- 1Institut Pasteur de Tunis, LR19IPTX, Service d’Entomologie Médicale, Université de Tunis El Manar, Tunis, Tunisia
- 2Innovation and Development Laboratory, Innovation and Development Center, Instituto Butantan, São Paulo, Brazil
- 3Institute of Parasitology, Biology Centre, Czech Academy of Sciences, České Budějovice, Czechia
- 4Department of Medical Biology, Faculty of Science, University of South Bohemia in České Budějovice, České Budějovice, Czechia
Immunodeficiency disorders and autoimmune diseases are common, but a lack of effective targeted drugs and the side-effects of existing drugs have stimulated interest in finding therapeutic alternatives. Naturally derived substances are a recognized source of novel drugs, and tick saliva is increasingly recognized as a rich source of bioactive molecules with specific functions. Ticks use their saliva to overcome the innate and adaptive host immune systems. Their saliva is a rich cocktail of molecules including proteins, peptides, lipid derivatives, and recently discovered non-coding RNAs that inhibit or modulate vertebrate immune reactions. A number of tick saliva and/or salivary gland molecules have been characterized and shown to be promising candidates for drug development for vertebrate immune diseases. However, further validation of these molecules at the molecular, cellular, and organism levels is now required to progress lead candidates to clinical testing. In this paper, we review the data on the immuno-pharmacological aspects of tick salivary compounds characterized in vitro and/or in vivo and present recent findings on non-coding RNAs that might be exploitable as immunomodulatory therapies.
Introduction
The vertebrate immune system is a sophisticated and highly developed network of cells, tissues, and organs that together identify and neutralize foreign and endogenous threats. Immunodeficiencies represent a breakdown in these highly organized processes caused by a lack or dysfunction of specific immune cell subpopulations or soluble effectors (1). Immune disorders also arise due to excessive immune responses, such as in allergic reactions or autoimmunity (2), usually as a result of dysregulation or overexpression of specific cytokines and their related immune signaling pathways (3). The human immune system is also affected, and often weakened, by stress, malnutrition, and age-related changes, which increase susceptibility to infectious diseases and other pathologies such as cancer (4–6). Current therapies for immune-related illnesses usually have side-effects or suboptimal efficacy (7, 8), leading to efforts to identify alternative therapies that might naturally inhibit and/or modulate specific immune system targets without significant off-target effects (9), including bioactive molecules derived from natural sources (10). Compounds with therapeutic potential have traditionally been extracted from plants (phytotherapy) (11), but other sources have included scorpion and snake venom (12, 13) and arthropods (14). However, substances extracted from natural sources and applied in practice remain limited, and their clinical utility is hampered by the risk of contamination with impurities (15). More positively, drug discovery has been assisted by the development of new protein production methods using various expression systems (16–18), and advances in new technologies such as next-generation sequencing (NGS) and mass spectrometry (MS) have revolutionized screening for novel natural substances (19, 20).
Tick salivary glands are now recognized as a rich source of pharmaco-active molecules (21). Tick saliva contains a rich cocktail of bioactive molecules including protein and lipid derivatives with a remarkable binding affinity, avidity, and selectivity for their targets in various host defense systems (22). Ticks are obligatory hematophagous that, in order to feed, must overcome the evolutionarily sophisticated immune defense systems of their vertebrate hosts. To achieve this, they secrete a wide variety of molecules in their saliva with immunomodulatory, anti-inflammatory, anti-clotting, and anti-platelet effects (23, 24).
Despite the identification of a large number of bioactive tick salivary molecules, their investigation for therapeutic purposes remains in its outset. Only a limited number of tick salivary compounds, mostly proteins, have been tested pre-clinically (25, 26). Here we discuss the literature on therapeutically valuable tick salivary molecules with function(s) known to be directly related to host immune responses. In doing so, we identify the most promising salivary candidates with drug development potential, including newly discovered non-coding (nc) RNAs.
Tick Saliva Targets at the Tick-Host Interface
Ticks are obligate hematophagous ectoparasites of amphibians, reptiles, birds, and mammals that cause host blood loss and skin damage (27). Compared to other blood feeders, tick feeding behavior is unique. Hard ticks discretely and solidly attach to their hosts for several days to weeks to complete their blood uptake (28), with the skin representing the main interface where tick salivary compounds meet host defense systems (29, 30) (Figure 1). Furthermore, the skin is the site of multiplication and persistence of several tick-borne pathogens (31, 32). In the local environment of the skin, the tick-host molecular interaction can be viewed as a competition between host defense mechanisms against the ectoparasite and tick evasion strategies (30). To ensure an uninterrupted blood meal, ticks have developed myriad strategies to overcome the complex homeostatic and immune responses that are raised against them (22). As long-term pool feeders, they create an immunologically privileged micro-environment in the host’s skin, in which they secrete an impressive mixture of proteins, peptides, and non-peptide molecules (Figure 1), thereby modulating wound healing, hemostasis, inflammation, and both the innate and adaptive immune responses (33). These molecules interfere with various host molecules including enzymes, cytokines, complement components, antibodies, cell signaling molecules, and immune cell receptors (33).
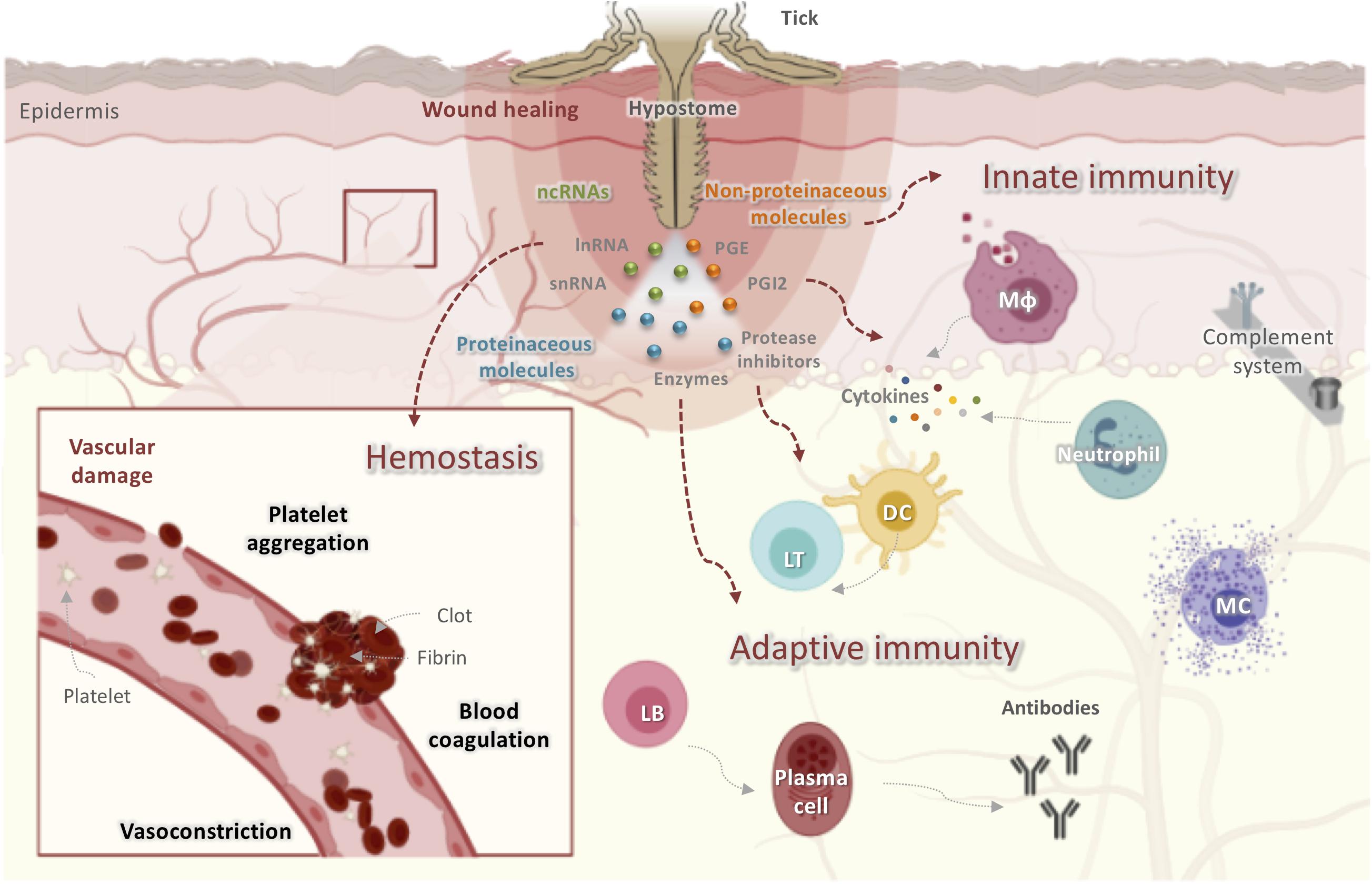
Figure 1. Obstacles faced by ticks at the host-tick interface when taking a blood meal. Ticks initiate feeding by inserting their hypostomes into host skin, resulting in tissue and vascular damage. The host has developed several mechanisms to prevent blood loss including activating hemostasis, innate and adaptive immunity, the complement pathway, and inflammatory responses leading to wound healing and tissue remodeling, all of which disrupt tick feeding. Ticks, in turn, secrete saliva at the bite site that contains proteinaceous molecules (enzymes, lipocalins, protease inhibitors, etc.), non-proteinaceous molecules (prostaglandins, prostacyclins), and ncRNAs (miRNAs and lncRNAs). These molecules display anticoagulatory, antiplatelet, vasodilatory, anti-inflammatory, and immunomodulatory activities to counter host reactions and to guarantee a successful blood meal. DC, dendritic cells; LT, lymphocyte T; LB, lymphocyte T; Mφ, macrophage.
Hemostasis is triggered within seconds of tissue damage and is the product of the triad of blood coagulation, platelet aggregation, and vasoconstriction, a process mainly controlled by serine proteases (34). The anti-hemostatic properties of tick salivary secretions are reviewed elsewhere (23, 35). In addition to hemostasis, complement components and inflammatory mediators are also initial tick saliva targets (36, 37). When tick mouthparts are inserted into the skin, pre-positioned sentinel leukocytes of the epidermis and dermis, including mast cells (MCs), eosinophils, dendritic cells (DCs), and macrophages, as well as keratinocytes and endothelial cells, are activated by mediators released from damaged skin cells or expressed by pathogens transmitted by ticks (38). The resident macrophages release pro-inflammatory chemokines and cytokines including interleukin-8 (IL-8), tumor necrosis factor (TNF), and IL-1β (38). These chemoattractants recruit blood-borne innate immune cells such as neutrophils and monocytes to the bite site, which intensify the stimulation of local and infiltrating innate immune cells (30, 39). Monocytes secrete growth hormones that induce fibroblast proliferation and extracellular matrix deposition, thus contributing to wound healing (39). Given their parasitic lifestyle, ticks must overcome innate immunity during their primary infestation and both innate and adaptive immunity during secondary or subsequent infestations (22). Following tick feeding, tick-derived antigens may be presented to naïve B and T cells by activated DCs that have acquired foreign antigens and have migrated to skin-draining lymph nodes (40). Both the humoral and cellular branches of host adaptive immunity are activated, thereby resulting in the generation of antigen-specific antibodies and T lymphocytes (40). In subsequent infestations, activated memory T and B lymphocytes secrete cytokines and produce specific antibodies that target tick salivary or mouthpart-derived antigens in an effort to reject the tick (41).
Impact of Tick Salivary Compounds on Host Immune Responses
The complex mixture of tick salivary compounds specifically and selectively targets host immune reactions, subverting the rejection and death of the tick (24). This specificity and selectivity may therefore be exploited for diseases in which dysfunction of the same host immune reactions is implicated in their pathogenesis, for instance the innate immune system in Sjögren’s syndrome (42), adaptive immunity in Tregopathies (43) and autoimmune encephalomyelitis (44), and complement activation in local and/or systemic inflammation, tissue damage, and disease (45).
Some tick salivary components have immune system specificity at different steps of immune recognition (21). Despite this specificity, tick salivary component targets often show redundancy at the molecular, cellular, and functional level (i.e., may be targeted by more than one tick salivary molecule) (29). Furthermore, several tick salivary compounds are pleiotropic, targeting both hemostatic and immune system components (29). For instance, Salp15, a multifunctional protein, was shown to bind specifically to dendritic cell, inhibit CD4 + T cell activation and proliferation, and block the liberation of interleukin 2 (IL-2) (46). Apart from its immunomodulatory activities, it has also been described to modulate host coagulation (47). This pleiotropicity could be problematic in targeted immunotherapy, which relies mainly on single target drugs and is often considered as a serious limitation in drug conception. Nevertheless, this issue does not exclude the possibility of using these salivary components in other therapeutic applications. Multi-target drugs, also known as multifunctional drugs or network therapeutics are more suitable as potential therapeutic solutions in diseases of complex etiology, such as Alzheimer’s disease, Parkinson’s disease, and neglected tropical diseases (48). Single-target drugs, although highly selective and specific, may not necessarily have better efficacy in these cases (49). Indeed, multi- target drugs tend to be beneficial to face complex disorders, multifactorial health conditions and drug resistance issues (50, 51). Some studies trend to changing paradigm from “one target one ligand” toward “multi-target” as they does not provide a complete solution for multifactorial diseases (49). Therefore, tick salivary compounds with broad-spectrum targets might be useful in these diseases mediated by multiple processes.
The immunomodulatory properties of whole tick saliva and/or salivary gland extracts were previously reviewed by Kotál and colleagues (52), and some comprehensive and recent reviews have focused on the composition and role of saliva in tick feeding and tick-host-pathogen interactions (21, 53, 54). These articles have tended to focus on proteinaceous salivary component families including lipocalins, protease inhibitors such as Kunitz-type domains containing proteins, serpins, and cystatins, metalloproteases, basic tail secreted proteins, small peptide inhibitors, and some protein families unique to ticks. The potential of non-peptide molecules, lipid derivatives, and the recently discovered ncRNAs are rarely mentioned. This review therefore focuses on immunomodulatory substances that have been shown to target both the innate and adaptive host immune systems in in vitro and/or in vivo animal models of human diseases and in doing so we identify salivary candidates with promise for targeted immunotherapy. A limited number of salivary molecules are progressing in preclinical trials. This includes Amblyomin-X, Ir-CPI, TAP, OmCI, and Ra-HBP, among others. Most of these molecules have been described as anti-hemostatic and anti-tumor candidates (55). Only OmCI and Ra-HBP target immune responses, therefore, their potential applications will be discussed later in this review. Amblyomin-X, a Kunitz-type protease inhibitor, is profoundly exploited in pre-clinical testing and is under development for cancer treatment (26). It selectively induces apoptosis in tumor cells and promotes tumor reduction in vivo in melanoma animal models and reduce metastasis and tumor growth in in vivo experiments (56). In its pre-clinical evaluation, this protein was proven to significantly decrease lung metastasis in a mice orthotopic kidney tumor model (25). More interestingly, Amblyomin-X does not seem to cause any mortality; and symptoms of toxicity were subtle, reversible, and seen only at higher doses, thus demonstrating a safety profile for injection in mice. More recently, it was investigated on Amblyomin-X-treated horse melanomas showing significant reduction in the tumor size (57). Ir-CPI (Ixodes ricinus contact phase inhibitor), an antithrombotic protein, has proven its effectiveness in inhibiting the contact phase of the coagulation cascade in preclinical trials, preventing clotting in catheter and arteriovenous shunt rabbit models during Cardiopulmonary Bypass (58). TAP, a Kunitz domain protease inhibitor from Ornithodoros moubata, has been described as an anticoagulant candidate, showing promising results in in vivo models of venous and arterial thrombosis (59). Its efficacy on blood coagulation has been approved in preliminary preclinical experiments; however, it has never been inquired in humans mainly due to its antigenicity (59).
Innate Immune Responses
The host innate immune response forms the first and immediate line of defense to tick attachment (52). Activated resident cells in the cutaneous barrier including MCs, macrophages, and DCs stimulate host awareness to the injury followed by removal of feeding ticks (Figure 2).
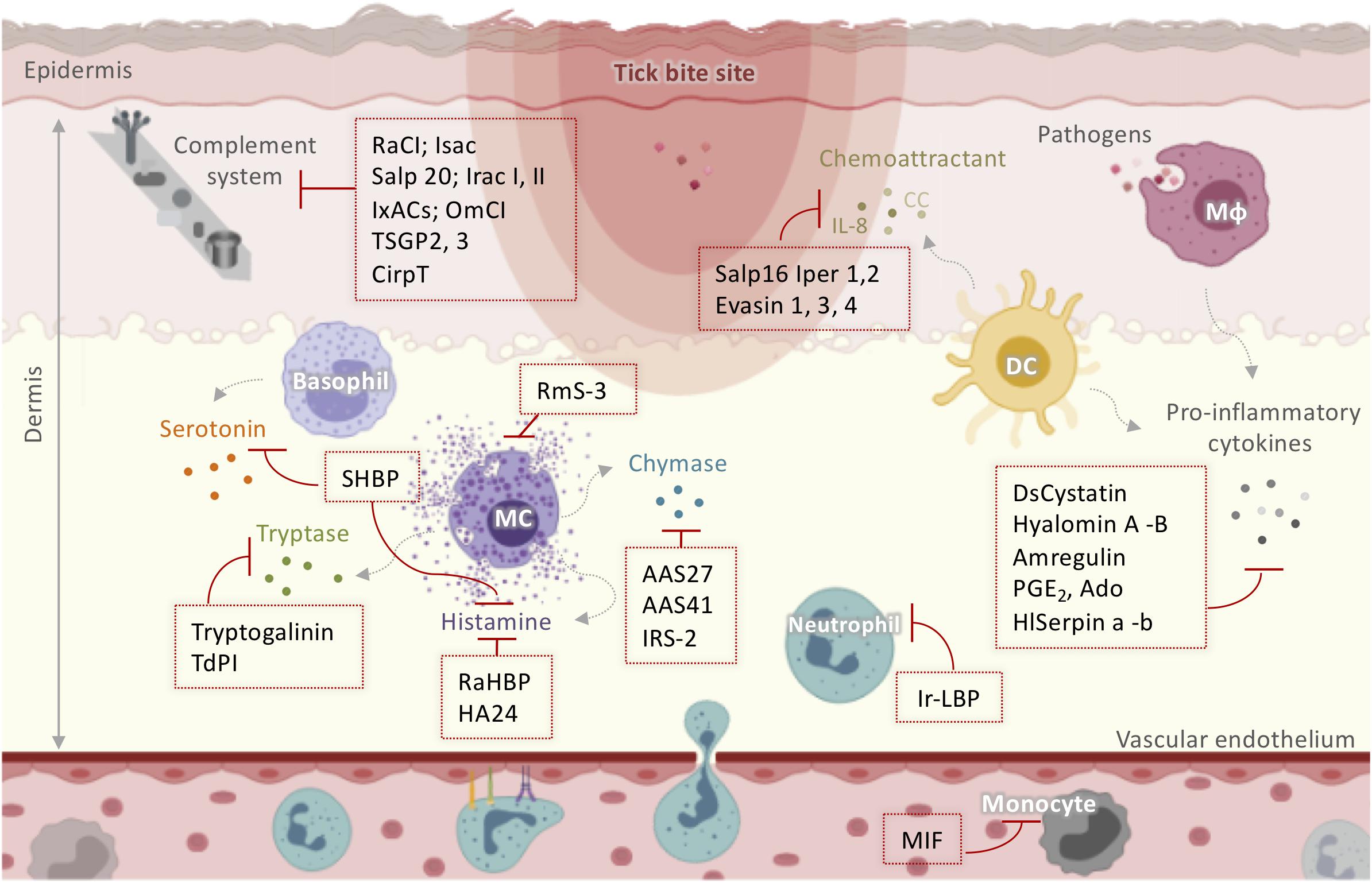
Figure 2. Schematic representation of tick salivary molecules implicated in the modulation of the complement system and innate immunity. Activated resident cells in the dermis stimulate host awareness of injury and removal of the feeding ticks. Tick saliva neutralizes itch and pain through salivary components that sequester histamine and/or serotonin (such as SHBP, Ra-HBPs, and HA24) or modulate MC function (such as RmS-3). TdPI and Tryptogalinin inhibit tryptase released by MCs. AAS27, AAS41, and IRS-2 inhibit chymase liberated by MCs. Neutrophil and monocyte recruitment is suppressed by MIF and Ir-LBP. Moreover, ticks manipulate the host cytokine network by inhibiting cytokines and chemokines using Salp16 Iper 1,2; Evasin 1, 3, 4; DsCystatin; Hyalomin-A, –B; Amregulin; PGE2; Ado; and HlSerpin-a, –b. Several anti-complement molecules have been identified in tick salivary glands including RaCI; Isac; Salp 20; Irac I, II; IxACs; OmCI; TSGP2, 3; and CirpT. AAS: Amblyomma americanum serpin; DC: dendritic cells; IL: interleukin; Irac: I. ricinus anticomplement; IRS-2: I. ricinus Serpin-2; Isac: I. scapularis salivary anticomplement; IxACs: Ixodes anticomplement proteins; MIF: macrophage migration inhibitory factor; Mφ: macrophage; OmCI: O. moubata complement inhibitor; PGE2: prostaglandin E2; Ra-HBPs: R. appendiculatus histamine-binding proteins; RmS: Rhipicephalus microplus serpin; Salp: salivary protein; SHBP: serotonin- and histamine-binding protein; TdPI: tick-derived protease inhibitor.
Itch and Pain
Mast cells and basophils degranulate to release soluble mediators such as histamine and serotonin, which cause local itch and pain at the infestation site (60). However, ticks alleviate itch and pain through salivary components that sequester histamine and/or serotonin or modulate MC function. RmS-3, a novel serpin extracted from the salivary glands of Rhipicephalus microplus (61), has been shown to modulate MC function by inhibiting chymase and vascular permeability in acute inflammation (Figure 2). The hard tick Rhipicephalus appendiculatus uses the histamine-binding proteins Ra-HBPs (in the lipocalin family) to bind histamine with high affinity during early feeding (62). Different Ra-HBPs were identified in male and female ticks: male-specific histamine-binding salivary protein Ra-HBP (M) and two female-specific histamine-binding salivary proteins RaHBP(F)-1,2 (Figure 2). Ra-HBP2 sequestered two histamine molecules with different affinities, emphasizing its therapeutic potential by targeting multiple effectors (63). Another lipocalin showing histamine binding capacity, HA24, was identified in the salivary glands of Hyalomma asiaticum (Figure 2) (64). In vitro and in vivo histamine binding assays showed that recombinant HA24 bound specifically to histamine in a dose-dependent manner and relieved allergic asthma in mice (64). SHBP (serotonin and histamine-binding protein), isolated from Dermacentor reticulatus (65), simultaneously interfered with the activity of both serotonin and histamine (Figure 2).
In addition to biogenic amine production, MCs contribute to the inflammatory process by releasing a wide range of highly bioactive effectors after degranulation, tryptases being the most abundant (66). Tryptases are implicated in the pathogenesis of allergic inflammatory diseases, cardiovascular disease, lung fibrosis, and even cancer, so their inhibition may be useful therapeutically (66, 67). Interestingly, tick-derived protease inhibitor (TdPI) was identified in the salivary glands of R. appendiculatus and was shown to suppress the activity of human β-tryptase and trypsin and, to a lesser extent, plasmin (68). TdPI accumulates in the cytosolic granules of mouse MCs, presumably blocking the autocatalytic activation of tryptase, thereby suppressing inflammation in the host animal’s skin (Figure 2) (69). Tryptogalinin, an Ixodes scapularis salivary Kunitz-type protein, was also found to inhibit β-tryptase (Figure 2) and other MC serine proteases such as α-chymotrypsin, plasmin, matriptase, and elastase and participated in inflammation and tissue remodeling (70). β-tryptases are MC-specific serine proteases with roles in inflammation that are used clinically as biomarker of MCs and their activation (67). Both tryptogalinin and TdPI could be engineered as highly specific pharmacological inhibitors of MC tryptases for the treatment of allergic inflammatory disorders like asthma (67–70).
Recruitment of Blood-Borne Innate Immune Cells
Within the first few hours after attachment, blood-borne leukocytes are recruited to the site of injury, triggered by a set of mediators including complement components, eicosanoids, chemokines, and cytokines (71). Neutrophil and monocyte recruitment has been shown to be strongly suppressed by tick salivary compounds. A homolog of the vertebrate macrophage migration inhibitory factor (MIF) was identified in Amblyomma americanum (72, 73) and Haemaphysalis longicornis (74). In both ticks, functional in vitro assays revealed that MIF inhibited the migration of human monocytes, suggesting that it might decrease monocyte recruitment in vivo. Ir-LBP from I. ricinus inhibited neutrophil chemotaxis and activation in vitro by binding specifically and with high affinity to leukotriene B4, an important inflammatory mediator (75). Ir-LBP was also shown to inhibit inflammatory responses in rabbits by decreasing the number of neutrophils located at the tick bite site (75). Therefore, Ir-LBP may have therapeutic use in inflammatory diseases or illnesses associated with increased leukotriene B4 production. Moreover, ticks employ salivary inhibitors of CXCL8 (IL-8) and CC chemokines to manipulate the host cytokine network. Salp16 Iper1 and Salp16 Iper2 (Figure 2), salivary proteins from Ixodes persulcatus, have been shown to have anti-IL-8 activity, thereby impairing neutrophil chemotaxis (76). A very recent review summarized the data on Evasins, which are secreted by hard ticks and bind to host chemokines to inhibit their activation of chemokine receptors (77). Of the Evasins, three from Rhipicephalus sanguineus (Evasin-1, -3, and -4) were selective for different chemokines (78). Evasin-1 bound to the CC chemokine members CCL3, CCL4, and CCL18 (78) and inhibited neutrophil, T cell, and macrophage migration and the production of inflammatory cytokines in vitro (78). Russo and colleagues demonstrated the high efficiency of Evasin-1 in reducing CCL3-induced influx of neutrophils in murine bleomycin-induced lung fibrosis (79). Evasin-1 has also been shown to reduce graft-versus-host disease in mice, which may be particularly useful in patients undergoing bone marrow transplantation (80). Moreover, the administration of recombinant Evasin-1 reduced skin inflammation and decreased mortality in mice deficient in the chemokine receptor D6, which renders then highly susceptible to inflammation (81). Evasin-3 is specific for the CXC chemokines CXCL8 and CXCL1 and was recently found to disrupt the interaction between CXCL8 and glycosaminoglycans and CXCR2 (Figure 2), which modulate neutrophil migration (82). Several studies have demonstrated the effectiveness of Evasin-3 in different neutrophil-dependent disease models. A single administration of Evasin-3 during mouse myocardial ischemia/reperfusion injury effectively reduced infarct size and decreased CXC chemokine-induced neutrophil recruitment (83). Evasin-3 also reduced atherosclerotic vulnerability to ischemic stroke in an in vivo murine model (84) and inhibited neutrophil-mediated pancreatic and lung inflammation in a mouse model of acute pancreatitis (85). Evasin-4 interacts with at least 18 CC chemokines (78). Similar to Evasin-3, Evasin-4 was effective against post-infarction myocardial injury and remodeling (86) and decreased the abundance of macrophages in the lungs without affecting the pancreas in the acute pancreatitis model (85). Due to its broad CC chemokine-binding spectrum, Evasin-4 is considered the most suitable candidate for therapeutic development (86). More recently, Evasin-inspired artificial peptides dramatically reduced inflammation in vivo by targeting multiple chemokines (87). These peptides might therefore provide a route to the development of new anti-inflammatory therapeutics for chronic autoimmune diseases such as rheumatoid arthritis and inflammatory diseases such as atherosclerosis. Moreover, the mechanism of action of these peptides suggests that they might also be useful in acute infectious diseases such as influenza or COVID-19, where exuberant cytokine responses are thought to be at least partially responsible for tissue injury (87).
Inflammation
Activated resident cells secrete several pro-inflammatory mediators, which initiate and then reinforce the local inflammatory process at the damaged site. Tick saliva controls inflammation by decreasing or increasing the secretion of pro- and anti-inflammatory cytokines, respectively (29). Two immunoregulatory peptides, Hyalomin-A and –B from H. asiaticum asiaticum, overcome host inflammation by modulating cytokine expression, inhibiting the secretion of pro-inflammatory TNF-α, MCP-1, and IFN-γ and stimulating the secretion of the immunosuppressant cytokine IL-10 (88). Amregulin from Amblyomma variegatum saliva was found to suppress the in vitro production of TNF-α, IL-1, IL-8, and IFN-γ in a dose-dependent manner (89). Hyalomin-A and –B and Amregulin significantly inhibited adjuvant-induced paw inflammation in mouse models in vivo (88, 89). HlSerpin-a and –b, novel serpins from the hard tick H. Longicornis, suppressed the expression of TNF-α, IL-6, and IL-1β from LPS-stimulated mouse bone marrow-derived macrophages (BMDMs) or mouse bone marrow-derived dendritic cells (BMDCs) (90). Three salivary gland serpins, AAS27 and AAS41 from A. americanum (91, 92) and IRS-2 from I. ricinus (93), inhibited inflammation by targeting chymase (Figure 2), an enzyme produced by activated MCs. IRS-2 also inhibited Cathepsin G, which is involved in tissue remodeling during inflammation and modulates the production of IL-6 by DCs, which subsequently impairs Th17 differentiation and maturation. DsCystatin from Dermacentor silvarum salivary glands was shown to impair the expression of the inflammatory cytokines IL-1β, IFN-γ, TNF-α, and IL-6 from mouse BMDMs (94), and the authors proposed that DsCystatin might be useful for the treatment of inflammatory diseases since it suppressed joint inflammation induced by complete Freund’s adjuvant (CFA) and Borrelia burgdorferi in a mouse arthritis model (95).
Another category of anti-inflammatory compounds are non-proteinaceous substances. It is important to point out that bioactive lipidic salivary component despite being abundant in the saliva of hard ticks; mainly prostaglandins PGE2 and PGF2α, only a few studies describe their pharmaceutical use (96, 97). Cannabinoids have also been detected in Amblyomma ticks and have been proposed to act as analgesic and anti-inflammatory compounds (98). However, these lipid derivatives have not been thoroughly investigated and require more rigorous investigation to assess their pharmacological interest. The two most documented non-proteinaceous compounds are purine nucleoside adenosine (Ado) and prostaglandin PGE2 (Figure 2) present in the saliva of R. sanguineus (99). Both compounds impaired the production of pro-inflammatory IL-12p40 and TNF-α and stimulated the release of anti-inflammatory IL-10 by murine DCs (99). In humans, Ado is a homeostatic regulator and a “danger signal” for cells and organs, since it is expressed during trauma or stress (100, 101). The therapeutic potential of Ado is well documented elsewhere (100–102). Ado is protective against several pathologies including inflammation and various forms of neuronal hyperexcitability and/or toxicity including hypoxia, seizures, and chronic pain (101). Similarly, PGE2 has pharmacological proprieties in vitro and in vivo associated with inflammation (103) and cancer (97) influencing cell proliferation, apoptosis, angiogenesis, inflammation, and immune surveillance (104). Thus, Ado and PGE2 derived from R. sanguineus saliva could be used for therapeutic purposes.
Complement System
The complement system was originally regarded as a support to innate immunity against microbial invaders (45). More recently, complement has been recognized as having functions beyond microbial elimination such as clearance of immune complexes (105), complementing T and B cell immune functions (36), and tissue regeneration (106). Complement links the host innate and adaptive immune responses and is activated via three pathways (alternative, classical, and lectin) (31, 85). The excessive activation of complement components is responsible for a wide range of immune-mediated diseases (107) including autoimmune diseases, Alzheimer’s disease, schizophrenia, atypical hemolytic-uremic syndrome, angioedema, macular degeneration, and Crohn’s disease (108). Since the introduction of the first complement-specific drug, eculizumab, into the clinic, over 20 candidate drugs are now being evaluated in clinical trials and additional agents are in preclinical development (109). Several molecules with promising anti-complement activities were identified in tick salivary glands. For instance, Isac from I. scapularis (110) and its paralogs IRAC I and II from I. ricinus (111) specifically blocked binding of complement factor B to complement C3b in vitro, inhibiting the formation of the C3 convertase of the alternative pathway. Since convertases mediate nearly all complement effector functions, they are ideal targets for therapeutic inhibition (112). Selective inhibition of complement precursors and regulators is also of great therapeutic interest (109). Salp20 from I. scapularis (113) and IxACs from I. ricinus (114) inhibit the alternative pathway by binding properdin, a positive regulator of the pathway. Soft ticks have been reported to inhibit the classical complement pathway, with the lipocalins OmCI from O. moubata (115, 116) and TSGP2 and TSGP3 from Ornithodoros savignyi (117) specifically targeting C5 activation. The recombinant version of OmCI (also known as Coversin or rEV576) has been tested in several animal models of complement-mediated diseases and has shown efficacy in a clinical trial (115, 118, 119). By inhibiting C5 activation, OmCI significantly reduced excessive inflammatory reactions associated with severe forms of influenza virus A (IAV) in mice (116). In addition to its effect on C5, OmCI significantly decreased leukotriene B4 levels in septic pigs (120). In the same study, combined inhibition of complement C5 by OmCI and CD14 with anti-CD14 antibodies significantly attenuated inflammation, thrombogenicity, and hemodynamic abnormalities (115, 120), suggesting OmCI might be useful for the treatment of sepsis. OmCI was also effective in preventing experimental autoimmune myasthenia gravis induced by passive transfer in normal Lewis rats (118). RaCI, from R. appendiculatus salivary glands, bound human C5 and blocked C5a generation and membrane attack complex formation (121). RaCI exhibits cross-species reactivity, allowing it to be used in disease models to test therapeutic effectiveness. An OmCI and RaCI homolog, CirpT, is a new class of complement inhibitor identified in Rhipicephalus pulchellus saliva that binds C5 in vitro via a unique binding site that does not overlap with other known inhibitors (122). Eculizumab, an antibody against C5, is currently prescribed for diseases characterized by excessive activation of the alternative pathway, including paroxysmal nocturnal hemoglobinuria and atypical hemolytic uremic syndrome (123, 124). Despite its effectiveness, eculizumab is one of the most expensive drugs in the world (121). Nevertheless, these other proteins derived from tick saliva offer exciting prospects for C5-targeted therapeutics of complement-mediated diseases and could be alternatives to eculizumab. In addition to the alternative pathway, the lectin pathway is also targeted by the tick salivary lectin pathway inhibitor (TSLPI), identified from the salivary glands of I. scapularis (125). The lectin pathway is activated in an antibody and C1-independent manner, upon the interaction of Mannose-binding lectin (MBL) with hyper glycosylated pathogen-associated molecular patterns (PAMPs) on microbial surfaces (126). TSLPI was described to directly inhibit MBL complement pathway activation (125). Additionally, both TSLPI and its ortholog from I. ricinus were shown to protect Borrelia burgdorferi sensu lato from complement-mediated killing, by inhibiting MBL function (127, 128). MBL appears to influence the severity of several diseases including pancreatic ductal adenocarcinoma (PDAC) (129). During a Pancreatic Cancer, MBL is required for oncogenic progression, whereas inhibition of MBL by TSLPI could be protective against tumor growth.
Acquired Immune Responses
During the first encounter with ticks, antigen-presenting cells, mainly DCs, present salivary immunogens to lymphocytes, which trigger cell-mediated and antibody responses (22). Sets of immunoglobulins and long-lived memory T and B cells are induced in the host (Figure 3). In subsequent contacts with the same tick, acquired immunity is quickly activated to yield a more specific protective response. It has been reported that tick saliva suppresses adaptive immunity in various ways (33). Some tick salivary compounds interfere with DCs and alter their capacity to present antigens. Other compounds prevent lymphocyte proliferation or T cell cytokine production.
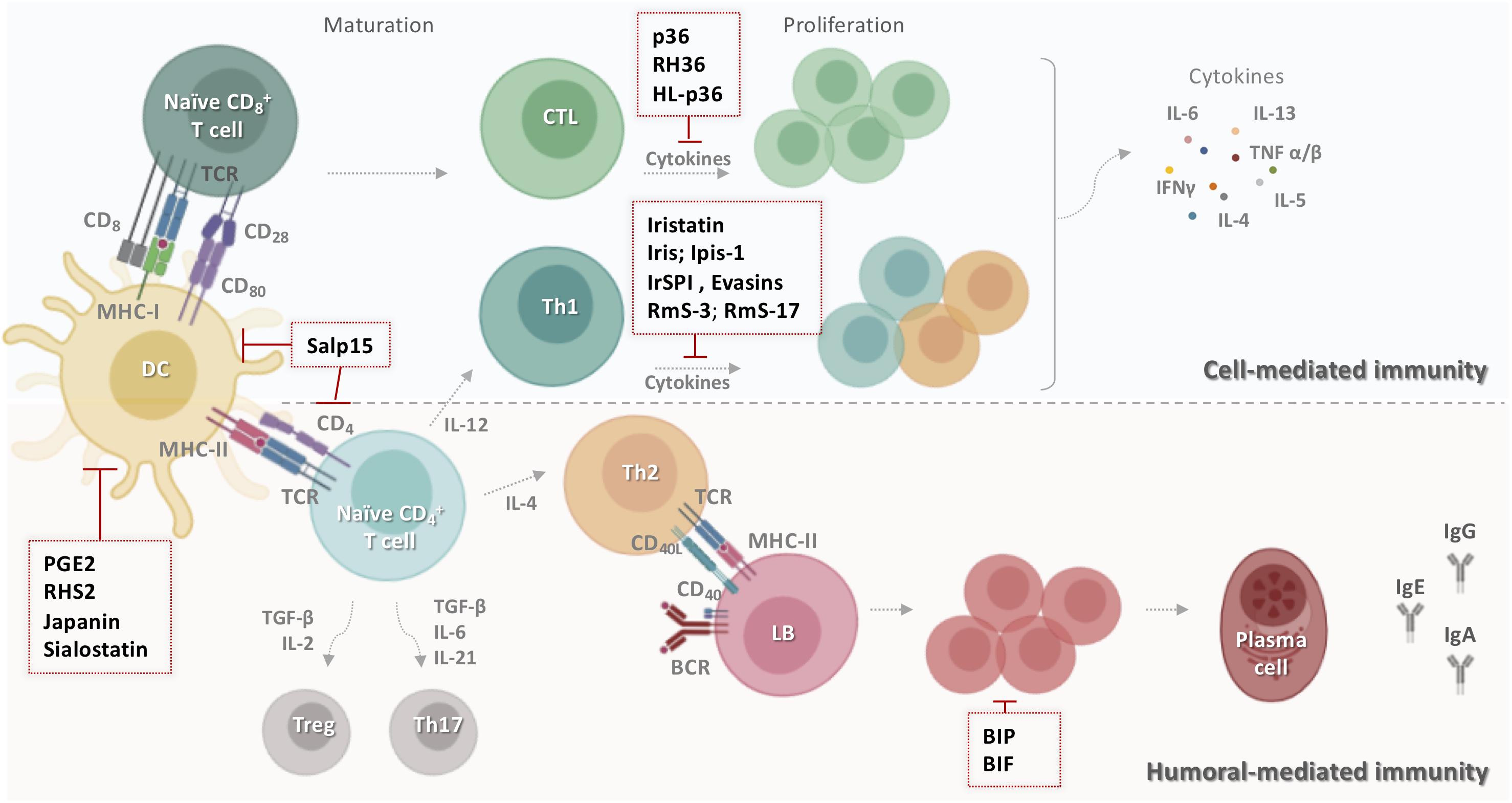
Figure 3. Schematic representation of tick salivary molecules targeting adaptive immunity. During the primary contact with ticks, dendritic cells present salivary antigens to lymphocytes, which trigger cell- and humoral-mediated responses. Some tick salivary molecules prevent the initiation of adaptive immunity by targeting DCs, including Salp15, PGE2, RHS2, Japanin, and sialostatin. Other compounds block the proliferation of lymphocytes and/or inhibit the production of T cell cytokines such as p36, RH36, HL-p36, Iristatin, Iris, Ipis-1, IrSPI, Evasins, RmS-3, and RmS-17. However, BIP and BIF disarm humoral host immunity by inhibiting B cell responses, including the production of specific anti-tick antibodies. BCR: B cell receptor; BIF: B cell inhibitory factor; BIP: B cell inhibitory protein; CD: cluster of differentiation; CTL: cytotoxic T lymphocyte; DC: dendritic cells; HL-p36: Haemaphysalis longicornis p-36; IFN-γ: interferon gamma; Ig: immunoglobulin; IL: interleukin; LB: lymphocyte B; MHC: major histocompatibility complex; PGE2: prostaglandin E2; RmS: Rhipicephalus microplus serpin; Salp: salivary protein; TCR: T cell receptor; TGF-β: transforming growth factor-beta; Th: T helper; TNF: tumor necrosis factor; Treg: regulatory T cell.
Antigen Processing and Presentation
Several tick salivary molecules target DCs, thereby preventing the initiation of adaptive immunity. PGE2 has been described as the major DC inhibitor (Figure 3) in both I. scapularis (130) and Amblyomma sculptum saliva (131). RHS2 from Rhipicephalus haemaphysaloides can inhibit the differentiation of BMDCs into DCs in vitro and promote their differentiation into macrophages (132). RHS2 also inhibits the expression of CD80, CD86, and the major histocompatibility complex class II (MHCII), thereby preventing DC maturation. DC differentiation from monocytes is blocked by Japanin (Figure 3), a salivary gland lipocalin from R. appendiculatus (133). Moreover, Japanin was found to reprogram DC responses to a broad variety of stimuli in vitro, radically altering the expression of co-stimulatory and co-inhibitory molecules and modifying the production of diverse cytokines (134).
Humoral Immunity
Tick salivary components also disarm host humoral immunity by inhibiting B cells responses, including their production of specific anti-tick antibodies (134). BIP (B cell inhibitory protein) (Figure 3) derived from I. ricinus inhibited B lymphocyte proliferation induced by B. burgdorferi lipoproteins (135). Similarly, BIF (B cell inhibitory factor) from H. asiaticum asiaticum salivary glands (Figure 3) was found to suppress B cell responses (136).
Cell-Mediated Immunity
Numerous inhibitors of T cell functions have been identified in tick saliva and salivary glands. Three immunosuppressant salivary proteins, p36 from Dermacentor andersoni (137, 138), HL-p36 from H. longicornis (139), and RH36 from R. haemaphysaloides (140) suppressed T lymphocyte proliferation in vitro. Recombinant HL-p36 and RH36 also directly inhibited the proliferation of several mitogen-stimulated cells in vivo and the expression of several cytokines such as IL-2, IL-12, and TNF-α (141, 142). In addition to its effect on DCs, RHS2 can prevent the activation of CD4+ and CD8+ T cells, leading to inhibition of the Th1 immune response (132). Two salivary cystatins from I. scapularis, Sialostatin L and Sialostatin L2, have shown promising anti-inflammatory and immunosuppressive activity in vitro and in vivo (141, 142). Sialostatin L was immunosuppressive in mammalian models of immune-related diseases, suppressing the proliferation of both CD4 and CD8 T cells, neutrophil migration in severe inflammation, and the secretion of cytokines by MCs, DCs, and lymphocytes (130, 141). Sialostatin L reduced the release of IL-9 by Th9 cells, which might explain its suppression of airway hyperresponsiveness and eosinophilia in an experimental asthma model (143). In the experimental autoimmune encephalomyelitis (EAE) mouse model of multiple sclerosis, in vivo administration of Sialostatin L significantly prevented disease symptoms (144). Furthermore, Sialostatin L effectively altered lysosomal cysteine cathepsins L, C, V, S, X and papain activity, critical elements in antigen presentation (141). Sialostatin L2 suppressed IFN-β-mediated immune reactions in murine DCs (145), and was also found to inhibit cathepsins C, L, S, and V and decrease IL-1β and IL-18 secretion by macrophages (146). Similar to sialostatins, Iristatin, a novel type 2 cystatin from I. ricinus, inhibited the proteolytic activity of cathepsins L and C (147) and diminished IL-2, IL-4, IL-9, and IFN-γ production by different T cell populations, IL-6 and IL-9 production by MCs, and nitric oxide production by macrophages. Iristatin inhibited OVA antigen-induced CD4+ T cell proliferation and leukocyte recruitment in vivo and in vitro (Figure 3). With such a wide spectrum of immunosuppressive activities, Iristatin may be exploitable as an immunotherapeutic.
T cell inhibitory salivary molecules also include Iris and IrSPI from I. ricinus (148), Ipis-1 from I. persulcatus (149), RmS-3 and RmS-17 from R. microplus (61), and Salp15 from I. scapularis (47). Iris was found to suppress T cell proliferation, promote a Th2-type response, and bind to monocytes/macrophages, inhibiting their ability to secrete pro-inflammatory cytokines IL-6 and TNF-α (150). Similarly, IrSPI suppressed proliferation of CD4+ T lymphocytes and pro-inflammatory cytokine secretion (Figure 3) from splenocytes and macrophages (151). The Iris homolog Ipis-1 inhibited cellular proliferation and the production of IFN-γ in bovine peripheral blood mononuclear cells, suggesting that Ipis could directly interact with T cells and inhibit their functions (149). RmS-3 and RmS-17 inhibited the metabolic activity of lymphocytes, decreasing lymphocyte proliferation (61). One of the most extensively studied components of tick saliva is Salp15, an immunosuppressive cysteine-rich glycoprotein (47). Salp15 binds specifically to CD4 co-receptors on the surface of T lymphocytes, interfering with TCR-mediated signaling transduction and impeding IL-2 secretion in a dose-dependent manner (47, 148, 149). A subsequent study reported that Salp15 interacts with DC-SIGN on DCs, triggering a novel Raf-1/MEK-dependent signaling pathway, thereby impairing cytokine production and T cell proliferation (152). Apart from its effect on adaptive immunity, Salp15 inhibited TLR2-dependent keratinocyte inflammation in vitro (153). Together, these findings make this salivary protein a potential candidate for the treatment of T cell-mediated autoimmune diseases. Indeed, the immunomodulatory effect of Salp15 has been investigated in various models in vivo. Paveglio and colleagues (154) were the first to show that Salp15 has a therapeutic effect in a mouse model of allergic asthma. The specific binding of Salp15 to CD4 inhibited the proliferation and differentiation of CD4+ T cells toward Th2 cells and suppressed the production of inflammatory cytokines, significantly reducing symptoms of allergic asthma in treated mice. Moreover, Salp15 prevented the association of HIV-1 gp120 and CD4 in an experimental HIV infection (155). gp120 is an HIV-1 envelope glycoprotein which, by binding to CD4, promotes penetration of HIV-1 into the host cell (156). The authors suggested that Salp15 competes with gp120 for an association with CD4 due to its ability to interact with CD4 on T cells. Another investigation showed the opposite effect in murine EAE/multiple sclerosis (157). The occurrence and progression of EAE is associated with myelin-specific CD4+ T cell activation and secretion of IL-17 and INF-γ by Th17 and Th1 cells, respectively (158). Unexpectedly, Salp15 worsened the pathology in mice upon induction of EAE. A possible reason for this might be that Salp15 promoted Th17 activation, increasing IL-17 levels in vivo, and it also enhanced Th17 differentiation in the presence of IL-6 and absence of TGF-β in vitro (157). More recently, it was shown that Salp15 binding to CD4 is persistent and induces a long-lasting immunomodulatory effect in a murine model of hematopoietic transplantation (159). Based on these results, Salp15 could provide important opportunities for the development of novel and sophisticated therapies for human disease mainly those mediated by multiple processes.
Tick Non-Coding RNAs: New Alternatives in Targeted Immunomodulatory Therapy?
Tick salivary molecules are pluripotent (i.e., one molecule may affect more than one host cell population) and show considerable functional redundancy (i.e., the same host cell population may be targeted by more than one tick salivary molecule) (160). Tick saliva not only contains proteins and metabolites but also non-proteinaceous species including nucleic acids (22, 161) and RNA silencing signals, which are now recognized as crucial for cross-species communication across diverse biological niches (162, 163). With respect to the tripartite tick-pathogen-host interaction, recent transcriptomic and proteomic projects, facilitated by rapid developments in high-throughput sequencing, have revealed several tick genes/transcripts of unknown function or transcripts without an open reading frame (ORF) (160). These projects have also revealed a significant number of ncRNAs in tick saliva that are predicted to be functionally involved in the vector-host interaction (164). ncRNAs are important in vector-host interactions at the tick-host interface: ticks use ncRNAs, broadly divided by length into long non-coding RNAs (lncRNAs) (>200 nucleotides (nt) long) and small non-coding RNAs (sncRNAs) (<200 nt long), to hijack host defense mechanisms (165). They are suggested to be secreted via exosomes (166, 167) and released in the host cells, to manipulate gene expression and deregulate host defense pathways (165). LncRNAs are not translated into proteins and include mRNA-like intergenic transcripts (lincRNAs), antisense transcripts of protein-coding genes, and primary RNA polymerase II (Pol II) transcript-derived unconventional lncRNAs (168). LncRNAs are involved in numerous important biological phenomena such as imprinting genomic loci, shaping chromosome conformation and allosterically regulating enzymatic activity (169). On the other hand, sncRNAs represent a diverse set of molecules that control the expression of most vertebrate genes and include small-interfering RNAs (siRNAs), microRNAs (miRNAs), and piwi-interacting RNAs (piRNAs) (170). miRNAs have been extensively studied in several organisms and their function in gene regulation is well understood. The first attempt to identify host targets of tick ncRNAs was performed by Hackenberg and colleagues (171). Their in silico study showed that saliva-specific miRNAs from I. ricinus might have combinatorial effects on the host targetome: different tick miRNAs may target vertebrate host genes in the same host homeostatic pathway, and the expression of a given host gene may also be regulated by more than one saliva-specific tick miRNA (171). The authors suggested combinatorial effects of vector miRNAs on host target genes, which may be of importance in evolutionary terms to maintain robust regulation of host genes and pathways important in the tick-host interaction. miR-8-3p, bantam-3p, mir-317-3p, and miR-279a-3p from I. ricinus were predicted to target host KEGG pathways such as “gap junction” and “inflammatory mediator regulation of TRP channels,” which play a role in the host homeostatic response (Figure 4A).
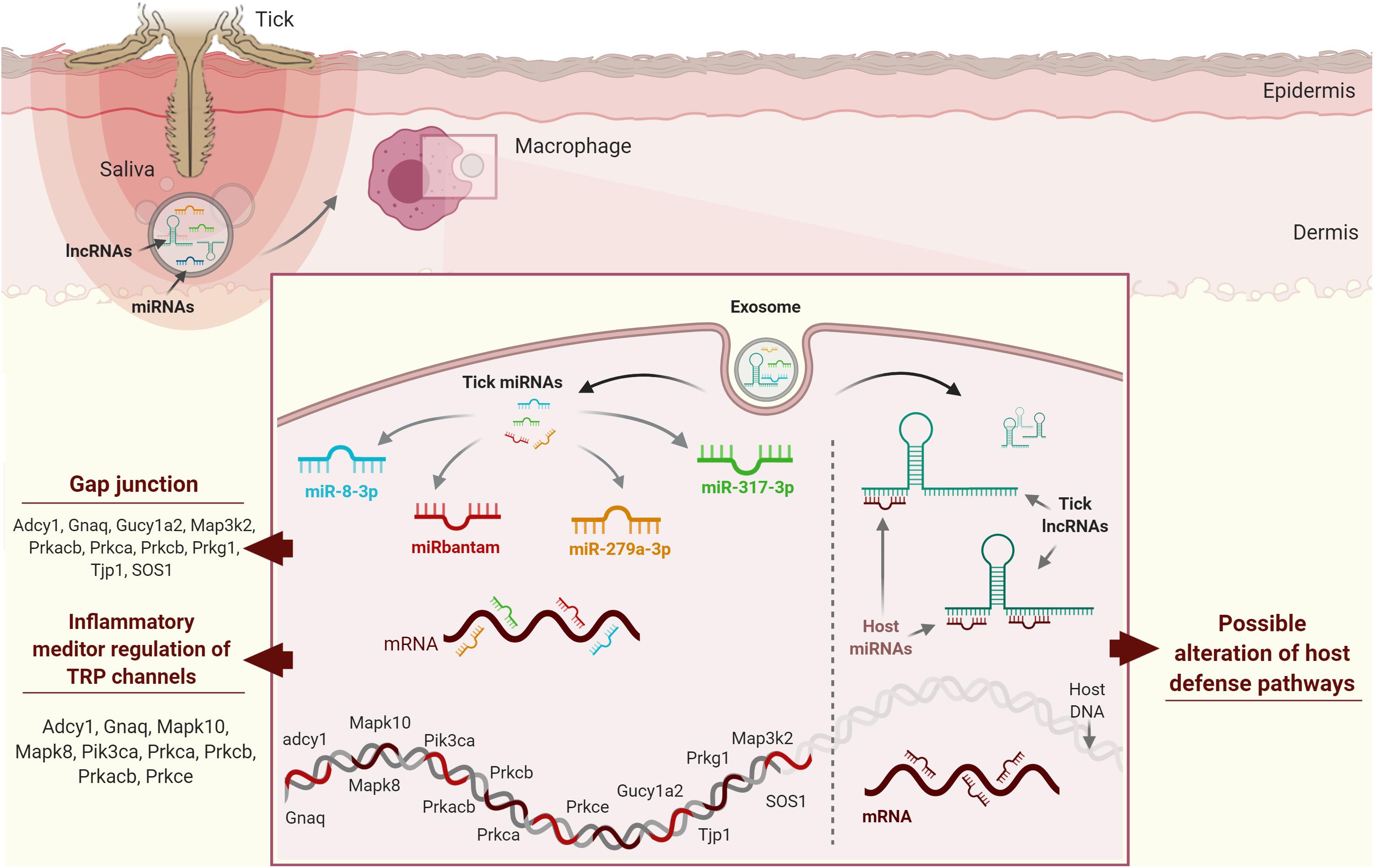
Figure 4. Possible strategies used by tick ncRNAs in host cells. (A) miR-8-3p, bantam-3p, mir-317-3p, and miR-279a-3p from Ixodes ricinus have been predicted to target gap junctions and inflammatory mediator regulation of TRP channels, which play a role in host homeostatic responses. (B) Host miRNAs regulate protein coding genes through mRNA cleavage and/or direct translational repression and/or mRNA destabilization. Ticks secrete exosomes in their saliva containing lncRNAs, which may compete with host mRNAs for host miRNA binding by acting as “sponge” molecules that inhibit host miRNA interactions with host mRNAs, thus affecting host homeostatic responses to tick feeding.
The huge number of ncRNA sequences revealed by high-throughput projects suggests that tick ncRNAs might also modulate host gene expression by binding to regulatory host miRNAs involved in host immune cell responses. Tick ncRNAs could regulate protein-coding genes through mRNA cleavage and/or direct translational repression and/or mRNA destabilization. We hypothesize that lncRNAs compete with host mRNAs for host miRNA binding by acting as “sponge” molecules that inhibit host miRNA interactions with host mRNAs, thus affecting host homeostatic responses to tick feeding (Figure 4B). In silico predictions now need to be validated using systems-based approaches in order to characterize tick ncRNAs and to understand their involvement in tick-host interactions. Various academic and commercial research groups are now exploring ncRNA-based therapies and exploring the potential of miRNA therapeutics, as miRNAs are the most studied ncRNAs to date (172). A few miRNAs have entered preclinical and clinical testing, so might soon be available on the market for use in humans. As tick ncRNAs are likely to be less immunogenic by their intrinsic ability to hijack and bypass host immunity, we strongly believe that this flourishing field might lead to ncRNA therapeutics useful for the treatment of various diseases. Emerging data on the possible regulatory function of ncRNAs, the vector, the pathogen, and the host, the role of ncRNA in the vector or host-pathogen remain quite challenging, due to several biological and technical aspects. For example, the used techniques for ncRNAs study can generate several biases in the results. New scRNA-seq technology that is showing its efficiency to counter this issue, but sufficient read depths of rarely expressed RNAs can also be misleading, and vector, host or pathogen-derived RNAs information could be easily lost. LncRNAs data analyses also remain controversial due to the lack of accurate transcript models in current annotations. LncRNAs could also be pluripotent, and just as the salivary proteins, this would also be a serious limitation in any potential therapeutic applications. Overall, given the arising data on the ability of ncRNAs produced in tick salivary glands and also the possible regulatory function of cellular RNAs on the vector, pathogen or host, the role of ncRNA in the vector or host-pathogen crosstalk might be even more sophisticated than what is expected. Development of research projects that employ single-cell/single molecule sequencing methodologies and do not require nucleic acid amplification will definitely enable less biased and reliable data output.
Concluding Remarks
Inflammatory, autoimmune, metabolic, and neurodegenerative diseases remain critical health problems worldwide, creating an enormous need for new, effective, and specific medicines. Over the last few decades, ticks have shown their “good side” as promising sources of new drugs targeting distinct pathophysiological pathways in mammals. In this review, we summarized potential drug candidates from tick salivary glands that might have therapeutic use in immune disorders and other related diseases. We have also highlighted that tick ncRNAs are possible new alternatives for targeted immunomodulatory therapy. The molecules described in this review have been tested in vitro and/or in various animal models of human disease and have shown encouraging results. However, only a few of them have advanced to (pre-)clinical investigations, and further in-depth molecular and cellular studies are required to further develop candidates for clinical trials. The major challenges to drug development are (i) specificity and efficacy, since broad-spectrum targets can generate unwanted side-effects; and (ii) safety, with drug-induced toxicity and immunogenicity critical limitations of many drug candidates. Addressing these challenges, tick salivary molecules, at least in theory, might have a somewhat higher clinical success rate than molecules from other origins, since many tick-derived products have an intrinsically low risk of toxicity and immunogenicity and high target specificity to execute their intended functions. From the pharmaceutical perspective, some molecules might be unstable or have a short half-lives, so pharmacokinetic, dose optimization, and modification studies are critical research priorities. While experimental validation and ultimately clinical trialing remain the cornerstone of successful therapeutic development, computational methods might play a role as time-saving, cost-effective, and productive approaches to design and discover novel lead compounds for associated disease targets. Modeling and simulation of preclinical and clinical trials could also be used to bridge the gap between the early stages of drug development and their potential effects on humans.
Author Contributions
All authors searched and read the literature and edited the manuscript. HA, CB, and MK wrote the manuscript.
Funding
This study was financed by the Coordenação de Aperfeiçoamento de Pessoal de Nível Superior – Brazil (CAPES) – Finance Code 001; by FAPESP (CETICS: 2013/07467–1) and Fundação Butantan. It was also financed by the Grant Agency of the Czech Republic (Grant19-382 07247S to MK and 19-14704Y to JC) and by ERD Funds, project CePaVip OPVVV (No. 384 CZ.02.1.01/0.0/0.0/16_019/0000759 to MK).
Conflict of Interest
The authors declare that the research was conducted in the absence of any commercial or financial relationships that could be construed as a potential conflict of interest.
Abbreviations
AAS, Amblyomma americanum serpin; BIF, B cell inhibitory factor; BIP, B cell inhibitory protein; BMDCs, mouse bone marrow-derived dendritic cells; BMDMs, mouse bone marrow-derived macrophages; CD, cluster of differentiation; CFA, complete freund’s adjuvant; DC, dendritic cells; EAE, experimental autoimmune encephalomyelitis; HIV, human immunodeficiency viruses; HL-p36, Haemaphysalis longicornis p-36; IFN-γ, interferon gamma; IL, interleukin; Irac, Ixodes ricinus anticomplement; IRS-2, Ixodes ricinus Serpin- 2; Isac, Ixodes scapularis salivary anticomplement; LPS, lipopolysaccharide; MBL, mannose-binding lectin; MC, Mast cells; MCP-1 monocyte chemoattractant protein 1; MHC-I, -II, major histocompatibility complex-I, -II; MIF, macrophage migration inhibitory factor; MS, mass spectrometry; NGS, next-generation sequencing; OmCI, Ornithodoros moubata complement inhibitor; PDAC, pancreatic ductal adenocarcinoma; PGE2, prostaglandin E2; Ra-HBPs, Rhipicephalus appendiculatus histamine-binding proteins; RmS-3, Rhipicephalus microplus serpin 3; Salp, salivary protein; SHBP, serotonin and histamine-binding protein; TCR, T cell receptor; TdPI, Tick-derived protease inhibitor; TGF-β, transforming growth factor-beta; Th, T helper; TLR, toll-like receptors; TNF, tumor necrosis factor; TSLPI, tick salivary lectin pathway inhibitor.
References
1. Raje N, Dinakar C. Overview of immunodeficiency disorders. Immunol Allergy Clin North Am. (2015) 35:599–623. doi: 10.1016/j.iac.2015.07.001
2. Wang L, Wang FS, Gershwin ME. Human autoimmune diseases: a comprehensive update. J Int Med. (2015) 278:369–95. doi: 10.1111/joim.12395
3. Bogdanos DP, Smyk DS, Rigopoulou EI, Mytilinaiou MG, Heneghan MA, Selmi C, et al. Twin studies in autoimmune disease: genetics, gender and environment. J Autoimmun. (2012) 38:J156–69. doi: 10.1016/j.jaut.2011.11.003
4. Mahoney EJ, Veling MC, Mims JW. Food allergy in adults and children. Otolaryngol Clin North Am. (2011) 44:815–33. doi: 10.1016/j.otc.2011.03.014
5. Peden DB. Effect of pollution on allergy/immunology. J Allergy Clin Immunol. (2018) 141:878–9. doi: 10.1016/j.jaci.2018.01.017
6. Nikolich-Žugich J. The twilight of immunity: emerging concepts in aging of the immune system. Nat Immunol. (2018) 19:10–9. doi: 10.1038/s41590-017-0006-x
7. Krones E, Högenauer C. Diarrhea in the immunocompromised patient. Gastroenterol Clin North Am. (2012) 41:677–701. doi: 10.1016/j.gtc.2012.06.009
8. Girish MB. Emerging drugs for the treatment of human immunodeficiency virus. Recent Pat AntiInfect Drug Discov. (2012) 7:45–52. doi: 10.2174/157489112799829729
9. Bucktrout SL, Bluestone JA, Ramsdell F. Recent advances in immunotherapies: from infection and autoimmunity, to cancer, and back again. Genome Med. (2018) 10:79. doi: 10.1186/s13073-018-0588-4
10. Li G, Lou H-X. Strategies to diversify natural products for drug discovery. Med Res Rev. (2018) 38:1255–94. doi: 10.1002/med.21474
11. Lautié E, Russo O, Ducrot P, Boutin JA. Unraveling plant natural chemical diversity for drug discovery purposes. Front Pharmacol. (2020) 11:397. doi: 10.3389/fphar.2020.00397
12. Uzair B, Bint-e-Irshad S, Khan BA, Azad B, Mahmood T, Rehman MU, et al. Scorpion venom peptides as a potential source for human drug candidates. Protein Pept Lett. (2018) 25:702–8. doi: 10.2174/0929866525666180614114307
13. Perumal Samy R, Stiles BG, Franco OL, Sethi G, Lim LHK. Animal venoms as antimicrobial agents. Biochem Pharmacol. (2017) 134:127–38. doi: 10.1016/j.bcp.2017.03.005
14. Franchin M, Freires IA, Lazarini JG, Nani BD, da Cunha MG, Colón DF, et al. The use of Brazilian propolis for discovery and development of novel anti-inflammatory drugs. Eur J Med Chem. (2018) 153:49–55. doi: 10.1016/j.ejmech.2017.06.050
15. Katz L, Baltz RH. Natural product discovery: past, present, and future. J Ind Microbiol Biotechnol. (2016) 43:155–76. doi: 10.1007/s10295-015-1723-5
16. Gileadi O. Recombinant protein expression in E. coli: a historical perspective. Methods Mol Biol. (2017) 1586:3–10. doi: 10.1007/978-1-4939-6887-9_1
17. Baghban R, Farajnia S, Ghasemi Y, Mortazavi M, Zarghami N, Samadi N. New developments in Pichia pastoris expression system, review and update. Curr Pharm Biotechnol. (2018) 19:451–67. doi: 10.2174/1389201019666180718093037
18. Rozov SM, Permyakova NV, Deineko EV. Main strategies of plant expression system glycoengineering for producing humanized recombinant pharmaceutical proteins. Biochemistry. (2018) 83:215–32. doi: 10.1134/S0006297918030033
19. Metzker ML. Sequencing technologies the next generation. Nat Rev Genet. (2010) 11:31–46. doi: 10.1038/nrg2626
20. Wingfield J, Wilson ID. Advances in mass spectrometry within drug discovery. J Biomol Screen. (2016) 21:109–10. doi: 10.1177/1087057115623454
21. Chmelar J, Kotál J, Kovaríková A, Kotsyfakis M. The use of tick salivary proteins as novel therapeutics. Front Physiol. (2019) 10:812. doi: 10.3389/fphys.2019.00812
22. Francischetti IMB, Sa-Nunes A, Mans BJ, Santos IM, Ribeiro JMC. The role of saliva in tick feeding. Front Biosci. (2009) 14:2051–88. doi: 10.2741/3363
23. Chmelar J, Calvo E, Pedra JHF, Francischetti IMB, Kotsyfakis M. Tick salivary secretion as a source of antihemostatics. J Proteomics. (2012) 75:3842–54. doi: 10.1016/j.jprot.2012.04.026
24. Boulanger N. [Immunomodulatory effect of tick saliva in pathogen transmission]. Biolo Aujourd’hui. (2018) 212:107–17. doi: 10.1051/jbio/2019001
25. Maria DA, Will SEAL, Bosch RV, Souza JG, Sciani JM, Goldfeder MB, et al. Preclinical evaluation of Amblyomin-X, a Kunitz-type protease inhibitor with antitumor activity. Toxicol Rep. (2019) 6:51–63. doi: 10.1016/j.toxrep.2018.11.014
26. Boufleur P, Sciani JM, Goldfeder M, Faria F, Branco V, Chudzinski-Tavassi AM. Biodistribution and pharmacokinetics of Amblyomin-X, a novel antitumour protein drug in healthy mice. Eur J Drug Metab Pharmacokinet. (2019) 44:111–20. doi: 10.1007/s13318-018-0500-z
27. Jongejan F, Uilenberg G. The global importance of ticks. Parasitology. (2004) 129:S3–14. doi: 10.1017/S0031182004005967
28. Sonenshine DE. Biology of ticks. Vet Clin North Am Small Anim Pract. (1991) 21:1–26. doi: 10.1016/S0195-5616(91)50001-2
29. Nuttall PA. Wonders of tick saliva. Ticks Tick Borne Dis. (2019) 10:470–81. doi: 10.1016/j.ttbdis.2018.11.005
30. Castelli E, Caputo V, Morello V, Tomasino RM. Local reactions to tick bites. Am J Dermatopathol. (2008) 30:241–8. doi: 10.1097/DAD.0b013e3181676b60
31. Bernard Q, Grillon A, Lenormand C, Ehret-Sabatier L, Boulanger N. Skin interface, a key player for Borrelia multiplication and persistence in lyme borreliosis. Trends Parasitol. (2020) 36:304–14. doi: 10.1016/j.pt.2019.12.017
32. Hermance ME, Thangamani S. Tick–virus–host interactions at the cutaneous interface: the nidus of flavivirus transmission. Viruses. (2018) 10:362. doi: 10.3390/v10070362
33. Kazimírová M, Štibrániová I. Tick salivary compounds: their role in modulation of host defences and pathogen transmission. Front Cell Infect Microbiol. (2013) 3:43. doi: 10.3389/fcimb.2013.00043
34. Versteeg HH, Heemskerk JWM, Levi M, Reitsma PH. New fundamentals in hemostasis. Physiol Rev. (2013) 93:327–58. doi: 10.1152/physrev.00016.2011
35. Chudzinski-Tavassi AM, Faria F, Flores MPA. Anticoagulants from hematophagous. In: MB Mijovski editor. Anticoagulant Drugs. London: InTech. (2018). doi: 10.5772/intechopen.78025.
36. Carroll MC, Isenman DE. Regulation of humoral immunity by complement. Immunity. (2012) 37:199–207. doi: 10.1016/j.immuni.2012.08.002
37. Dunkelberger JR, Song WC. Complement and its role in innate and adaptive immune responses. Cell Res. (2010) 20:34–50. doi: 10.1038/cr.2009.139
38. Wikel S. Ticks and tick-borne pathogens at the cutaneous interface: host defenses, tick countermeasures, and a suitable environment for pathogen establishment. Front Microbiol. (2013) 4:337. doi: 10.3389/fmicb.2013.00337
39. Glatz M, Means T, Haas J, Steere AC, Müllegger RR. Characterization of the early local immune response to Ixodes ricinus tick bites in human skin. Exp Dermatol. (2017) 26:263–9. doi: 10.1111/exd.13207
41. Krause PJ, Grant-Kels JM, Tahan SR, Dardick KR, Alarcon-Chaidez F, Bouchard K, et al. Dermatologic changes induced by repeated Ixodes scapularis bites and implications for prevention of tick-borne infection. Vector Borne Zoonotic Dis. (2009) 9:603–10. doi: 10.1089/vbz.2008.0091
42. Kiripolsky J, McCabe LG, Kramer JM. Innate immunity in Sjögren’s syndrome. Clin Immunol. (2017) 182:4–13. doi: 10.1016/j.clim.2017.04.003
43. Cepika AM, Sato Y, Liu JMH, Uyeda MJ, Bacchetta R, Roncarolo MG. Tregopathies: monogenic diseases resulting in regulatory T-cell deficiency. J Allergy Clin Immunol. (2018) 142:1679–95. doi: 10.1016/j.jaci.2018.10.026
44. Anderton SM. Peptide immunotherapy in experimental autoimmune encephalomyelitis. Biomed J. (2015) 38:206–14. doi: 10.4103/2319-4170.158510
45. Morgan BP, Harris CL. Complement, a target for therapy in inflammatory and degenerative diseases. Nat Rev Drug Discov. (2015) 14:857–77. doi: 10.1038/nrd4657
46. Anguita J, Ramamoorthi N, Hovius JWR, Das S, Thomas V, Persinski R, et al. Salp15, an Ixodes scapularis salivary protein, inhibits CD4(+) T cell activation. Immunity. (2002) 16:849–59. doi: 10.1016/s1074-7613(02)00325-4
47. Wen S, Wang F, Ji Z, Pan Y, Jian M, Bi Y, et al. Salp15, a multifunctional protein from tick saliva with potential pharmaceutical effects. Front Immunol. (2019) 10:3067. doi: 10.3389/fimmu.2019.03067
48. Yang T, Sui X, Yu B, Shen Y, Cong H. Recent advances in the rational drug design based on multi-target ligands. Curr Med Chem. (2020) 27:4720–40. doi: 10.2174/0929867327666200102120652
49. Kumar A, Tiwari A, Sharma A. Changing paradigm from one target one ligand towards multi-target directed ligand design for key drug targets of alzheimer disease: an important role of in silico methods in multi-target directed ligands design. Curr Neuropharmacol. (2018) 16:726–39. doi: 10.2174/1570159X16666180315141643
50. Talevi A. Multi-target pharmacology: possibilities and limitations of the “skeleton key approach” from a medicinal chemist perspective. Front Pharmacol. (2015) 6:205. doi: 10.3389/fphar.2015.00205
51. Talevi A. Tailored multi-target agents. applications and design considerations. Curr Pharm Des. (2016) 22:3164–70. doi: 10.2174/1381612822666160308141203
52. Kotál J, Langhansová H, Lieskovská J, Andersen JF, Francischetti IMB, Chavakis T, et al. Modulation of host immunity by tick saliva. J Proteomics. (2015) 128:58–68. doi: 10.1016/j.jprot.2015.07.005
53. Almazan C, Tipacamu GA, Rodriguez S, Mosqueda J, Perez de Leon A. Immunological control of ticks and tick-borne diseases that impact cattle health and production. Front Biosci. (2018) 23:1535–51. doi: 10.2741/4659
54. Šimo L, Kazimirova M, Richardson J, Bonnet SI. The essential role of tick salivary glands and saliva in tick feeding and pathogen transmission. Front Cell Infect Microbiol. (2017) 7:281. doi: 10.3389/fcimb.2017.00281
55. Štibrániová I, Bartíková P, Holíková V, Kazimírová M. Deciphering biological processes at the tick-host interface opens new strategies for treatment of human diseases. Front Physiol. (2019) 10:830. doi: 10.3389/fphys.2019.00830
56. Schmidt MCB, Morais KLP, Almeida MES, Iqbal A, Goldfeder MB, Chudzinski-Tavassi AM. Amblyomin-X, a recombinant Kunitz-type inhibitor, regulates cell adhesion and migration of human tumor cells. Cell Adh Migr. (2020) 14:129–38. doi: 10.1080/19336918.2018.1516982
57. Lichtenstein F, Iqbal A, de Lima Will SEA, Bosch RV, DeOcesano-Pereira C, Goldfeder MB, et al. Modulation of stress and immune response by Amblyomin-X results in tumor cell death in a horse melanoma model. Sci Rep. (2020) 10:6388. doi: 10.1038/s41598-020-63275-2
58. Pireaux V, Tassignon J, Demoulin S, Derochette S, Borenstein N, Ente A, et al. Anticoagulation with an inhibitor of factors XIa and XIIa during cardiopulmonary bypass. J Am Coll Cardiol. (2019) 74:2178–89. doi: 10.1016/j.jacc.2019.08.1028
59. Yeh CH, Fredenburgh JC, Weitz JI. Oral direct factor Xa inhibitors. Circ Res. (2012) 111:1069–78. doi: 10.1161/CIRCRESAHA.112.276741
60. Borriello F, Iannone R, Marone G. Histamine release from mast cells and basophils. In: Hattori Y, Seifert R editors. Handbook of Experimental Pharmacology. New York: Springer. (2017). p. 1–19. doi: 10.1007/164_2017_18
61. Coutinho ML, Bizzarro B, Tirloni L, Berger M, Freire Oliveira CJ, Sá-Nunes A, et al. Rhipicephalus microplus serpins interfere with host immune responses by specifically modulating mast cells and lymphocytes. Ticks Tick Borne Dis. (2020) 11:101425. doi: 10.1016/j.ttbdis.2020.101425
62. Paesen GC, Adams PL, Harlos K, Nuttall PA, Stuart DI. Tick histamine-binding proteins: isolation, cloning, and three-dimensional structure. Mol Cell. (1999) 3:661–71. doi: 10.1016/S1097-2765(00)80359-7
63. Paesen GC, Adams PL, Nuttall PA, Stuart DL. Tick histamine-binding proteins: lipocalins with a second binding cavity. Biochim Biophys Acta. (2000) 1482:92–101. doi: 10.1016/S0167-4838(00)00168-0
64. Wang Y, Li Z, Zhou Y, Cao J, Zhang H, Gong H, et al. Specific histamine binding activity of a new lipocalin from Hyalomma asiaticum (Ixodidae) and therapeutic effects on allergic asthma in mice. Parasit Vectors. (2016) 9:506. doi: 10.1186/s13071-016-1790-0
65. Sangamnatdej S, Paesen GC, Slovak M, Nuttall PA. A high affinity serotonin- and histamine-binding lipocalin from tick saliva. Insect Mol Biol. (2002) 11:79–86. doi: 10.1046/j.0962-1075.2001.00311.x
66. Mohajeri M, Kovanen PT, Bianconi V, Pirro M, Cicero AFG, Sahebkar A. Mast cell tryptase – marker and maker of cardiovascular diseases. Pharmacol Ther. (2019) 199:91–110. doi: 10.1016/j.pharmthera.2019.03.008
67. Ni WW, Da Cao M, Huang W, Meng L, Wei JF. Tryptase inhibitors: a patent review. Expert Opin Ther Pat. (2017) 27:919–28. doi: 10.1080/13543776.2017.1322064
68. Paesen GC, Siebold C, Harlos K, Peacey MF, Nuttall PA, Stuart DIA. Tick protein with a modified kunitz fold inhibits human tryptase. J Mol Biol. (2007) 368:1172–86. doi: 10.1016/j.jmb.2007.03.011
69. Valdés JJ, Schwarz A, Cabeza de Vaca I, Calvo E, Pedra JHF, Guallar V, et al. Tryptogalinin is a tick kunitz serine protease inhibitor with a unique intrinsic disorder. PLoS One. (2013) 8:e62562. doi: 10.1371/journal.pone.0062562
70. Sommerhoff C, Schaschke N. Mast cell tryptase β as a target in allergic inflammation: an evolving story. Curr Pharm Des. (2006) 13:313–32. doi: 10.2174/138161207779313579
71. Andrade BB, Teixeira CR, Barral A, Barral-Netto M. Haematophagous arthropod saliva and host defense system: a tale of tear and blood. An Acad Bras Cienc. (2005) 77:665–93. doi: 10.1590/S0001-37652005000400008
72. Jaworski DC, Jasinskas A, Metz CN, Bucala R, Barbour AG. Identification and characterization of a homologue of the pro-inflammatory cytokine macrophage migration inhibitory factor in the tick, Amblyomma americanum. Insect Mol Biol. (2001) 10:323–31. doi: 10.1046/j.0962-1075.2001.00271.x
73. Bowen CJ, Jaworski DC, Wasala NB, Coons LB. Macrophage migration inhibitory factor expression and protein localization in Amblyomma americanum (Ixodidae). Exp Appl Acarol. (2010) 50:343–52. doi: 10.1007/s10493-009-9324-5
74. Umemiya R, Hatta T, Liao M, Tanaka M, Zhou J, Inoue N, et al. Haemaphysalis longicornis: molecular characterization of a homologue of the macrophage migration inhibitory factor from the partially fed ticks. Exp Parasitol. (2007) 115:135–42. doi: 10.1016/j.exppara.2006.07.006
75. Beaufays J, Adam B, Menten-Dedoyart C, Fievez L, Grosjean A, Decrem Y, et al. Ir-LBP, an Ixodes ricinus tick salivary ltb4-binding lipocalin, interferes with host neutrophil function. PLoS One. (2008) 3:e3987. doi: 10.1371/journal.pone.0003987
76. Hidano A, Konnai S, Yamada S, Githaka N, Isezaki M, Higuchi H, et al. Suppressive effects of neutrophil by Salp16-like salivary gland proteins from Ixodes persulcatus Schulze tick. Insect Mol Biol. (2014) 23:466–74. doi: 10.1111/imb.12101
77. Bhusal RP, Eaton JRO, Chowdhury ST, Power CA, Proudfoot AEI, Stone MJ, et al. Evasins: tick salivary proteins that inhibit mammalian chemokines. Trends Biochem Sci. (2020) 45:108–22. doi: 10.1016/j.tibs.2019.10.003
78. Frauenschuh A, Power CA, Déruaz M, Ferreira BR, Silva JS, Teixeira MM, et al. Molecular cloning and characterization of a highly selective chemokine-binding protein from the tick Rhipicephalus sanguineus. J Biol Chem. (2007) 282:27250–8. doi: 10.1074/jbc.M704706200
79. Russo RC, Alessandri AL, Garcia CC, Cordeiro BF, Pinho V, Cassali GD, et al. Therapeutic effects of evasin-1, a chemokine binding protein, in bleomycin-induced pulmonary fibrosis. Am J Respir Cell Mol Biol. (2011) 45:72–80. doi: 10.1165/rcmb.2009-0406OC
80. Castor MGM, Rezende B, Resende CB, Alessandri AL, Fagundes CT, Sousa LP, et al. The CCL3/Macrophage inflammatory protein-1a-binding protein evasin-1 protects from graft-versus-host disease but does not modify graft-versus-leukemia in mice. J Immunol. (2010) 184:2646–54. doi: 10.4049/jimmunol.0902614
81. Déruaz M, Frauenschuh A, Alessandri AL, Dias JM, Coelho FM, Russo RC, et al. Ticks produce highly selective chemokine binding proteins with antiinflammatory activity. J Exp Med. (2008) 205:2019–31. doi: 10.1084/jem.20072689
82. Denisov SS, Ippel JH, Heinzmann ACA, Koenen RR, Ortega-Gomez A, Soehnlein O, et al. Tick saliva protein Evasin-3 modulates chemotaxis by disrupting CXCL8 interactions with glycosaminoglycans and CXCR2. J Biol Chem. (2019) 294:12370–9. doi: 10.1074/jbc.RA119.008902
83. Montecucco F, Lenglet S, Braunersreuther V, Pelli G, Pellieux C, Montessuit C, et al. Single administration of the CXC chemokine-binding protein evasin-3 during ischemia prevents myocardial reperfusion injury in mice. Arterioscler Thromb Vasc Biol. (2010) 30:1371–7. doi: 10.1161/ATVBAHA.110.206011
84. Copin JC, Da Silva RF, Fraga-Silva RA, Capettini L, Quintao S, Lenglet S, et al. Treatment with Evasin-3 reduces atherosclerotic vulnerability for ischemic stroke, but not brain injury in mice. J Cereb Blood Flow Metab. (2013) 33:490–8. doi: 10.1038/jcbfm.2012.198
85. Montecucco F, Mach F, Lenglet S, Vonlaufen A, Gomes Quinderé AL, Pelli G, et al. Treatment with Evasin-3 abrogates neutrophil-mediated inflammation in mouse acute pancreatitis. Eur J Clin Investig. (2014) 44:940–50. doi: 10.1111/eci.12327
86. Braunersreuther V, Montecucco F, Pelli G, Galan K, Proudfoot AE, Belin A, et al. Treatment with the CC chemokine-binding protein Evasin-4 improves post-infarction myocardial injury and survival in mice. Thromb Haemost. (2013) 110:807–25. doi: 10.1160/TH13-04-0297
87. Darlot B, Eaton JRO, Geis-Asteggiante L, Yakala GK, Karuppanan K, Davies G, et al. Engineered anti-inflammatory peptides inspired by mapping an evasin-chemokine interaction. J Biol Chem. (2020) 295:10926–39. doi: 10.1074/jbc.ra120.014103
88. Wu J, Wang Y, Liu H, Yang H, Ma D, Li J, et al. Two immunoregulatory peptides with antioxidant activity from tick salivary glands. J Biol Chem. (2010) 285:16606–13. doi: 10.1074/jbc.M109.094615
89. Tian Y, Chen W, Mo G, Chen R, Fang M, Yedid G, et al. An immunosuppressant peptide from the hard tick Amblyomma variegatum. Toxins. (2016) 8:133. doi: 10.3390/toxins8050133
90. Wang F, Gong H, Zhang H, Zhou Y, Cao J, Zhou J. Lipopolysaccharide-induced differential expression of mirnas in male and female Rhipicephalus haemaphysaloides ticks. PLoS One. (2015) 10:e0139241. doi: 10.1371/journal.pone.0139241
91. Kim TK, Tirloni L, Berger M, Diedrich JK, Yates JR, Termignoni C, et al. Amblyomma americanum serpin 41 (AAS41) inhibits inflammation by targeting chymase and chymotrypsin. Int J Biol Macromol. (2020) 156:1007–21. doi: 10.1016/j.ijbiomac.2020.04.088
92. Tirloni L, Kim TK, Berger M, Termignoni C, Da Silva Vaz I, Mulenga A. Amblyomma americanum serpin 27 (AAS27) is a tick salivary anti-inflammatory protein secreted into the host during feeding. PLoS Negl Trop Dis. (2019) 13:e0007660. doi: 10.1371/journal.pntd.0007660
93. Chmelar J, Oliveira CJ, Rezacova P, Francischetti IMB, Kovarova Z, Pejler G, et al. A tick salivary protein targets cathepsin G and chymase and inhibits host inflammation and platelet aggregation. Blood. (2011) 117:736–44. doi: 10.1182/blood-2010-06-293241
94. Páleníková J, Lieskovská J, Langhansová H, Kotsyfakis M, Chmelaø J, Kopeck? J. Ixodes ricinus salivary serpin IRS-2 affects Th17 differentiation via inhibition of the interleukin-6/STAT-3 signaling pathway. Infect Immun. (2015) 83:1949–56. doi: 10.1128/IAI.03065-14
95. Sun T, Wang F, Pan W, Wu Q, Wang J, Dai J. An immunosuppressive tick salivary gland protein DsCystatin interferes with toll-like receptor signaling by downregulating TRAF6. Front Immunol. (2018) 9:1245. doi: 10.3389/fimmu.2018.01245
96. Dickinson RG, O’Hagan JE, Schotz M, Binnington KC, Hegarty MP. Prostaglandin in the saliva of the cattle tick Boophilus microplus. Aust J Exp Biol Med Sci. (1976) 54:475–86. doi: 10.1038/icb.1976.48
97. Ruan D, So S-P. Prostaglandin E2 produced by inducible COX-2 and mPGES-1 promoting cancer cell proliferation in vitro and in vivo. Life Sci. (2014) 116:43–50. doi: 10.1016/j.lfs.2014.07.042
98. Fezza F, Dillwith JW, Bisogno T, Tucker JS, Di Marzo V, Sauer JR. Endocannabinoids and related fatty acid amides, and their regulation, in the salivary glands of the lone star tick. Biochim Biophys Acta. (2003) 1633:61–7. doi: 10.1016/s1388-1981(03)00087-8
99. Oliveira CJF, Sá-Nunes A, Francischetti IMB, Carregaro V, Anatriello E, Silva JS, et al. Deconstructing tick saliva: non-protein molecules with potent immunomodulatory properties. J Biol Chem. (2011) 286:10960–9. doi: 10.1074/jbc.M110.205047
100. Della Latta V, Cabiati M, Rocchiccioli S, Del Ry S, Morales MA. The role of the adenosinergic system in lung fibrosis. Pharmacol Res. (2013) 76:182–9. doi: 10.1016/j.phrs.2013.08.004
101. Jarvis MF. Therapeutic potential of adenosine kinase inhibition–revisited. Pharmacol Res Perspect. (2019) 7:506. doi: 10.1002/prp2.506
102. Cai Y, Feng L, Wang X. Targeting the tumor promoting effects of adenosine in chronic lymphocytic leukemia. Crit Rev Oncol Hematol. (2018) 126:24–31. doi: 10.1016/j.critrevonc.2018.03.022
103. Honda T, Matsuoka T, Ueta M, Kabashima K, Miyachi Y, Narumiya S. Prostaglandin E2-EP3 signaling suppresses skin inflammation in murine contact hypersensitivity. J Allergy Clin Immunol. (2009) 124:809–27. doi: 10.1016/j.jaci.2009.04.029
104. Nakanishi M, Rosenberg DW. Multifaceted roles of PGE2 in inflammation and cancer. Semin Immunopathol. (2013) 35:123–37. doi: 10.1007/s00281-012-0342-8
105. Łukawska E, Polcyn-Adamczak M, Niemir ZI. The role of the alternative pathway of complement activation in glomerular diseases. Clin Exp Med. (2018) 18:297–318. doi: 10.1007/s10238-018-0491-8
106. Thorgersen EB, Barratt-Due A, Haugaa H, Harboe M, Pischke SE, Nilsson PH, et al. The role of complement in liver injury, regeneration, and transplantation. Hepatology. (2019) 70:725–36. doi: 10.1002/hep.30508
107. Tichaczek-Goska D. Deficiencies and excessive human complement system activation in disorders of multifarious etiology. Adv Clin Exp Med. (2012) 21:105–14.
108. Ricklin D, Reis ES, Lambris JD. Complement in disease: a defence system turning offensive. Nat Rev Nephrol. (2016) 12:383–401. doi: 10.1038/nrneph.2016.70
109. Ricklin D, Mastellos DC, Reis ES, Lambris JD. The renaissance of complement therapeutics. Nat Rev Nephrol. (2017) 14:26–47. doi: 10.1038/nrneph.2017.156
110. Valenzuela JG, Charlab R, Mather TN, Ribeiro JMC. Purification, cloning, and expression of a novel salivary anticomplement protein from the tick Ixodes scapularis. J Biol Chem. (2000) 275:18717–23. doi: 10.1074/jbc.M001486200
111. Schroeder H, Daix V, Gillet L, Renauld JC, Vanderplasschen A. The paralogous salivary anti-complement proteins IRAC I and IRAC II encoded by Ixodes ricinus ticks have broad and complementary inhibitory activities against the complement of different host species. Microb Infect. (2007) 9:247–50. doi: 10.1016/j.micinf.2006.10.020
112. Zwarthoff SA, Berends ETM, Mol S, Ruyken M, Aerts PC, Józsi M, et al. Functional characterization of alternative and classical pathway C3/C5 convertase activity and inhibition using purified models. Front Immunol. (2018) 9:1691. doi: 10.3389/fimmu.2018.01691
113. Hourcade DE, Akk AM, Mitchell LM, Zhou HF, Hauhart R, Pham CTN. Anti-complement activity of the Ixodes scapularis salivary protein Salp20. Mol Immunol. (2016) 69:62–9. doi: 10.1016/j.molimm.2015.11.008
114. Couvreur B, Beaufays J, Charon C, Lahaye K, Gensale F, Denis V, et al. Variability and action mechanism of a family of anticomplement proteins in Ixodes ricinus. PLoS One. (2008) 3:e1400. doi: 10.1371/journal.pone.0001400
115. Barratt-Due A, Thorgersen EB, Lindstad JK, Pharo A, Lissina O, Lambris JD, et al. Ornithodoros moubata complement inhibitor is an equally effective C5 Inhibitor in pigs and humans. J Immunol. (2011) 187:4913–9. doi: 10.4049/jimmunol.1101000
116. Nunn MA, Sharma A, Paesen GC, Adamson S, Lissina O, Willis AC, et al. Complement inhibitor of C5 activation from the soft tick Ornithodoros moubata. J Immunol. (2005) 174:2084–91. doi: 10.4049/jimmunol.174.4.2084
117. Mans BJ, Ribeiro JMC. Function, mechanism and evolution of the moubatin-clade of soft tick lipocalins. Insect Biochem Mol Biol. (2008) 38:841–52. doi: 10.1016/j.ibmb.2008.06.007
118. Hepburn NJ, Williams AS, Nunn MA, Chamberlain-Banoub JC, Hamer J, Morgan BP, et al. In vivo characterization and therapeutic efficacy of a C5-specific inhibitor from the soft tick Ornithodoros moubata. J Biol Chem. (2007) 282:8292–9. doi: 10.1074/jbc.M609858200
119. Kuhn N, Schmidt CQ, Schlapschy M, Skerra A. PASylated coversin, a C5-specific complement inhibitor with extended pharmacokinetics, shows enhanced anti-hemolytic activity in vitro. Bioconjug Chem. (2016) 27:2359–71. doi: 10.1021/acs.bioconjchem.6b00369
120. Barratt-Due A, Thorgersen EB, Egge K, Pischke S, Sokolov A, Hellerud BC, et al. Combined inhibition of complement (C5) and CD14 markedly attenuates inflammation, thrombogenicity, and hemodynamic changes in porcine sepsis. J Immunol. (2013) 191:819–27. doi: 10.4049/jimmunol.1201909
121. Jore MM, Johnson S, Sheppard D, Barber NM, Li YI, Nunn MA, et al. Structural basis for therapeutic inhibition of complement C5. Nat Struct Mol Biol. (2016) 23:378–86. doi: 10.1038/nsmb.3196
122. Reichhardt MP, Johnson S, Tang T, Morgan T, Tebeka N, Popitsch N, et al. An inhibitor of complement C5 provides structural insights into activation. Proc Natl Acad Sci USA. (2020) 117:362–70. doi: 10.1073/pnas.1909973116
123. Yuan X, Gavriilaki E, Thanassi JA, Yang G, Baines AC, Podos SD, et al. Small-molecule factor D inhibitors selectively block the alternative pathway of complement in paroxysmal nocturnal hemoglobinuria and atypical hemolytic uremic syndrome. Haematologica. (2017) 102:466–75. doi: 10.3324/haematol.2016.153312
124. Rother RP, Rollins SA, Mojcik CF, Brodsky RA, Bell L. Discovery and development of the complement inhibitor eculizumab for the treatment of paroxysmal nocturnal hemoglobinuria. Nat Biotechnol. (2007) 25:1256–64. doi: 10.1038/nbt1344
125. Schuijt TJ, Coumou J, Narasimhan S, Dai J, Deponte K, Wouters D, et al. A tick mannose-binding lectin inhibitor interferes with the vertebrate complement cascade to enhance transmission of the lyme disease agent. Cell Host Microbe. (2011) 10:136–46. doi: 10.1016/j.chom.2011.06.010
126. Dommett RM, Klein N, Turner MW. Mannose-binding lectin in innate immunity: past, present and future. Tissue Antigens. (2006) 68:193–209. doi: 10.1111/j.1399-0039.2006.00649.x
127. Wagemakers A, Coumou J, Schuijt TJ, Oei A, Nijhof AM, van ’t Veer C, et al. An Ixodes ricinus tick salivary lectin pathway inhibitor protects borrelia burgdorferi sensu lato from human complement. Vector Borne Zoonotic Dis. (2016) 16:223–8. doi: 10.1089/vbz.2015.1901
128. Coumou J, Wagemakers A, Narasimhan S, Schuijt TJ, Ersoz JI, Oei A, et al. The role of mannose binding lectin in the immune response against Borrelia burgdorferi sensu lato. Sci Rep. (2019) 9:1431. doi: 10.1038/s41598-018-37922-8
129. Kaźmierczak-Siedlecka K, Dvor̆ák A, Folwarski M, Daca A, Przewłócka K, Makarewicz W. Fungal gut microbiota dysbiosis and its role in colorectal, oral, and pancreatic carcinogenesis. Cancers. (2020) 12:1326. doi: 10.3390/cancers12051326
130. Sá-Nunes A, Bafica A, Lucas DA, Conrads TP, Veenstra TD, Andersen JF, et al. Prostaglandin E 2 is a major inhibitor of dendritic cell maturation and function in Ixodes scapularis Saliva. J Immunol. (2007) 179:1497–505. doi: 10.4049/jimmunol.179.3.1497
131. Esteves E, Bizzarro B, Costa FB, Ramírez-Hernández A, Peti APF, Cataneo AHD, et al. Amblyomma sculptum salivary PGE 2 modulates the dendritic cell Rickettsia rickettsii interactions in vitro and in vivo. Front Immunol. (2019) 10:118. doi: 10.3389/fimmu.2019.00118
132. Xu Z, Lin Z, Wei N, Di Q, Cao J, Zhou Y, et al. Immunomodulatory effects of Rhipicephalus haemaphysaloides serpin RHS2 on host immune responses. Parasit Vectors. (2019) 12:341. doi: 10.1186/s13071-019-3607-4
133. Preston SG, Majtán J, Kouremenou C, Rysnik O, Burger LF, Cabezas Cruz A, et al. Novel immunomodulators from hard ticks selectively reprogramme human dendritic cell responses. PLoS Pathog. (2013) 9:e1003450. doi: 10.1371/journal.ppat.1003450
134. Hannier S, Liversidge J, Sternberg JM, Bowman AS. Ixodes ricinus tick salivary gland extract inhibits IL-10 secretion and CD69 expression by mitogen-stimulated murine splenocytes and induces hyporesponsiveness in B lymphocytes. Parasit Immunol. (2003) 25:27–37. doi: 10.1046/j.1365-3024.2003.00605.x
135. Hannier S, Liversidge J, Sternberg JM, Bowman AS. Characterization of the B-cell inhibitory protein factor in Ixodes ricinus tick saliva: a potential role in enhanced Borrelia burgdoferi transmission. Immunology. (2004) 113:401–8. doi: 10.1111/j.1365-2567.2004.01975.x
136. Yu D, Liang J, Yu H, Wu H, Xu C, Liu J, et al. A tick B-cell inhibitory protein from salivary glands of the hard tick, Hyalomma asiaticum asiaticum. Biochem Biophys Res Commun. (2006) 343:585–90. doi: 10.1016/j.bbrc.2006.02.188
137. Dk B, Mj P, Mj C, Jd R, Sk W. Isolation and molecular cloning of a secreted immunosuppressant protein from Dermacentor andersoni salivary gland. J Parasitol. (2000) 86:516–25.
138. Alarcon-Chaidez FJ, Müller-Doblies UU, Wikel S. Characterization of a recombinant immunomodulatory protein from the salivary glands of Dermacentor andersoni. Parasit Immunol. (2003) 25:69–77. doi: 10.1046/j.1365-3024.2003.00609.x
139. Konnai S, Nakajima C, Imamura S, Yamada S, Nishikado H, Kodama M, et al. Suppression of cell proliferation and cytokine expression by HL-p36, a tick salivary gland-derived protein of Haemaphysalis longicornis. Immunology. (2009) 126:209–19. doi: 10.1111/j.1365-2567.2008.02890.x
140. Wang F, Lu X, Guo F, Gong H, Zhang H, Zhou Y, et al. The immunomodulatory protein RH36 is relating to blood-feeding success and oviposition in hard ticks. Vet Parasitol. (2017) 240:49–59. doi: 10.1016/j.vetpar.2017.03.017
141. Kotsyfakis M, Horka H, Salat J, Andersen JF. The crystal structures of two salivary cystatins from the tick Ixodes scapularis and the effect of these inhibitors on the establishment of Borrelia burgdorferi infection in a murine model. Mol Microbiol. (2010) 77:456–70. doi: 10.1111/j.1365-2958.2010.07220.x
142. Kotsyfakis M, Sá-Nunes A, Francischetti IMB, Mather TN, Andersen JF, Ribeiro JMC. Antiinflammatory and immunosuppressive activity of sialostatin L, a salivary cystatin from the tick Ixodes scapularis. J Biol Chem. (2006) 281:26298–307. doi: 10.1074/jbc.M513010200
143. Horka H, Staudt V, Klein M, Taube C, Reuter S, Dehzad N, et al. The tick salivary protein Sialostatin L Inhibits the Th9-derived production of the asthma-promoting cytokine il-9 and is effective in the prevention of experimental asthma. J Immunol. (2012) 188:2669–76. doi: 10.4049/jimmunol.1100529
144. Sá-Nunes A, Bafica A, Antonelli LR, Choi EY, Francischetti IMB, Andersen JF, et al. The immunomodulatory action of Sialostatin L on dendritic cells reveals its potential to interfere with autoimmunity. J Immunol. (2009) 182:7422–9. doi: 10.4049/jimmunol.0900075
145. Lieskovská J, Páleníková J, Širmarová J, Elsterová J, Kotsyfakis M, Campos Chagas A, et al. Tick salivary cystatin sialostatin L2 suppresses IFN responses in mouse dendritic cells. Parasit Immunol. (2015) 37:70–8. doi: 10.1111/pim.12162
146. Chen G, Wang X, Severo MS, Sakhon OS, Sohail M, Brown LJ, et al. The tick salivary protein sialostatin L2 inhibits caspase-1-mediated inflammation during Anaplasma phagocytophilum infection. Infect Immun. (2014) 82:2553–64. doi: 10.1128/IAI.01679-14
147. Kotál J, Stergiou N, Buša M, Chlastáková A, Beránková Z, R̆ezáčová P, et al. The structure and function of Iristatin, a novel immunosuppressive tick salivary cystatin. Cell Mol Life Sci. (2019) 76:2003–13. doi: 10.1007/s00018-019-03034-3
148. Leboulle G, Crippa M, Decrem Y, Mejri N, Brossard M, Bollen A, et al. Characterization of a novel salivary immunosuppressive protein from Ixodes ricinus ticks. J Biol Chem. (2002) 277:10083–9. doi: 10.1074/jbc.M111391200
149. Toyomane K, Konnai S, Niwa A, Githaka N, Isezaki M, Yamada S, et al. Identification and the preliminary in vitro characterization of IRIS homologue from salivary glands of Ixodes persulcatus schulze. Ticks Tick Borne Dis. (2016) 7:119–25. doi: 10.1016/j.ttbdis.2015.09.006
150. Prevot PP, Beschin A, Lins L, Beaufays J, Grosjean A, Bruys L, et al. Exosites mediate the anti-inflammatory effects of a multifunctional serpin from the saliva of the tick Ixodes ricinus. FEBS J. (2009) 276:3235–46. doi: 10.1111/j.1742-4658.2009.07038.x
151. Blisnick AA, Šimo L, Grillon C, Fasani F, Brûlé S, Le Bonniec B, et al. The immunomodulatory effect of IrSPI, a tick salivary gland serine protease inhibitor involved in Ixodes ricinus tick feeding. Vaccines. (2019) 7:148. doi: 10.3390/vaccines7040148
152. Garg R, Juncadella IJ, Ramamoorthi N, Ashish, Ananthanarayanan SK, Thomas V, et al. Cutting edge: CD4 is the receptor for the tick saliva immunosuppressor, Salp15. J Immunol. (2006) 177:6579–83. doi: 10.4049/jimmunol.177.10.6579
153. Marchal C, Schramm F, Kern A, Luft BJ, Yang X, Schuijt TJ, et al. Antialarmin effect of tick saliva during the transmission of Lyme disease. Infect Immun. (2011) 79:774–85. doi: 10.1128/IAI.00482-10
154. Paveglio SA, Allard J, Mayette J, Whittaker LA, Juncadella I, Anguita J, et al. The tick salivary protein, Salp15, inhibits the development of experimental asthma. J Immunol. (2007) 178:7064–71. doi: 10.4049/jimmunol.178.11.7064
155. Juncadella IJ, Garg R, Bates TC, Olivera ER, Anguita J. The Ixodes scapularis salivary protein, salp15, prevents the association of HIV-1 gp120 and CD4. Biochem Biophys Res Commun. (2008) 367:41–6. doi: 10.1016/j.bbrc.2007.12.104
156. Liu Q, Acharya P, Dolan MA, Zhang P, Guzzo C, Lu J, et al. Quaternary contact in the initial interaction of CD4 with the HIV-1 envelope trimer. Nat Struct Mol Biol. (2017) 24:370–8. doi: 10.1038/nsmb.3382
157. Juncadella IJ, Bates TC, Suleiman R, Monteagudo-Mera A, Olson CM, Navasa N, et al. The tick saliva immunosuppressor, Salp15, contributes to Th17-induced pathology during experimental autoimmune encephalomyelitis. Biochem Biophys Res Commun. (2010) 402:105–9. doi: 10.1016/j.bbrc.2010.09.125
158. Robinson AP, Harp CT, Noronha A, Miller SD. The experimental autoimmune encephalomyelitis (EAE) model of MS. utility for understanding disease pathophysiology and treatment. Handb Clin Neurol. (2014) 122:173–89. doi: 10.1016/B978-0-444-52001-2.00008-X
159. Tomás-Cortázar J, Martín-Ruiz I, Barriales D, Pascual-Itoiz MÁ, de Juan VG, Caro-Maldonado A, et al. The immunosuppressive effect of the tick protein, Salp15, is long-lasting and persists in a murine model of hematopoietic transplant. Sci Rep. (2017) 7:10740. doi: 10.1038/s41598-017-11354-2
160. Chmelar̆ J, Kotál J, Karim S, Kopacek P, Francischetti IMB, Pedra JHF, et al. Sialomes and mialomes: a systems-biology view of tick tissues and tick-host interactions. Trends Parasitol. (2016) 32:242–54. doi: 10.1016/j.pt.2015.10.002
161. Lefebvre FA, Lécuyer E. Small luggage for a long journey: transfer of vesicle-enclosed small RNA in interspecies communication. Front Microbiol. (2017) 8:377. doi: 10.3389/fmicb.2017.00377
162. Knip M, Constantin ME, Thordal-Christensen H. Trans-kingdom cross-talk: small RNAs on the move. PLoS Genet. (2014) 10:e1004602. doi: 10.1371/journal.pgen.1004602
163. Weiberg A, Bellinger M, Jin H. Conversations between kingdoms: small RNAs. Curr Opin Biotechnol. (2015) 32:207–15. doi: 10.1016/j.copbio.2014.12.025
164. Barrero RA, Keeble-Gagnère G, Zhang B, Moolhuijzen P, Ikeo K, Tateno Y, et al. Evolutionary conserved microRNAs are ubiquitously expressed compared to tick-specific miRNAs in the cattle tick Rhipicephalus (Boophilus) microplus. BMC Genomics. (2011) 12:328. doi: 10.1186/1471-2164-12-328
165. Bensaoud C, Hackenberg M, Kotsyfakis M. Noncoding RNAs in parasite–vector–host interactions. Trends Parasitol. (2019) 35:715–24. doi: 10.1016/j.pt.2019.06.012
166. Chávez ASO, O’Neal AJ, Santambrogio L, Kotsyfakis M, Pedra JHF. Message in a vesicle – trans-kingdom intercommunication at the vector-host interface. J Cell Sci. (2019) 132:jcs224212. doi: 10.1242/jcs.224212
167. Hackenberg M, Kotsyfakis M. Exosome-mediated pathogen transmission by arthropod vectors. Trends Parasitol. (2018) 34:549–52. doi: 10.1016/j.pt.2018.04.001
168. Yao R-W, Wang Y, Chen L-L. Cellular functions of long noncoding RNAs. Nat Cell Biol. (2019) 21:542–51. doi: 10.1038/s41556-019-0311-8
169. Quinn JJ, Chang HY. Unique features of long non-coding RNA biogenesis and function. Nat Rev Genet. (2016) 17:47–62. doi: 10.1038/nrg.2015.10
170. Gomes AQ, Nolasco S, Soares H. Non-coding RNAs: multi-tasking molecules in the cell. Int J Mol Sci. (2013) 14:16010–39. doi: 10.3390/ijms140816010
171. Hackenberg M, Langenberger D, Schwarz A, Erhart J, Kotsyfakis M. In silico target network analysis of de novo-discovered, tick saliva-specific microRNAs reveals important combinatorial effects in their interference with vertebrate host physiology. RNA. (2017) 23:1259–69. doi: 10.1261/rna.061168.117
Keywords: tick saliva, salivary glands, host immunity, immunomodulation, drug discovery
Citation: Aounallah H, Bensaoud C, M’ghirbi Y, Faria F, Chmelar̆ J and Kotsyfakis M (2020) Tick Salivary Compounds for Targeted Immunomodulatory Therapy. Front. Immunol. 11:583845. doi: 10.3389/fimmu.2020.583845
Received: 15 July 2020; Accepted: 02 September 2020;
Published: 23 September 2020.
Edited by:
Nathalie Boulanger, Université de Strasbourg, FranceReviewed by:
Maria Kazimirova, Institute of Zoology (SAS), SlovakiaSukanya Narasimhan, Yale University, United States
Copyright © 2020 Aounallah, Bensaoud, M’ghirbi, Faria, Chmelar̆ and Kotsyfakis. This is an open-access article distributed under the terms of the Creative Commons Attribution License (CC BY). The use, distribution or reproduction in other forums is permitted, provided the original author(s) and the copyright owner(s) are credited and that the original publication in this journal is cited, in accordance with accepted academic practice. No use, distribution or reproduction is permitted which does not comply with these terms.
*Correspondence: Michail Kotsyfakis, bWljaF9rb3RzeWZha2lzQHlhaG9vLmNvbQ==
†These authors have contributed equally to this work