- Division of Microbiology, Paul-Ehrlich-Institut, Langen, Germany
Despite continuous exposure and development of specific immunity, Staphylococcus aureus (Sa) remains one of the leading causes of severe infections worldwide. Although innate immune defense mechanisms are well understood, the role of the T cell response has not been fully elucidated. Here, we demonstrate that Sa and one of its major virulence factors protein A (SpA) induce human regulatory T cells (Tregs), key players in immune tolerance. In human PBMC and MoDC/T cell cocultures CD4+CD25+CD127dim Tregs were induced upon stimulation with Sa and to a lower extent with SpA alone. Treg induction was strongly, but not exclusively, dependent on SpA, and independent of antigen presentation or T cell epitope recognition. Lastly, soluble factors in the supernatant of SpA-stimulated MoDC were sufficient to trigger Treg formation, while supernatants of MoDC/T cell cocultures containing Sa-triggered Tregs displayed T cell suppressive activity. In summary, our findings identify a new immunosuppressory function of SpA, which leads to release of soluble, Treg-inducing factors and might be relevant to establish colonization.
Introduction
The human commensal Staphylococcus aureus (Sa) is a major pathogen and leading cause of nosocomial infections, resulting in tens of thousands of deaths worldwide and causing billions of dollar economical damage per year (1–3). About 30% of the human population are colonized (4). The increasing antibiotic resistance of Sa strains [so called MRSA, (5)], resulting in long-lasting infections, illustrates the urgent need for a protective vaccine. However, despite promising approaches during the last decades and successful in vivo studies, all vaccine attempts have failed to date (6–8). One of the many reasons is the lack of essential pieces in understanding the complex human immune response against this pathogen.
For many years, research focused on the humoral immune response against Sa since antibodies against this bacterium can be found in asymptomatically colonized individuals as well as in patients (9, 10). However recently, more and more research has been dedicated to T cell-mediated immunity, demonstrating that this arm of the immune system plays an important role in Sa clearance. Several models of infection in mice and in human have shown that CD4+ T cells are important for the immune response to Sa, as reviewed elsewhere in detail (6, 11, 12). Infections were more severe in ifnγr-deficient mice (13) and patients with Th1-deficiencies are more prone for infection (14). Lin at al. showed that protection in vaccinated mice was caused by secretion of IFNγ and IL-17 from CD4+ T cells (15). Several others also proved that Th17-mediated immunity is essential during Sa infection (16–19). Furthermore, we and others demonstrated that a human memory T cell repertoire exists in healthy individuals (20–22), underlining the importance of previous exposure.
One of the many reasons, Sa is such a potent immune activator is its vast variety of virulence factors, among them staphylococcal protein A (SpA). This cell-wall anchored protein (23) is expressed by almost all Sa isolates and highly abundant on the staphylococcal cell wall (24). It harbors 4-5 Ig-binding domains (25) and by binding the Fcγ domain of IgG (26) it can prevent opsonization (24) and FcR-mediated phagocytosis (27). Additionally, SpA binds the B cell receptor (28) of VH3+ B cells [30%–60% of B cells in human (29)]. This leads to B cell proliferation, apoptosis (30) and with the help of plasmacytoid dendritic cells, to formation of regulatory B cells (Bregs). These cells secrete IL-10, a cytokine associated with suppression of antigen presentation and T cell responses (31, 32).
Another important cell subset to prevent and control overshooting immune responses are regulatory T cells [Tregs, (33)]. Through secretion of immunosuppressive cytokines such as IL-10 and TGFβ, consumption of immunostimulatory cytokines, secretion of cytolytic factors and interaction with antigen-presenting cells (APC) they maintain immune homeostasis (34). Due to their strong immunosuppressive properties they are being evaluated for treatment of autoimmune diseases (35). CD4+ Tregs are described as Forkhead-Box-Protein P3+ (FoxP3), CD25+ [IL-2 receptor α chain), and CD127dim/- (IL-7 receptor, (36)].
Several groups demonstrated a potential role of Tregs in post-infectious arthritis following Sa infection (37–39) and in atopic dermatitis (40). Furthermore, the Sa virulence factor phenol-soluble modulin (PSMα) was shown to modulate human and mouse APC surface marker expression and cytokine secretion to induce Treg in vitro and in vivo (41–43). Moreover, it has been shown that staphylococcal superantigens induce human Tregs in PBMC (44) and convert peripheral CD4+CD25- T cells to a regulatory phenotype with suppressive function (45, 46).
In earlier studies, we saw that SpA induces Treg-associated cytokines in vitro (20). This study aimed to investigate the potential of this B cell superantigen in the induction of human Tregs.
Materials and Methods
Bacteria
Staphylococcus aureus WT strain SA113, spa-deficient mutant SA113 Δspa and SA113Δlgt lacking TLR2 activity (provided by Friedrich Götz, Tübingen) were grown on Columbia blood agar plates (supplemented with 20 µg/ml Erythromycin for mutant strains) overnight at 37°C.
Reagents, Stimuli, and Antibodies
Stimulation of cell culture was carried out with 1 μg/ml protein A (SpA, isolated from S. aureus SAC, GE Healthcare, Uppsala, Sweden), 1 µg/ml recombinant SpA (Sigma-Aldrich, Munich, Germany), 100 ng/ml synthetic lipopeptide P3C (Pam3CSK4, EMC, Tübingen, Germany) or anti-CD3/CD28 microbeads (Miltenyi Biotech, Bergisch-Gladbach, Germany).
Microbeads used for cell isolation via AutoMACS and recombinant cytokines (IL-4 and GM-CSF) were obtained from Miltenyi Biotech (Bergisch-Gladbach, Germany).
Antibodies used for flow cytometry, purity determination and cell sorting were purchased from BD Biosciences, Heidelberg, Germany, if not indicated otherwise: CD4 PerCP, CD4 PE, CD3 BV605 (BioLegend, U.S.), CD3 AF700 (BioLegend, U.S.), CD25 APC (BioLegend, U.S.), CD25 FITC, CD127 BV421, CD127 AF647, FoxP3 BV421 (BioLegend, U.S.), CCR4 PE-Cy7 (BioLegend, U.S.), ICOS BV605 (BioLegend, U.S.), CTLA4 BV421 (BioLegend, U.S.), PD-1 PE (BioLegend, U.S.) and CD14 V450. Viability staining was performed with the LIVE/DEAD Fixable dead Cell stain Kit (Thermo Fisher Scientific, U.K.).
Isolation of PBMC and T Cells
Use of human peripheral blood mononuclear cells (PBMC) from buffy coats was approved by the local institutional review board (Ethics committee of the Medical Faculty of the University of Frankfurt, Germany, #154/15). Buffy coats of anonymized healthy donors were obtained from the German Red Cross South transfusion center (Frankfurt am Main, Germany).
PBMC were isolated by Pancoll gradient centrifugation (PAN-Biotech, Aidenbach, Germany). T cells were isolated by positive selection with anti-CD4 microbeads via AutoMACS. Purity was determined by CD4 antibody and was always ≥97% (Figure S1).
Generation of MoDC and Cocultures With Autologous T Cells or PBMC
MoDC were generated by positive CD14+ isolation via AutoMACS and culture in medium containing 50 ng/ml human GM-CSF and 20 ng/ml human lL-4. Fresh medium supplemented with 100 ng/ml GM-CSF and 40 ng/ml IL-4 was added on day 3 and MoDC were cultured for 6d, as described earlier (20). Remaining PBMC were frozen in 50% FCS and 50% freezing medium (RPMI 1640, supplemented with 20% FCS and 20% DMSO (both from Sigma-Aldrich, Munich, Germany)) for subsequent isolation of autologous T cells.
If not stated otherwise, culture of PBMC, MoDC/PBMC cocultures or MoDC/T cell cocultures was carried out under Ig-free conditions in RPMI 1640 (Gibco by Life science, Darmstadt, Germany), supplemented with 5% Xerumfree (TNC Bio, Eindhoven, Netherlands) as serum-free alternative, 1% penicillin/streptomycin (10,000 IU/ml and 10,000 μg/ml) and 1% 200 mM L-Glutamine (all from Biochrom AG, Berlin, Germany). For some experiments, instead of Xerumfree, 5% heat-inactivated human serum (Biochrom AG, Berlin, Germany) or chicken serum (in house) was added. In this case, SpA was incubated with the indicated media for 2 h rolling before being added to the cells, to ensure blocking of SpA’s Ig-binding sites.
1*105 PBMC or 1*105 CD4+ T cells were seeded in 96 well plates alone or in coculture with 2*104 MoDC in 200 µl medium total. Cells were stimulated with bacteria at a ratio 1:10 (MOI=10, determined by McFarland 1) or 1 μg/ml SpA and incubated for 5d at 37°C and 5% CO2 for Treg induction or cytokine measurement.
For generation of ivt spa mRNA and MoDC transfection, see (20) for details.
For determination of MoDC-derived cytokines or generation of MoDC SN for T cell stimulation, MoDC only were seeded with a density of 1*105/96 well in 200 µl medium total and stimulated with the indicated stimuli for 24h at 37°C and 5% CO2. The plate was centrifuged, SN taken and used for cytokine determination by MagPix XMAP technology.
For analysis of Treg-inducing capacity, SN was re-centrifuged for 6 min at 1300 rpm and frozen for minimum 1 h at -20°C before being added to T cells, to avoid any contamination by remaining MoDC.
Flow Cytometry and Cell Sorting
Flow cytometry was performed at a FACS LSRII SORP (BD Biosciences, Heidelberg, Germany). Data was analyzed by Kaluza 2.1 Software (Beckman Coulter, U.S.).
For cell sorting, PBMC were isolated from buffy coats and stored overnight at 4°C in PBS, supplemented with 5% Xerum free. The next day, cells were washed, stained with CD4 PerCP, CD3 BV605, CD25 FITC, CD127 AF 647 and Tregs were depleted with a 70 μm nozzle with BD FACSAria Fusion (BD Biosciences, Heidelberg, Germany), using the BD FACS Diva software version 8.0.1. After sorting, cells were collected in tubes coated with 10% BSA overnight (to increase cell recovery rate) and containing RPMI with 5% Xerum free, 1% Pen/Strep, and 1% L-Glutamine. As control, full PBMC were also stained and sorted, but Tregs were not depleted (for sorting scheme see Figure S7A). Purity of sorted cells was confirmed by re-analysis and was >90% (Figure S7B).
After sorting, cells were stimulated with the indicated stimuli, incubated for 5d and re-stained with CD25 FITC, CD4 PerCP, and FoxP3 BV421, using the FoxP3 Fix/Perm Buffer Set (BioLegend, U.S.) and following the manufacturer’s protocol.
Suppression Assays
For generation of Treg-enriched SN, 6*105 CD4+ T cells were seeded in 24 well plates together with 1.2*105 MoDC in 250 µl medium each (RPMI with 5% human serum, 1% Pen/Strep, 1% L-Glutamine) and were stimulated with Sa WT MOI= 10. Culture in 24 well plates was carried out in a total volume of 1 ml.
To avoid remaining viable bacteria in the SN during subsequent stimulation, Sa was heat-inactivated by incubation at 60°C for 30 min (HISA). Heat-inactivation was confirmed by spreading bacterial suspension on Columbia blood agar plates and incubation at 37°C overnight. No bacterial growth was detected.
After 5d incubation, plates were centrifuged, induction of Tregs was confirmed by flow cytometry as described above and SN was collected, re-centrifuged and frozen once to lyse remaining cells.
Next, to determine suppressive capacity of Treg-enriched SN, autologous CD4+ T cells were isolated from frozen PBMC by AutoMACS as described above, stained with CFSE (Thermo Fisher Scientific, U.K.) and seeded in 100 µl medium (10% human serum, 2% Pen/Strep, 2% L-Glutamine) at a density of 5*105/ml. For cell stimulation, 0.5 µl anti-CD3/CD28 beads (≙100.000 beads; cell: bead ratio 1:1) and either 100 µl Treg-enriched SN (considered serum-free) or 100 µl RPMI pure were added to the autologous T cells. Cell proliferation was assessed after 4d by measurement of viable, CFSElow cells by flow cytometry.
Cytokine Measurements
Cytokines in supernatants from MoDC cultures or MoDC/T cell cocultures were quantified by the ELISA Multiplex MagPix XMAP technology (Luminex, 640 Austin, U.S.), detecting and quantifying multiple cytokines in one assay. The Milliplex Human Th17 Magnetic Bead Panel Kit (Merck Millipore, Darmstadt, Germany) was used to analyze cytokine levels in supernatants of MoDC stimulated for one day with SpA or ivt mRNA. T cell cytokines in MoDC/T cell cocultures stimulated with bacteria for 5d were quantified using the Bio-Plex Pro Human Cytokine 17-plex Assay (Bio-Rad Laboratories GmbH, Munich, Germany).
Statistical Analysis
Statistical analysis of results was carried out using GraphPad Prism 8.01 (Graphpad Software Inc. San Diego, USA). If not stated otherwise, following testing for normal distribution (Shapiro-Wilk-Test), paired two-tailed Student’s t-test was used, treating samples as paired data points. One-way ANOVA with LSD was used for testing multiple groups. Each experiment was carried out at least in duplicates in at least two independent experiments. Results were considered statistically significant at *p < 0.05, **p < 0.01, ***p < 0.001, and ****p < 0.0001. p ≥ 0.05 = not significant (ns).
Results
S. aureus Induces T Cell-Associated Cytokines in Cocultures With MoDC in a SpA-Dependent Manner
The immunostimulatory effect of SpA on B cells is well known. However, so far, there is only few data on this important virulence factor on the activation of T cells. Previously, we described the induction of IL-2, G-CSF, and IL-10 in cocultures of monocyte-derived dendritic cells (MoDC) and T cells upon SpA stimulation (20). These cytokines are associated with immunosuppression and/or Treg development.
To analyze the impact of SpA on this cytokine profile, we stimulated human MoDC/CD4+ T cell cocultures with viable Sa (SA113 WT) or a spa-deficient mutant (SA113 Δspa). After incubation for 5d, cytokine secretion was analyzed in the supernatants by MagPix, a multiplex ELISA system (Figure 1).
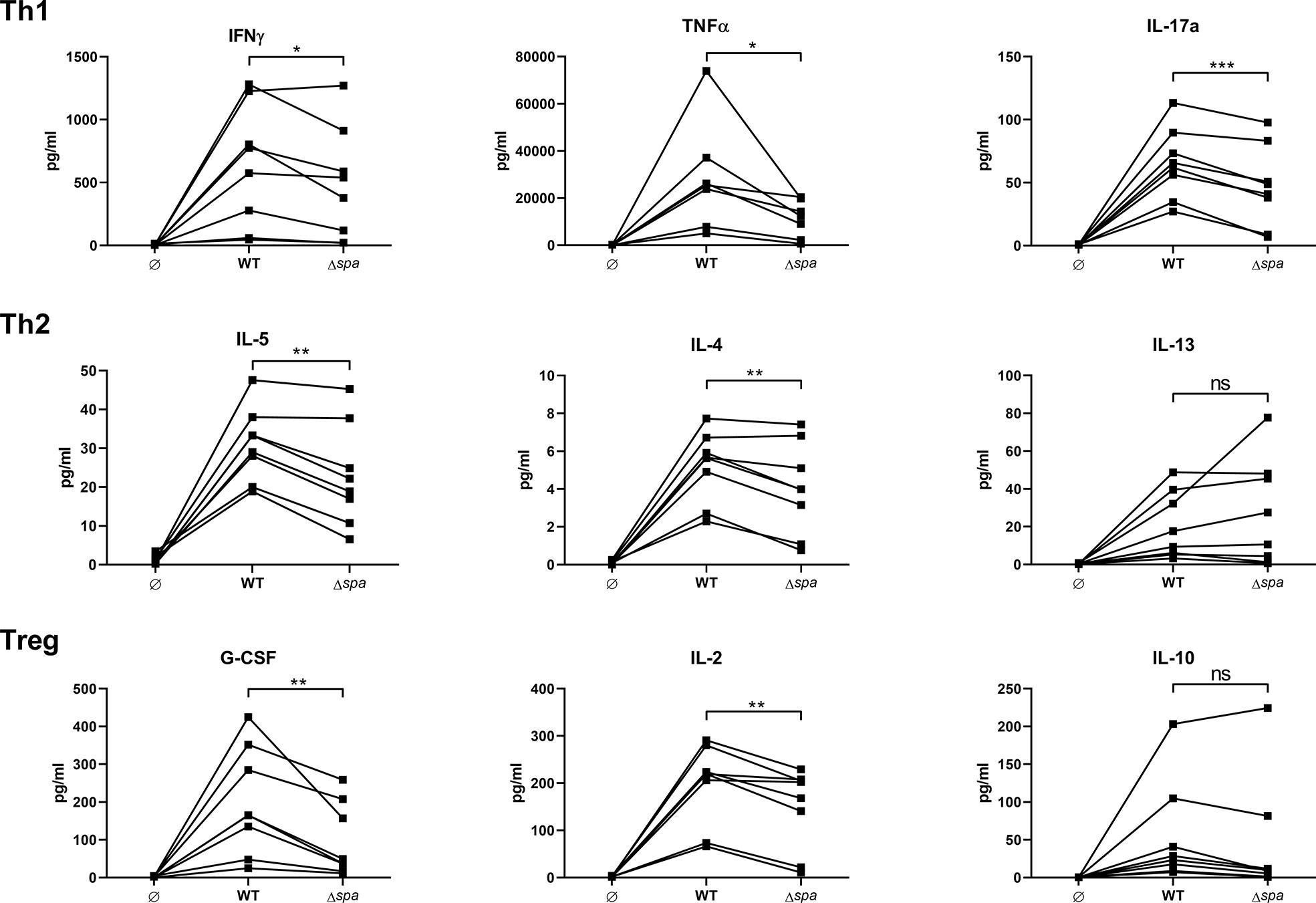
Figure 1 Staphylococcal protein A (SpA) induces T cell cytokine secretion. MoDC/CD4+ T cell cocultures were stimulated with SA113 WT or spa-deficient strain (Δspa). Th1 (upper row), Th2 (middle row), and Treg (lower row) associated cytokines were analyzed in 5d supernatant by Magpix, a multiplex ELISA technology. N = 8 different donors of four independent experiments are displayed as individual, linked points. * and ** indicate statistical significance: *p < 0.05, **p < 0.01.
Overall, the wild type strain led to higher secretion of almost all detected cytokines. There was a significant decrease in Th1/Th17 cytokines (IFNγ, TNF and IL-17) when cells were stimulated with the SA strain lacking SpA expression (Δspa). Albeit the Th2 response was generally less pronounced, Δspa induced significantly less IL-5 and IL-4. The Treg-associated and immunosuppressive cytokines G-CSF and IL-2 were also significantly decreased after Δspa stimulation. IL-10 levels were low, and even less in the absence of SpA expression. The same tendency was seen with IL-12p40, IL-1β and IL-6 (Figure S2). Thus, it was demonstrated that SpA is responsible for the strong immunostimulatory reactivity to Sa in MoDC/T cell cocultures.
S. aureus and SpA Induce Human Tregs
Next, we wanted to analyze whether SpA induces not only Treg-associated cytokines, but also Tregs. We therefore stimulated PBMC or MoDC/T cell cocultures with SA113 WT, SA113 Δspa or SpA protein only. Experiments were carried out in media lacking immunoglobulins to prevent blocking of the SpA Ig-binding sites. On day 5, we stained cells for CD3+CD4+CD25+CD127dim expression (Figure S3) and analyzed them by flow cytometry. Indeed, SpA induced a significant proportion of Tregs compared to the unstimulated control in PBMC (Figure 2A) and MoDC/T cell cocultures (Figures 2B, C). Interestingly, stimulation of PBMC with full bacteria induced an even higher percentage of Tregs, which was significantly reduced when bacteria lacked spa expression. Nevertheless, this proportion was still higher than induction of Tregs by SpA alone, indicating that additional immunostimulatory signals are important for induction of this cell type. This induction pattern was reproducible in MoDC/T cell cocultures (Figure 2B). Furthermore, we did not see differences in Treg levels when PBMC were stimulated with SA113 Δlgt, lacking TLR2-activating lipoproteins, compared to SA113 WT (Figure S4).
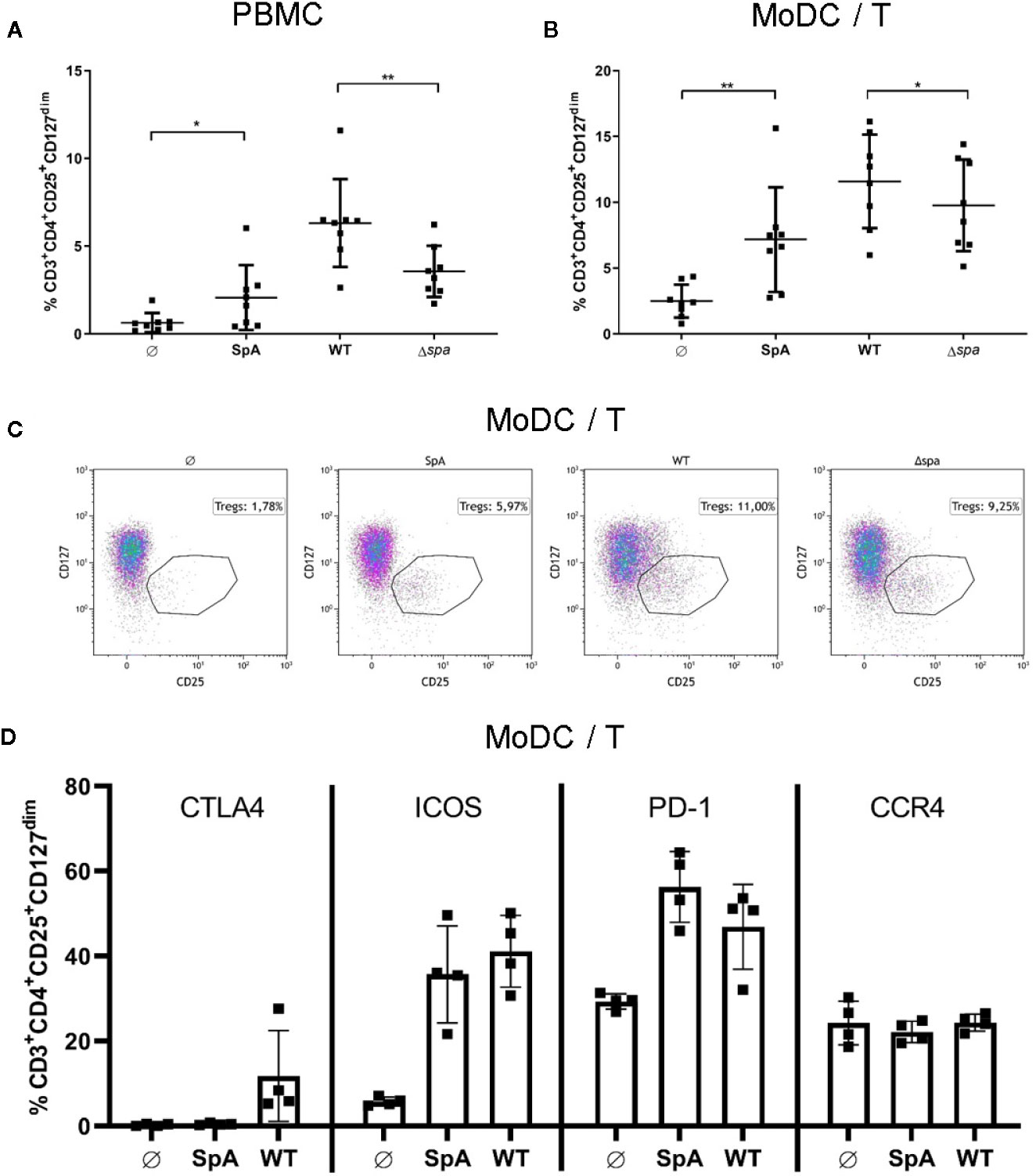
Figure 2 Staphylococcal protein A (SpA) is crucial for Treg induction. (A) peripheral blood mononuclear cell (PBMC) or (B) monocyte-derived dendritic cell (MoDC)/T cell cocultures were stimulated with SpA, SA113 WT or Δspa bacteria. After 5d cells, were stained for viability and CD3+CD4+CD25+CD127dim and were analyzed by flow cytometry. Results are shown from four independent experiments as single values of n=8 different donors with ± SD. Statististical significance is provided by * (*p < 0.05) and ** (**p < 0.01). (C) Induction of CD3+CD4+CD25+CD127dim Tregs in MoDC/T cell cocultures upon stimulation with SpA, SA113 WT or Δspa. Dot plots of one representative donor are shown. (D) CD3+CD4+CD25+CD127dim Tregs in MoDC/T cell cocultures upon stimulation with SpA or SA113 WT were analyzed for CTLA4, ICOS, PD-1 and CCR4 expression after 5d of culture by flow cytometry. Results are shown from two independent experiments as single values of n=4 different donors with ± SD.
To analyze the SpA and SA-induced Tregs in more detail, we stained the Treg with anti-CTLA4, anti-PD-1, anti-ICOS, and anti-CCR4 antibodies and quantified expression of these markers by flow cytometry (Figure 2D). The results showed that ICOS and PD-1 expression is induced upon formation of Tregs after stimulation with SpA protein or SA113 WT bacteria. Upregulation of CTLA4 was lower and only observed with bacterial stimulation. By contrast, CCR4 expression was not altered by SpA or SA113 WT stimulation. We conclude that SpA-dependent Treg induction promotes expression of ICOS and PD-1 but has little effect on CTLA4 and CCR4 expression.
The Induction of Tregs by SpA Is Independent on Antigen Presentation
Next, we asked whether SpA has to be presented by APC or whether it directly acts on T cells. To this end, we choose a technical approach where antigen is delivered as mRNA to the dendritic cells. The spa gene was in vitro transcribed (ivt) and APCs were transfected with ivt mRNA. Upon uptake by MoDC, ivt mRNA is translated, antigen processed and presented to T cells. As control of unspecific stimulation by ivt mRNA nonantigen encoding mRNA (NC mRNA), as well as only the transfection reagent lipofectamine (LF) were used. In comparison to native SpA, Treg induction by ivt mRNA in MoDC/T cell cocultures was assessed after 5d by flow cytometry.
As displayed in Figure 3, Tregs were only induced when antigen was delivered as protein, whereas Treg induction in conditions with ivt mRNA and LF remained at unstimulated levels. Results could be reproduced in cocultures of MoDC and PBMC (Figure S5). Please note, that although ivt mRNA did not induce Tregs, cytokine secretion could be measured in MoDC cultures upon transfection, confirming that cell stimulation itself by transfection was successful (Figure S6). This led to the conclusion, that the native protein conformation of SpA is necessary for Treg induction and that processing and presentation of the antigen is not sufficient.
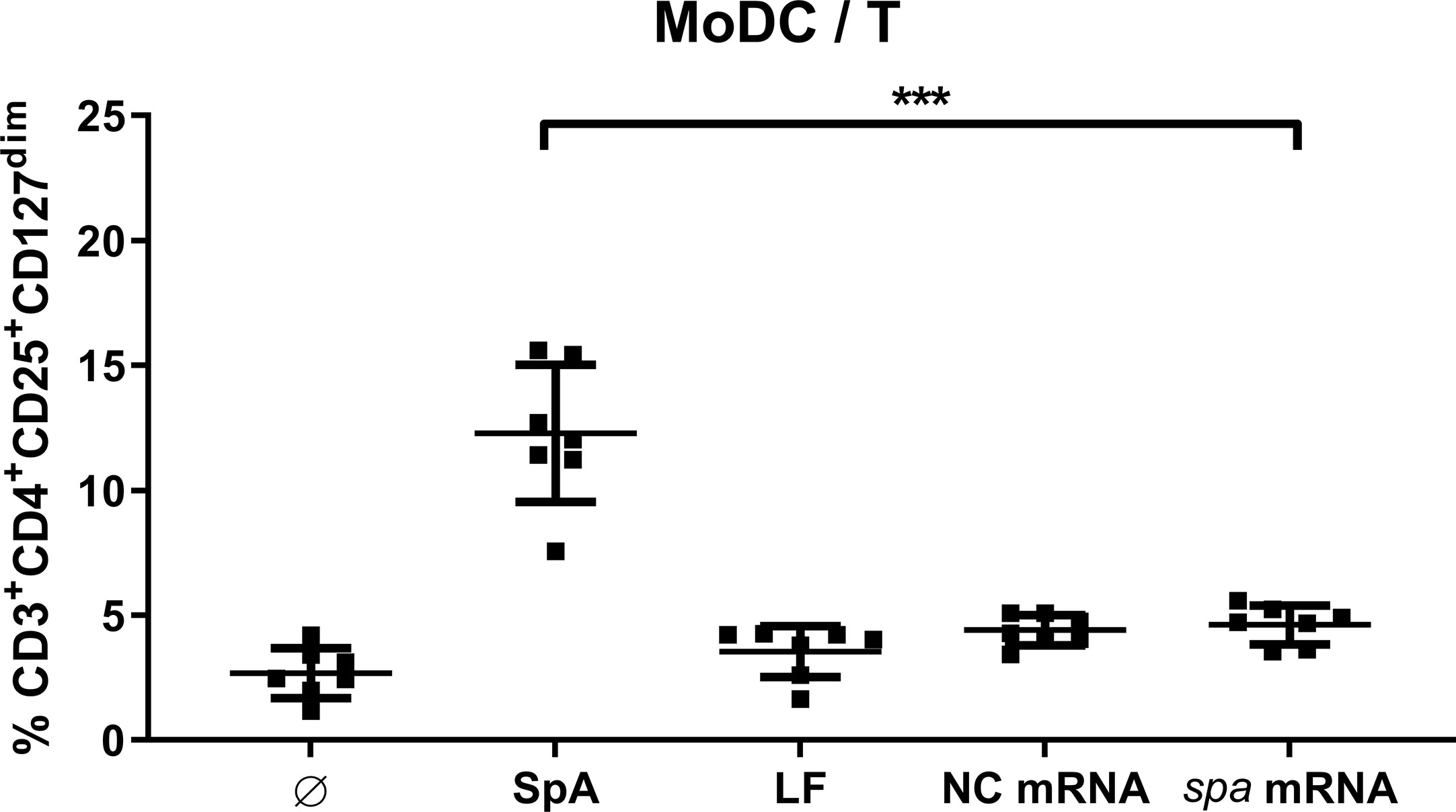
Figure 3 Native protein conformation of SpA is mandatory for Treg induction. Monocyte-derived dendritic cell (MoDC) were stimulated with staphylococcal protein A (SpA) or transfected with 100 ng mRNA (encoding spa or noncoding, NC) in cocultures with CD4+ T cells. As transfection control served lipofectamine (LF). After 5d, cells were stained for viability, CD3+CD4+CD25+CD127dim expression and were analyzed by flow cytometry. Results are shown from three independent experiments as single values of n = 7 different donors with ± SD ***p< 0.001.
SpA-Induced Treg Formation Is Blocked in the Presence of Human Immunoglobulins
Since native SpA’s most prominent characteristic is its Ig-binding capacity, we addressed this issue by adding different sera in the culture media to block the Ig-binding sites. We analyzed culture in medium supplemented with chicken serum or human serum. SpA does not bind chicken Ig (47), but human Ig with high affinity. All sera were heat-inactivated prior use and SpA was incubated 2 h with the media containing 5% of the indicated serum before being added to the cells.
As displayed in Figure 4, culture in media with chicken serum resulted in higher background of Tregs in the unstimulated control. Nevertheless, SpA induced significantly more Tregs in PBMC (Figure 4A) as well as in MoDC/T cell cocultures (Figure 4B). As seen before in Ig-free serum (Figures 2A, B), wild type bacteria led to a higher percentage of Tregs than Sa Δspa. However, this finding was only significant in PBMC cultures (Figure 4A). Induction of Tregs by SpA only was completely blocked compared to the unstimulated control in PBMC (Figure 4A) or MoDC/T cell cultures (Figure 4B) when human serum was supplemented in the culture media.
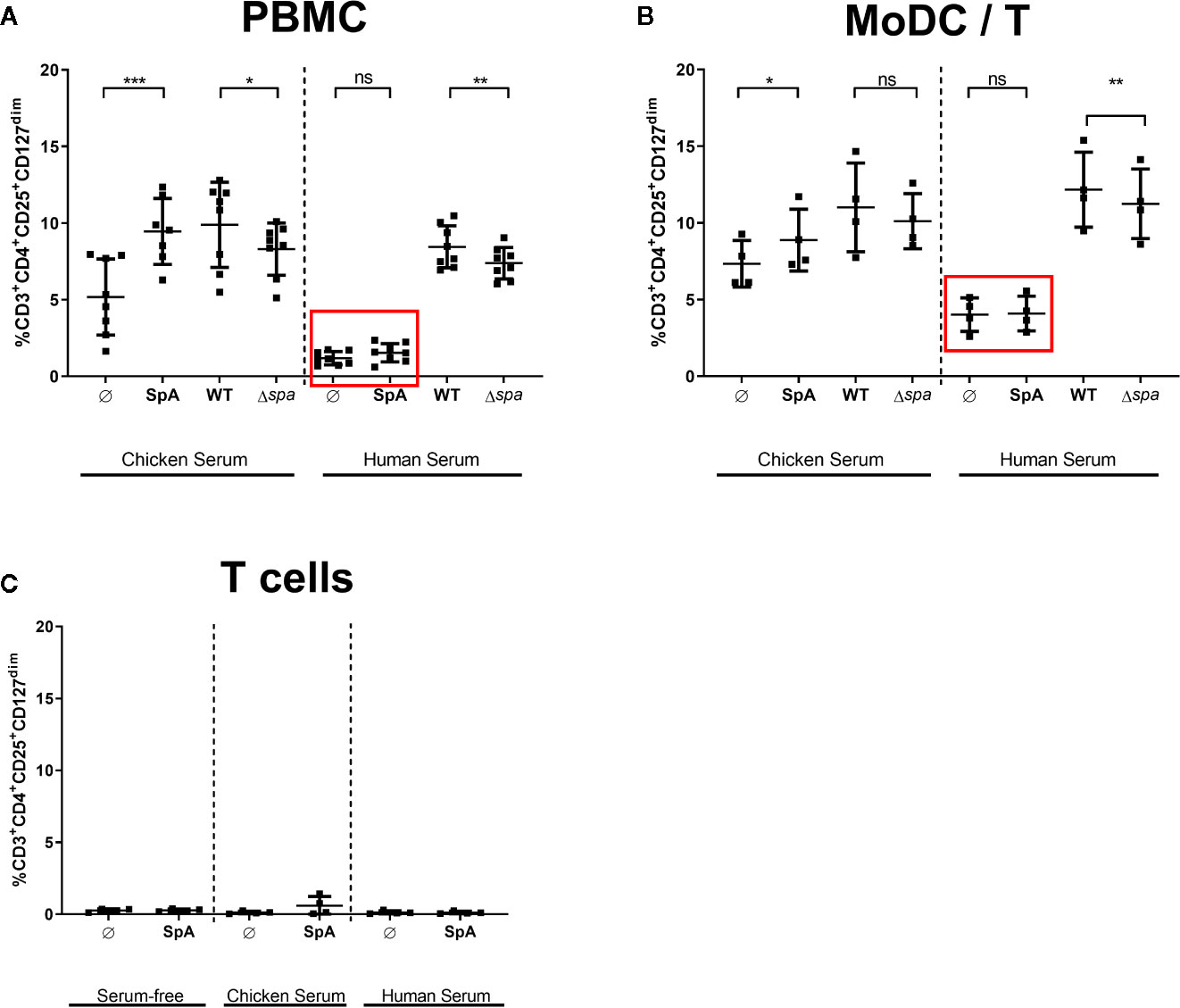
Figure 4 Treg induction by staphylococcal protein A (SpA) in blocked in human serum. (A) Peripheral blood mononuclear cells (PBMC), (B) monocyte-derived dendritic cell (MoDC)/T cell cocultures, or (C) CD4+ T cells only were stimulated with SpA, SA113 WT or Sa Δspa for 5d in media containing chicken, human or no serum supplement (serum-free). CD3+CD4+CD25+CD127dim cells were stained for viability and analyzed by flow cytometry. Results are shown from at least two independent experiments as single values of a minimum of four different donors with ± SD. The stars indicate statistical significance levels defined as *p < 0.05, **p < 0.01 and ***p < 0.001.
These results indicated that the Ig-binding sites of SpA protein might be crucial for Treg induction. Importantly, this effect was exclusively seen in the presence of APC, since no Treg induction was detected when protein was incubated with T cells only, independent on the medium (Figure 4C). By contrast, Treg induction by whole bacteria remained comparable in human serum, indicating that other bacterial factors contribute to activation of Tregs and that Treg induction by whole bacteria is not influenced by presence of immunoglobulins.
S. aureus Induces Tregs De Novo in Treg-Depleted PBMC
Next, we asked whether Sa can mediate de novo formation of Tregs or whether it induces proliferation of the existing Treg pool. To this end, we depleted Tregs from full PBMC via cell sorting, stimulated with SA113 WT or Δspa, respectively, and quantified CD25+FoxP3+ Tregs after 5 days (for sorting scheme, see Figure S7).
Interestingly, within 5d, the Treg population in PBMCΔTreg, left unstimulated as well as stimulated with SA113 WT was restored to approximate levels of full PBMC (Figure 5). However, SA113 Δspa did not induce CD25+FoxP3+ Tregs in full PBMC nor in PBMCΔTreg (Figure 5), and neither did SpA protein (data not shown). Nevertheless, the data clearly demonstrated that SA113 WT restored Treg numbers within 5 days.
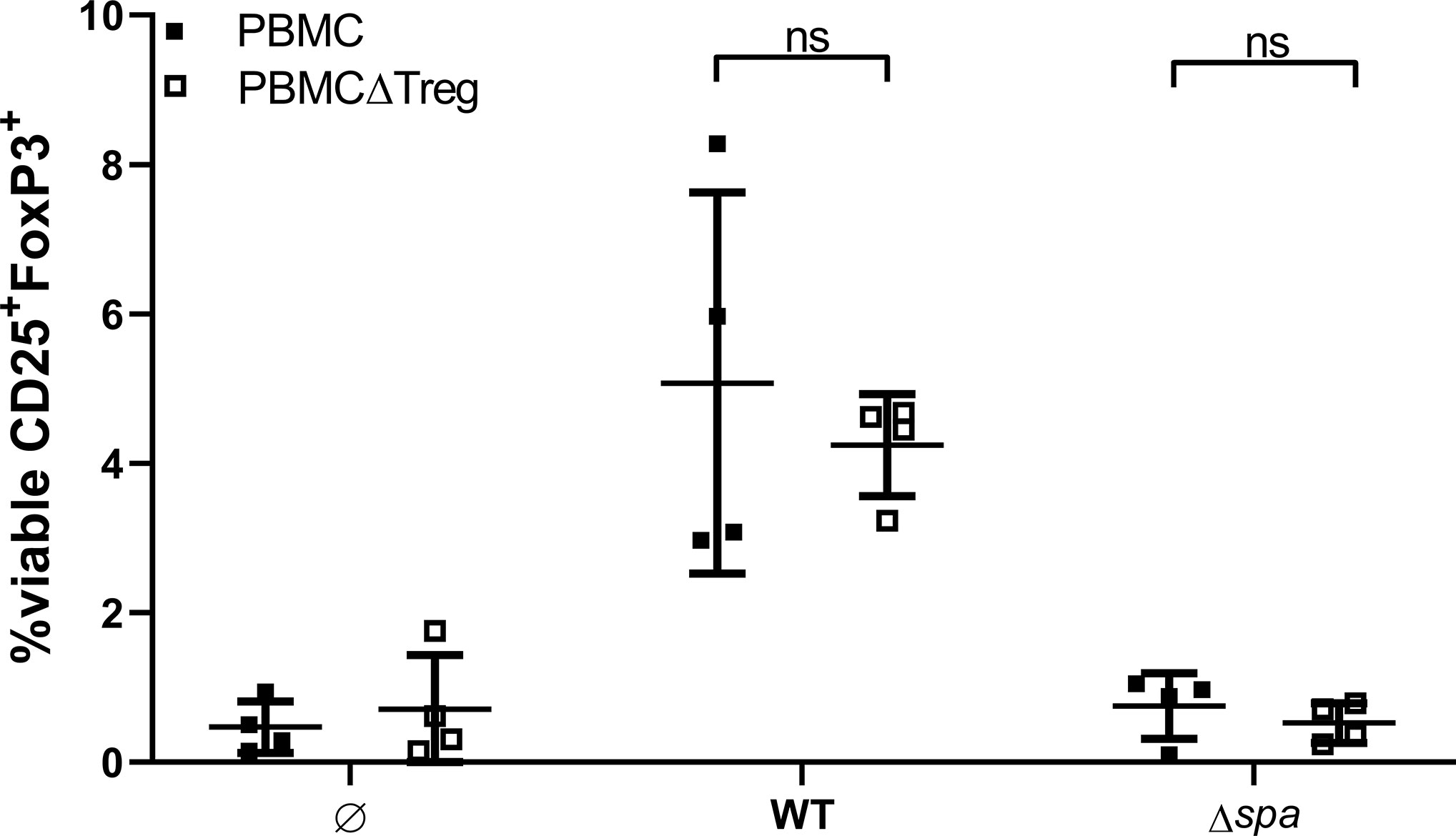
Figure 5 Sa restores Tregs in Treg-depleted Peripheral blood mononuclear cells (PBMC). Full PBMC (full squares) or PBMC depleted from CD3+CD4+CD25+CD127dim cells by flow cytometry (empty squares) were stimulated with SA113 WT or Δspa. After 5d, cells were re-stained and viable Tregs were detected by CD25+FoxP3+ expression. Two independent experiments with a total of n = 4 different donors are depicted as single values ± SD.
SpA Induces MoDC-Derived Soluble Factors That Are Sufficient to Generate Tregs
Furthermore, we analyzed whether direct cell contact between APC and T cells is required for Treg induction. To this end, we stimulated MoDC with SpA overnight. Since S. aureus is known to be a strong inducer of TLR2-mediated immune responses (48), we chose the TLR2 ligand P3C, as control that leads to high levels of IL-12p40 in MoDC (49). After stimulation of MoDC, their cell-free supernatants (SN) were applied to T cells only and Treg induction was analyzed after 5 days of culture (Figure 6). SN of SpA-stimulated MoDC induced comparable amounts of Tregs as shown above in direct MoDC/T cell cocultures (Figure 2B). Induced Treg levels in the condition with SN from P3C-stimulated MoDC were comparable to the unstimulated background control. In conclusion, soluble SpA-induced factors secreted by MoDC are sufficient to induce Tregs and direct cell-cell contact between APC and T cell is not required.
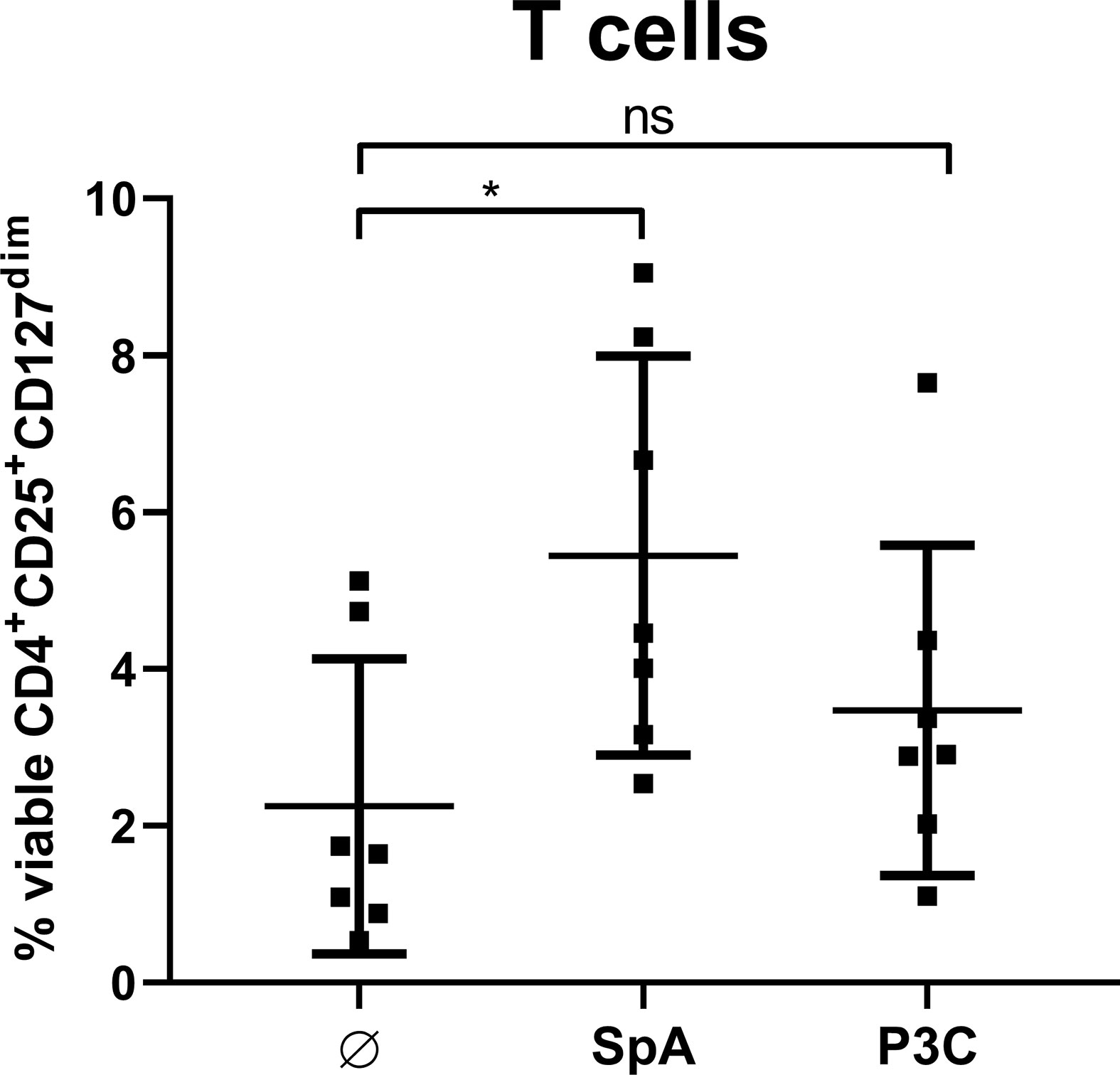
Figure 6 Soluble factors produced by monocyte-derived dendritic cells (MoDC) are sufficient to induce Treg. MoDC were stimulated with staphylococcal protein A (SpA) or P3C for 24 h. Their cell-free supernatant (SN) was added to autologous CD4+ T cells for 5d and Treg induction was measured by flow cytometry. Results are shown as values from n = 7 single donors ± SD, analyzed in three independent experiments. * indicates statistical significance level *p < 0.05.
S. aureus-Induced Treg-Enriched Supernatant Inhibits T Cell Proliferation
Finally, we wanted to address the question whether Sa-induced Treg cultures exert suppressive activity. We stimulated MoDC/T cell cocultures with SA113 WT and collected cell-free supernatants after 5d of culture. Induction of Tregs in these cocultures with HISA was confirmed by flow cytometry (Figure S8A) and Treg levels upon SA-stimulation did not differ when cells were stimulated with HISA or viable SA, or in different well sizes (Figure S8B). Next, supernatants from Treg-enriched cultures or fresh medium were added to autologous, CFSE-labeled CD4+ T cells, which were subsequently stimulated with anti-human CD3/CD28 beads. Proliferation was analyzed by flow cytometry after 4 days of culture.
Notably, addition of the Treg-enriched supernatant to CD3/CD28-stimulated T cells almost completely blocked proliferation (from 19.42% to 4.75% in average) reducing it to unstimulated level (1.2% in average). Thus, Treg-enriched SN did not induce nonspecific proliferation (Figure 7). Furthermore, T cell proliferation was not affected when SN from SA-stimulated MoDC cultures (without T cells) was used (data not shown). Thus, our results demonstrate suppressive activity of soluble factors released in Sa-induced Treg cocultures.
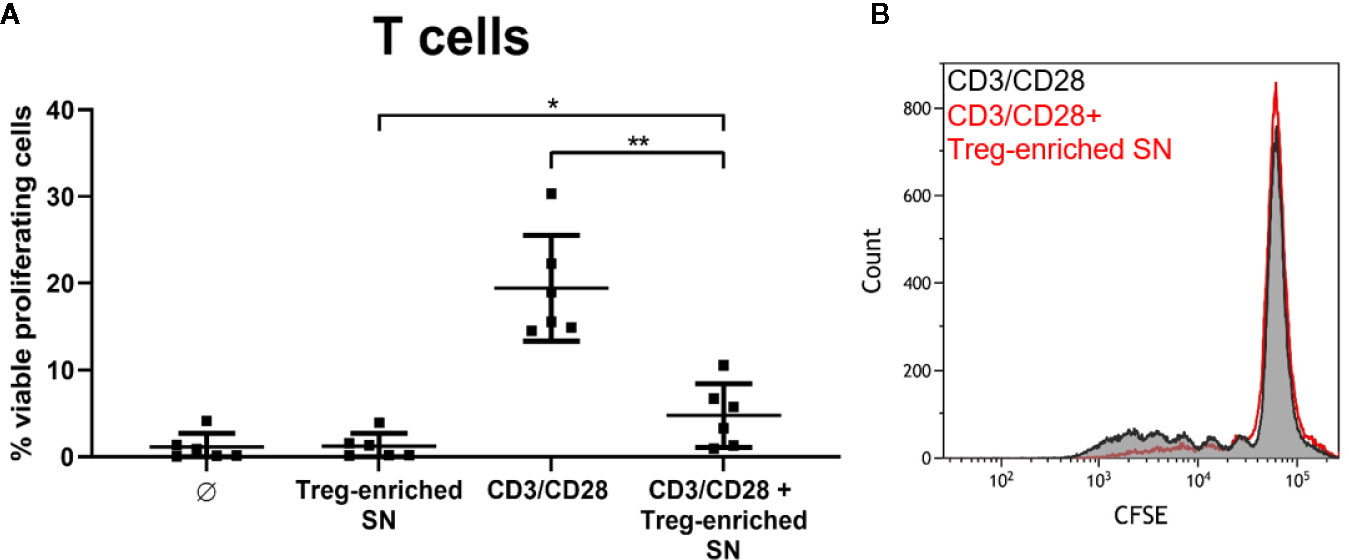
Figure 7 SA-induced, Treg-enriched supernatant has suppressive function. (A) Supernatant from monocyte-derived dendritic cells (MoDC)/T cell cocultures, stimulated with SA113 WT, was added to autologous CFSE-labeled CD4+ T cells. Upon stimulation with anti-CD3/CD28 beads, proliferation was analyzed by flow cytometry after 4d. Results are displayed as values from n = 6 single donors ± SD, analyzed in three independent experiments. Stars indicate statistical significance defined as *p < 0.05 and **p < 0.01. (B) CD4+ T cell proliferation upon stimulation with anti-CD3/CD28 beads ± Treg-enriched supernatant (SN) of one representative donor is shown as histogram.
Discussion
Due to high infestation rates and frequent exposure, the human immune system mounts an immune response but repeatedly fails to prevent Sa infection. This highlights the superb capacity of Sa to trigger immunoregulatory processes that suppress proinflammatory immune responses required for clearance of Sa (20). For instance, one of the major Sa virulence factors SpA is able to activate B cells, which leads to formation of IL-10-secreting plasmablasts and subsequent unspecific release of antibodies (31), well in line with the occurrence of nonprotective antibodies described in (50). Here, we describe a so far, unknown role of SpA in the induction of human Tregs.
Pathogen-induced control of T cell responses via induction of Bregs and Tregs may represent a prerequisite for colonization of the human body. A recent report highlighted a regulatory role of B cell superantigens in maintaining the balance of intestinal microbiota and immune response (51). Immune regulatory functions of SpA could, thus, support colonization of Sa in the respiratory tract.
Recent publications have highlighted other Sa virulence factors with Treg-inducing potential (41, 44–46). Here, we show that SpA is able to induce Tregs in PBMC and MoDC/T cell cocultures. Although SpA is well known as B cell superantigen, comparable Treg levels in MoDC/T cell cultures demonstrated that the presence of B cells is not required for Treg formation. Moreover, our data showed a prominent role for SpA in Treg induction because Treg induction was lower with the SA113 Δspa mutant (Figures 2A, B). However, the effect was enhanced when whole Sa cells were used for stimulation (Figures 2A, B). Thus, dual stimulation via SpA in combination with other bacterial compounds might increase the immunostimulatory signal (52, 53). Well in line with this concept, previous studies described PSMα as an inducer of tolerogenic, Treg-inducing dendritic cells and Tregs (43, 54). Notably, costimulation with TLR4 ligand LPS enhanced this effect (41). However, experiments performed with SA113 Δlgt, which lacksTLR2-activating lipoproteins, indicated that TLR2 ligands are not required for SpA-mediated Treg induction (Figure S4). Furthermore, absence of PSMα in SA113 strain (55) excludes synergistic activity of SpA and PSMα. However, close physical association of peptidoglycan with SpA could provide an additional stimulus supporting Treg-development (53, 56).
However, also an accessory role of other bacterial molecules such as contaminating enterotoxins cannot be excluded (44). Indeed, the SA113 strain expresses low levels of enterotoxins (57), which might be responsible for the residual Treg-inducing capacity observed with the SA113 Δspa mutant (Figures 2A, B).
Importantly, induction of Treg was not an antigen-dependent but rather an unspecific event because SpA antigen delivered as mRNA to MoDC failed to induce Treg (Figure 3). Assuming that comparable epitopes were presented to T cells (20), this suggests that SpA-mediated effects occur independently of antigen-specific T cell receptor activation. This was further corroborated by the finding that cell-cell contact was not required for Treg formation (Figure 6): supernatant of SpA- stimulated MoDC was sufficient to induce Tregs, comparable to levels obtained in SpA-stimulated MoDC/T cell cocultures (Figure 2B).
Of note, residual SpA in the Treg-inducing MoDC supernatants did not directly affect Treg induction because we saw no effect of SpA on T cells only (Figure 4C). Also, SpA did neither induce T cell proliferation nor apoptosis in cultures of CD4+ T cells only (Figure S9). Hence, in contrast to T cell superantigens such as enterotoxins that retain activity in the absence of MHCII binding (58), SpA interaction with the APC, rather than with the T cell, was crucial for Treg induction. Nevertheless, due to its agr-deficiency the Sa strain used in this study does not express PSM (55) and only low levels of enterotoxins (57). This, again, argues in favor of the Treg-inducing potential of SpA.
S. aureus is a strong regulator of the adaptive immune response (48). Interestingly, SpA induced low levels of IL-12p70, a cytokine associated with induction of Th1 cells. However, some publications also describe a role of IL-12 in inducing Tregs and other immunosuppressive cells (59, 60). Plus, IL-12 subunits are part of the cytokine family consisting of IL-23, IL-27 and IL-35, also involved in immune regulation (61).
So far, we can only speculate whether SpA, similar to PSMα (41, 42, 54) and staphylococcal enterotoxins (62) favors the generation of tolerogenic DC (tDC) or myeloid suppressor cells, cell subsets with strong Treg-inducing capacities (63, 64). While Schreiner et al. described IL-10 and TGFβ as relevant mediators (43), Richardson et al. suggested that Indolamin-2,3-Dioxygenase (IDO), a soluble mediator with immunosuppressive function (65) as important player in Sa-triggered Treg induction. However, blocking of IDO even increased Treg numbers (41). However, as shown in Figure S6 and published earlier (20), SpA did not induce strong secretion of proinflammatory cytokines or IL-10 in MoDC as seen with PSMα (41).
Other studies provided evidence that APC are important players in Treg induction in the context of Sa infection. Experiments carried out with cell-free supernatant of an enterotoxin-expressing Sa strain, induced expression of IL-10, IFNγ and IL-17A in FoxP3+ cells. This effect was decreased when monocytes were depleted from PBMC. However, in this study the main activating Sa component remained unidentified (66). When Rabe et al. blocked the interaction between PD-1 and PD-L1, the proportion of FOXP3+ CD25+ CD127dim T cells was reduced upon Sa stimulation (46), highlighting the role of APC in this process. Taken together, our data complement the previous findings by providing evidence that interaction of SpA with APC is mandatory for release of Treg-inducing soluble factors. However, future work is needed to identify the driving factors.
Interestingly, the Treg-inducing potential of SpA was completely blocked in the presence of human serum (Figure 4). This might support the hypothesis that Ig-binding sites are crucial for Treg induction but it could also result from the presence of SpA neutralizing antibodies. Thus, we can only speculate whether the SpA Ig–binding sites interact with an APC surface receptor, or whether formation of regular or aberrant immune complexes of SpA and Ig hinders interaction with APC. Previous work suggested that the SpA Ig-binding sites are required for shedding of soluble TNF-receptor I from the surface of airway cells, leading to anti-inflammatory immune responses (67, 68). Although this was an attractive hypothesis, we were not able to confirm TNFR1 binding or engagement of an alternative receptor specific for SpA in pulldown assays and overexpression studies performed in our lab (31).
We further hypothesize that post-translational modifications of SpA such as glycosylation may also play a role in interaction with MoDC surface receptors because induction of Tregs in MoDC/T cell cocultures was not seen when cells were stimulated with recombinant SpA (Figure S10). By contrast, Treg induction by whole bacteria was not altered in different sera, indicating that formation of functional immune complexes does not influence Treg induction. Also, reduction of Treg levels with Sa Δspa and overall Treg percentage was comparable to experiments carried out in Ig-free medium (Figures 4A, B). Of note, Treg induction did not depend on vitaPAMPs such as DNA or RNA, because Treg levels were comparable when cells were stimulated with HISA (Figure S8).
Tolerogenic DC can convert naïve T cells into suppressive Tregs (63). Depletion of CD3+CD4+CD25+CD127dim Tregs from PBMC and subsequent stimulation with Sa WT or Δspa for 5 days led to recovery of CD4+CD25+FoxP3+Tregs of comparable levels to baseline. We are aware that these two Treg populations are not identical and gating strategies not completely overlapping (69), which might be a reason why Treg percentages in Figure 5 did not reach Treg levels displayed in Figure 2A. However, in some donors, cells were unspecifically activated through sorting, resulting in big proportion of CD25high T cells. For this reason, we decided to look for expression of CD25 in combination with CD4 and FoxP3 after sort. It has been shown by others that Sa (46) and Sa superantigens (45) are able to convert CD25- T cells into Tregs with regulatory function. Also Richardson and colleagues demonstrated the induction of Tregs from naïve T cells upon stimulation with PSMα (41) and Björkander et al. showed de novo expression of FoxP3 in CD4+CD25+-depleted T cell cultures after stimulation with cell free Sa supernatant (66).
Taken together, our data provide evidence for a novel immunosuppressory role of Sa virulence factor SpA in the induction of Tregs, including a pivotal role of APC. Importantly, our data demonstrate that Sa–induced Tregs possess strong suppressive capacities. Supernatants from Sa-derived Treg-enriched MoDC/T cell cocultures almost completely blocked T cell proliferation upon polyclonal stimulation by anti-CD3/CD28 beads (Figure 7).
We are aware that our data was fully acquired in vitro. Hence, we can only speculate whether SpA induces Tregs in vivo and whether this interferes with development of a protective immune response or whether it could be beneficial for the human host by blocking excessive inflammatory responses. Against this background, Sa-mediated immune suppression might represent a challenge for vaccine effectiveness. Future work should take this into account.
Data Availability Statement
All datasets presented in this study are included in the article/Supplementary Material.
Author Contributions
JU and IB-D designed the study and planned the experiments. JU, KH, and OT performed and analyzed the experiments. JU, OT, and IB-D interpreted the results. JU and OT prepared the figures. JU and IB-D wrote the manuscript.
Funding
This research was funded by the Paul-Ehrlich-Institut. It received no specific grant from any funding agency in the public, commercial, or not-for-profit sectors.
Conflict of Interest
The authors declare that the research was conducted in the absence of any commercial or financial relationships that could be construed as a potential conflict of interest.
Acknowledgments
We thank Bjoern Becker for technical support with cytokine multiplex assays, Alexander Pastura and Christoph Stein for support with MoDC cultures, and Prof. Friedrich Götz (University of Tübingen, Germany) for providing SA113 strains and mutants.
Supplementary Material
The Supplementary Material for this article can be found online at: https://www.frontiersin.org/articles/10.3389/fimmu.2020.581713/full#supplementary-material
References
1. Klein E, Smith DL, Laxminarayan R. Hospitalizations and Deaths Caused by Methicillin-Resistant Staphylococcus Aureus, United States, 1999–2005. Emerg Infect Dis (2007) 13:1840–6. doi: 10.3201/Eid1312.070629
2. Schmidt A, Benard S, Cyr S. Hospital Cost of Staphylococcal Infection After Cardiothoracic or Orthopedic Operations in France: a Retrospective Database Analysis. Surg Infect (Larchmt) (2015) 16:428–35. doi: 10.1089/Sur.2014.045
3. Antonanzas F, Lozano C, Torres C. Economic Features of Antibiotic Resistance: the Case of Methicillin-Resistant Staphylococcus Aureus. Pharmacoeconomics (2015) 33:285–325. doi: 10.1007/S40273-014-0242-Y
4. Sakr A, Brégeon F, Mège J-L, Rolain J-M, Blin O. Staphylococcus Aureus Nasal Colonization: an Update on Mechanisms, Epidemiology, Risk Factors, and Subsequent Infections. Front Microbiol (2018) 9:2419 1–15. doi: 10.3389/Fmicb.2018.02419
5. Sakoulas G. Moellering Rc. Increasing Antibiotic Resistance Among Methicillin-Resistant Staphylococcus Aureus Strains. Clin Infect Dis (2008) 46(Suppl 5):S360–7. doi: 10.1086/533592
6. O’brien EC, Mcloughlin RM. Considering the ‘Alternatives’ for Next-Generation Anti-Staphylococcus Aureus Vaccine Development. Trends Mol Med (2019) 25:171–84. doi: 10.1016/J.Molmed.2018.12.010
7. Giersing BK, Dastgheyb SS, Modjarrad K, Moorthy V. Status of Vaccine Research and Development of Vaccines for Staphylococcus Aureus. Vaccine (2016) 34:2962–6. doi: 10.1016/J.Vaccine.2016.03.110
8. Fowler VG, Proctor RA. Where Does a Staphylococcus Aureus Vaccine Stand? Clin Microbiol Infect (2014) 20(Suppl 5):66–75. doi: 10.1111/1469-0691.12570
9. Dryla A, Prustomersky S, Gelbmann D, Hanner M, Bettinger E, Kocsis B, et al. Comparison of Antibody Repertoires Against Staphylococcus Aureus in Healthy Individuals and in Acutely Infected Patients. Clin Diagn Lab Immunol (2005) 12:387–98. doi: 10.1128/Cdli.12.3.387-398.2005
10. Van Belkum A, Verkaik NJ, De Vogel CP, Boelens HA, Verveer J, Nouwen JL, et al. Reclassification of Staphylococcus Aureus Nasal Carriage Types. J Infect Dis (2009) 199:1820–6. doi: 10.1086/599119
11. Bröker BM, Mrochen D, Péton V. The T Cell Response to Staphylococcus Aureus. Pathogens (2016) 5:31. doi: 10.3390/Pathogens5010031
12. Karauzum H, Datta SK. Adaptive Immunity Against Staphylococcus Aureus. Curr Top Microbiol Immunol (2017) 409:419–39. doi: 10.1007/82_2016_1
13. Zhao YX, Tarkowski A. Impact of Interferon-Gamma Receptor Deficiency on Experimental Staphylococcus Aureus Septicemia and Arthritis. J Immunol (1995) 155:5736–42.
14. Wiese L, Mejer N, Schønheyder HC, Westh H, Jensen AG, Larsen AR, et al. A Nationwide Study of Comorbidity and Risk of Reinfection After Staphylococcus Aureus Bacteraemia. J Infect (2013) 67:199–205. doi: 10.1016/J.Jinf.2013.04.018
15. Lin L, Ibrahim AS, Xu X, Farber JM, Avanesian V, Baquir B, et al. Th1-Th17 Cells Mediate Protective Adaptive Immunity Against Staphylococcus Aureus and Candida Albicans Infection in Mice. PLoS Pathog (2009) 5:E1000703. doi: 10.1371/Journal.Ppat.1000703
16. Mancini F, Monaci E, Lofano G, Torre A, Bacconi M, Tavarini S, et al. One Dose of Staphylococcus Aureus 4c-Staph Vaccine Formulated With a Novel Tlr7-Dependent Adjuvant Rapidly Protects Mice Through Antibodies, Effector Cd4+ T Cells, and Il-17a. PLoS One (2016) 11:E0147767. doi: 10.1371/Journal.Pone.0147767
17. Montgomery CP, Daniels M, Zhao F, Alegre M-L, Chong AS, Daum RS. Protective Immunity Against Recurrent Staphylococcus Aureus Skin Infection Requires Antibody and Interleukin-17a. Infect Immun (2014) 82:2125–34. doi: 10.1128/Iai.01491-14
18. Zielinski CE, Mele F, Aschenbrenner D, Jarrossay D, Ronchi F, Gattorno M, et al. Pathogen-Induced Human Th17 Cells Produce Ifn-Γ or Il-10 and Are Regulated by Il-1β. Nature (2012) 484:514–8. doi: 10.1038/Nature10957
19. Misstear K, Mcneela EA, Murphy AG, Geoghegan JA, O’keeffe KM, Fox J, et al. Targeted Nasal Vaccination Provides Antibody-Independent Protection Against Staphylococcus Aureus. J Infect Dis (2014) 209:1479–84. doi: 10.1093/Infdis/Jit636
20. Uebele J, Stein C, Nguyen M-T, Schneider A, Kleinert F, Ticha O, et al. Antigen Delivery to Dendritic Cells Shapes Human Cd4+ and Cd8+ T Cell Memory Responses to Staphylococcus Aureus. PLoS Pathog (2017) 13:E1006387. doi: 10.1371/Journal.Ppat.1006387
21. Brown AF, Murphy AG, Lalor SJ, Leech JM, O’keeffe KM, Mac Aogain M, et al. Memory Th1 Cells Are Protective in Invasive Staphylococcus Aureus Infection. PLoS Pathog (2015) 11:E1005226. doi: 10.1371/Journal.Ppat.1005226
22. Kolata JB, Kühbandner I, Link C, Normann N, Vu CH, Steil L, et al. The Fall of a Dogma? Unexpected High T-Cell Memory Response to Staphylococcus Aureus in Humans. J Infect Dis (2015) 215(5):830–8. doi: 10.1093/Infdis/Jiv128
23. Dedent AC, Mcadow M, Schneewind O. Distribution of Protein a on the Surface of Staphylococcus Aureus. J Bacteriol (2007) 189:4473–84. doi: 10.1128/Jb.00227-07
24. Forsgren A. Protein a From Staphylococcus Aureus. 8. Production of Protein a by Bacterial and L-Forms of S. Aureus. Acta Pathol Microbiol Scand (1969) 75:481–90.
25. Falugi F, Kim HK, Missiakas DM, Schneewind O. Role of Protein a in the Evasion of Host Adaptive Immune Responses by Staphylococcus Aureus. Mbio (2013) 4:13. doi: 10.1128/Mbio.00575-13
26. Sjodahl J. Structural Studies on the Four Repetitive Fc-Binding Regions in Protein a From Staphylococcus Aureus. Eur J Biochem (1977) 78:471–90. doi: 10.1111/j.1432-1033.1977.tb11760.x.
27. Dossett JH, Kronvall G, Williams RC Jr, Quie PG. Antiphagocytic Effects of Staphylococcal Protein A. J Immunol (1969) 103:1405–10.
28. Goodyear CS, Silverman GJ. Death by a B Cell Superantigen: in Vivo Vh-Targeted Apoptotic Supraclonal B Cell Deletion by a Staphylococcal Toxin. J Exp Med (2003) 197:1125–39. doi: 10.1084/Jem.20020552
29. Anderson AL, Sporici R, Lambris J, Larosa D, Levinson AI. Pathogenesis of B-Cell Superantigen-Induced Immune Complex-Mediated Inflammation. Infect Immun (2006) 74:1196–203. doi: 10.1128/Iai.74.2.1196-1203.2006
30. Palmqvist N, Silverman GJ, Josefsson E, Tarkowski A. Bacterial Cell Wall-Expressed Protein a Triggers Supraclonal B-Cell Responses Upon in Vivo Infection With Staphylococcus Aureus. Microbes Infect (2005) 7:1501–11. doi: 10.1016/J.Micinf.2005.05.008
31. Parcina M, Miranda-Garcia MA, Durlanik S, Ziegler S, Over B, Georg P, et al. Pathogen-Triggered Activation of Plasmacytoid Dendritic Cells Induces Il-10-Producing B Cells in Response to Staphylococcus Aureus. J Immunol (2013) 190:1591–602. doi: 10.4049/Jimmunol.1201222
32. Ticha O, Moos L, Wajant H, Bekeredjian-Ding I. Expression of Tumor Necrosis Factor Receptor 2 Characterizes Tlr9-Driven Formation of Interleukin-10-Producing B Cells. Front Immunol (2018) 8:1951. doi: 10.3389/Fimmu.2017.01951
33. Sakaguchi S, Sakaguchi N, Asano M, Itoh M, Toda M. Immunologic Self-Tolerance Maintained by Activated T Cells Expressing Il-2 Receptor Alpha-Chains (Cd25). Breakdown of a Single Mechanism of Self-Tolerance Causes Various Autoimmune Diseases. the. J Immunol (1995) 155:1151–64.
34. Sakaguchi S, Yamaguchi T, Nomura T, Ono M. Regulatory T Cells and Immune Tolerance. Cell (2008) 133:775–87. doi: 10.1016/J.Cell.2008.05.009
35. Sharabi A, Tsokos MG, Ding Y, Malek TR, Klatzmann D, Tsokos GC. Regulatory T Cells in the Treatment of Disease. Nat Rev Drug Discov. (2018) 17:823–44. doi: 10.1038/Nrd.2018.148
36. Yu N, Li X, Song W, Li D, Yu D, Zeng X, et al. Cd4(+)Cd25 (+)Cd127 (Low/-) T Cells: a More Specific Treg Population in Human Peripheral Blood. Inflammation (2012) 35:1773–80. doi: 10.1007/S10753-012-9496-8
37. Dey I, Bishayi B. Role of Th17 and Treg Cells in Septic Arthritis and the Impact of the Th17/Treg -Derived Cytokines in the Pathogenesis of S. Aureus Induced Septic Arthritis in Mice. Microb Pathog (2017) 113:248–64. doi: 10.1016/J.Micpath.2017.10.033
38. Dey I, Bishayi B. Impact of Simultaneous Neutralization of Il-17a and Treatment With Recombinant Il-2 on Th17-Treg Cell Population in S.Aureus Induced Septic Arthritis. Microb Pathog (2020) 139:103903. doi: 10.1016/J.Micpath.2019.103903
39. Ali A, Welin A, Schwarze J-C, Svensson MN, Na M, Jarneborn A, et al. Ctla4 Immunoglobulin But Not Anti-Tumor Necrosis Factor Therapy Promotes Staphylococcal Septic Arthritis in Mice. J Infect Dis (2015) 212:1308–16. doi: 10.1093/Infdis/Jiv212
40. Ou L-S, Goleva E, Hall C, Leung DY. T Regulatory Cells in Atopic Dermatitis and Subversion of Their Activity by Superantigens. J Allergy Clin Immunol (2004) 113:756–63. doi: 10.1016/J.Jaci.2004.01.772
41. Richardson JR, Armbruster NS, Günter M, Henes J, Autenrieth SE. Staphylococcus Aureus Psm Peptides Modulate Human Monocyte-Derived Dendritic Cells to Prime Regulatory T Cells. Front Immunol (2018) 9:2603. doi: 10.3389/Fimmu.2018.02603
42. Richardson JR, Armbruster NS, Günter M, Biljecki M, Klenk J, Heumos S, et al. Psm Peptides From Community-Associated Methicillin-Resistant Staphylococcus Aureus Impair the Adaptive Immune Response Via Modulation of Dendritic Cell Subsets in Vivo. Front Immunol (2019) 10:995. doi: 10.3389/Fimmu.2019.00995
43. Schreiner J, Kretschmer D, Klenk J, Otto M, Bühring H-J, Stevanovic S, et al. Staphylococcus Aureus Phenol-Soluble Modulin Peptides Modulate Dendritic Cell Functions and Increase in Vitro Priming of Regulatory T Cells. J Immunol (2013) 190:3417–26. doi: 10.4049/Jimmunol.1202563
44. Lee J, Park N, Park JY, Kaplan BL, Pruett SB, Park JW, et al. Induction of Immunosuppressive Cd8+Cd25+Foxp3+ Regulatory T Cells by Suboptimal Stimulation With Staphylococcal Enterotoxin C1. J Immunol (2018) 200:669–80. doi: 10.4049/Jimmunol.1602109
45. Taylor AL, Llewelyn MJ. Superantigen-Induced Proliferation of Human Cd4+Cd25– T Cells Is Followed by a Switch to a Functional Regulatory Phenotype. J Immunol (2010) 185:6591–8. doi: 10.4049/Jimmunol.1002416
46. Rabe H, Nordström I, Andersson K, Lundell A-C, Rudin A. Staphylococcus Aureus Convert Neonatal Conventional Cd4(+) T Cells Into Foxp3(+) Cd25(+) Cd127(Low) T Cells Via the Pd-1/Pd-L1 Axis. Immunology (2014) 141:467–81. doi: 10.1111/Imm.12209
47. Larsson A, Wejåker PE, Sjöquist J. Chicken Anti-Protein a for the Detection and Capturing of Protein a From Staphylococcus Aureus in the Presence or Absence of Mammalian Igg. Hybridoma (1992) 11:239–43. doi: 10.1089/Hyb.1992.11.239
48. Bekeredjian-Ding I, Stein C, Uebele J. The Innate Immune Response Against Staphylococcus Aureus. Curr Top Microbiol Immunol (2015) 409:385–418. doi: 10.1007/82_2015_5004
49. Nguyen M-T, Uebele J, Kumari N, Nakayama H, Peter L, Ticha O, et al. Lipid Moieties on Lipoproteins of Commensal and Non-Commensal Staphylococci Induce Differential Immune Responses. Nat Commun (2017) 8:1–12. doi: 10.1038/S41467-017-02234-4
50. Pauli NT, Hk K, Falugi F, Huang M, Dulac J, Henry Dunand C, et al. Staphylococcus Aureus Infection Induces Protein A-Mediated Immune Evasion in Humans. J Exp Med (2014) 211:2331–9. doi: 10.1084/Jem.20141404
51. Bunker JJ, Drees C, Watson AR, Plunkett CH, Nagler CR, Schneewind O, et al. B Cell Superantigens in the Human Intestinal Microbiota. Sci Transl Med (2019) 11:1–14. doi: 10.1126/Scitranslmed.Aau9356
52. Bekeredjian-Ding I, Inamura S, Giese T, Moll H, Endres S, Sing A, et al. Staphylococcus Aureus Protein a Triggers T Cell-Independent B Cell Proliferation by Sensitizing B Cells for Tlr2 Ligands. J Immunol (2007) 178:2803–12. doi: 10.4049/Jimmunol.178.5.2803
53. Kim HK, Falugi F, Missiakas DM, Schneewind O. Peptidoglycan-Linked Protein a Promotes T Cell-Dependent Antibody Expansion During Staphylococcus Aureus Infection. Proc Natl Acad Sci U S A (2016) 113(20):5718–23. doi: 10.1073/Pnas.1524267113
54. Armbruster NS, Richardson C, Schreiner J, Klenk J, Günter M, Autenrieth SE. Staphylococcus Aureus Psm Peptides Induce Tolerogenic Dendritic Cells Upon Treatment With Ligands of Extracellular and Intracellular Tlrs. Int J Med Microbiol (2016) 306:1–16. doi: 10.1016/J.Ijmm.2016.09.002
55. Schlatterer K, Beck C, Hanzelmann D, Lebtig M, Fehrenbacher B, Schaller M, et al. The Mechanism Behind Bacterial Lipoprotein Release: Phenol-Soluble Modulins Mediate Toll-Like Receptor 2 Activation Via Extracellular Vesicle Release From Staphylococcus Aureus. Mbio (2018) 9:1–13. doi: 10.1128/Mbio.01851-18
56. O’halloran DP, Wynne K, Geoghegan JA. Protein a Is Released Into the Staphylococcus Aureus Culture Supernatant With an Unprocessed Sorting Signal. Infect Immun (2015) 83:1598–609. doi: 10.1128/Iai.03122-14
57. Cao R, Zeaki N, Wallin-Carlquist N, . Skandamis PN, Schelin J, Rådström P. Elevated Enterotoxin a Expression and Formation in Staphylococcus Aureus and Its Association With Prophage Induction. Appl Environ Microbiol (2012) 78:4942–8. doi: 10.1128/Aem.00803-12
58. Stich N, Waclavicek M, Model N, Eibl MM. Staphylococcal Superantigen (Tsst-1) Mutant Analysis Reveals That T Cell Activation Is Required for Biological Effects in the Rabbit Including the Cytokine Storm. Toxins (2010) 2:2272–88. doi: 10.3390/Toxins2092272
59. Verma ND, Hall BM, Plain KM, Robinson CM, Boyd R, Tran GT, et al. Interleukin-12 (Il-12p70) Promotes Induction of Highly Potent Th1-Like Cd4+Cd25+ T Regulatory Cells That Inhibit Allograft Rejection in Unmodified Recipients. Front Immunol (2014) 5:190. doi: 10.3389/Fimmu.2014.00190
60. Heim CE, Vidlak D, Scherr TD, Hartman CW, Garvin KL, Kielian T. Il-12 Promotes Myeloid-Derived Suppressor Cell Recruitment and Bacterial Persistence During Staphylococcus Aureus Orthopedic Implant Infection. J Immunol (2015) 194:3861–72. doi: 10.4049/Jimmunol.1402689
61. Sun L, He C, Nair L, Yeung J, Egwuagu CE. Interleukin 12 (Il-12) Family Cytokines: Role in Immune Pathogenesis and Treatment of Cns Autoimmune Disease. Cytokine (2015) 75:249–55. doi: 10.1016/J.Cyto.2015.01.030
62. Stoll H, Ost M, Singh A, Mehling R, Neri D, Schäfer I, et al. Staphylococcal Enterotoxins Dose-Dependently Modulate the Generation of Myeloid-Derived Suppressor Cells. Front Cell Infect Microbiol (2018) 8:321. doi: 10.3389/Fcimb.2018.00321
63. Domogalla MP, Rostan PV, Raker VK, Steinbrink K. Tolerance Through Education: How Tolerogenic Dendritic Cells Shape Immunity. Front Immunol (2017) 8:1764. doi: 10.3389/Fimmu.2017.01764
64. Tebartz C, Horst SA, Sparwasser T, Huehn J, Beineke A, Peters G, et al. A Major Role for Myeloid-Derived Suppressor Cells and a Minor Role for Regulatory T Cells in Immunosuppression During Staphylococcus Aureus Infection. J Immunol (2015) 194:1100–11. doi: 10.4049/Jimmunol.1400196
65. Harden JL, Egilmez NK. Indoleamine 2,3-Dioxygenase and Dendritic Cell Tolerogenicity. Immunol Invest (2012) 41:738–64. doi: 10.3109/08820139.2012.676122
66. Björkander S, Hell L, Johansson MA, Forsberg MM, Lasaviciute G, Roos S, et al. Staphylococcus Aureus -Derived Factors Induce Il-10, Ifn-Γ and Il-17a-Expressing Foxp3 + Cd161 + T-Helper Cells in a Partly Monocyte-Dependent Manner. Sci Rep (2016) 6:1–13. doi: 10.1038/Srep22083
67. Gómez MI, Lee A, Reddy B, Muir A, Soong G, Pitt A, et al. Staphylococcus Aureus Protein a Induces Airway Epithelial Inflammatory Responses by Activating Tnfr1. Nat Med (2004) 10:842–8. doi: 10.1038/Nm1079
68. Ledo C, Gonzalez CD, Poncini CV, Mollerach M, Gómez MI. Tnfr1 Signaling Contributes to T Cell Anergy During Staphylococcus Aureus Sepsis. Front Cell Infect Microbiol (2018) 8:259. doi: 10.3389/Fcimb.2018.00259
Keywords: Staphylococcus aureus, Treg—regulatory T cell, Ig-binding proteins, protein A, tolerance, immune suppression, immune evasion, human
Citation: Uebele J, Habenicht K, Ticha O and Bekeredjian-Ding I (2020) Staphylococcus aureus Protein A Induces Human Regulatory T Cells Through Interaction With Antigen-Presenting Cells. Front. Immunol. 11:581713. doi: 10.3389/fimmu.2020.581713
Received: 09 July 2020; Accepted: 14 September 2020;
Published: 30 September 2020.
Edited by:
Paul Laszlo Bollyky, Stanford University, United StatesReviewed by:
Zhipeng Xu, Nanjing Medical University, ChinaGiang Tran, University of New South Wales, Australia
Copyright © 2020 Uebele, Habenicht, Ticha and Bekeredjian-Ding. This is an open-access article distributed under the terms of the Creative Commons Attribution License (CC BY). The use, distribution or reproduction in other forums is permitted, provided the original author(s) and the copyright owner(s) are credited and that the original publication in this journal is cited, in accordance with accepted academic practice. No use, distribution or reproduction is permitted which does not comply with these terms.
*Correspondence: Isabelle Bekeredjian-Ding, aXNhYmVsbGUuYmVrZXJlZGppYW4tZGluZ0BwZWkuZGU=