- 1Maoming Branch, Guangdong Laboratory for Lingnan Modern Agriculture, Guangdong Provincial Key Laboratory of Animal Nutrition Control, National Engineering Research Center for Breeding Swine Industry, College of Animal Science, South China Agricultural University, Guangzhou, China
- 2National Engineering Laboratory for Pollution Control and Waste Utilization in Livestock and Poultry Production, Key Laboratory of Agro-ecological Processes in Subtropical Region, Institute of Subtropical Agriculture, Chinese Academy of Sciences, Changsha, China
Gallic acid (GA) is a naturally occurring polyphenol compound present in fruits, vegetables, and herbal medicines. According to previous studies, GA has many biological properties, including antioxidant, anticancer, anti-inflammatory, and antimicrobial properties. GA and its derivatives have multiple industrial uses, such as food supplements or additives. Additionally, recent studies have shown that GA and its derivatives not only enhance gut microbiome (GM) activities, but also modulate immune responses. Thus, GA has great potential to facilitate natural defense against microbial infections and modulate the immune response. However, the exact mechanisms of GA acts on the GM and immune system remain unclear. In this review, first the physicochemical properties, bioavailability, absorption, and metabolism of GA are introduced, and then we summarize recent findings concerning its roles in gastrointestinal health. Furthermore, the present review attempts to explain how GA influences the GM and modulates the immune response to maintain intestinal health.
Introduction
Gallic acid (GA), 3,4,5-trihydroxybenzoic acid, is a polyphenol compound (1) and has gradually won a considerable amount of attention because it is ubiquitous in fruits, vegetables, and herbal medicines, such as grapes (2–4), gallnuts (5, 6), pomegranates (7, 8), and tea leaves (9, 10). In 1786, Carl Wilhelm Scheele, a famous Swedish chemist, was the first to identify and isolate GA and pyrogallic acid from plants (11). Since then, reports on GA and its derivatives have gradually increased, which has increased awareness in the understanding of GA. In addition to the edible uses of GA and its ester derivatives as flavoring agents and preservatives in the food industry (12, 13), there are also various kinds of studies on their biological and pharmacological activities, including antioxidant (14, 15), antimicrobial (16, 17), anticancer (18, 19), anti-inflammatory (20, 21), gastroprotective (22–25), cardioprotective (26, 27), neuroprotective (28–30), and metabolic disease prevention activities (31–33). To date, however, virtually no published studies exist on the mechanisms of action of GA through the gut microbiome (GM) and immune response.
Therefore, in this review, we first cover the physicochemical properties, absorption, and metabolism of GA and then summarize recent findings concerning their roles in gastrointestinal diseases. Moreover, the current review tries to shed light on the regulatory mechanism of GA through modulation of the GM and immune response. Finally, we summarize our findings based on the obtained information and provide an outlook for further investigations. Relevant references and data for this review were derived from the Web of Science and PubMed databases, from which we chose the most relevant literatures that have investigated the effect of GA and its derivatives on the treatment or prevention of gastrointestinal diseases, especially focusing on the GM and immune response.
Physicochemical Properties of GA
Frequently, polyphenols are mainly divided into two categories, including flavonoids (anthocyanins, flavanols, flavanones, flavonols, flavonones, and isoflavones) and non-flavonoids (phenolic acids, xanthones, stilbenes, lignans, and tannins). Phenolic acids arise from two major phenolic compounds: benzoic acids and cinnamic acids, separately based on the C1-C6 and C3-C6 backbones. p-Hydroxybenzoic acid, protocatechuic acid, vanillic acid, GA, and syringic acid are hydroxybenzoic derivatives, and hydroxycinnamic acids include p-coumaric acid, ferulic acid, caffeic acid, and sinapic acid (34, 35). Due to their different structures, hydroxycinnamic acids show higher antibacterial activity than hydroxybenzoic acids (36). Tannins are classified as hydrolysable tannins (HTs) and condensed tannins (CTs) (11). HTs contain a glucose unit and esterified gallic acid. As presented in Figure 1, GA is a trihydroxybenzoic acid with the molecular formula C7H6O5 and molecular weight of 170.12 g/mol, and hydroxy groups are at positions 3, 4, and 5. It is a colorless or slightly yellow crystalline compound, and the melting point is 210°C, with decomposition between 235°C and 240°C producing carbon dioxide and carbon monoxide. Its density is 1.69 kg/L, its pKa is 4.40, and its log P is 0.70 at 20°C. It is soluble in water, alcohol, ether, and glycerol, and practically insoluble in benzene, chloroform, and ether petroleum (1). GA is a secondary metabolite widely distributed in several fruits, vegetables, and herbal medicines (37), and it is used in photography, pharmaceuticals, and analytical reagents (38). GA is found both free and as part of HTs. It is the most basic constituent donor used to synthesize HTs through esterification of GA with glucose and products of their oxidative reactions. HTs contains mainly glucogallin, gallotannins, ellagitannins, and their derivatives (39). Tannase (a glycoprotein esterase) hydrolyzes GA from gallotannins, thereby increasing available GA absorbed in the gastrointestinal tract (GIT) (40, 41). The GA groups are usually bonded to form dimers, such as ellagic acid.
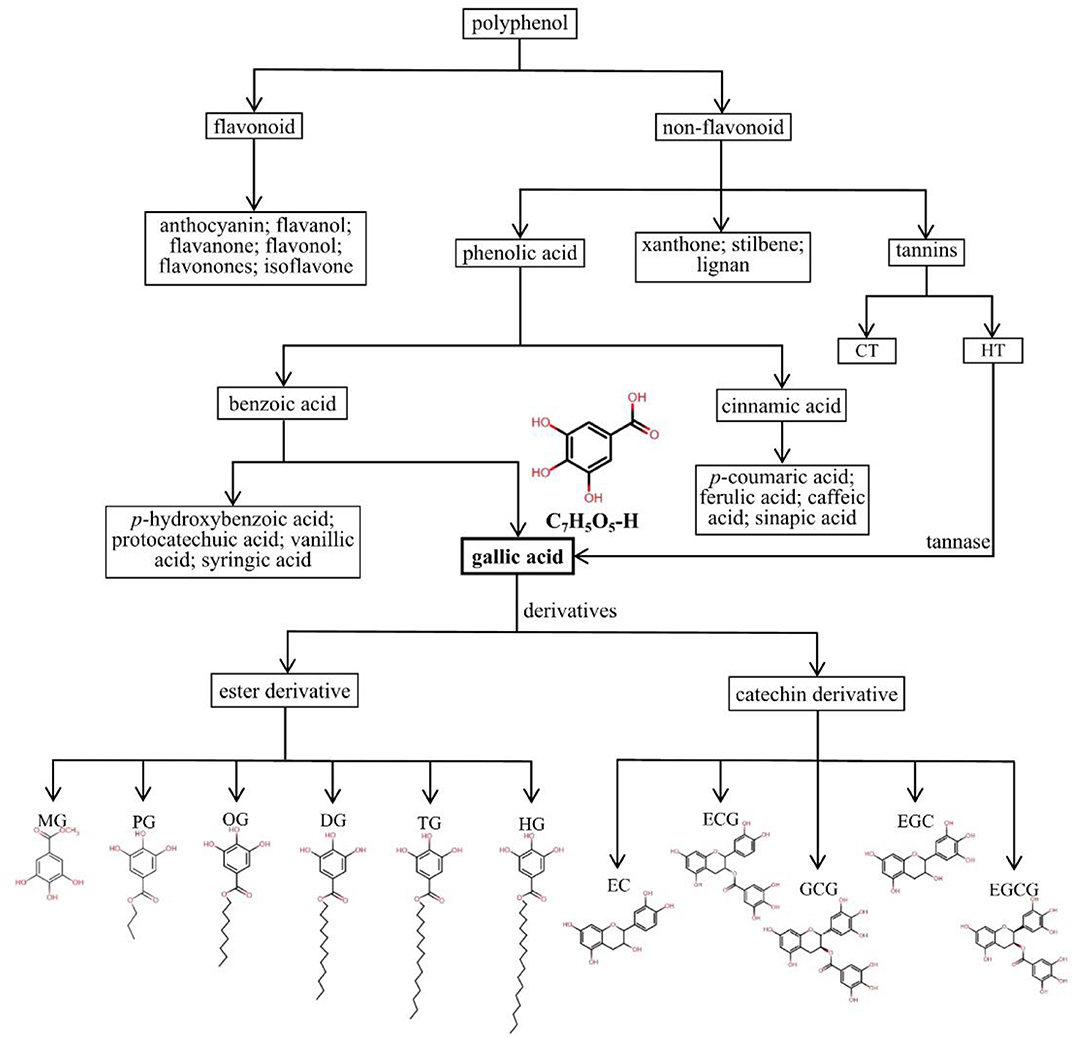
Figure 1. Detailed classification and chemical structures of polyphenols, phenolic acids, GA, and its derivatives. HTs, hydrolysable tannins; CTs, condensed tannins; MG, methyl gallate, C7H5O5-CH3; PG, propyl gallate, C7H5O5-(CH2)2-CH3; OG, octyl gallate, C7H5O5-(CH2)7-CH3; DG, dodecyl gallate, C7H5O5-(CH2)11-CH3; TG, tetradecyl gallate, C7H5O5-(CH2)13-CH3; HG, hexadecyl gallate, C7H5O5-(CH2)15-CH3; EC, epicatechin, C15H14O6; ECG, epicatechin gallate, C22H18O10; GCG, gallocatechin gallate, C22H18O11; EGC, epigallocatechin, C15H14O7; EGCG, epigallocatechin gallate, C22H18O11.
The GA derivatives include two types: ester and catechin derivatives. The most common ester derivatives of GA are alkyl esters, which are composed mainly of methyl gallate (MG), propyl gallate (PG), octyl gallate (OG), dodecyl gallate (DG), tetradecyl gallate (TG), and hexadecyl gallate (HG), and some of the main catechin derivatives are epicatechin (EC), epicatechin gallate (ECG), epigallocatechin (EGC), gallocatechin gallate (GCG), and epigallocatechin gallate (EGCG) (42–45). In particular, EGCG, a main bioactive compound, has been observed to have potent anticancer activities and protective effects on cardiovascular and metabolic diseases with multiple mechanisms (46–49). Owing to the properties of potent antioxidants scavenging of reactive oxygen species, several GA derivatives, such as DG, PG, OG, TG, and HG, are widely used in the food manufacturing, pharmaceutical, and cosmetic industries (43, 45, 50). The detailed classification and chemical structures of polyphenols, phenolic acids, GA and its derivatives are shown in Figure 1.
Bioavailability, Absorption, and Metabolism of GA
It has been widely claimed that polyphenols are good source of natural health products and are beneficial for human health (51–55). Oliver et al. found that polyphenols have high instability to light, heat, and pH due to the existence of multiple hydroxyl groups (56). To a great extent, these external factors affect their commercial popularization and application. In addition, the poor solubility characteristics limit their wide application in the fields of food products and supplements (57, 58). Moreover, polyphenols are quickly absorbed in the GIT, with rapid metabolism within the human gut and a high elimination rate in vivo, resulting in low and inconsistent oral bioavailability (59–61). Similarly, as a phenolic acid in polyphenols, GA and its derivatives also have the above disadvantageous properties, poor bioavailability, stability, and solubility (3, 62). Fortunately, the developing colloidal delivery systems could significantly improve its bioavailability, which brings large possibility for application in human.
The 4-O-Methygallic acid (4-OMeGA) is the primary metabolite of GA in human plasma and urine (3, 63, 64). After oral administration, nearly 70% of GA is absorbed and then excreted via urine as 4-OMeGA (65, 66). Barnes et al. identified GA metabolites (pyrogallol-1-O-glucuronide, 4-OMeGA, 4-OMeGA-3-O-sulfate, pyrogallol-O-sulfate, deoxypyrogallol-O-sulfate, and O-methylpyrogallol-O-sulfate) in the urine of healthy volunteers over a 12 h period by tandem mass spectrometry (MS/MS) analysis after the consumption of 400 g/d Keitt mango for 10 days (67). Further study indicated that after a single oral administration of Polygonum capitatum extract at 60 mg/kg (equivalent to 12 mg/kg GA), GA was distributed mainly in rat kidney tissue (1,218.62 ng/g); the lung tissue had the second highest GA content (258.08 ng/g); the concentration of GA in the liver and heart was slightly lower than that of the lung; the spleen contained very little GA; and GA could not be found in brain tissue (62). However, a study suggested that the rat brain deposition of GA increased with repeated dosing of grape seed polyphenolic extract (3). In a urinary excretion study, approximately 16.67% of the intake GA was excreted in an unchanged form, and the predominant metabolite 4-OMeGA of GA was detected in the urine sample (62). The theaflavin galloyl moiety of black tea was consumed by GM, and the released GA was further transformed to 3-O-methyl GA (3-OMeGA), 4-OMeGA, pyrogallol-1-sulfate, and pyrogallol-2-sulfate, which were excreted via urine amounts to 94% of the intake (68). Figure 2 shows the absorption, metabolism, and distribution of GA in vivo.
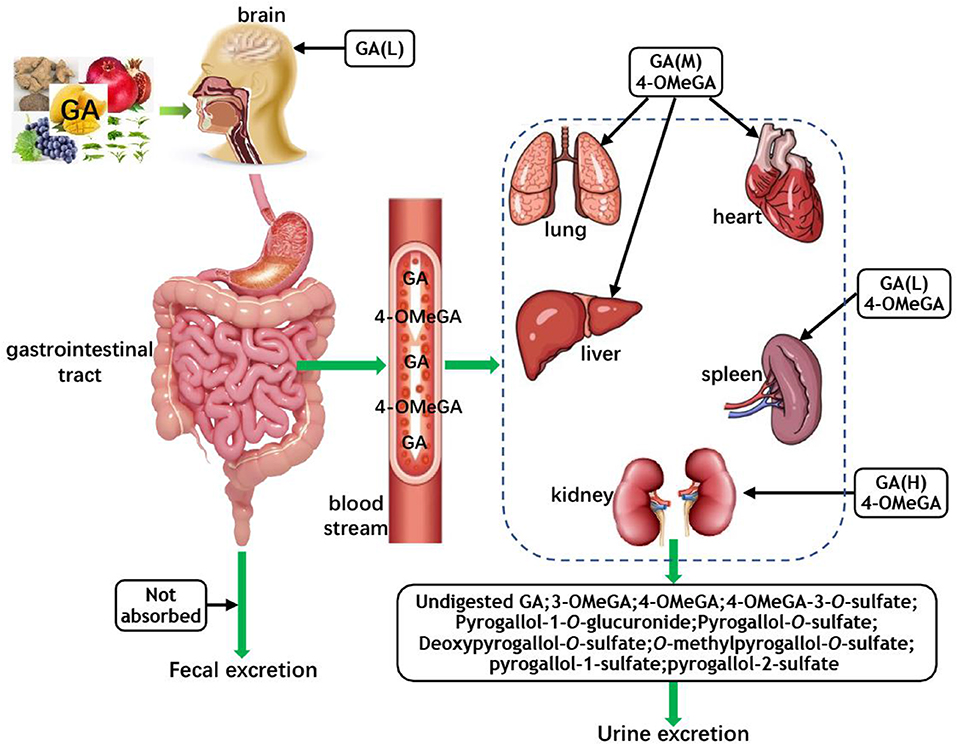
Figure 2. The absorption, metabolism, and distribution of GA. GA, gallic acid; 4-OMeGA, 4-O-methygallic acid; 3-OMeGA, 3-O-methygallic acid; H, M, and L represent the high, middle, and low content of GA in corresponding tissues and organs, respectively; the 4-OMeGA in black boxes represent that it is the primary metabolite of GA in different organs.
These research results indicate that GA undergoes extensive metabolism after digestion, but its effectiveness is limited because of rapid metabolism and elimination. How to improve the bioavailability of GA remains a problem. To overcome these challenges, colloidal delivery systems have been tested to increase the intestinal absorption of GA and subsequently enhance its bioavailability in corresponding target organs and tissues. Natural proteins, polysaccharides, and biopolymer-based delivery systems have been widely used in the research of polyphenols (69), and colloidal encapsulation could enhance the efficacy of polyphenols in the field of food and biomedical applications (70, 71). Similarly, the phospholipid complexation delivery system also shows a good effect on GA. GA–phospholipid complex improved the bioavailability of GA by increasing absorption, decreasing elimination rate, and lengthening duration of action in rat serum (72). GA liposomes decorated with lactoferrin (LF-GA-LIP) could be developed as a favorable delivery system because it displayed a delayed-release effect in simulated digestion (73). Recent studies reported that the addition of tannase could enhance GA bioaccessibility in green tea and mango juice (40, 41). Repeated dosing and the use of structural analogs or derivative compounds of GA also were effective measures to improve the plasma levels of GA (3).
However, the application of these colloidal delivery systems still has many difficulties, such as the astringent taste of GA, and the bioavailability and potential toxicity of GA complexations should be explored through in vitro and in vivo trials. A more comprehensive understanding of GA is necessary. Only then can GA complexations be used safely and reasonably as supplements and drugs in production.
GA in Gastrointestinal Health and Disease
Over the past decade, researchers have provided plenty of emerging evidence that the GM plays a crucial role in the maintenance of physiological homeostasis within the GIT, and microbiome dysbiosis is directly related to many health problems, such as gastrointestinal disease. Several studies in animal models investigate the effects of GA consumption on gastrointestinal diseases and its mechanisms of action.
Gastric Cancer
Gastric cancer (GC) is one of the main causes of cancer deaths in the world and over 1 million new cases were diagnosed in 2018 (74). Gastric microbiota plays an initial role in GC (75), and infection with Helicobacter pylori (H. pylori) is the strongest risk factor linked to GC (76). Almost all cases of GC can be related to H. pylori (77). An aqueous extract of ginger (GA and cinnamic acid) could protect the gastric mucosa against stress induced mucosal lesions by suppressing H. pylori, blocking H+, K+-ATPase action, and providing antioxidant protection (25). The study suggests that GA has potential for prevention and treatment of GC through decreasing H. pylori; however, the aqueous extract was a mixture instead of pure GA; thus, the mechanism of action is uncertain, and further research is needed.
Meanwhile, GA has potent therapeutic effects on the non-steroidal anti-inflammatory drug (NSAID)-induced gastric mucosal damage by preventing oxidative stress and inhibiting the activation of the mitochondrial pathway of apoptosis in gastric mucosal cells (78). Similarly, gastric adenocarcinoma cell metastasis was inhibited by GA, whose possible mechanism may occur through inhibitory effects on the Ras/PI3K/AKT signaling pathway and transcriptional factor NF-κB, resulting in the antimetastatic effects (79). A study verified the protective mechanism of GA and its novel derivative [(E)-3,4,5-trihydroxy-N-(2-(piperazin-1-yl) ethyl) benzimidic acid] against ethanol-induced gastric ulcerogenesis, suggesting that the gastroprotective activity may be related to antioxidant properties, immunomodulatory markers, Hsp70 and Bcl-2-associated X protein, and inhibition of mitochondrial apoptosis (80). Interestingly, the combination of GA plus famotidine exhibited a synergistic role in the protection of rat gastric mucosa (81). This study provides a possibility for GA to enhance the therapeutic effect of antibiotics.
Colorectal Cancer
Colorectal cancer (CRC) has the third highest cancer incidence around the world, and it constitutes a major global health burden threatening public health (82). The high number of studies have found that the GM plays a crucial role in colorectal carcinogenesis (83). Previous studies reported that dietary polyphenols benefit colorectal tissue integrity and function, gut bacterial growth and activities (84, 85). Although there is no direct evidence to suggest that GA prevents the occurrence of CRC by changing GM, we can indirectly speculate that GA influence the GM of CRC based on the related literatures of polyphenols and CRC.
GA and its derivative 3-OMeGA decreased human colon cancer cell viability by suppressing cell proliferation and regulating the signaling pathways of NF-κB, AP-1, STAT-1, and OCT-1 (86). Additionally, polymer nanoparticles assembled from GA-grafted chitosan (GA-g-CS) and caseinophosphopeptides (CPPs) were developed to deliver (-)-EGCG as novel functional foods. The GA-g-CS-CPP nanoparticles demonstrated powerful antioxidant activity and cytotoxicity against Caco-2 colon cancer cells, and the EGCG-loaded GA-g-CS-CPP nanoparticles further amplified the anticancer activity against Caco-2 cells (87). Similarly, GA-conjugated chitosan efficiently inhibited pulmonary metastasis of CT26 mouse colorectal carcinoma cells (88). In 1,2-dimethyl hydrazine-induced colon carcinogenesis in rats, the activity of phase II enzymes decreases, and phase I enzymes increases, whereas it is interesting to note that GA treatment could shift the above changes toward normal levels (89).
Inflammatory Bowel Disease
Inflammatory bowel disease (IBD), including Crohn's disease (CD) and ulcerative colitis (UC), has long been doubted to correlate of an abnormal host reaction to GM (90). Both diseases are chronic and inflammatory disorders in the GIT with an increasing incidence rate being related to the rapid development of industrialization (91). Patients with these disorders have greater incidence to evolve into colon cancer (92, 93). Numerous experimental and clinical studies have indicated that various dietary polyphenols have beneficial effects against IBD (94–96).
GA could inhibit inflammation in dextran sulfate sodium (DSS)-induced colitis in mice through the suppression of p65-NF-κB and IL-6/p-STAT3Y705 activation (22), and suppress lipopolysaccharide (LPS)-induced inflammation in transgenic mice by regulating immune system processes and downregulating the NF-κB pathway (97). Li et al. was the first to perform 16S gene sequencing on mice fecal and combined with metabolomics analysis; the results indicated that GA significantly attenuated UC by influencing composition of mice GM and metabolites (98). What makes us delighted is that a pilot study in patients with IBD found mango pulp (gallotannins and GA) intake markedly increased the abundance of beneficial bacteria such as Lactobacillus spp., Lactobacillus plantarum, Lactobacillus reuteri, and Lactobacillus lactis, which was accompanied by increased fecal butyric acid production (99).
However, there is only very limited evidence on the effectiveness of GA in GIT health, and very few human studies have been conducted on the impact of GA on GIT health. There is no adequate evidence to confirm the impact of GA on GIT health and disease. Further high-quality researches are needed to establish the mechanism of action of GA and its derivatives on GIT health. Several human studies have preliminarily interpreted the link between the GM and IBD. Thus, the GM could be a research direction between GA and GIT health in the future.
Effects of GA on the GM
The GM is a key modulator of human health (100, 101). Trillions of microbes living in GIT finely regulate homeostasis in GIT ecosystem, most of which are beneficial to human health, affecting maintenance of the metabolic function of the host, development of the innate and adaptive immune systems, and resistance against invasion of enteric pathogens (102). In recent years, it has become a popular research hotspot in biomedical research because researchers have identified relationships between GM compositions and health (103). The microbial diversity and homeostatic configuration of the GM are affected by various factors, and diet appears to exert the greatest influence on the GM. Dietary components are utilized by the GM to produce energy and metabolites, which can mostly enter the bloodstream to affect intestinal function and the immune system (104). As an active ingredient in dietary polyphenols, GA has potent antimicrobial properties and is beneficial to human and animal health.
Antimicrobial Properties in vitro
GA has broad-spectrum therapeutic properties including antibacteria, antifungal, and antiviral activities in vitro (Table 1). An in vitro study reported that GA suppressed viable bacteria and Escherichia coli (E. coli) biofilm formation by regulating pgaABCD gene expression (105); meanwhile, GA effectively inhibited Shigella flexneri biofilm formation and activity by regulating the expression of the mdoH gene and the OpgH protein (106), and had a specific antibiofilm effect on Staphylococcus aureus (S. aureus) by regulating the expression of the ica operon (107). Additionally, GA not only has potent anti-bacteria activity, but also against Eumycetes (36, 119). A storage test performed on fresh black truffles revealed the antimicrobial activity of GA observed in vitro, with a dramatic decline in the abundances of not only Pseudomonas spp., but also Enterobacteriaceae and Eumycetes (108), and it was observed that GA has a broad-spectrum antifungal activity for all tested dermatophyte strains (Trichophyton rubrum, Trichophyton mentagrophytes, Trichophyton violaceum, Microsporum canis, Trichophyton verrucosum, and Trichophyton schoenleinii) and Candida strains (Candida glabrata, C. albicans, and Candida tropicalis) (109). In addition, GA might be a sensitive reagent inhibiting influenza A (H1N1) virus infection (110) and has anti-HBV activity (111). Based on its powerful antimicrobial activity, GA is used to synthesize a kind of antimicrobial agent, such as trimethoprim, to treat some microbial infectious diseases (120).
The synergistic effects of natural antimicrobial compounds could increase the antimicrobial potential. A combination of GA and octyl gallate enhanced the antimicrobial activity for Enterococcus faecalis compared with the efficacy of individual compounds (112). A laccase-catalyzed chitosan–GA derivative markedly suppressed the growth of E. coli and S. aureus, and it could disrupt their cell membranes causing leakage of cytoplasm and increasing relative conductivity. Further, the cytotoxicity was notably decreased by proper modification of chitosan with GA (113). Furthermore, synthesized GA-chitosan-modified silver nanoparticles (GC-AgNps) exhibited good antibacterial activity against E. coli (114). LF-GA-LIP also exerted greater antibacterial capabilities against E. coli and S. aureus than GA-LIP (73). A GA-grafted chitin-glucan complex (GA-g-chitin-glucan complex) showed better antibacterial activity than the unmodified chitin-glucan complex (115). Shamsi et al. reported that a GA-loaded graphene oxide-based nanoformulation (GAGO) could be used as a potential antibacterial agent against S. aureus (116). The ZnO nanoparticles functionalized with GA displayed stronger antibacterial activity against methicillin-resistant S. aureus and E. coli compared with non-functionalized ZnO nanoparticles (117). Similarly, a study on chickens displayed a synergistic effect of GA and eugenol in reducing the heat lethality of Salmonella spp. (121).
GA and its derivatives (octyl gallate, propyl gallate) as well as binary combinations exhibit significant inhibition against Carnobacterium divergens ATCC 35677 and Leuconostoc carnosum ATCC 49367 originating from meat, and octyl gallate and propyl gallate were more effective than GA (17). Halogenated GA analogs might be promising drugs. Sherin et al. synthesized fifteen novel GA esters, and the most effective compound found was 3-chloropropyl 3,4,5-trihydroxybenzoate, a debenzylation of gallic acid ester, specifically against resistant gram-negative strains, such as P. aeruginosa, E. coli and E. aerogenes (118).
Such meaningful observations in vitro indicate that GA and its derivatives have antimicrobial activities, which can be strengthened by a favorable delivery system. However, in vitro studies raise a question of whether GA exerts healthy effects by changing the GM composition in vivo. Thus, studies in vivo in animals and humans need to be carried out.
Action of GA on the GM in Animals and Humans
Most plant-derived polyphenols must be transformed through the GM and intestinal enterocyte enzymes to be absorbed at enterocyte and colonocyte levels. The GM could transform polyphenols to final bioactive derivatives exhibiting antimicrobial properties. Therefore, an appropriate GM is extremely important for fighting against infectious diseases (122). Similarly, metabolism of GA in the GIT also requires the participation of the GM and intestinal enterocyte enzymes. As described in Figure 3 by Pereira-Caro et al. the principle pathways for GA in the colonic microbiota and mammalian phase II metabolism are proposed (68). The effect between the GM and GA is mutual; intestinal bacteria has the ability to metabolize GA, and GA also can induce changes in the microbiota toward a more favorable composition and activity, including the production of short-chain fatty acids (SCFAs) in the colon (22).
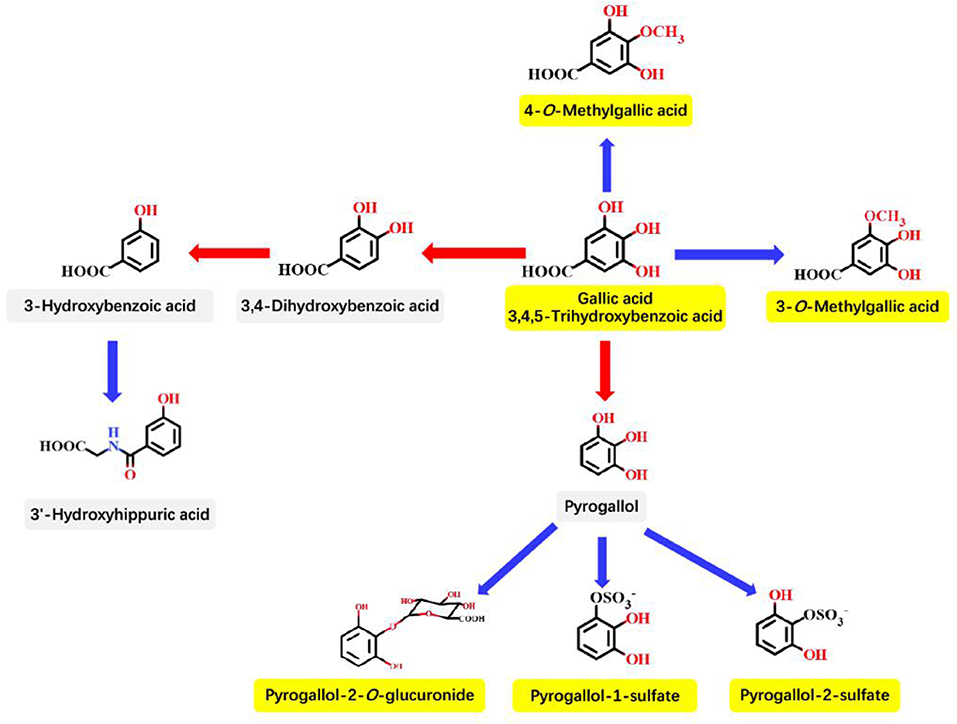
Figure 3. Proposed principle pathways for GA in the colonic microbiota and mammalian phase II metabolism. Red arrows indicate microbiota-mediated steps, and blue arrows represent mammalian enzyme-mediated conversions. The names in yellow boxes indicate the main metabolites accumulating in urine after GA intake. This figure is quoted from Pereira-Caro et al. (68).
From the GM point of view, A recent study of animal model on the attenuation of DSS-induced rat UC by GA showed that GA dramatically decreased the GM abundance but had little impact on diversity. Changes in microbiota induced by DSS were characterized by a decrease in some probiotics predominantly in the Lactobacillaceae and Prevotellaceae families, and an increase in some pathogenic bacteria dominated by the Firmicutes and Proteobacteria phyla; GA reversed the above-mentioned changes and make them similar to the control group (22). Because fecal metabolites are byproducts of the interplay between the host and microbiota, changes in metabolites in vivo can act as evidence for alteration of the microbiota. Detection of the fecal SCFAs by gas chromatography concluded that the SCFAs contents were higher in the control group than GA and DSS group. Analysis of nuclear magnetic resonance-identified metabolites further revealed GA-induced metabolites changes mainly in increasing carbohydrate metabolism and bile acid metabolism and decreasing amino acid metabolism (22). All of above results demonstrate that GA-induced alterations in metabolites and GM in DSS-colitis provide new insight into the attenuation of UC by GA. Metabolomics data of rat plasma, liver, urine, and feces were analyzed by nuclear magnetic resonance whose results showed that changes in metabolites correlates to GA intake, and GA effectively promoted glycogenolysis, glycolysis, and TCA cycle and had positive effects on the metabolism of nucleotides, choline, bile acids, and amino acids (123). Fecal propionate and butyrate are fermentation products of insoluble polysaccharides and proteins (124). Remarkable increases in the levels of fecal propionate and butyrate and decreases in the levels of pyruvate, 2-ketoglutarate, lysine, alanine, and keto-acids suggested that GA could promote the GM fermentation of both proteins and polysaccharides. Research evidence suggests that GA has a great potential to be a natural antifungal agent for clinical application. A study in mice proved that intraperitoneal injection of GA markedly improved the rate of curability in a mouse model of systemic fungal infection (109).
In summary, available results in vitro and limited animal researches in vivo show GA can positively affect the composition of the GM or suppress the growth of pathogenic bacteria. However, it is a great pity that studies on the effects of GA and its derivatives on the human GM are lacking. The analysis of metabolites levels in human feces, urine, and blood combined with metagenomic analysis could offer a in-depth understanding of the impact of GA on humans.
GA in Immunomodulation
The gut is an immune organ in which more than half of all immune cells are concentrated. The gut immune system linked to obesity, diabetes, food allergies, and IBD (125), thus, the gut immune function is closely related to human health. Various factors affect the development of the gut immune system, especially the GM and antigens, and they can drive the maintenance of gut barrier function and the development of the mucosal immune system (126). The mucus layer serves as the first protective barrier of the gut composed of an outer, loosely adhered layer and an inner, denser layer adhered to the underlying epithelium; the outer mucus layer is generally related to the GM (127). Immune dysfunction in the intestinal mucosa increases the risk of diarrhea in the host and has a negative impact on the balance of the GM (128), which could result in many serious consequences. Many studies have confirmed the regulatory role of plant-derived polyphenols in gut immune function (129, 130), thus fruits and vegetables rich in polyphenols are considered to be a preventive agent to promote intestinal health via modulating the intestinal mucosal immune response (127, 131).
The majority of immune-related disorders, such as pathogen-mediated infectious diseases, allergic diseases, and cancers, linked to inflammation (132). In the allograft model, GA accelerated the differentiation of T cells, increased the number of Tregs and exerted an anti-inflammatory effect, so GA has potential to treat diseases caused by excessive activation of immune cells (20). GA could decrease the exacerbated response of the body against an infectious agent to enhance innate immune activation by reducing the anti-apoptotic role of LPS, blocking the induction of neutrophil extracellular traps and preventing the formation of free radicals induced by LPS (133).
GA exhibited a protective effect against oxidative stress-induced cellular injury in human lymphocytes through immunomodulatory, antioxidant, and cytoprotective properties (134) and provided effective prevention against complications relating to immunological and thrombo-regulatory mechanisms via reverting the ATP and ADP hydrolysis and adenosine deaminase activity in lymphocytes, and preventing the increase in nucleoside triphosphate diphosphohydrolase, and adenosine deaminase activities in platelets (135). Additionally, GA inhibited the production of reactive oxygen species and nitric oxide, proinflammatory cytokine release, and phagocytes-induced lymphocyte proliferation in human peripheral blood mononuclear cells (136). The synergistic effect of GA and asparaginase also improved the antiproliferative effect on lymphoblastic cells (137).
GA could improve immunomodulatory activity by increasing of phagocytic capability, lysosomal volume, nitrite release, and intracellular calcium () levels in macrophages (138) and downregulate the MAPK-linked phagocytic signaling pathway in mouse murine macrophages (139). The efflux transporters P-glycoprotein and multidrug resistance proteins might participate in the transport of GA, and paracellular transport appeared to be the major limiting factor for the uptake of GA in Caco-2 cell monolayers (140). Polysaccharide nanofibers improved GA and EGCG permeability by opening the tight junctions of human differentiated epithelial Caco-2 cell monolayers and inhibiting efflux transporters (141). Cotreatment with curcumin and GA normalized the circulatory pro-inflammatory, anti-inflammatory cytokines, chemokines, N-εCML, CRP, and HbA1c (142). In addition, The GA derivatives (G-4, G-7, G-9, G-10, G-12, and G-13) also exhibited immunomodulatory activity and had high binding affinities for the INFα-2, IL-6, and IL-4 receptors, among which G-7 has the greatest immunomodulatory activity (143).
The possible mechanism of action of GA on the remission of immune-related diseases is summarized in Figure 4.
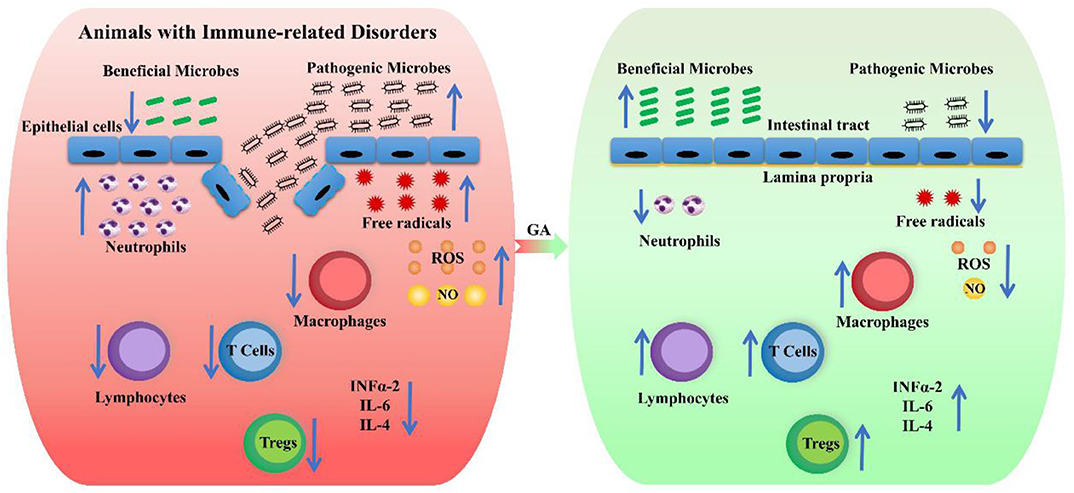
Figure 4. The possible mechanism of action of GA on the remission of immune-related diseases. The red box represents animals with immune-related disorders, and the green box indicates the attenuation effect of GA on immune-related disorders. The up arrows indicate a rising trend, and the down arrows show a declining trend.
The immunomodulatory activities of GA have not been deeply studied, and researchers should conduct a large number of experiments and preclinical studies on the immunomodulatory potential of GA to provide enough evidence to confirm the effectiveness and safety of GA. Furthermore, patients with immune-related diseases should be chosen as the research objects so that GA is further developed as a therapeutic agent for immune-related disorders.
Conclusion and Outlook
This review summarizes the physicochemical properties and bioavailability of GA, and reports related to the impact of GA on gastrointestinal health focus mainly on GM, immunomodulation and mechanisms of action. According to these existing studies, GA and its derivatives have the potential to be novel agents for the treatment and prevention of gastrointestinal diseases through interaction with the GM and modulation of the immune response. Current in vitro evidence and results in animal models confirm the pharmacological and therapeutic interventions of GA. However, there is very limited clinical evidence for the effectiveness of GA in human gastrointestinal health and disease, and the exact underlying mechanisms of action are still obscure and unexplored. To clarify the interactions among the GM, immune response, and gastrointestinal disease in humans upon GA intervention, further investigation in other animal models and in humans is needed to verify the previous findings from animal models. Additionally, more efficient GA delivery systems need to be developed to improve GA bioavailability. With the rapid development of omics techniques, it is necessary and important to integrate genomics, transcriptomics, proteomics, and metabolomics to phenotyping to explore the molecular effects of GA in order to clarify its underlying mechanism of action.
Author Contributions
KY generated ideas and wrote the initial manuscript. YY and BD guided and revised the manuscript. CT and JD made feasible suggestions for the manuscript. LZ, PL, ZXia, FZ, DS, and ZXin contributed to the collection and arrangement of literatures. All authors contributed to the article and approved the submitted version.
Funding
This project was supported by National Key R&D Program of China (Grant No. 2018YFD0500600), National Natural Science Foundation of China (Grant No. 31790411), Natural Science Foundation of Guangdong Province (Grant No. 2020A1515010322), Innovation Team Project in Universities of Guangdong Province (2017KCXTD002), Guangdong Basic and Applied Basic Research Foundation (2019B1515210002) and Guangdong Provincial Promotion Project on Preservation and Utilization of Local Breed of Livestock and Poultry.
Conflict of Interest
The authors declare that the research was conducted in the absence of any commercial or financial relationships that could be construed as a potential conflict of interest.
References
1. National Center for Biotechnology Information. PubChem Database. Gallic Acid, CID=370. (2020). Available online at: https://pubchem.ncbi.nlm.nih.gov/compound/Gallic-acid (accessed on Oct. 21, 2019).
2. Cedó L, Castell-Auví A, Pallarès V, Macià A, Blay M, Ardévol A, et al. Gallic acid is an active component for the anticarcinogenic action of grape seed procyanidins in pancreatic cancer cells. Nutr Cancer. (2014) 66:88–96. doi: 10.1080/01635581.2014.851714
3. Ferruzzi MG, Lobo JK, Janle EM, Cooper B, Simon JE, Wu QL, et al. Bioavailability of gallic acid and catechins from grape seed polyphenol extract is improved by repeated dosing in rats: implications for treatment in alzheimer's disease. J Alzheimers Dis. (2009) 18:113–24. doi: 10.3233/JAD-2009-1135
4. Scoccia J, Perretti MD, Monzón DM, Crisóstomo FP, Martín VS, Carrillo R. Sustainable oxidations with air mediated by gallic acid: potential applicability in the reutilization of grape pomace. Green Chem. (2016) 18:2647–50. doi: 10.1039/C5GC02966J
5. Liu S, Li S, Lin G, Markkinen N, Yang H, Zhu B, et al. Anthocyanin copigmentation and color attributes of bog bilberry syrup wine during bottle aging: effect of tannic acid and gallic acid extracted from Chinese gallnut. J Food Process Pres. (2019) 43:e14041. doi: 10.1111/jfpp.14041
6. Wang C, Li W. Optimization technology of the LHS-1 strain for degrading gallnut water extract and appraisal of benzene ring derivatives from fermented gallnut water extract pyrolysis by Py-GC/MS. Molecules. (2017) 22:2253. doi: 10.3390/molecules22122253
7. Zhang J, Li B, Yue H, Wang J, Zheng Y. Highly selective and efficient imprinted polymers based on carboxyl-functionalized magnetic nanoparticles for the extraction of gallic acid from pomegranate rind. J Sep Sci. (2018) 41:540–7. doi: 10.1002/jssc.201700822
8. Singh M, Jha A, Kumar A, Hettiarachchy N, Rai AK, Sharma D. Influence of the solvents on the extraction of major phenolic compounds (punicalagin, ellagic acid and gallic acid) and their antioxidant activities in pomegranate aril. J Food Sci Tech. (2014) 51:2070–7. doi: 10.1007/s13197-014-1267-0
9. Jiang H, Yu F, Qin L, Zhang N, Cao Q, Schwab W, et al. Dynamic change in amino acids, catechins, alkaloids, and gallic acid in six types of tea processed from the same batch of fresh tea (Camellia sinensis L.) leaves. J Food Compos Anal. (2019) 77:28–38. doi: 10.1016/j.jfca.2019.01.005
10. Ye Y, Yan J, Cui J, Mao S, Li M, Liao X, et al. Dynamic changes in amino acids, catechins, caffeine and gallic acid in green tea during withering. J Food Compos Anal. (2018) 66:98–108. doi: 10.1016/j.jfca.2017.12.008
11. Fischer E. Synthesis of depsides, lichen-substances and tannins. J Am Chem Soc. (1914) 36:1170–201. doi: 10.1021/ja02183a009
12. Alfei S, Oliveri P, Malegori C. Assessment of the efficiency of a nanospherical gallic acid dendrimer for long-term preservation of essential oils: an integrated chemometric-assisted FTIR study. Chemistryselect. (2019) 4:8891–901. doi: 10.1002/slct.201902339
13. Fereidoonfar H, Salehi-Arjmand H, Khadivi A, Akramian M, Safdari L. Chemical variation and antioxidant capacity of sumac (Rhus coriaria L.). Ind Crop Prod. (2019) 139:111518. doi: 10.1016/j.indcrop.2019.111518
14. Phonsatta N, Deetae P, Luangpituksa P, Grajeda-Iglesias C, Figueroa-Espinoza MC, Le Comte J, et al. Comparison of antioxidant evaluation assays for investigating antioxidative activity of gallic acid and its alkyl esters in different food matrices. J Agr Food Chem. (2017) 65:7509–18. doi: 10.1021/acs.jafc.7b02503
15. Wang Y, Xie M, Ma G, Fang Y, Yang W, Ma N, et al. The antioxidant and antimicrobial activities of different phenolic acids grafted onto chitosan. Carbohyd Polym. (2019) 225:115–238. doi: 10.1016/j.carbpol.2019.115238
16. Wang Q, Leong WF, Elias RJ, Tikekar RV. UV-C irradiated gallic acid exhibits enhanced antimicrobial activity via generation of reactive oxidative species and quinone. Food Chem. (2019) 287:303–12. doi: 10.1016/j.foodchem.2019.02.041
17. Del Valle P, Rosario Garcia-Armesto M, Campos J, Posado-Fernandez A, De Arriaga D, Rua J. Antimicrobial effects of gallic acid, octyl gallate and propyl gallate on Carnobacterium divergens and Leuconostoc carnosum originating from meat. J Food Nutr Res. (2018) 57:76–86.
18. Zhang T, Ma L, Wu P, Li W, Li T, Gu R, et al. Gallic acid has anticancer activity and enhances the anticancer effects of cisplatin in non-small cell lung cancer A549 cells via the JAK/STAT3 signaling pathway. Oncol Rep. (2019) 41:1779–88. doi: 10.3892/or.2019.6976
19. Rosman R, Saifullah B, Maniam S, Dorniani D, Hussein MZ, Fakurazi S. Improved anticancer effect of magnetite nanocomposite formulation of gallic acid (Fe3O4-PEG-GA) against lung, breast and colon cancer cells. Nanomaterials. (2018) 8:83. doi: 10.3390/nano8020083
20. Hyun KH, Gil KC, Kim SG, Park S, Hwang KW. Delphinidin chloride and its hydrolytic metabolite gallic acid promote differentiation of regulatory T cells and have an anti-inflammatory effect on the allograft model. J Food Sci. (2019) 84:920–30. doi: 10.1111/1750-3841.14490
21. BenSaad LA, Kim KH, Quah CC, Kim WR, Shahimi M. Anti-inflammatory potential of ellagic acid, gallic acid and punicalagin A&B isolated from Punica granatum. BMC Complem Altern Med. (2017) 17:47. doi: 10.1186/s12906-017-1555-0
22. Pandurangan AK, Mohebali N, Esa NM, Looi CY, Ismail S, Saadatdoust Z. Gallic acid suppresses inflammation in dextran sodium sulfate-induced colitis in mice: possible mechanisms. Int Immunopharmacol. (2015) 28:1034–43. doi: 10.1016/j.intimp.2015.08.019
23. Pandurangan AK, Mohebali N, Norhaizan ME, Looi CY. Gallic acid attenuates dextran sulfate sodium-induced experimental colitis in BALB/c mice. Drug Des Dev Ther. (2015) 9:3923–34. doi: 10.2147/DDDT.S86345
24. Dhingra MS, Dhingra S, Chadha R, Singh T, Karan M. Design, synthesis, physicochemical, and pharmacological evaluation of gallic acid esters as non-ulcerogenic and gastroprotective anti-inflammatory agents. Med Chem Res. (2014) 23:4771–88. doi: 10.1007/s00044-014-1041-x
25. Nanjundaiah SM, Annaiah HNM, Dharmesh SM. Gastroprotective effect of ginger rhizome (zingiber officinale) extract: role of gallic acid and cinnamic acid in H+, K+-ATPase/H. pylori inhibition and anti-oxidative mechanism. Evid-Based Complalt Alternat Med. (2011) 2011:24987. doi: 10.1093/ecam/nep060
26. El-Hussainy EMA, Hussein AM, Abdel-Aziz A, El-Mehasseb I. Effects of aluminum oxide (Al2O3) nanoparticles on ECG, myocardial inflammatory cytokines, redox state, and connexin 43 and lipid profile in rats: possible cardioprotective effect of gallic acid. Can J Physiol Pharm. (2016) 94:868–78. doi: 10.1139/cjpp-2015-0446
27. Priscilla DH, Prince PSM. Cardioprotective effect of gallic acid on cardiac troponin-T, cardiac marker enzymes, lipid peroxidation products and antioxidants in experimentally induced myocardial infarction in wistar rats. Chem Biol Interact. (2009) 179:118–24. doi: 10.1016/j.cbi.2008.12.012
28. Maya S, Prakash T, Madhu K. Assessment of neuroprotective effects of gallic acid against glutamate-induced neurotoxicity in primary rat cortex neuronal culture. Neurochem Int. (2018) 121:50–8. doi: 10.1016/j.neuint.2018.10.011
29. Sun J, Ren D, Wan J, Chen C, Chen D, Yang H, et al. Desensitizing mitochondrial permeability transition by ERK-cyclophilin D axis contributes to the neuroprotective effect of gallic acid against cerebral ischemia/reperfusion injury. Front Pharmacol. (2017) 8:184. doi: 10.3389/fphar.2017.00184
30. Sun J, Li Y, Ding Y, Wang J, Geng J, Yang H, et al. Neuroprotective effects of gallic acid against hypoxia/reoxygenation-induced mitochondrial dysfunctions in vitro and cerebral ischemia/reperfusion injury in vivo. Brain Res. (2014) 1589:126–39. doi: 10.1016/j.brainres.2014.09.039
31. Bak E, Kim J, Jang S, Woo G, Yoon H, Yoo Y, et al. Gallic acid improves glucose tolerance and triglyceride concentration in diet-induced obesity mice. Scand J Clin Lab Inv. (2013) 73:607–14. doi: 10.3109/00365513.2013.831470
32. Abdel-Moneim A, Abd El-Twab SM, Yousef AI, Reheim ESA, Ashour MB. Modulation of hyperglycemia and dyslipidemia in experimental type 2 diabetes by gallic acid and p-coumaric acid: the role of adipocytokines and PPAR gamma. Biomed Pharmacother. (2018) 105:1091–7. doi: 10.1016/j.biopha.2018.06.096
33. Huang D, Chang W, Wu JS, Shih R, Shen S. Gallic acid ameliorates hyperglycemia and improves hepatic carbohydrate metabolism in rats fed a high-fructose diet. Nutr Res. (2016) 36:150–60. doi: 10.1016/j.nutres.2015.10.001
34. Tsao R. Chemistry and biochemistry of dietary polyphenols. Nutrients. (2010) 2:1231–46. doi: 10.3390/nu2121231
35. Durazzo A, Lucarini M, Souto EB, Cicala C, Caiazzo E, Izzo AA, et al. Polyphenols: a concise overview on the chemistry, occurrence, and human health. Phytother Res. (2019) 33:2221–43. doi: 10.1002/ptr.6419
36. Borges A, Ferreira C, Saavedra MJ, Simões M. Antibacterial activity and mode of action of ferulic and gallic acids against pathogenic bacteria. Microb Drug Resist. (2013) 19:256–65. doi: 10.1089/mdr.2012.0244
37. Khan BA, Mahmood T, Menaa F, Shahzad Y, Yousaf AM, Hussain T, et al. New perspectives on the efficacy of gallic acid in cosmetics & nanocosmeceuticals. Curr Pharm Design. (2018) 24:5181–7. doi: 10.2174/1381612825666190118150614
38. Wang J, Shen X, Yuan Q, Yan Y. Microbial synthesis of pyrogallol using genetically engineered Escherichia coli. Metab Eng. (2018) 45:134–41. doi: 10.1016/j.ymben.2017.12.006
39. Cai Y, Zhang J, Chen NG, Shi Z, Qiu J, He C, et al. Recent advances in anticancer activities and drug delivery systems of tannins. Med Res Rev. (2017) 37:665–701. doi: 10.1002/med.21422
40. Sirven MA, Negrete M, Talcott ST. Tannase improves gallic acid bioaccessibility and maintains the quality of mango juice. Int J Food Sci Tech. (2019) 54:1523–9. doi: 10.1111/ijfs.13929
41. Xu X, Meng J, Mao Q, Shang A, Li B, Zhao C, et al. Effects of tannase and ultrasound treatment on the bioactive compounds and antioxidant activity of green tea extract. Antioxidants. (2019) 8:362. doi: 10.3390/antiox8090362
42. Kim H, Quon MJ, Kim J. New insights into the mechanisms of polyphenols beyond antioxidant properties; lessons from the green tea polyphenol, epigallocatechin 3-gallate. Redox Biol. (2014) 2:187–95. doi: 10.1016/j.redox.2013.12.022
43. Choubey S, Varughese LR, Kumar V, Beniwal V. Medicinal importance of gallic acid and its ester derivatives: a patent review. Pharm Pat Anal. (2015) 4:305–15. doi: 10.4155/ppa.15.14
44. Peluso I, Serafini M. Antioxidants from black and green tea: from dietary modulation of oxidative stress to pharmacological mechanisms. Brit J Pharmacol. (2017) 174:1195–208. doi: 10.1111/bph.13649
45. Locatelli C, Filippin-Monteiro FB, Creczynski-Pasa TB. Alkyl esters of gallic acid as anticancer agents: a review. Eur J Med Chem. (2013) 60:233–9. doi: 10.1016/j.ejmech.2012.10.056
46. Gan R, Li H, Sui Z, Corke H. Absorption, metabolism, anti-cancer effect and molecular targets of epigallocatechin gallate (EGCG): an updated review. Crit Rev Food Sci. (2018) 58:924–41. doi: 10.1080/10408398.2016.1231168
47. Eng QY, Thanikachalam PV, Ramamurthy S. Molecular understanding of epigallocatechin gallate (EGCG) in cardiovascular and metabolic diseases. J Ethnopharmacol. (2018) 210:296–310. doi: 10.1016/j.jep.2017.08.035
48. Fujiki H, Watanabe T, Sueoka E, Rawangkan A, Suganuma M. Cancer prevention with green tea and its principal constituent, EGCG: from early investigations to current focus on human cancer stem cells. Mol Cells. (2018) 41:73–82. doi: 10.14348/molcells.2018.2227
49. Negri A, Naponelli V, Rizzi F, Bettuzzi S. Molecular targets of epigallocatechingallate (EGCG): a special focus on signal transduction and cancer. Nutrients. (2018) 10:1936. doi: 10.3390/nu10121936
50. Savi LA, Leal PC, Vieira TO, Rosso R, Nunes RJ, Yunes RA, et al. Evaluation of anti-herpetic and antioxidant activities, and cytotoxic and genotoxic effects of synthetic alkyl-esters of gallic acid. Arzneimittel Forschung. (2005) 55:66–75. doi: 10.1055/s-0031-1296825
51. Rahimifard M, Maqbool F, Moeini-Nodeh S, Niaz K, Abdollahi M, Braidy N, et al. Targeting the TLR4 signaling pathway by polyphenols: a novel therapeutic strategy for neuroinflammation. Ageing Res Rev. (2017) 36:11–9. doi: 10.1016/j.arr.2017.02.004
52. Costa C, Tsatsakis A, Mamoulakis C, Teodoro M, Briguglio G, Caruso E, et al. Current evidence on the effect of dietary polyphenols intake on chronic diseases. Food Chem Toxicol. (2017) 110:286–99. doi: 10.1016/j.fct.2017.10.023
53. Yahfoufi N, Alsadi N, Jambi M, Matar C. The immunomodulatory and anti-inflammatory role of polyphenols. Nutrients. (2018) 10:1618. doi: 10.3390/nu10111618
54. Kawabata K, Yoshioka Y, Terao J. Role of intestinal microbiota in the bioavailability and physiological functions of dietary polyphenols. Molecules. (2019) 24:370. doi: 10.3390/molecules24020370
55. Fraga CG, Croft KD, Kennedy DO, Tomas-Barberan FA. The effects of polyphenols and other bioactives on human health. Food Funct. (2019) 10:514–28. doi: 10.1039/C8FO01997E
56. Oliver S, Vittorio O, Cirillo G, Boyer C. Enhancing the therapeutic effects of polyphenols with macromolecules. Polym Chem. (2016) 7:1529–44. doi: 10.1039/C5PY01912E
57. Ariyarathna IR, Karunaratne DN. Microencapsulation stabilizes curcumin for efficient delivery in food applications. Food Packaging Shelf. (2016) 10:79–86. doi: 10.1016/j.fpsl.2016.10.005
58. Rodriguez J, Martin MJ, Ruiz MA, Clares B. Current encapsulation strategies for bioactive oils: from alimentary to pharmaceutical perspectives. Food Res Int. (2016) 83:41–59. doi: 10.1016/j.foodres.2016.01.032
59. Fernandes I, Perez-Gregorio R, Soares S, Mateus N, de Freitas V. Wine flavonoids in health and disease prevention. Molecules. (2017) 22:292. doi: 10.3390/molecules22020292
60. Laddomada B, Caretto S, Mita G. Wheat bran phenolic acids: bioavailability and stability in whole wheat-based foods. Molecules. (2015) 20:15666–85. doi: 10.3390/molecules200915666
61. Crozier A, Jaganath IB, Clifford MN. Dietary phenolics: chemistry, bioavailability and effects on health. Nat Prod Rep. (2009) 26:1001–43. doi: 10.1039/b802662a
62. Ma F, Deng Q, Zhou X, Gong X, Zhao Y, Chen H, et al. The tissue distribution and urinary excretion study of gallic acid and protocatechuic acid after oral administration of polygonum capitatum extract in rats. Molecules. (2016) 21:399. doi: 10.3390/molecules21040399
63. Abd El Mohsen MM, Kuhnle G, Rechner AR, Schroeter H, Rose S, Jenner P, et al. Uptake and metabolism of epicatechin and its access to the brain after oral ingestion. Free Radical Bio Med. (2002) 33:1693–702. doi: 10.1016/S0891-5849(02)01137-1
64. Shahrzad S, Bitsch I. Determination of gallic acid and its metabolites in human plasma and urine by high-performance liquid chromatography. J Chromatogr B Biomed Sci Appl. (1998) 705:87–95. doi: 10.1016/S0378-4347(97)00487-8
65. Konishi Y, Hitomi Y, Yoshioka E. Intestinal absorption of p-coumaric and gallic acids in rats after oral administration. J Agr Food Chem. (2004) 52:2527–32. doi: 10.1021/jf035366k
66. Daglia M, Di Lorenzo A, Nabavi SF, Talas ZS, Nabavi SM. Polyphenols: well beyond the antioxidant capacity: gallic acid and related compounds as neuroprotective agents: you are what you eat! Curr Pharm Biotechnol. (2014) 15:362–72. doi: 10.2174/138920101504140825120737
67. Barnes RC, Krenek KA, Meibohm B, Mertens Talcott SU, Talcott ST. Urinary metabolites from mango (Mangifera indica L. cv. Keitt) galloyl derivatives and in vitro hydrolysis of gallotannins in physiological conditions. Mol Nutr Food Res. (2016) 60:542–50. doi: 10.1002/mnfr.201500706
68. Pereira-Caro G, Moreno-Rojas JM, Brindani N, Del Rio D, Lean MEJ, Hara Y, et al. Bioavailability of black tea theaflavins: absorption, metabolism, and colonic catabolism. J Agr Food Chem. (2017) 65:5365–74. doi: 10.1021/acs.jafc.7b01707
69. Zhang L, McClements DJ, Wei Z, Wang G, Liu X, Liu F. Delivery of synergistic polyphenol combinations using biopolymer-based systems: advances in physicochemical properties, stability and bioavailability. Crit Rev Food Sci Nutr. (2019) 60:2083–97. doi: 10.1080/10408398.2019.1630358
70. Cirillo G, Curcio M, Vittorio O, Iemma F, Restuccia D, Spizzirri UG, et al. Polyphenol conjugates and human health: a perspective review. Crit Rev Food Sci. (2016) 56:326–37. doi: 10.1080/10408398.2012.752342
71. Etxabide A, Garrido T, Uranga J, Guerrero P, de la Caba K. Extraction and incorporation of bioactives into protein formulations for food and biomedical applications. Int J Biol Macromol. (2018) 120:2094–105. doi: 10.1016/j.ijbiomac.2018.09.030
72. Bhattacharyya S, Ahammed SM, Saha BP, Mukherjee PK. The gallic acid–phospholipid complex improved the antioxidant potential of gallic acid by enhancing its bioavailability. Aaps Pharmscitech. (2013) 14:1025–33. doi: 10.1208/s12249-013-9991-8
73. Zhang Y, Pu C, Tang W, Wang S, Sun Q. Gallic acid liposomes decorated with lactoferrin: characterization, in vitro digestion and antibacterial activity. Food Chem. (2019) 293:315–22. doi: 10.1016/j.foodchem.2019.04.116
74. Rawla P, Barsouk A. Epidemiology of gastric cancer: global trends, risk factors and prevention. Prz Gastroenterol. (2019) 14:26–38. doi: 10.5114/pg.2018.80001
75. Stewart OA, Wu F, Chen Y. The role of gastric microbiota in gastric cancer. Gut Microbes. (2020) 11:1220–30. doi: 10.1080/19490976.2020.1762520
76. Wroblewski LE, Peek RM Jr, Wilson KT. Helicobacter pylori and gastric cancer: factors that modulate disease risk. Clin Microbiol Rev. (2010) 23:713–39. doi: 10.1128/CMR.00011-10
77. Chen Y, Segers S, Blaser MJ. Association between helicobacter pylori and mortality in the NHANES III study. Gut. (2013) 62:1262–9. doi: 10.1136/gutjnl-2012-303018
78. Pal C, Bindu S, Dey S, Alam A, Goyal M, Iqbal MS, et al. Gallic acid prevents nonsteroidal anti-inflammatory drug-induced gastropathy in rat by blocking oxidative stress and apoptosis. Free Radical Bio Med. (2010) 49:258–67. doi: 10.1016/j.freeradbiomed.2010.04.013
79. Ho H, Chang C, Ho W, Liao S, Wu C, Wang C. Anti-metastasis effects of gallic acid on gastric cancer cells involves inhibition of NF-kappa B activity and downregulation of PI3K/AKT/small GTPase signals. Food Chem Toxicol. (2010) 48:2508–16. doi: 10.1016/j.fct.2010.06.024
80. Abdelwahab SI. Protective mechanism of gallic acid and its novel derivative against ethanol-induced gastric ulcerogenesis: involvement of immunomodulation markers, Hsp70 and Bcl-2-associated X protein. Int Immunopharmacol. (2013) 16:296–305. doi: 10.1016/j.intimp.2013.04.005
81. Asokkumar K, Sen S, Umamaheswari M, Sivashanmugam AT, Subhadradevi V. Synergistic effect of the combination of gallic acid and famotidine in protection of rat gastric mucosa. Pharmacol Rep. (2014) 66:594–9. doi: 10.1016/j.pharep.2014.01.006
82. Siegel R, DeSantis C, Jemal A. Colorectal cancer statistics, 2014. CA Cancer J Clin. (2014) 64:104–17. doi: 10.3322/caac.21220
83. Keku TO, Dulal S, Deveaux A, Jovov B, Han X. The gastrointestinal microbiota and colorectal cancer. Am J Physiol Gastr Liver Physiol. (2015) 308:G351–63. doi: 10.1152/ajpgi.00360.2012
84. Yang R, Shan S, Zhang C, Shi J, Li H, Li Z. Inhibitory effects of bound polyphenol from foxtail millet bran on colitis-associated carcinogenesis by the restoration of gut microbiota in a mice model. J Agr Food Chem. (2020) 68:3506–17. doi: 10.1021/acs.jafc.0c00370
85. Araujo JR, Goncalves P, Martel F. Chemopreventive effect of dietary polyphenols in colorectal cancer cell lines. Nutr Res. (2011) 31:77–87. doi: 10.1016/j.nutres.2011.01.006
86. Forester SC, Choy YY, Waterhouse AL, Oteiza PI. The anthocyanin metabolites gallic acid, 3-O-methylgallic acid, and 2,4,6-trihydroxybenzaldehyde decrease human colon cancer cell viability by regulating pro-oncogenic signals. Mol Carcinogen. (2014) 53:432–9. doi: 10.1002/mc.21974
87. Hu B, Wang Y, Xie M, Hu G, Ma F, Zeng X. Polymer nanoparticles composed with gallic acid grafted chitosan and bioactive peptides combined antioxidant, anticancer activities and improved delivery property for labile polyphenols. J Funct Foods. (2015) 15:593–603. doi: 10.1016/j.jff.2015.04.009
88. Lee HL, Choi CW, Kim J, Cha B, Nah JW, Hong JS, et al. Antimetastatic activity of gallic acid-conjugated chitosan against pulmonary metastasis of colon carcinoma cells. Bull Korean Chem Soc. (2018) 39:90–6. doi: 10.1002/bkcs.11351
89. Senapathy JG, Jayanthi S, Viswanathan P, Umadeyi P, Nalini N. Effect of gallic acid on xenobiotic metabolizing enzymes in 1,2-dimethyl hydrazine induced colon carcinogenesis in wistar rats-A chemopreventive approach. Food Chem Toxicol. (2011) 49:887–92. doi: 10.1016/j.fct.2010.12.012
90. Sekirov I, Russell SL, Antunes LCM, Finlay BB. Gut microbiota in health and disease. Physiol Rev. (2010) 90:859–904. doi: 10.1152/physrev.00045.2009
91. Ng SC, Bernstein CN, Vatn MH, Lakatos PL Jr, Loftus EV, Tysk C, et al. Geographical variability and environmental risk factors in inflammatory bowel disease. Gut. (2013) 62:630–49. doi: 10.1136/gutjnl-2012-303661
92. Muller M, Hansmannel F, Arnone D, Choukour M, Ndiaye NC, Kokten T, et al. Genomic and molecular alterations in human inflammatory bowel disease-associated colorectal cancer. United Eur Gastroenterol J. (2020) 8:675–84. doi: 10.1177/2050640620919254
93. Ocansey DKW, Qiu W, Wang J, Yan Y, Qian H, Zhang X, et al. The achievements and challenges of mesenchymal stem cell-based therapy in inflammatory bowel disease and its associated colorectal cancer. Stem Cells Int. (2020) 2020:7819824. doi: 10.1155/2020/7819824
94. Rahman SU, Li Y, Huang Y, Zhu L, Feng S, Wu J, et al. Treatment of inflammatory bowel disease via green tea polyphenols: possible application and protective approaches. Inflammopharmacology. (2018) 26:319–30. doi: 10.1007/s10787-018-0462-4
95. Wang K, Jin X, Li Q, Sawaya ACHF, Le Leu RK, Conlon MA, et al. Propolis from different geographic origins decreases intestinal inflammation and Bacteroides spp. populations in a model of DSS-induced colitis. Mol Nutr Food Res. (2018) 62:1800080. doi: 10.1002/mnfr.201800080
96. Denis MC, Roy D, Yeganeh PR, Desjardins Y, Varin T, Haddad N, et al. Apple peel polyphenols: a key player in the prevention and treatment of experimental inflammatory bowel disease. Clin Sci. (2016) 130:2217–37. doi: 10.1042/CS20160524
97. Hsiang C, Hseu Y, Chang Y, Kumar KJS, Ho T, Yang H. Toona sinensis and its major bioactive compound gallic acid inhibit LPS-induced inflammation in nuclear factor-kappa B transgenic mice as evaluated by in vivo bioluminescence imaging. Food Chem. (2013) 136:426–34. doi: 10.1016/j.foodchem.2012.08.009
98. Li Y, Xie Z, Gao T, Li L, Chen Y, Xiao D, et al. A holistic view of gallic acid-induced attenuation in colitis based on microbiome-metabolomics analysis. Food Funct. (2019) 10:4046–61. doi: 10.1039/C9FO00213H
99. Kim H, Venancio VP, Fang C, Dupont AW, Talcott ST, Mertens-Talcott SU. Mango (Mangifera indica L.) polyphenols reduce IL-8, GRO, and GM-SCF plasma levels and increase Lactobacillus species in a pilot study in patients with inflammatory bowel disease. Nutr Res. (2020) 75:85–94. doi: 10.1016/j.nutres.2020.01.002
100. Kashyap PC, Chia N, Nelson H, Segal E, Elinav E. Microbiome at the frontier of personalized medicine. Mayo Clin Proc. (2017) 92:1855–64. doi: 10.1016/j.mayocp.2017.10.004
101. Wang B, Yao M, Lv L, Ling Z, Li L. The human microbiota in health and disease. Engineering. (2017) 3:71–82. doi: 10.1016/J.ENG.2017.01.008
102. Clemente JC, Ursell LK, Parfrey LW, Knight R. The impact of the gut microbiota on human health: an integrative view. Cell. (2012) 148:1258–70. doi: 10.1016/j.cell.2012.01.035
103. Turnbaugh PJ, Ridaura VK, Faith JJ, Rey FE, Knight R, Gordon JI. The effect of diet on the human gut microbiome: a metagenomic analysis in humanized gnotobiotic mice. Sci Transl Med. (2009) 1:6ra14. doi: 10.1126/scitranslmed.3000322
104. Wikoff WR, Anfora AT, Liu J, Schultz PG, Lesley SA, Peters EC, et al. Metabolomics analysis reveals large effects of gut microflora on mammalian blood metabolites. Proc Natl Acad Sci USA. (2009) 106:3698–703. doi: 10.1073/pnas.0812874106
105. Kang J, Li Q, Liu L, Jin W, Wang J, Sun Y. The specific effect of gallic acid on Escherichia coli biofilm formation by regulating pgaABCD genes expression. Appl Microbiol Biotechnol. (2018) 102:1837–46. doi: 10.1007/s00253-017-8709-3
106. Kang J, Liu L, Liu M, Wu X, Li J. Antibacterial activity of gallic acid against Shigella flexneri and its effect on biofilm formation by repressing mdoH gene expression. Food Control. (2018) 94:147–54. doi: 10.1016/j.foodcont.2018.07.011
107. Liu M, Wu X, Li J, Liu L, Zhang R, Shao D, et al. The specific anti-biofilm effect of gallic acid on Staphylococcus aureus by regulating the expression of the ica operon. Food Control. (2017) 73:613–8. doi: 10.1016/j.foodcont.2016.09.015
108. Sorrentino E, Succi M, Tipaldi L, Pannella G, Maiuro L, Sturchio M, et al. Antimicrobial activity of gallic acid against food-related Pseudomonas strains and its use as biocontrol tool to improve the shelf life of fresh black truffles. Int J Food Microbiol. (2018) 266:183–9. doi: 10.1016/j.ijfoodmicro.2017.11.026
109. Li Z, Liu M, Dawuti G, Dou Q, Ma Y, Liu H, et al. Antifungal activity of gallic acid in vitro and in vivo. Phytother Res. (2017) 31:1039–45. doi: 10.1002/ptr.5823
110. You H, Huang C, Chen C, Chang C, Liao P, Huang S. Anti-pandemic influenza A (H1N1) virus potential of catechin and gallic acid. J Chin Med Assoc. (2018) 81:458–68. doi: 10.1016/j.jcma.2017.11.007
111. Alam P, Parvez MK, Arbab AH, Al-Dosari MS. Quantitative analysis of rutin, quercetin, naringenin, and gallic acid by validated RP- and NP-HPTLC methods for quality control of anti-HBV active extract of guiera senegalensis. Pharm Biol. (2017) 55:1317–23. doi: 10.1080/13880209.2017.1300175
112. Gutiérrez-Fernández J, García-Armesto MR, Álvarez-Alonso R, Del Valle P, de Arriaga D, Rúa J. Antimicrobial activity of binary combinations of natural and synthetic phenolic antioxidants against Enterococcus faecalis. J Dairy Sci. (2013) 96:4912–20. doi: 10.3168/jds.2013-6643
113. Li K, Guan G, Zhu J, Wu H, Sun Q. Antibacterial activity and mechanism of a laccase-catalyzed chitosan-gallic acid derivative against Escherichia coli and Staphylococcus aureus. Food Control. (2019) 96:234–43. doi: 10.1016/j.foodcont.2018.09.021
114. Guzman K, Kumar B, Jose Vallejo M, Grijalva M, Debut A, Cumbal L. Ultrasound-assisted synthesis and antibacterial activity of gallic acid-chitosan modified silver nanoparticles. Prog Org Coat. (2019) 129:229–35. doi: 10.1016/j.porgcoat.2019.01.009
115. Singh A, Dutta PK, Kumar H, Kureel AK, Rai AK. Improved antibacterial and antioxidant activities of gallic acid grafted chitin-glucan complex. J Polym Res. (2019) 26:234. doi: 10.1007/s10965-019-1893-3
116. Shamsi S, Elias N, Sarchio SNE, Yasin FM. Gallic acid loaded graphene oxide based nanoformulation (GAGO) as potential anti-bacterial agent against Staphylococcus aureus. Mater Today Proc. (2018) 5:S160–5. doi: 10.1016/j.matpr.2018.08.059
117. Lee JM, Choi K, Min J, Kim H, Jee J, Park BJ. Functionalized ZnO nanoparticles with gallic acid for antioxidant and antibacterial activity against methicillin-resistant S-aureus. Nanomaterials Basel. (2017) 7:365. doi: 10.3390/nano7110365
118. Sherin L, Shujaat S, Sohail A, Arif F. Synthesis and biological evaluation of novel gallic acid analogues as potential antimicrobial and antioxidant agents. Croat Chem Acta. (2018) 91:551–65. doi: 10.5562/cca3429
119. Gutiérrez-Larraínzar M, Rúa J, Caro I, de Castro C, de Arriaga D, García-Armesto MR, et al. Evaluation of antimicrobial and antioxidant activities of natural phenolic compounds against foodborne pathogens and spoilage bacteria. Food Control. (2012) 26:555–63. doi: 10.1016/j.foodcont.2012.02.025
120. Bajpai B, Patil S. A new approach to microbial production of gallic acid. Braz J Microbiol. (2008) 39:708–11. doi: 10.1590/S1517-83822008000400021
121. López-Romero JC, Valenzuela-Melendres M, Juneja VK, García-Dávila J, Camou JP, Peña-Ramos A, et al. Effects and interactions of gallic acid, eugenol and temperature on thermal inactivation of salmonella spp. in ground chicken. Food Res Int. (2018) 103:289–94. doi: 10.1016/j.foodres.2017.10.055
122. Marín L, Miguélez EM, Villar CJ, Lombó F. Bioavailability of dietary polyphenols and gut microbiota metabolism: antimicrobial properties. Biomed Res Int. (2015) 2015:905215. doi: 10.1155/2015/905215
123. Shi X, Xiao C, Wang Y, Tang H. Gallic acid intake induces alterations to systems metabolism in rats. J Proteome Res. (2013) 12:991–1006. doi: 10.1021/pr301041k
124. Miller TL, Wolin MJ. Fermentations by saccharolytic intestinal bacteria. Am J Clin Nutr. (1979) 32:164–72. doi: 10.1093/ajcn/32.1.164
125. Yap YA, Marino E. An insight into the intestinal web of mucosal immunity, microbiota, and diet in inflammation. Front Immunol. (2018) 9:2617. doi: 10.3389/fimmu.2018.02617
126. Honda K, Littman DR. The microbiome in infectious disease and inflammation. Annu Rev Immunol. (2012) 30:759–95. doi: 10.1146/annurev-immunol-020711-074937
127. Broom LJ, Kogut MH. Gut immunity: its development and reasons and opportunities for modulation in monogastric production animals. Anim Health Res Rev. (2018) 19:46–52. doi: 10.1017/S1466252318000026
128. Williams AR, Krych L, Fauzan Ahmad H, Nejsum P, Skovgaard K, Nielsen DS, et al. A polyphenol-enriched diet and Ascaris suum infection modulate mucosal immune responses and gut microbiota composition in pigs. PLoS ONE. (2017) 12:e0186546. doi: 10.1371/journal.pone.0186546
129. Campbell NK, Fitzgerald HK, Fletcher JM, Dunne A. Plant-derived polyphenols modulate human dendritic cell metabolism and immune function via AMPK-dependent induction of heme oxygenase-1. Front Immunol. (2019) 10:345. doi: 10.3389/fimmu.2019.00345
130. Zhao J, Zang J, Lin Y, Wang Y, Li D, Meng X. Polyphenol-rich blue honeysuckle extract alleviates silica-induced lung fibrosis by modulating Th immune response and NRF2/HO-1 MAPK signaling. J Funct Foods. (2019) 53:176–86. doi: 10.1016/j.jff.2018.12.030
131. Ding S, Jiang H, Fang J. Regulation of immune function by polyphenols. J Immunol Res. (2018) 2018:1264074. doi: 10.1155/2018/1264074
132. Jantan I, Haque MA, Ilangkovan M, Arshad L. An insight into the modulatory effects and mechanisms of action of Phyllanthus species and their bioactive metabolites on the immune system. Front Pharmacol. (2019) 10:878. doi: 10.3389/fphar.2019.00878
133. Haute GV, Caberlon E, Squizani E, de Mesquita FC, Pedrazza L, Martha BA, et al. Gallic acid reduces the effect of LPS on apoptosis and inhibits the formation of neutrophil extracellular traps. Toxicol In Vitro. (2015) 30:309–17. doi: 10.1016/j.tiv.2015.10.005
134. De Bona KS, Bonfanti G, Bitencourt PE, Da ST, Borges RM, Boligon A, et al. Protective effect of gallic acid and Syzygium cumini extract against oxidative stress-induced cellular injury in human lymphocytes. Drug Chem Toxicol. (2016) 39:256–63. doi: 10.3109/01480545.2015.1084631
135. Pereira ADS, de Oliveira LS, Lopes TF, Baldissarelli J, Palma TV, Soares MSP, et al. Effect of gallic acid on purinergic signaling in lymphocytes, platelets, and serum of diabetic rats. Biomed Pharmacother. (2018) 101:30–6. doi: 10.1016/j.biopha.2018.02.029
136. Jantan I, Ilangkovan M, Yuandani, Mohamad HF. Correlation between the major components of Phyllanthus amarus and Phyllanthus urinaria and their inhibitory effects on phagocytic activity of human neutrophils. BMC Complem Altern Med. (2014) 14:429. doi: 10.1186/1472-6882-14-429
137. Sourani Z, Shirzad H, Shirzad M, Pourgheysari B. Interaction between gallic acid and asparaginase to potentiate anti-proliferative effect on lymphoblastic leukemia cell line. Biomed Pharmacother. (2017) 96:1045–54. doi: 10.1016/j.biopha.2017.11.122
138. Alves MMDM, Brito LM, Souza AC, Queiroz BCSH, de Carvalho TP, Batista JF, et al. Gallic and ellagic acids: two natural immunomodulator compounds solve infection of macrophages by leishmania major. Naunyn Schmiedebergs Arch Pharmaco. (2017) 390:893–903. doi: 10.1007/s00210-017-1387-y
139. Reyes AWB, Arayan LT, Hop HT, Ngoc Huy TX, Vu SH, Min W, et al. Effects of gallic acid on signaling kinases in murine macrophages and immune modulation against Brucella abortus 544 infection in mice. Microb Pathog. (2018) 119:255–9. doi: 10.1016/j.micpath.2018.04.032
140. Mao X, Wu L, Zhao H, Liang W, Chen W, Han S, et al. Transport of corilagin, gallic acid, and ellagic acid from fructus phyllanthi tannin fraction in Caco-2 cell monolayers. Evid Based Compl Alt. (2016) 2016:9205379. doi: 10.1155/2016/9205379
141. Faralli A, Shekarforoush E, Mendes A, Chronakis I. Enhanced transepithelial permeation of gallic acid and (–)-epigallocatechin gallate across human intestinal Caco-2 cells using electrospun xanthan nanofibers. Pharmaceutics. (2019) 11:155. doi: 10.3390/pharmaceutics11040155
142. Sowndhar Rajan B, Manivasagam S, Dhanusu S, Chandrasekar N, Krishna K, Kalaiarasu LP, et al. Diet with high content of advanced glycation end products induces systemic inflammation and weight gain in experimental mice: protective role of curcumin and gallic acid. Food Chem Toxicol. (2018) 114:237–45. doi: 10.1016/j.fct.2018.02.016
Keywords: polyphenol, gallic acid, gut microbiome, immune response, gastrointestinal health
Citation: Yang K, Zhang L, Liao P, Xiao Z, Zhang F, Sindaye D, Xin Z, Tan C, Deng J, Yin Y and Deng B (2020) Impact of Gallic Acid on Gut Health: Focus on the Gut Microbiome, Immune Response, and Mechanisms of Action. Front. Immunol. 11:580208. doi: 10.3389/fimmu.2020.580208
Received: 05 July 2020; Accepted: 17 August 2020;
Published: 16 September 2020.
Edited by:
Kai Wang, Chinese Academy of Agricultural Sciences (CAAS), ChinaReviewed by:
Nicolò Merendino, University of Tuscia, ItalyM. Angeles Martin, Instituto de Ciencia y Tecnología de Alimentos y Nutrición (ICTAN), Spain
Mengzhi Wang, Yangzhou University, China
Copyright © 2020 Yang, Zhang, Liao, Xiao, Zhang, Sindaye, Xin, Tan, Deng, Yin and Deng. This is an open-access article distributed under the terms of the Creative Commons Attribution License (CC BY). The use, distribution or reproduction in other forums is permitted, provided the original author(s) and the copyright owner(s) are credited and that the original publication in this journal is cited, in accordance with accepted academic practice. No use, distribution or reproduction is permitted which does not comply with these terms.
*Correspondence: Yulong Yin, eWlueXVsb25nJiN4MDAwNDA7aXNhLmFjLmNu; Baichuan Deng, ZGVuZ2JhaWNodWFuJiN4MDAwNDA7c2NhdS5lZHUuY24=