, 16, 24). However, alum lacks the capability to promote the activation of CD4+ and CD8+ T cell responses, which has been demonstrated to coordinate with the antibody responses to provide protective immunity against the SARS-CoV-2 (33). Other adjuvants, e.g., emulsion adjuvants and TLR agonists, which have been shown to induce both humoral and cellular immune responses could be more favorable. However, no phase III clinical trial results of COVID-19 vaccines are published so far, thus, there is no direct evidence to indicate which type of immune response induced by vaccine plays a more critical protective role in SARS-CoV-2 infection. Knowing these uncertainties, an overview of previous CoV vaccine studies using different adjuvants would be indispensable for the design and development of a COVID-19 vaccine.
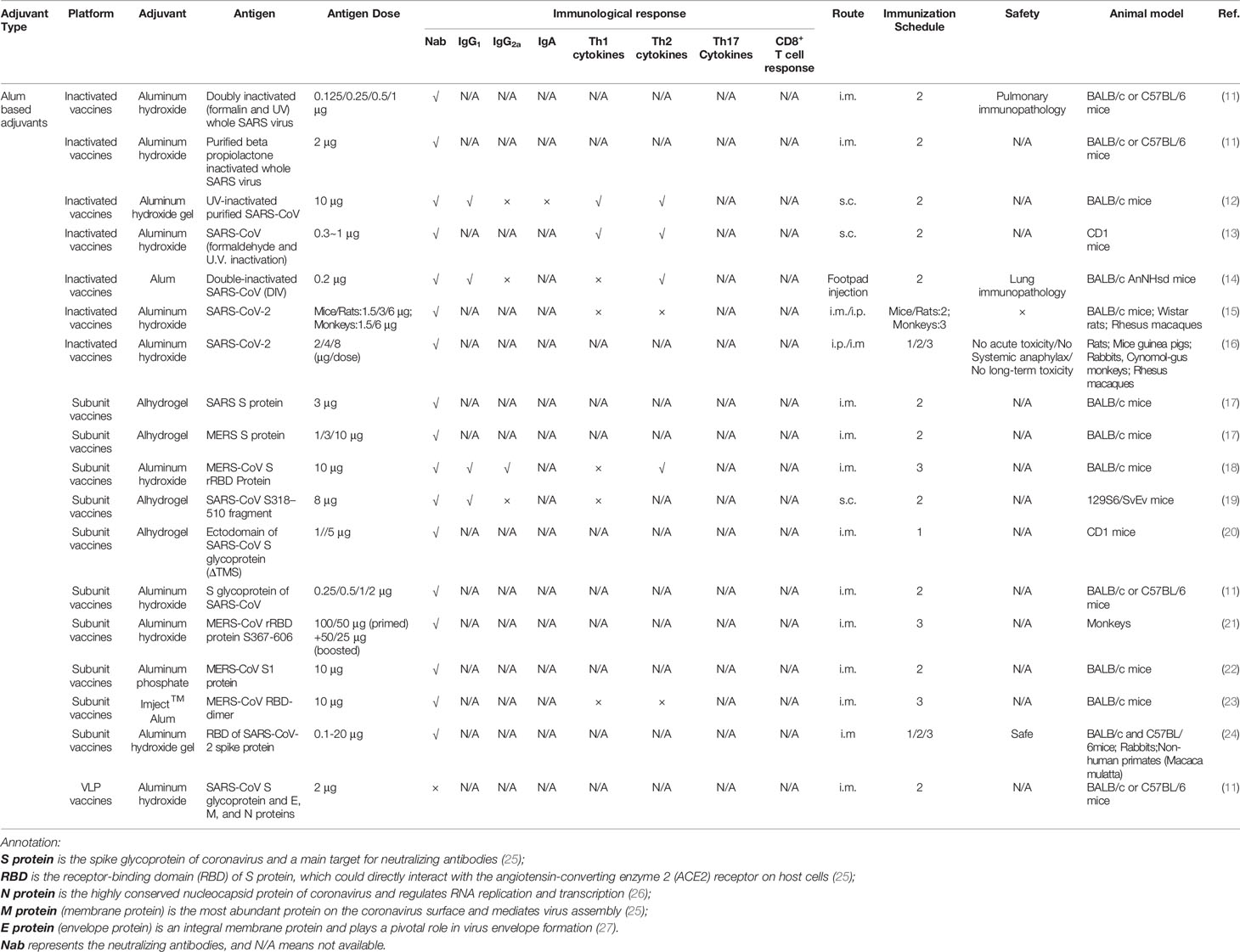
Table 1 Alum based adjuvants used in the coronavirus vaccine formulations under exploratory and pre-clinical investigations.
The SARS-CoV-2 is a novel strain of the coronavirus, and very little is known about its epidemiology and pathogenesis. Therefore, extreme cautions should be taken when considering vaccine formulations that can achieve the desired efficacy and safety profiles. The selection of adjuvants should consider the magnitude, affinity, isotype, and durability of antibodies that are critical for coronavirus vaccine developments (34). It should be noted that low antibody production may lead to antibody-dependent enhancement (ADE) manifested by severe liver damage and enhanced infection (35), while high affinity neutralizing antibodies could help to avoid ADE. Additionally, the proper application of adjuvants also depends on the choice of antigens. The full-length S protein is more likely to trigger ADE due to mild antibody production (36). In comparison, the N protein is generally highly conserved, and it is associated with the ability to induce cytotoxic T lymphocytes (CTL). However, N protein could potentiate pro-inflammatory cytokine production and lead to severe lung pathology (37). In addition, previous study on respiratory syncytial virus (RSV) vaccine also indicated that immunization with whole inactivated virus could lead to vaccine-associated enhanced respiratory disease (VAERD), manifested by allergic inflammation and Th2 type immune responses (38). Altogether, these studies suggest that vaccines formulated with various antigen isotypes may require proper adjuvant selection to achieve the desired immune protection. In this paper, we reviewed adjuvants that have already been incorporated in the coronavirus vaccines under exploratory and pre-clinical investigations. By reviewing the vaccine formulations and the types of immune responses that were induced, we provide information that will enable proper adjuvant selection for COVID-19 vaccines to facilitate rapid vaccine delivery.
Aluminum Salt-Based Adjuvants
Aluminum salt-based adjuvants (alum) were the first adjuvants used in licensed human vaccines. They are still the most widely used because of their wide-spectrum ability to strengthen immune responses and their excellent track record of safety (39–41). In limited coronavirus vaccine studies, it has been suggested that neutralizing antibody against the spike protein might be mechanistically correlated with immune protection (42). When alum was formulated with S protein or receptor-binding domain (RBD), it significantly enhanced humoral immune responses. This was demonstrated by higher titers of serum IgG1, increased high affinity viral neutralizing antibodies, and the generation of long-lasting memory B cells in mice (13, 17–19). Additionally, Alum was formulated with the inactivated and VLP vaccines containing E, M, and N proteins (11, 12, 14) (Table 1) that showed enhanced IgG1 and neutralizing antibody titers (14) and prolonged durability (12). Studies also demonstrated that alum adjuvant plays an essential role in the dose-sparing of CoV vaccines. In a SARS S protein subunit vaccine, the alum-adjuvanted S protein (1 μg) group showed neutralizing antibody titers similar to or higher than the non-adjuvanted S protein (50 μg) group. The alum-adjuvanted S protein (5 μg) group showed a geometric mean titer (GMT) twice as high as the non-adjuvanted S protein (50 μg) group (20). It should also be noted that different types of alum were selected in the studies, including Alhydrogel, which is chemically crystalline aluminum oxyhydroxide (43), aluminum hydroxide (11), aluminum phosphate (22), and Imject™ Alum (23), which is a mixture of aluminum hydroxide and magnesium hydroxide. Even though there is no specific description regarding the aluminum hydroxide in reported literature (11, 18, 21), it can also be referred to Aluminum oxyhydroxide (44). However, these studies lacked systematic comparisons with regards to their adjuvanticity and how various alum-based adjuvants differed in their ability to induce neutralizing antibodies.
It is worth noting that inactivated SARS-CoV or S protein-based vaccines are associated with Th2-type immunopathology, which is characterized by an increase in eosinophils and inflammatory infiltrates (14, 30, 37, 45). Moreover, the addition of alum adjuvant exacerbated the immunopathologic reactions (14, 45). In alum-adjuvanted SARS-CoV double-inactivated vaccine (DIV), there was a skew in the N or S protein-specific antibodies toward IgG1, when compared with the more balanced antibody production in the nonadjuvanted DIV vaccine (14). These observations raise significant concerns regarding the safety of adjuvanted coronavirus vaccines. On the other hand, it has been shown that alum can reduce immunopathology in SARS-CoV vaccines containing either a double-inactivated virus or S protein (11). Furthermore, in a recent study, a purified inactivated SARS-CoV-2 vaccine (PiCoVacc) adjuvanted with aluminum hydroxide conferred complete protection in non-human primates (rhesus macaques) with potent humoral responses but without lung immunopathology (15). This finding raises the question of the mechanism of eosinophilic immunopathology. While commonly thought of as the product of Th2 responses, recent studies have indicated that tissue eosinophilia can also be controlled by Th17 responses (46). Thus, the proper selection of CoV antigens and adjuvants that can shift host responses away from a Th17-bias appears to be critical. In addition, other studies have demonstrated that the Th2 immunopathology may be associated with SARS N or S protein that results in enhanced eosinophilic immunopathology (11, 37, 47). However, more studies are required, as the preliminary data is limited. Additionally, the Th-2–biased immune responses may raise the concern on vaccine-enhanced respiratory disease (VAERD) (38, 48), however, there are no evidences that alum-adjuvanted CoV vaccines show the effect.
When alum was used as an adjuvant in CoV vaccines (Table 1), there was a lack of Th1 CD4+ T cell and cytotoxic CD8+ T cell immune responses, which is typical for alum-adjuvanted vaccines (49). However, recent study has demonstrated that the SARS-CoV-2–specific adaptive immune response correlated with milder disease, indicating that coordinated CD4+ and CD8+ T cell responses play a synergistic effect in the protective immunity of COVID-19 (33). Several other adjuvants, which are capable of inducing more balanced Th1/Th2 or Th1-biased immune responses, have been formulated in CoV vaccines and will be discussed in the following sections.
Emulsion Adjuvants
The emulsion adjuvants, MF59, and AS03 have already been used in licensed human vaccines to improve the immunogenicity of the antigens (50, 51). Compared with alum that lacks the capability to mediate cell-mediated immunity (49), MF59 and AS03 can elicit more balanced immunity, possibly by improving antigen uptake, recruiting immune cells, and promoting the migration of activated antigen-presenting cells (28, 50, 52). Emulsion adjuvants have already been used in preclinical studies of vaccines against coronavirus. MF59 used in inactivated SARS and MERS vaccines, as well as vaccines containing the RBD domain of the MERS-CoV spike (S) protein, has exhibited excellent adjuvanticity, with potent humoral immune responses, i.e., high titers of neutralizing antibodies, and cell-mediated immunity in the coronavirus vaccines (53–55). In addition, depending on the types of antigen, cell-mediated immunity induced by MF59 differs. When formulated with the MERS-CoV S protein, MF59 enhanced both effective CD4+ and CD8+ T-cell immune responses. In comparison, when combined with inactivated SARS CoV, MF59 induced significant CD4+ T cell, but not CD8+ T cell responses (56, 57). However, in another study by Zhang et al., it was demonstrated that when MERS S protein was adjuvanted with MF59, it induced higher IgG1 and IgG2a antibodies with a slightly Th2-biased response (54). Subsequent studies also showed that ferritin-based MERS-CoV S protein, adjuvanted with MF59, promoted multiple antibody responses, including high levels of IgA antibody titers that resulted in potent mucosal immune responses (58). A study by Tang et al. has indicated that there are no significant differences in the neutralizing activity of the serum derived from mice immunized with MERS S377-588 at 1, 5, and 20 μg in the presence of MF59, suggesting the dose-sparing effect of MF59 when it was formulated with MERS S protein (57). However, an immunopathologic lung reaction, as well as an increase in IL-5 and IL-13 cytokines, was seen in animal studies using both MF59-adjuvanted and adjuvant-free inactivated MERS-CoV vaccines (53). It has shown that eosinophil infiltrations with higher Th2-type cytokine secretion aggravated the hypersensitivity-type pulmonary immunopathology when vaccinated with MF59-adjuvanted inactivated virus vaccines as compared with the inactivated virus vaccines alone (53).
Another emulsion adjuvant, AS03, elicits both potent humoral and cellular immune responses to an inactivated whole virion SARS-CoV (WI-SARS) vaccines (59) compared with the virion without adjuvants. Moreover, in the presence of the AS03 adjuvant, an identical trend toward specific CD4+ T cell responses was observed when immunized with SARS-CoV containing the equivalent of 0.5 or 1.5 μg of S protein (59). Therefore, the addition of AS03 tends to potentiate the immune responses with a lower dosage of antigen. Considering its capability to induce both arms of the immune system, S protein, RBD domain, and N protein can also be formulated with AS03. Currently, GSK is sharing its AS03 adjuvant with COVID-19 vaccine developers globally (29).
Besides MF59 and AS03, other emulsion-based adjuvants such as Freund’s adjuvant and Montanide ISA51 have also been formulated in CoV vaccines (54). By evaluating the titers of specific serum antibody responses, it has been demonstrated that Freund’s adjuvant and ISA51 elicited significant Th1 antibody responses (IgG2a) with no clear Th2 responses (IgG1) (54, 59).
TLR Agonists and Other Adjuvants
Toll-like receptors (TLRs), a category of pattern-recognition receptors, are critical to pathogen recognition. This allows for rapid activation of innate immunity, and subsequently, effective adaptive immunity. TLR agonists have been extensively studied as vaccine adjuvants (60, 61). CpG, Poly I:C, glucopyranosyl lipid A (GLA), and resiquimod (R848) are agonists for TLR9, TLR3, TLR4, and TLR7/8, respectively. These adjuvants have been evaluated in candidate vaccines against SARS CoV (62, 63).
In addition to neutralizing antibodies and CD4+ T cells, optimal protection against coronavirus probably involves the synergistic effect of CD8+ T cells (64). Memory CD8+ T cells solve the problem of neutralizing antibodies only existing for short periods and providing long-term protective cellular immunity (64). Among the TLR agonists, CpG significantly augments the CD8+ T cell immune response higher than the others (63). Indeed, it has been demonstrated that CpG can also stimulate enhanced IgG production in animals immunized with an inactivated SARS-CoV vaccine (62). In addition to IgG, IgA production was also enhanced, only when CpG was administered via intranasal (i.n.) administration (62), indicating immune activation in the mucosal compartment. Although CpG is capable of inducing both cellular and humoral immune responses, it preferentially induces responses that are Th1-biased. Moreover, CpG can divert pre-existing Th2 responses to a Th1 phenotype, which has laid a foundation for the combination of CpG with other adjuvants, most commonly alum (65). In SARS-CoV or MERS-CoV subunit vaccines, studies have found that the combination of alum and CpG elicited higher neutralization antibody titers and a more robust cellular immune response compared with alum alone or alum with other TLR agonists (18, 19). In addition to alum, CpG is combined with Montanide ISA-51, a type of water-in-oil emulsion adjuvant. When the combined adjuvants were formulated with SARS S or N protein, they were capable of promoting robust neutralizing antibody production (66). However, vaccinated with only SARS N protein, animals showed immune responses biased dramatically toward Th1 (67). In addition, it is reported that R848 could enhance antigen-specific CTL response and induce a fast, robust and durable IFN-α production in vivo among humanized mice, which is distinct from the experimental findings based on common mouse models (68). However, further studies on R848 adjuvanticity should stress more on vaccine formulation. A recent study by Gadd et al. indicated that only when R848 was conjugated with DOPE (1,2−di−(9Z−octadecenoyl)−sn−glycero−3−phosphoethanolamine):DDA (dimethyldioctadecylammonium bromide salt) multilamella liposomes rather than linear mixed, a high potency of immunostimulatory activity was observed (69). Moreover, an R848-encapsulating PLGA nanoparticle can bring down the excessive level of inflammatory cytokines induced by free R848, which could be benefit to provide long-term safety and appropriate immune response (70).
Although CpG had been shown to exhibit considerable potential as a coronavirus-specific adjuvant, studies have found that it might be a poor inducer of long-term immune memory (46). A recent study indicated that single-stranded RNAs (ssRNAs) derived from the Cricket paralysis virus (CrPV) intergenic region (IGR) internal ribosome entry sites (IRES) could function as vaccine adjuvants endowing long-lasting immunity. This adjuvant significantly activates innate immune response through activating TLR7 and enhancing the chemotaxis of professional antigen-presenting cells (APC) (71). Moreover, some novel adjuvants such as STING agonist, Advax, and AS01B, which is an adjuvant formulated in recombinant zoster vaccine Shingrix, exhibit advantages for long-lasting immune responses (46, 59, 72). Advax, a delta inulin microparticle adjuvant, augmented the induction of neutralizing antibodies along with the existence of memory B cells and a robust, long-lasting T-cell IFN-γ response when it was formulated in recombinant or inactivated SARS-CoV vaccines (46). Moreover, Matrix M1, a saponin-based adjuvant, has been demonstrated to be more effective than alum adjuvant in inducing neutralizing antibodies to SARS S protein or MERS S protein (17). This might address the concern that S protein may lead to antibody-dependent enhancement (ADE), which is more likely to be triggered by mild antibody production (36).
The SARS-CoV-2 infections occur at the mucosal surface of the upper respiratory tract (73). Thus, the elicitation of protective immune responses at the mucosa is critical. TLR agonists, such as flagellin (74) and CpG ODN (62), have been used as mucosal adjuvants. As discussed above, the CpG ODN can elicit neutralizing antibodies in mucosal compartments (62) when formulated with inactivated SARS-CoV. Additionally, the STING agonist, bis-(3′,5′)-cyclic dimeric guanosine monophosphate (c-di-GMP or cdGMP), has been reported as a potent mucosal vaccine adjuvant that induces Th1 and Th17 cytokines in a plant-derived H5 influenza vaccine after intranasal vaccination (75). In a very recent study, it was demonstrated that pulmonary surfactant–biomimetic liposomes encapsulating STING agonists could be used as mucosal adjuvants for universal influenza vaccines that trigger rapid humoral and cellular immune responses and exhibit sustained cross-protection against influenza (76). Though cdGMP in polymeric nanoparticle formulations has been used as adjuvants with MERS-CoV S-RBD protein, its ability to induce mucosal immunity was not specifically examined (72). Thus, further studies are warranted to examine both the efficacy and safety of mucosal adjuvants in coronavirus vaccines.
Conclusion and Perspectives
In this article, we provided an overview of previously studied adjuvants in candidate inactivated and subunit coronavirus vaccines with a focus on the types of adjuvants in the vaccine formulations and the nature of immune responses to the formulated vaccines. These previous studies provided a convenient basis for the screening of adjuvants required to develop coronavirus vaccines. In-depth reviews of the various adjuvants, a comprehensive understanding of their impacts on the extent and types of immune responses, and an exploration of their combinations with various antigen types and vaccine platforms will facilitate the selection of adjuvants that provide the required immunological protection of coronavirus vaccines.
In the absence of a cure for COVD-19, effective and safe vaccines are urgently required. Adjuvants such as aluminum-based salts, TLR agonists, emulsions, and other novel adjuvants have distinctive physicochemical properties, which can be significant in regulating the strength, duration, and types of immune responses (19, 63, 77). Studies have suggested that neutralizing antibodies are critical for immune protection (34, 42).While mechanistic studies are still being conducted, emerging evidence has suggested that SRAS-CoV-2-specific CD4+ and CD8+ T cells in coordination with neutralizing antibodies are required for generating protective immunity against SARS-CoV-2 (33). Thus, the appropriate adjuvants should be selected to formulate specific antigens that will achieve optimal immunogenicity profiles. Current available studies have demonstrated the feasibility of formulating S protein, RBD domain, M protein and N protein with specific adjuvants.
It should be noted that the development of a COVID-19 vaccine has been on a fast track. Thus far, four non-replicating viral vector vaccines, three inactivated vaccines and two mRNA vaccines being under clinical phase III stage, with more are on the way (32). Though different types of adjuvants have been used in exploratory and pre-clinical studies (Tables 1–3), considering the need for rapid deployment of COVID-19 vaccines for the pandemic, alum, which had been formulated in many other licensed vaccines, have been prioritized (15, 16). In addition to the adjuvants described above, engineered nanomaterials also shed light adjuvant development. It has been shown that physicochemical characteristics of aluminum oxyhydroxide could affect the optimal immunogenicity profiles of vaccine formulations (41, 83, 84). Moreover, a recent study has shown that an alum-stabilized Pickering emulsion (PAPE) showed robust RBD-specific IgG1 and IgG2a titers and a high level of inducing IFN-γ-secreting T cells in a COVID-19 vaccine. Additionally, it has been shown that a natural and potent STING agonist encapsulated by pulmonary biomimetic liposomes triggered rapid humoral and cellular immune responses and exhibited a sustained cross-protection against influenza (76). However, more comprehensive mechanistic studies, including the nature of protective immune responses and screening of the various combinations of antigens and adjuvants, are needed for the successful development of a safe and effective COVID-19 vaccine.
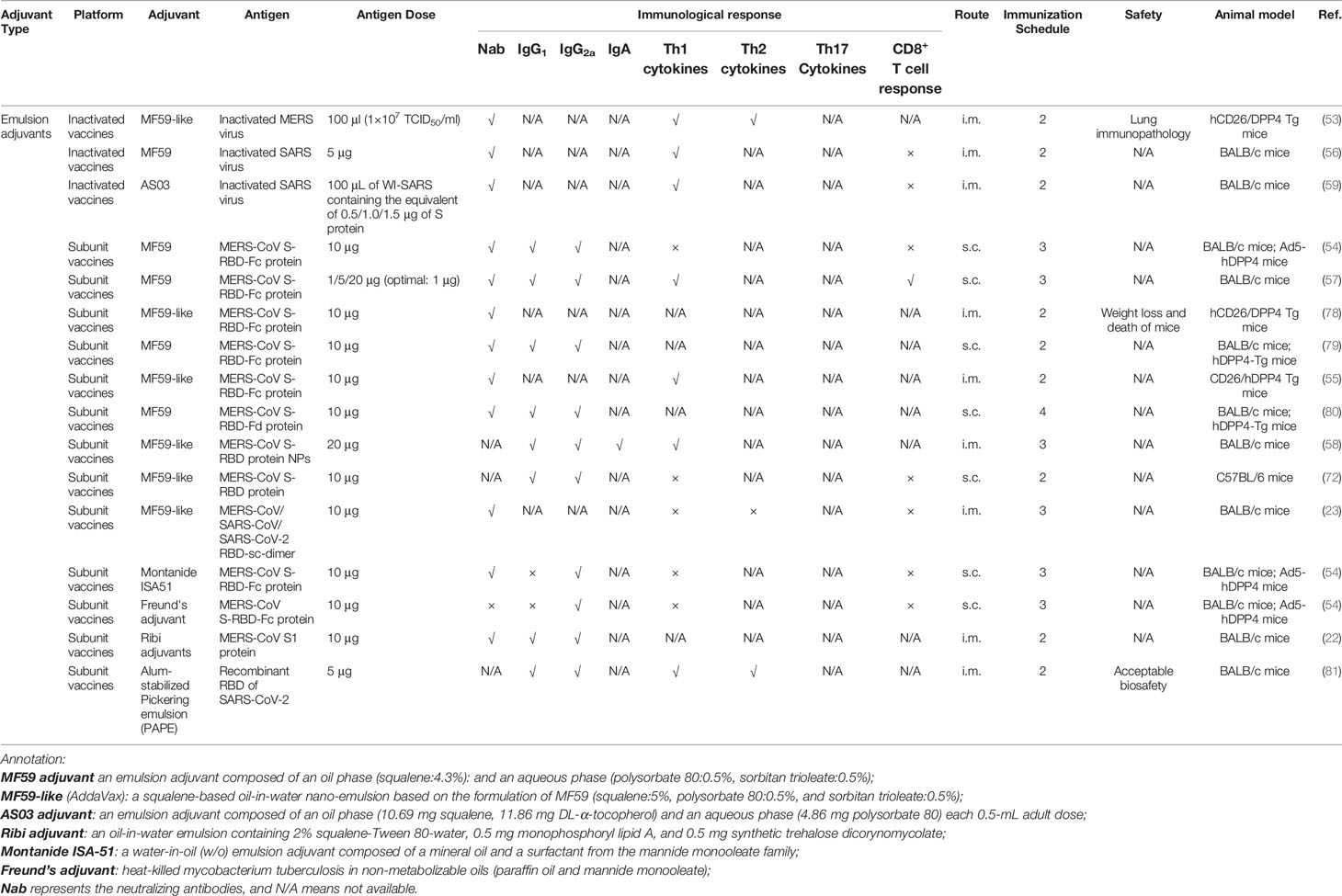
Table 2 Emulsion adjuvants used in the coronavirus vaccine formulations under exploratory and pre-clinical investigations.
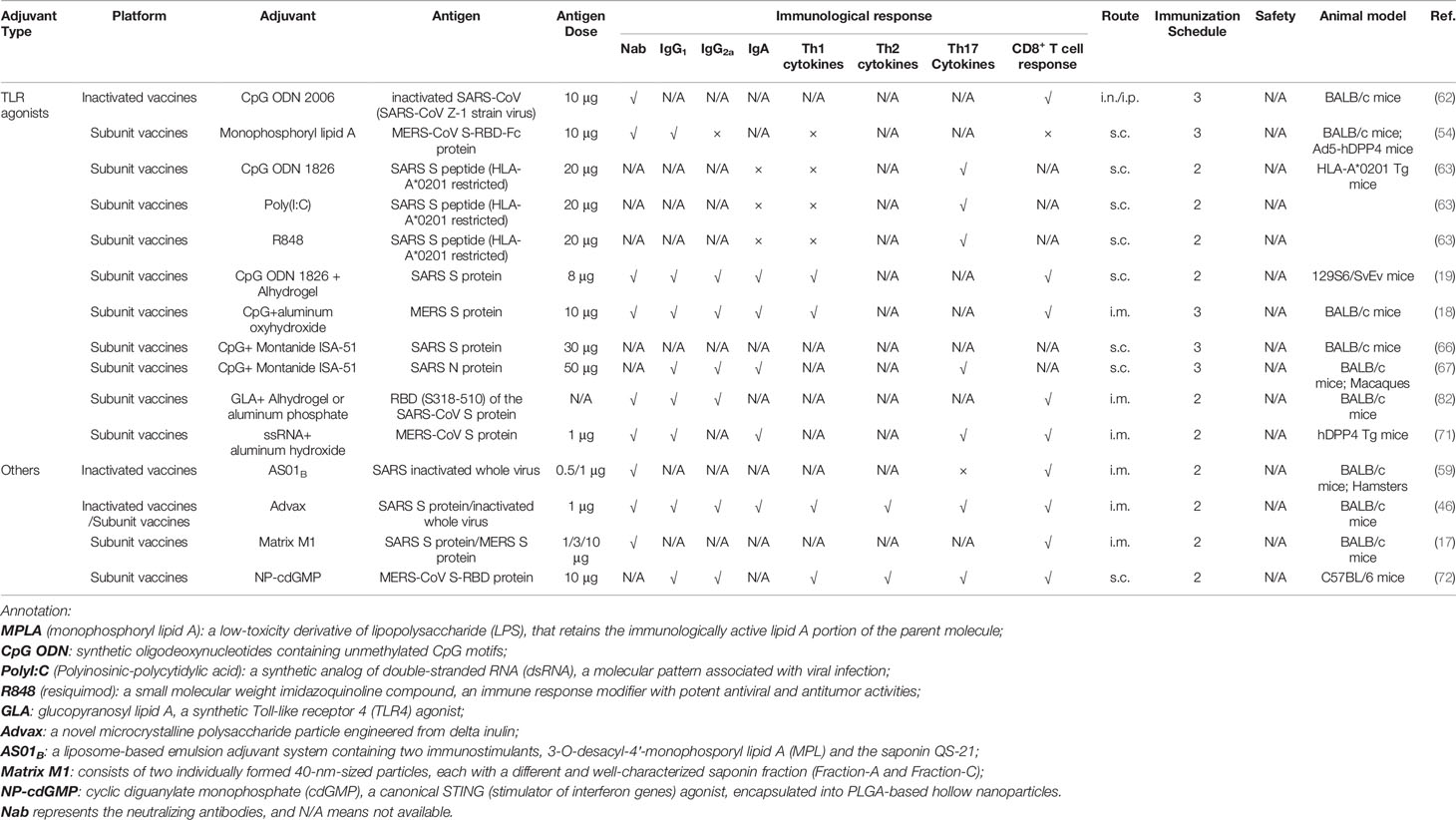
Table 3 TLR agonists and other adjuvants used in the coronavirus vaccine formulations under exploratory and pre-clinical investigations.
Author Contributions
ZLiang, HZhu, XW, BJ, and XX wrote the manuscript. BS, LS and HZeng conceived and revised the manuscript. HS, YY, and WZ provided critical suggestions. All authors contributed to the article and approved the submitted version.
Funding
This work was supported by the National Natural Science Foundation of China (31870919), Natural Science Foundation of Liaoning Province (No. 20180550597), LiaoNing Revitalization Talents Program (XLYC1807113), Dalian Science and Technology Innovation Fund (No. 2020JJ25CY015), and Fundamental Research Funds for the Central Universities (DUT19TD12).
Conflict of Interest
WZ was employed by NCPC Genetech Biotechnology Co., Ltd. LS was employed by Shanghai Zerun Biotechnology Co., Ltd.
The remaining authors declare that the research was conducted in the absence of any commercial or financial relationships that could be construed as a potential conflict of interest.
References
1. Fehr AR, Perlman S. Coronaviruses: an overview of their replication and pathogenesis. Methods Mol Biol (2015) 1282:1–23. doi: 10.1007/978-1-4939-2438-7_1
2. Lu S. Timely development of vaccines against SARS-CoV-2. Emerg Microbes Infect (2020) 9:542–4. doi: 10.1080/22221751.2020.1737580
3. Lau EHY, Hsiung CA, Cowling BJ, Chen CH, Ho LM, Tsang T, et al. A comparative epidemiologic analysis of SARS in Hong Kong, Beijing and Taiwan. BMC Infect Dis (2010) 10:50. doi: 10.1186/1471-2334-10-50
4. World Health Organization. Middle East respiratory syndrome coronavirus (MERS-CoV) (2020). Available at: http://www.who.int/emergencies/mers-cov/en/ (Accessed October 1, 2020).
5. World Health Organization. WHO COVID-19 dashboard (2020). Available at: https://www.who.int/emergencies/diseases/novel-coronavirus-2019 (Accessed October 1, 2020).
6. Liu C, Zhou Q, Li Y, Garner LV, Watkins SP, Carter LJ, et al. Research and Development on Therapeutic Agents and Vaccines for COVID-19 and Related Human Coronavirus Diseases. ACS Cent Sci (2020) 6:315–31. doi: 10.1021/acscentsci.0c00272
7. Van Doremalen N, Lambe T, Spencer A, Belij-Rammerstorfer S, Purushotham JN, Port JR, et al. ChAdOx1 nCoV-19 vaccination prevents SARS-CoV-2 pneumonia in rhesus macaques. BioRxiv (2020) 586:578–82. doi: 10.1101/2020.05.13.093195
8. Lasaro MO, Ertl HC. New insights on adenovirus as vaccine vectors. Mol Ther (2009) 17:1333–9. doi: 10.1038/mt.2009.130
9. Koirala A, Joo YJ, Khatami A, Chiu C, Britton PN. Vaccines for COVID-19: The current state of play. Paediatr Respir Rev (2020) 35:43–9. doi: 10.1016/j.prrv.2020.06.010
10. Okba NM, Raj VS, Haagmans BL. Middle East respiratory syndrome coronavirus vaccines: current status and novel approaches. Curr Opin Virol (2017) 23:49–58. doi: 10.1016/j.coviro.2017.03.007
11. Tseng CT, Sbrana E, Iwata-Yoshikawa N, Newman PC, Garron T, Atmar RL, et al. Immunization with SARS coronavirus vaccines leads to pulmonary immunopathology on challenge with the SARS virus. PLoS One (2012) 7:e35421. doi: 10.1371/journal.pone.0035421
12. Takasuka N, Fujii H, Takahashi Y, Kasai M, Morikawa S, Itamura S, et al. A subcutaneously injected UV-inactivated SARS coronavirus vaccine elicits systemic humoral immunity in mice. Int Immunol (2004) 16:1423–30. doi: 10.1093/intimm/dxh143
13. Spruth M, Kistner O, Savidis-Dacho H, Hitter E, Crowe B, Gerencer M, et al. A double-inactivated whole virus candidate SARS coronavirus vaccine stimulates neutralising and protective antibody responses. Vaccine (2006) 24:652–61. doi: 10.1016/j.vaccine.2005.08.055
14. Bolles M, Deming D, Long K, Agnihothram S, Whitmore A, Ferris M, et al. A double-inactivated severe acute respiratory syndrome coronavirus vaccine provides incomplete protection in mice and induces increased eosinophilic proinflammatory pulmonary response upon challenge. J Virol (2011) 85:12201–15. doi: 10.1128/JVI.06048-11
15. Gao Q, Bao L, Mao H, Wang L, Xu K, Yang M, et al. Rapid development of an inactivated vaccine for SARS-CoV-2. Science (2020) 369:77–81. doi: 10.1101/2020.04.17.046375
16. Wang H, Zhang Y, Huang B, Deng W, Quan Y, Wang W, et al. Development of an Inactivated Vaccine Candidate, BBIBP-CorV, with Potent Protection against SARS-CoV-2. Cell (2020) 182:713–21.e9. doi: 10.1016/j.cell.2020.06.008
17. Coleman CM, Liu YV, Mu H, Taylor JK, Massare M, Flyer DC, et al. Purified coronavirus spike protein nanoparticles induce coronavirus neutralizing antibodies in mice. Vaccine (2014) 32:3169–74. doi: 10.1016/j.vaccine.2014.04.016
18. Lan J, Deng Y, Chen H, Lu G, Wang W, Guo X, et al. Tailoring subunit vaccine immunity with adjuvant combinations and delivery routes using the Middle East respiratory coronavirus (MERS-CoV) receptor-binding domain as an antigen. PLoS One (2014) 9:e112602. doi: 10.1371/journal.pone.0112602
19. Zakhartchouk AN, Sharon C, Satkunarajah M, Auperin T, Viswanathan S, Mutwiri G, et al. Immunogenicity of a receptor-binding domain of SARS coronavirus spike protein in mice: implications for a subunit vaccine. Vaccine (2007) 25:136–43. doi: 10.1016/j.vaccine.2006.06.084
20. Zhou Z, Post P, Chubet R, Holtz K, McPherson C, Petric M, et al. A recombinant baculovirus-expressed S glycoprotein vaccine elicits high titers of SARS-associated coronavirus (SARS-CoV) neutralizing antibodies in mice. Vaccine (2006) 24:3624–31. doi: 10.1016/j.vaccine.2006.01.059
21. Lan J, Yao Y, Deng Y, Chen H, Lu G, Wang W, et al. Recombinant Receptor Binding Domain Protein Induces Partial Protective Immunity in Rhesus Macaques Against Middle East Respiratory Syndrome Coronavirus Challenge. Ebiomedicine (2015) 2:1438–46. doi: 10.1016/j.ebiom.2015.08.031
22. Wang L, Shi W, Joyce MG, Modjarrad K, Zhang Y, Leung K, et al. Evaluation of candidate vaccine approaches for MERS-CoV. Nat Commun (2015) 6:7712. doi: 10.1038/ncomms8712
23. Dai L, Zheng T, Xu K, Han Y, Xu L, Huang E, et al. A Universal Design of Betacoronavirus Vaccines against COVID-19, MERS, and SARS. Cell (2020) 182:722–33. doi: 10.1016/j.cell.2020.06.035
24. Yang J, Wang W, Chen Z, Lu S, Yang F, Bi Z, et al. A vaccine targeting the RBD of the S protein of SARS-CoV-2 induces protective immunity. Nature (2020) 586:572–7. doi: 10.1038/s41586-020-2599-8
25. Du L, He Y, Zhou Y, Liu S, Zheng BJ, Jiang S. The spike protein of SARS-CoV–a target for vaccine and therapeutic development. Nat Rev Microbiol (2009) 7:226–36. doi: 10.1038/nrmicro2090
26. Kang S, Yang M, Hong Z, Zhang L, Huang Z, Chen X, et al. Crystal structure of SARS-CoV-2 nucleocapsid protein RNA binding domain reveals potential unique drug targeting sites. Acta Pharm Sin B (2020) 10:1228–38. doi: 10.1016/j.apsb.2020.04.009
27. Maeda J, Repass JF, Maeda A, Makino S. Membrane topology of coronavirus E protein. Virology (2001) 281:163–9. doi: 10.1006/viro.2001.0818
28. Shi S, Zhu H, Xia X, Liang Z, Ma X, Sun B. Vaccine adjuvants: Understanding the structure and mechanism of adjuvanticity. Vaccine (2019) 37:3167–78. doi: 10.1016/j.vaccine.2019.04.055
29. Thanh Le T, Andreadakis Z, Kumar A, Gomez Roman R, Tollefsen S, Saville M, et al. The COVID-19 vaccine development landscape. Nat Rev Drug Discov (2020) 19:305–6. doi: 10.1038/d41573-020-00073-5
30. McPherson C, Chubet R, Holtz K, Honda-Okubo Y, Barnard D, Cox M, et al. Development of a SARS Coronavirus Vaccine from Recombinant Spike Protein Plus Delta Inulin Adjuvant. Methods Mol Biol (2016) 1403:269–84. doi: 10.1007/978-1-4939-3387-7_14
31. Jiang S, Lu L, Du L. Development of SARS vaccines and therapeutics is still needed. Future Virol (2013) 8:1–2. doi: 10.2217/fvl.12.126
32. World Health Organization. Draft landscape of COVID-19 candidate vaccines (2020). Available at: https://www.who.int/publications/m/item/draft-landscape-of-covid-19-candidate-vaccines (Accessed October 1, 2020).
33. Moderbacher CR, Ramirez SI, Dan JM, Grifoni A, Hastie KM, Weiskopf D, et al. Antigen-specific adaptive immunity to SARS-CoV-2 in acute COVID-19 and associations with age and disease severity. Cell (2020) 183:1–17. doi: 10.1016/j.cell.2020.09.038
34. Iwasaki A, Yang Y. The potential danger of suboptimal antibody responses in COVID-19. Nat Rev Immunol (2020) 20:339–41. doi: 10.1038/s41577-020-0321-6
35. Wang Q, Zhang L, Kuwahara K, Li L, Liu Z, Li T, et al. Immunodominant SARS Coronavirus Epitopes in Humans Elicited both Enhancing and Neutralizing Effects on Infection in Non-human Primates. ACS Infect Dis (2016) 2:361–76. doi: 10.1021/acsinfecdis.6b00006
36. Wan Y, Shang J, Sun S, Tai W, Chen J, Geng Q, et al. Molecular Mechanism for Antibody-Dependent Enhancement of Coronavirus Entry. J Virol (2020) 94:e02015–19. doi: 10.1128/jvi.02015-19
37. Yasui F, Kai C, Kitabatake M, Inoue S, Yoneda M, Yokochi S, et al. Prior immunization with severe acute respiratory syndrome (SARS)-associated coronavirus (SARS-CoV) nucleocapsid protein causes severe pneumonia in mice infected with SARS-CoV. J Immunol (2008) 181:6337–48. doi: 10.4049/jimmunol.181.9.6337
38. Graham BS, Henderson GS, Tang YW, Lu X, Neuzil KM, Colley DG. Priming immunization determines T helper cytokine mRNA expression patterns in lungs of mice challenged with respiratory syncytial virus. J Immunol (1993) 151:2032–40.
39. Li X, Wang X, Ito A. Tailoring inorganic nanoadjuvants towards next-generation vaccines. Chem Soc Rev (2018) 47:4954–80. doi: 10.1039/c8cs00028j
40. Singh M. Recent Advances in Vaccine Adjuvants. Pharm Res (2002) 19:715–28. doi: 10.1023/A:1016104910582
41. Sun B, Ji Z, Liao Y-P, Wang M, Wang X, Dong J, et al. Engineering an Effective Immune Adjuvant by Designed Control of Shape and Crystallinity of Aluminum Oxyhydroxide Nanoparticles. ACS Nano (2013) 7:10834–49. doi: 10.1021/nn404211j
42. Heaton PM. The Covid-19 Vaccine-Development Multiverse. N Engl J Med (2020). doi: 10.1056/NEJMe2025111
43. Bai S, Dong A. Effects of immobilization onto aluminum hydroxide particles on the thermally induced conformational behavior of three model proteins. Int J Biol Macromol (2009) 45:80–5. doi: 10.1016/j.ijbiomac.2009.04.008
44. Ruwona TB, Xu H, Li X, Taylor AN, Shi YC, Cui Z. Toward understanding the mechanism underlying the strong adjuvant activity of aluminum salt nanoparticles. Vaccine (2016) 34:3059–67. doi: 10.1016/j.vaccine.2016.04.081
45. See RH, Zakhartchouk AN, Petric M, Lawrence DJ, Mok CP, Hogan RJ, et al. Comparative evaluation of two severe acute respiratory syndrome (SARS) vaccine candidates in mice challenged with SARS coronavirus. J Gen Virol (2006) 87:641–50. doi: 10.1099/vir.0.81579-0
46. Honda-Okubo Y, Barnard D, Ong CH, Peng B-H, Tseng C-TK, Petrovsky N. Severe Acute Respiratory Syndrome-Associated Coronavirus Vaccines Formulated with Delta Inulin Adjuvants Provide Enhanced Protection while Ameliorating Lung Eosinophilic Immunopathology. J Virol (2015) 89:2995–3007. doi: 10.1128/jvi.02980-14
47. Deming D, Sheahan T, Heise M, Yount B, Davis N, Sims A, et al. Vaccine Efficacy in Senescent Mice Challenged with Recombinant SARS-CoV Bearing Epidemic and Zoonotic Spike Variants. PLoS Med (2006) 3:e525. doi: 10.1371/journal.pmed.0030525
48. Hotez PJ, Corry DB, Strych U, Bottazzi ME. COVID-19 vaccines: neutralizing antibodies and the alum advantage. Nat Rev Immunol (2020) 20:399–400. doi: 10.1038/s41577-020-0358-6
49. Kong SL, Chui P, Lim B, Salto-Tellez M. Elucidating the molecular physiopathology of acute respiratory distress syndrome in severe acute respiratory syndrome patients. Virus Res (2009) 145:260–9. doi: 10.1016/j.virusres.2009.07.014
50. O’Hagan DT, Ott GS, De Gregorio E, Seubert A. The mechanism of action of MF59 – An innately attractive adjuvant formulation. Vaccine (2012) 30:4341–8. doi: 10.1016/j.vaccine.2011.09.061
51. Garcon N, Vaughn DW, Didierlaurent AM. Development and evaluation of AS03, an Adjuvant System containing alpha-tocopherol and squalene in an oil-in-water emulsion. Expert Rev Vaccines (2012) 11:349–66. doi: 10.1586/erv.11.192
52. Morel S, Didierlaurent A, Bourguignon P, Delhaye S, Baras B, Jacob V, et al. Adjuvant System AS03 containing alpha-tocopherol modulates innate immune response and leads to improved adaptive immunity. Vaccine (2011) 29:2461–73. doi: 10.1016/j.vaccine.2011.01.011
53. Agrawal AS, Tao X, Algaissi A, Garron T, Narayanan K, Peng BH, et al. Immunization with inactivated Middle East Respiratory Syndrome coronavirus vaccine leads to lung immunopathology on challenge with live virus. Hum Vaccin Immunother (2016) 12:2351–6. doi: 10.1080/21645515.2016.1177688
54. Zhang N, Channappanavar R, Ma C, Wang L, Tang J, Garron T, et al. Identification of an ideal adjuvant for receptor-binding domain-based subunit vaccines against Middle East respiratory syndrome coronavirus. Cell Mol Immunol (2016) 13:180–90. doi: 10.1038/cmi.2015.03
55. Nyon MP, Du L, Tseng C-TK, Seid CA, Pollet J, Naceanceno KS, et al. Engineering a stable CHO cell line for the expression of a MERS-coronavirus vaccine antigen. Vaccine (2018) 36:1853–62. doi: 10.1016/j.vaccine.2018.02.065
56. Kong WP, Xu L, Stadler K, Ulmer JB, Abrignani S, Rappuoli R, et al. Modulation of the immune response to the severe acute respiratory syndrome spike glycoprotein by gene-based and inactivated virus immunization. J Virol (2005) 79:13915–23. doi: 10.1128/JVI.79.22.13915-13923.2005
57. Tang J, Zhang N, Tao X, Zhao G, Guo Y, Tseng C-TK, et al. Optimization of antigen dose for a receptor-binding domain-based subunit vaccine against MERS coronavirus. Hum Vaccin Immunother (2015) 11:1244–50. doi: 10.1080/21645515.2015.1021527
58. Kim Y-S, Son A, Kim J, Kwon SB, Kim MH, Kim P, et al. Chaperna-Mediated Assembly of Ferritin-Based Middle East Respiratory Syndrome-Coronavirus Nanoparticles. Front Immunol (2018) 9:1093. doi: 10.3389/fimmu.2018.01093
59. Roberts A, Lamirande EW, Vogel L, Baras B, Goossens G, Knott I, et al. Immunogenicity and protective efficacy in mice and hamsters of a beta-propiolactone inactivated whole virus SARS-CoV vaccine. Viral Immunol (2010) 23:509–19. doi: 10.1089/vim.2010.0028
60. Steinhagen F, Kinjo T, Bode C, Klinman DM. TLR-based immune adjuvants. Vaccine (2011) 29:3341–55. doi: 10.1016/j.vaccine.2010.08.002
61. Kaisho T, Akira S. Toll-like receptors as adjuvant receptors. Biochim Biophys Acta Mol Cell Res (2002) 1589:1–13. doi: 10.1016/S0167-4889(01)00182-3
62. Gai W, Zou W, Lei L, Luo J, Tu H, Zhang Y, et al. Effects of different immunization protocols and adjuvant on antibody responses to inactivated SARS-CoV vaccine. Viral Immunol (2008) 21:27–37. doi: 10.1089/vim.2007.0079
63. Zhao K, Wang H, Wu C. The immune responses of HLA-A*0201 restricted SARS-CoV S peptide-specific CD8+ T cells are augmented in varying degrees by CpG ODN, PolyI:C and R848. Vaccine (2011) 29:6670–8. doi: 10.1016/j.vaccine.2011.06.100
64. Channappanavar R, Fett C, Zhao J, Meyerholz DK, Perlman S. Virus-specific memory CD8 T cells provide substantial protection from lethal severe acute respiratory syndrome coronavirus infection. J Virol (2014) 88:11034–44. doi: 10.1128/JVI.01505-14
65. Weeratna RD, Brazolot Millan CL, McCluskie MJ, Davis HL. CpG ODN can re-direct the Th bias of established Th2 immune responses in adult and young mice. FEMS Immunol Med Microbiol (2001) 32:65–71. doi: 10.1111/j.1574-695X.2001.tb00535.x
66. Lien SP, Shih YP, Chen HW, Tsai JP, Leng CH, Lin MH, et al. Identification of synthetic vaccine candidates against SARS CoV infection. Biochem Biophys Res Commun (2007) 358:716–21. doi: 10.1016/j.bbrc.2007.04.164
67. Liu S, Leng C, Lien S, Chi H, Huang C, Lin C, et al. Immunological characterizations of the nucleocapsid protein based SARS vaccine candidates. Vaccine (2006) 24:3100–8. doi: 10.1016/j.vaccine.2006.01.058
68. Cheng L, Zhang Z, Li G, Li F, Wang L, Zhang L, et al. Human innate responses and adjuvant activity of TLR ligands in vivo in mice reconstituted with a human immune system. Vaccine (2017) 35:6143–53. doi: 10.1016/j.vaccine.2017.09.052
69. Gadd AJR, Castelletto V, Kabova E, Shankland K, Perrie Y, Hamley I, et al. High potency of lipid conjugated TLR7 agonist requires nanoparticulate or liposomal formulation. Eur J Pharm Sci (2018) 123:268–76. doi: 10.1016/j.ejps.2018.07.048
70. Dowling DJ. Recent Advances in the Discovery and Delivery of TLR7/8 Agonists as Vaccine Adjuvants. Immunohorizons (2018) 2:185–97. doi: 10.4049/immunohorizons.1700063
71. Kwak HW, Park H-J, Ko HL, Park H, Cha MH, Lee S-M, et al. Cricket paralysis virus internal ribosome entry site-derived RNA promotes conventional vaccine efficacy by enhancing a balanced Th1/Th2 response. Vaccine (2019) 37:5191–202. doi: 10.1016/j.vaccine.2019.07.070
72. Lin LC, Huang CY, Yao BY, Lin JC, Agrawal A, Algaissi A, et al. Viromimetic STING Agonist-Loaded Hollow Polymeric Nanoparticles for Safe and Effective Vaccination against Middle East Respiratory Syndrome Coronavirus. Adv Funct Mater (2019) 29:1807616. doi: 10.1002/adfm.201807616
73. V’kovski P, Gultom M, Steiner S, Kelly J, Russeil J, Mangeat B, et al. Disparate temperature-dependent virus-host dynamics for SARS-CoV-2 and SARS-CoV in the human respiratory epithelium. BioRxiv (2020). doi: 10.1101/2020.04.27.062315
74. Uematsu S, Fujimoto K, Jang MH, Yang BG, Jung YJ, Nishiyama M, et al. Regulation of humoral and cellular gut immunity by lamina propria dendritic cells expressing Toll-like receptor 5. Nat Immunol (2008) 9:769–76. doi: 10.1038/ni.1622
75. Madhun AS, Haaheim LR, Nostbakken JK, Ebensen T, Chichester J, Yusibov V, et al. Intranasal c-di-GMP-adjuvanted plant-derived H5 influenza vaccine induces multifunctional Th1 CD4+ cells and strong mucosal and systemic antibody responses in mice. Vaccine (2011) 29:4973–82. doi: 10.1016/j.vaccine.2011.04.094
76. Wang J, Li P, Yu Y, Fu Y, Jiang H, Lu M, et al. Pulmonary surfactant-biomimetic nanoparticles potentiate heterosubtypic influenza immunity. Science (2020) 367:869. doi: 10.1126/science.aau0810
77. Xia Y, Wu J, Wei W, Du Y, Wan T, Ma X, et al. Exploiting the pliability and lateral mobility of Pickering emulsion for enhanced vaccination. Nat Mater (2018) 17:187–94. doi: 10.1038/nmat5057
78. Tao X, Garron T, Agrawal AS, Algaissi A, Peng BH, Wakamiya M, et al. Characterization and Demonstration of the Value of a Lethal Mouse Model of Middle East Respiratory Syndrome Coronavirus Infection and Disease. J Virol (2016) 90:57–67. doi: 10.1128/JVI.02009-15
79. Wang Y, Tai W, Yang J, Zhao G, Sun S, Tseng CK, et al. Receptor-binding domain of MERS-CoV with optimal immunogen dosage and immunization interval protects human transgenic mice from MERS-CoV infection. Hum Vaccin Immunother (2017) 13:1615–24. doi: 10.1080/21645515.2017.1296994
80. Tai W, Zhao G, Sun S, Guo Y, Wang Y, Tao X, et al. A recombinant receptor-binding domain of MERS-CoV in trimeric form protects human dipeptidyl peptidase 4 (hDPP4) transgenic mice from MERS-CoV infection. Virology (2016) 499:375–82. doi: 10.1016/j.virol.2016.10.005
81. Peng S, Cao F, Xia Y, Gao XD, Dai L, Yan J, et al. Particulate Alum via Pickering Emulsion for an Enhanced COVID-19 Vaccine Adjuvant. Adv Mater (2020) 32:2004210. doi: 10.1002/adma.202004210
82. Jiang S, Bottazzi ME, Du L, Lustigman S, Tseng CT, Curti E, et al. Roadmap to developing a recombinant coronavirus S protein receptor-binding domain vaccine for severe acute respiratory syndrome. Expert Rev Vaccines (2012) 11:1405–13. doi: 10.1586/erv.12.126
83. Sun B, Ji Z, Liao YP, Chang CH, Wang X, Ku J, et al. Enhanced Immune Adjuvant Activity of Aluminum Oxyhydroxide Nanorods through Cationic Surface Functionalization. ACS Appl Mater Interfaces (2017) 9:21697–705. doi: 10.1021/acsami.7b05817
Keywords: coronavirus disease 2019, SARS-CoV-2, adjuvant, coronavirus vaccine, aluminum salt
Citation: Liang Z, Zhu H, Wang X, Jing B, Li Z, Xia X, Sun H, Yang Y, Zhang W, Shi L, Zeng H and Sun B (2020) Adjuvants for Coronavirus Vaccines. Front. Immunol. 11:589833. doi: 10.3389/fimmu.2020.589833
Received: 31 July 2020; Accepted: 14 October 2020;
Published: 06 November 2020.
Edited by:
Katie Ewer, University of Oxford, United KingdomReviewed by:
Anke Huckriede, University Medical Center Groningen, NetherlandsAndrew Ishizuka, National Institutes of Health (NIH), United States
Tamiru Alkie, Wilfrid Laurier University, Canada
Copyright © 2020 Liang, Zhu, Wang, Jing, Li, Xia, Sun, Yang, Zhang, Shi, Zeng and Sun. This is an open-access article distributed under the terms of the Creative Commons Attribution License (CC BY). The use, distribution or reproduction in other forums is permitted, provided the original author(s) and the copyright owner(s) are credited and that the original publication in this journal is cited, in accordance with accepted academic practice. No use, distribution or reproduction is permitted which does not comply with these terms.
*Correspondence: Bingbing Sun, YmluZ2JpbmdzdW5AZGx1dC5lZHUuY24=; Hao Zeng, emVuZzExMDlAMTYzLmNvbQ==
†Present Address: Li Shi, Immune Path Biotechnology Co., Ltd., Suzhou, China