- 1Centro de Investigação Translacional em Oncologia (CTO), Instituto do Câncer do Estado de São Paulo (ICESP), Faculdade de Medicina da Universidade de São Paulo (FMUSP), São Paulo, Brazil
- 2Departamento de Imunologia, Instituto de Ciências Biomédicas, Universidade de São Paulo, São Paulo, Brazil
- 3Department of Pediatrics, Faculdade de Medicina da Universidade de São Paulo (FMUSP), São Paulo, Brazil
- 4Laboratory of Medical Investigation in Pathogenesis and Targeted Therapy in Onco-Immuno-Hematology (LIM-31), Department of Hematology, Hospital das Clínicas HCFMUSP, Faculdade de Medicina, Universidade de Sao Paulo, Sao Paulo, Brazil
Immune evasion is an important cancer hallmark and the understanding of its mechanisms has generated successful therapeutic approaches. Induction of immunogenic cell death (ICD) is expected to attract immune cell populations that promote innate and adaptive immune responses. Here, we present a critical advance for our adenovirus-mediated gene therapy approach, where the combined p14ARF and human interferon-β (IFNβ) gene transfer to human melanoma cells led to oncolysis, ICD and subsequent activation of immune cells. Our results indicate that IFNβ alone or in combination with p14ARF was able to induce massive cell death in the human melanoma cell line SK-MEL-147, though caspase 3/7 activation was not essential. In situ gene therapy of s.c. SK-MEL-147 tumors in Nod-Scid mice revealed inhibition of tumor growth and increased survival in response to IFNβ alone or in combination with p14ARF. Emission of critical markers of ICD (exposition of calreticulin, secretion of ATP and IFNβ) was stronger when cells were treated with combined p14ARF and IFNβ gene transfer. Co-culture of previously transduced SK-MEL-147 cells with monocyte-derived dendritic cells (Mo-DCs) derived from healthy donors resulted in increased levels of activation markers HLA-DR, CD80, and CD86. Activated Mo-DCs were able to prime autologous and allogeneic T cells, resulting in increased secretion of IFNγ, TNF-α, and IL-10. Preliminary data showed that T cells primed by Mo-DCs activated with p14ARF+IFNβ-transduced SK-MEL-147 cells were able to induce the loss of viability of fresh non-transduced SK-MEL-147 cells, suggesting the induction of a specific cytotoxic population that recognized and killed SK-MEL-147 cells. Collectively, our results indicate that p14ARF and IFNβ delivered by our adenoviral system induced oncolysis in human melanoma cells accompanied by adaptive immune response activation and regulation.
Introduction
Suppression of the immune system during tumor progression and spread is an important cancer hallmark (1). Tumor cells actively evade recognition and destruction by both innate and adaptive branches of the immune system (2, 3). Acquisition of defects in anti-viral pathways in tumor cells, such as those mediated by interferons (IFNs), contributes to immune evasion (4, 5). This opens important perspectives for immunotherapies that seek to restore anti-tumor immune responses. Oncolysis, the rupture of malignant cells in response to a therapeutic modality, represents an important first step. However, to be effective, induction of oncolysis alone would have to reach essentially all tumor cells. Instead, oncolysis that is accompanied by immune activation would be expected to provide a broader effect at the site of treatment and may even provide a systemic benefit. This has been seen in therapy with oncolytic viruses, though combination with other immunotherapies may be required in order to achieve elimination of non-treated tumor foci (6, 7).
There are different strategies for inducing oncolysis, yet cell death, such as by apoptosis, may not elicit an immune response and may even lead to tolerance (8). In contrast, cell death accompanied by the release of immunogenic cell death (ICD) factors serves to alarm the immune system by attracting and activating immune cell populations (9–11). To this end, virus-mediated oncolysis, such as that seen with oncolytic virotherapy, promotes cell death along with the liberation of pathogen-associated molecular patterns (PAMPs), damage-associated molecular patterns (DAMPs), as well as tumor antigens that together can cooperate with the activation of dendritic cells which, in turn, prime tumor-specific T cells (5, 12–14). Viruses that trigger oncolysis have revealed strong potential as immunotherapeutic approaches (14, 15) with many clinical trials currently recruiting (as per clinicaltrials.gov), as well as FDA approval for use in patients (16, 17).
Our group has been working on the development of non-replicating adenoviral vectors that induce oncolysis due to the combined activity of the p14ARF and IFNβ transgenes as well as antiviral response (18). While p14ARF (alternate reading frame of the CDKN2a locus, p19Arf in mice, p14ARF in humans) acts as the functional partner of p53, IFNβ is a critical cytokine that contributes to innate and adaptive anti-tumor responses (19). Using mouse models, our data indicate that transgene activity plus an antiviral response culminate in ICD, thus our approach may be considered as a strategy for cancer immunotherapy (20–22). However, IFNβ acts in a species specific manner (23), thus the phenomena seen in mouse models must be verified using human cell lines.
Melanoma is a highly lethal neoplasm due to the difficulty in treatment after metastatic spread (24, 25). Peculiarities in the melanoma genotype shaped the rationale of the adenoviral strategy used in this work. Since 80% of melanomas preserve p53 in its wild-type form (26), we have incorporated a p53-responsive promoter (PG) to drive transgene expression (18). The p14ARF transgene is expected to activate endogenous p53, in turn providing both tumor suppressor function and transactivation from the PG promoter (27). In addition to its role in immune activation, the IFNβ pathway is also known to promote cell death via the p53/p14ARF axis (28, 29). Besides that, deletions are commonly found in the chromosome 9p21 gene cluster where CDKN2a, p14ARF, and IFNβ are located (30–33), reinforcing the importance of the p14ARF and IFNβ transgene combination.
Here, we show a critical advance in the development of our approach since we explore combined p14ARF and IFNβ gene transfer in a human melanoma cell line, SK-MEL-147. We confirmed oncolysis and also reveal that combined gene transfer is required for the induction of ICD, characterized by emission of DAMPS, activation of dendritic cells from healthy donors and their ability to prime T cells to, then, carry out tumor cell cytolysis. Thus, we suggest that the oncolysis and subsequent activation of immune functions predict that our adenovirus-mediated p14ARF plus IFNβ gene transfer approach could act as an immunotherapy in humans.
Material and Methods
Cell Lines
The SK-MEL-147 human melanoma cell line was authenticated by analysis of short tandem repeats using GenePrint 10 (Promega, Internal Standard-ILS 600, performed by the Rede Premium Core Facility, FMUSP) and tested negative for mycoplasma by a PCR assay using conditioned medium as template and amplification using the following oligonucleotides:
Myco F: 5’-GGG AGC AAA CAC GAT TAG ATA CCC T -3’
Myco R: 5’-TGC ATT ATC TGT CAC TCT GTT AAC CTC -3’
This cell line as well as HEK293 were cultured in DMEM with 10% fetal calf serum, supplemented with antibiotic-antimycotic (Thermo Fisher Scientific, Waltham, MA, USA) and maintained at 37°C and 5% CO2 atmosphere.
Construction, Production, and Titration of Adenoviral Vectors
The strategy for constructing the adenoviral vectors has been described previously (21).
For the generation of the recombinant adenovirus we first constructed the “pEntr-PG” vector containing transgenes of interest: i) Luc2, used as control, ii) Luc2-p14ARF, and iii) Luc2-hIFNβ (Figure S1). Next, site directed recombination was performed with the “destiny” vector encoding the Ad5 backbone (non-replicating, E1/E3 deleted, RGD modified fiber) utilizing Gateway L/R Clonase II Enzyme (Life Technologies, Carlsbad, CA, USA) as previously described (21, 34), giving rise to AdRGD-PG-Luc2, AdRGD-PG-Luc2-p14ARF, and AdRGD-PG-Luc2-hIFNβ. Following viral amplification, purification was performed using an iodixanol gradient followed by desalting, as described by Peng et al. (35) and as per our previous studies (21, 36). For the determination of biological titer, we used the Adeno-X Rapid Titer Kit (Clontech, Mountain View, CA, USA) which is based on immunodetection of the adenoviral hexon protein in transduced cells. The biological titer (transducing units per milliliter, TU/ml) was used for the calculation of the multiplicity of infection (MOI) indicated in each experiment.
Cell Transduction
SK-MEL-147 cells were seeded in medium containing 2% FBS together with the corresponding vectors at a final MOI of 50: i) AdRGD-PG-Luc2, ii) AdRGD-PG-Luc2-p14ARF, and iii) AdRGD-PG-Luc2-hIFNβ and iv) combination of AdRGD-PG-Luc2-p14ARF and AdRGD-PG-Luc2-hIFNβ (p14ARF+IFNβ, MOI 25 each). After 4 h incubation at 37°C, an equal volume of DMEM/10% FBS was added and the cells incubated for 24 and 48 h, unless otherwise noted. The described strategy was employed to guarantee the consistent number of viral particles for each treatment condition.
Transgene Expression
For validation of luciferase transgene activity, we used the Luciferase Assay System kit as per the manufacturer’s protocol (Promega, Madison, WI, USA). Sample luminescence was read on a 1420 Multilabel Counter VICTOR3 (Perkin Elmer, Waltham, MA, USA). The luminescence results were normalized by protein concentration. Interferon-β detection was performed using the Verikine human IFN beta ELISA kit (PBL Assay Science, Piscataway, NJ, USA) and conditioned medium derived from transduced cell cultures. For p14ARF, we use immunofluorescence and Western blot as previously described (37–39), using anti-p14ARF antibody (Santa Cruz Biotechnology, Dallas, TX, USA). Immunofluorescence detection was obtained using EVOS FL Cell Imaging System (Thermo Fisher Scientific).
Evaluation of Cell Death by Flow Cytometry
Cells were transduced and incubated as described before harvest of both detached and adherent cells, fixation in 70% ethanol, RNAse treatment and staining with PI (21). Alternatively, fresh cells were treated with the LIVE/DEAD ™ Fixable Green Dead Cell Stain Kit, for 488 nm excitation (Thermo Fisher Scientific, #L23101) following the manufacturer’s recommendations. For either assay, cells were subjected to flow cytometry and analysis using the manufacturer’s software (Attune™, Thermo Fisher Scientific). For measurement of caspase activity, transduced cells were collected and treated with Cell Event Caspase 3/7 Green Detection reagent (Thermo Fisher Scientific, Cat. No. C10423) diluted and incubated according to the recommendations of the manufacturer. The proportion of fluorescent cells resulting from activation of caspases 3 and 7 was assessed by flow cytometry (Attune™, Thermo Fisher Scientific).
In Situ Gene Therapy
All animal experimentation and the protocols described here were approved by the Ethics in Animal Use Committee (FMUSP) and performed at the Centro de Medicina Nuclear (CMN), FMUSP, São Paulo, Brazil. Assays were performed using 8–12 weeks old female Nod-Scid mice obtained from the Bioterio Central, FMUSP. After local trichotomy, SK-MEL-147 cells (1 × 106) were implanted subcutaneously in the left flank and animals were observed until tumors reached 60 mm3 and treatment was initiated. Intratumoral injection was performed on four occasions at 48-h intervals (days 1, 3, 5, and 7), applying 2 × 108 TU in 50 µl of 1× PBS as excipient. Tumors were treated with PBS, AdRGD-PG-Luc2 or AdRGD-PG-Luc2-IFNβ, AdRGD-PG-Luc2-p14ARF, and combination of p14ARF+IFNβ. The number of animals/group is indicated in the figure legends. Tumors were measured with calipers and the volume calculated according to the formula: ½ × (length) × (width)2 (39, 40). We consider the tumor volume of 1000 mm3 as experimental end point when the animals were anesthetized in a chamber with 4% isoflurane and euthanized by CO2 inhalation. Otherwise, animals that did not reach the end point were monitored until 60 days. Distribution of animals/cage was maintained according to the pre-defined experimental groups at the beginning of the treatment. Alternatively, on experimental day 9 (48 h after the last treatment), some of these animals were euthanized and samples collected for histologic analyses.
Immunogenic Cell Death Assays
The ICD markers were analyzed following the protocols previously described (21). For each assay, 1 × 105 cells were treated with the vectors for 48 h, and then, cells and supernatant were collected. For the evaluation of calreticulin exposure through flow cytometry, cells were probed with rabbit anti-calreticulin antibody (Novus Biologicals, Littleton, CO, USA), followed by the Alexa488-conjugated anti-rabbit secondary antibody (Thermo Fisher Scientific). The cells were then analyzed using the Attune cytometer (Thermo Fisher Scientific). The ATP secreted in the media was evaluated using the ENLITEN ATP Assay System (Promega), following the manufacturer protocol, and the luminescence obtained with tube reader Sirius L Tube Luminometer (Titertek Berthold, Germany).
Isolation of Peripheral Blood Mononuclear Cells (PBMCs), Mo-DCs Differentiation, and Activation With Transduced Tumor Cells
All experiments were performed after the approval of the institutional Committee for Ethics in Research (Fundação Pró-Sangue, CEP#03, FMUSP). Healthy donors’ peripheral blood was obtained from leukoreduction chambers (41) after signed written informed consents. PBMCs were isolated by density gradient centrifugation over Ficoll-Paque (GE Healthcare, Uppsala, Sweden). For the generation of monocyte-derived DCs, PBMCs were either plated for 2 h for adherence of monocytes to the plastic and subsequent removal of non-adherent cells (42) or by positive magnetic selection (Milteny Biotec, Bergisch Gladbach, Germany) for the isolation of CD14+ monocytes. The monocytes were cultured at 37°C and 5% CO2 in RPMI-1640 medium supplemented with 10% FBS, antibiotic-antimycotic (Thermo Fisher Scientific) and 50 ng/ml of IL-4 plus 50 ng/ml of GM-CSF (both from PeproTech, Rocky Hill, NJ, USA) for 5 days to obtain immature monocyte-derived dendritic cells (iDCs) (43). At day 5, iDCs cells were harvested, washed, counted, and activated for 24 h with previously transduced SK-MEL-147 cells at a 1:1 and 1:10 ratios. Cells were harvested and analyzed by flow cytometry using CD209 (DCN46, #551545, BD), HLA-DR (G46-6, #556643, BD), CD80 (L307.4, #340294, BD), CD83 (HB15e, #561132, BD), CD86 (2331, #561124, BD) or used for the priming of autologous T cells.
T Cell Isolation and Priming
T cells were enriched by either recovery of non-adherent cells after 2 h of PBMCs plastic adherence or by negative magnetic selection with the Pan T cell isolation kit (Milteny Biotec). Aliquots of T cells were cryopreserved until used in subsequent experiments. For T cell priming, activated Mo-DCs were harvest, washed, counted, and co-cultured for 7 days with autologous T cells at a 1:10 ratio in 96-well U-bottom plates (Corning, Tewksbury, MA, USA), with human IL-7 (50ng/ml), IL-2 (250 U/ml), and IL-15 (5 ng/ml) (all from Peprotech) with media replacement with fresh cytokines every 3 days. T cell proliferation was determined by carboxyfluorescein succinimidyl ester (CellTrace™ CFSE Cell Proliferation Kit, #C34554, Thermo Fisher Scientific) dilution, where T cells were previously stained with 5 μM CFSE. After 7 days, T cells were harvested and stained for surface markers CD3 (SK7, #340542, BD), PD1 (EH12.1, #561273, BD), TIM3 (F38-2E2, #25-3109-42, Thermo Fisher Scientific), LAG3 (3DS223H, #12-2239-41, Thermo Fisher Scientific), and CFSE dilution was determined by flow cytometry.
Cytolytic T Cell Activity
Primed T cells were seeded in 96-well U-bottom plates together with fresh non-transduced SK-MEL-147 in a 10: 1 ratio. After 48 h of incubation, SK-MEL-147 viability was assessed through LIVE/DEAD staining (Thermo Fisher Scientific) by flow cytometry. SK-MEL-147 cells were gated based on size (FSChi) and absence of CD3 staining.
Cytokine Measurements by CBA
To determine the cytokine profiles of stimulated T cells, we use co-cultures with allogeneic Mo-DCs primed with adenoviral-transduced SK-MEL-147 cells. Supernatants were collected after 3 days of cultures and analyzed by CBA. Analysis of IL-2, IL-4, IL-6, IL-10, IL-17A, TNF-α, and IFN-γ levels was performed using the Cytokine Bead Array Th1/Th2/Th17 kit (BD Biosciences, San Jose, CA, USA). Samples were acquired with a FACS II LRS Fortessa (Becton, Dickinson and Company, USA) according to the manufacturer’s instructions and analyzed using the FCAP Array Software 3.0 (BD Biosciences, San Jose, CA, USA).
Statistical Analysis
Statistical analyses were performed using GraphPad Prism software version 5.0 (GraphPad Software, San Diego, CA, USA). Statistical significance was calculated using one-way or two-way ANOVA when appropriate, followed by Bonferroni post-test. Differences were considered significant when p < 0.05. Number of replicates and sample sizes for all experiments are detailed in the figure legends.
Results
Adenovirus-Mediated Gene Transfer of p14ARF and IFNβ Induces Cell Death in Human Melanoma Cells
Adenoviral vectors encoding Luc2, p14ARF or IFNβ were constructed (Figure S1) and transgene expression was validated (Figures S2 and S3). In this work, we focus on the SK-MEL-147 human melanoma cell line since it harbors wild-type p53. Subsequently, we measured hypodiploid cell populations after transduction, revealing that the combination of adenoviruses p14ARF+IFNβwas superior to individual gene transfer for the induction of hypodiploidy (Figure 1A). Figure 1B shows the increase hypodiploidy over time, reaching 45% of cells in 48 h.
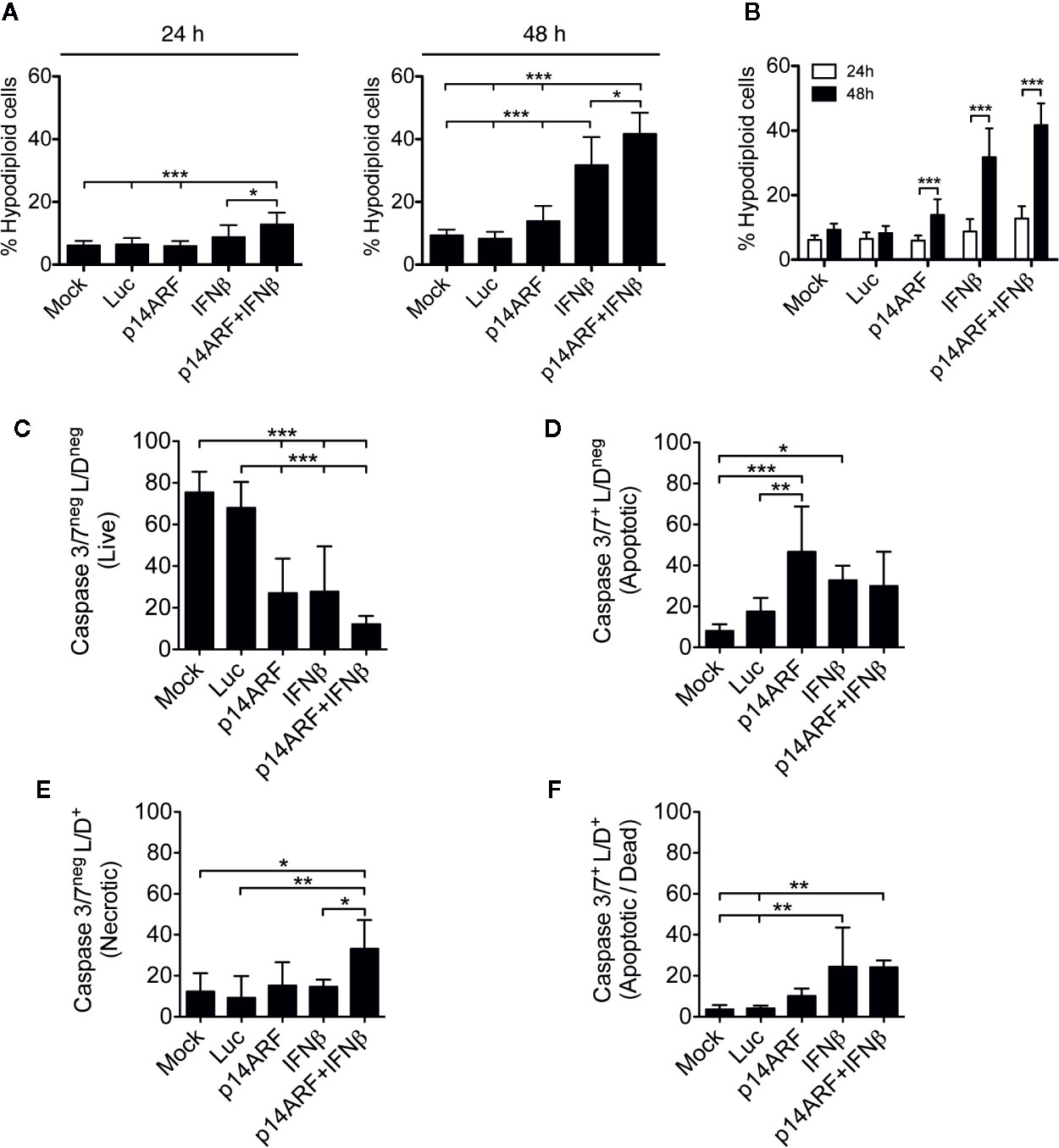
Figure 1 p14ARF and IFNβ gene transfer triggers cell death in human melanoma cells. SK-MEL-147 cells without treatment (Mock) or transduced with AdRGD-PG-Luc2 (Luc), AdRGD-Luc2-PG-p14ARF (p14ARF), AdRGD-PG-Luc2-IFNβ (IFNβ) or the combination (p14ARF + IFNβ) were subjected to cell death assays. (A, B) Graphs depict propidium iodide labeling performed 24 and 48 h after transduction. (C–F) Next, 48 h after transduction, cells were doubly marked for active caspase 3/7 and with LIVE/DEAD® (L/D) to measure cellular membrane permeability. Thus, the following graphs demonstrate: (C) Live: Caspase 3/7neg, L/Dneg, (D) Apoptotic: Caspase 3/7+, L/Dneg, (E) Necrotic: Caspase 3/7neg, L/D+, and (F) Apoptotic/Dead: Caspase 3/7+, L/D+. Samples were acquired by flow cytometry. Mean and standard deviation were obtained from three independent assays each performed with technical triplicates. Statistical analyses were performed using one-way ANOVA test followed by Bonferroni post-test. *p < 0.05, **p < 0.005, and ***p < 0.0005.
Next, we assessed the alteration of cell membrane permeability in a live/dead assay (L/D) and also measured caspase 3/7 activity 48 h after transduction. Figure 1C shows that the number of live cells (Caspase 3/7neg, L/Dneg) was significantly reduced in the groups that received IFNβ, p14ARF or the combination as compared to the Mock or Luc control groups. Representative dot-blot graphs are shown in Figure S4. Apoptotic cells, those positive for caspase 3/7 activity (Casp3/7+) and with cell membrane integrity (L/Dneg), were more frequent upon treatment with p14ARF (Figure 1D). In contrast, combined gene transfer was associated with necrosis (Casp3/7neg, L/D+), indicating loss of cell membrane integrity even without caspase 3/7 activity (Figure 1E). Caspase activity with concomitant loss of cell membrane integrity (Casp 3/7+, L/D+) was associated with IFNβ treatment alone or in combination with p14ARF (Figure 1F). These data indicate that treatment with p14ARF+IFNβ may induce cell death by a mechanism that is independent of caspase 3/7 activity.
Nod-Scid Mouse Model of In Situ Gene Therapy Reveals Prolonged Survival in Response to Combined Gene Transfer
After seeing that adenovirus carrying the p14ARF and IFNβ transgenes triggered cell death in human melanoma cells in vitro, we questioned whether this potential could also be observed in vivo. For this, tumors were established by injection (s.c.) of 1 × 106 cells in the right flank of Nod-Scid mice. Once the tumors had reached a volume of 60 mm3, we initiated the treatment regimen consisting of four doses of adenovirus (2 × 108 TU per dose) administered by intratumoral injections with 48-h intervals between doses. The first day of treatment is considered as day 1 (thus virus was administered on days 1, 3, 5, and 7). The experimental endpoint in the PBS and Luc controls was reached around the tenth day of treatment, while IFNβ alone or in combination with p14ARF show significantly reduced tumor growth (Figure 2A) and prolonged survival (Figure 2B). We noticed an intermediate effect when p14ARF was used alone. While IFNβ was sufficient to inhibit tumor progression, we postulate that the benefit of p14ARF may lie in the mechanism of cell killing and the impact of oncolysis on the host response.
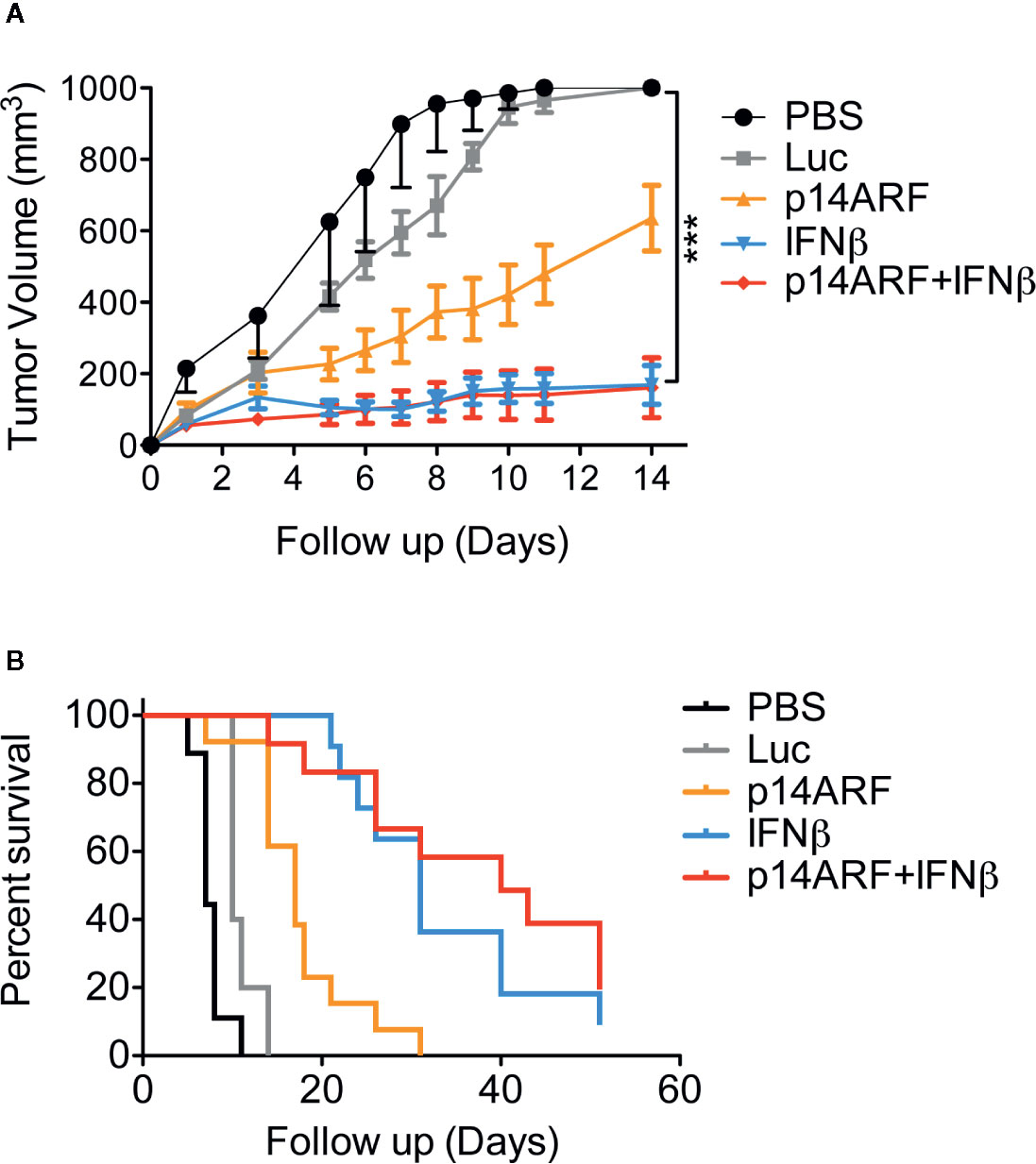
Figure 2 In situ gene therapy with the combination of p14ARF and IFNβ impaired tumor progression and improved survival rates in mouse xenograft model. Fresh SK-MEL-147 cells were inoculated in Nod-Scid mice, generating tumors after 7 days. The therapeutic regimen comprised four intra-tumoral injections at 48-h intervals with excipient alone (PBS, n = 9) or containing 2 × 108 TU of AdRGD-PG-Luc2 (Luc, n = 5), AdRGD-PG-Luc2-p14ARF (p14ARF, n = 13), AdRGD-PG-Luc2-IFNβ (IFNβ, n = 11) or the combination (p14ARF + IFNβ, 1 × 108 TU each virus, n = 12). (A) The tumor volume (mean +/- standard error) is shown in the graph. Statistical differences between IFNβ and p14ARF + IFNβ versus PBS and Luc were revealed on day 14 using unpaired t test with Welch’s correction. ***p < 0.0005. (B) Kaplan Meier graph shows survival (percent of mice with sub-maximum tumor size). Log-rank (Mantel-Cox) Test. p < 0.0001.
Evidence of Immunogenic Cell Death Resulting From Adenoviral Treatments
Having verified the potential to generate oncolysis, we investigated whether treatment with the adenoviral vectors would trigger ICD, measured by the emission of DAMPs and activation of cellular mediators of the immune response. For this, SK-MEL-147 cells were transduced with the vectors encoding p14ARF, IFNβ or the combination and then incubated for 48 h before observing the exposure of calreticulin in the cell membrane and the secretion of ATP. Figure 3A shows that the increase in calreticulin exposure in the cell membrane of transduced cells was greatest in response to the IFNβ and p14ARF combination. This condition also resulted in increased granularity, indicating cellular stress. Figure 3B shows that secretion of ATP is most intensely induced by the p14ARF+IFNβ combination. The production of IFN-β, another DAMP, is also supported by our gene transfer approach (Figure 3C). Thus, combined gene transfer of p14ARF and IFNβ resulted in the emission of three critical DAMPs expected to contribute to immune cell activation.
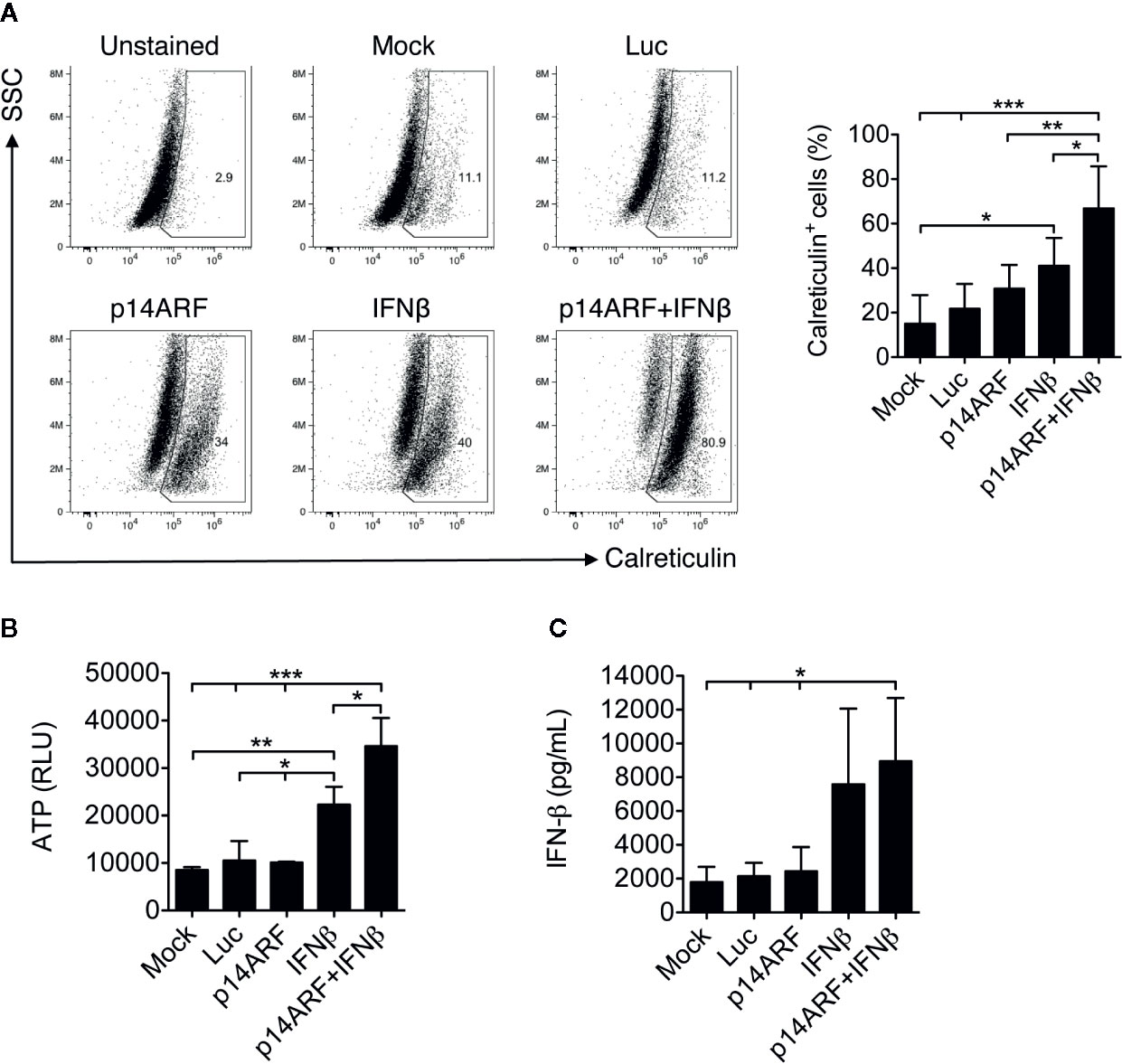
Figure 3 Emission of immunogenic cell death markers induced by combined adenoviral p14ARF + IFNβ gene transfer. SK-MEL-147 cells transduced as previously described, incubated for 48h h before cells and supernatants were collected for ICD assays. (A) Calreticulin exposure was assessed by flow cytometry after specific antibody staining. Representative dot plots and a bar graph showing the mean and standard deviation from three independent tests with three technical replicates each. (B) Supernatant from the same cultures were collected and evaluated for ATP secretion using a luciferase-based assay (RLU, relative light units). Data represent the mean and standard deviation from at least three independent experiments. (C) Detection of secreted IFNβ protein from cell supernatant by ELISA. Data represent the mean and standard deviation from at least three independent experiments. For both (A–C), statistical analyses were performed using one-way ANOVA test followed by the Bonferroni post-test. *p < 0.05, **p < 0.005, and ***p < 0.0005.
SK-MEL-147 Cells Transduced With p14ARF+IFNβ Adenoviral Vectors Induce Activation of Monocyte-Derived Dendritic Cells
To further investigate whether the induction of cell death and immunogenic factors upon transduction with the p14ARF+IFNβ adenoviral vectors could elicit an immune response, we exposed immature monocyte-derived dendritic cells (iDCs) to previously transduced SK-MEL-147 cells (Figure 4A). Combined p14ARF+IFNβ gene transfer was shown to be especially efficient for the release of ICD markers, thus was used in these assays. First, we verified that the viability of iDCs was not lost when co-cultured at different ratios (1:1 and 1:10) with SK-MEL-147 cells previously transduced at MOI 10, 50, or 100 (Figure S5). By gating on the live CD209+ iDCS, we are able to demonstrate that the frequency and expression level of HLA-DR, CD80, and CD86 increased in iDCs exposed to p14ARF+IFNβ-transduced SK-MEL-147 cells compared to iDCs exposed to mock-transduced SK-MEL-147 cells (representative histograms presented in Figure 4B). Statistically, iDCs behaved the same when treated at a ratio of 1:1 (black dots) or 5:1 (gray dots) iDC:SK-MEL-147 transduced cells, thus these results were pooled. When co-cultured in direct contact with iDC, p14ARF+IFNβ-transduced SK-MEL-147 cells induced an increase in expression of HLA-DR, CD80, and CD86 in iDCs, while co-culture in a transwell chamber revealed that soluble factors induced the upregulation of only HLA-DR in iDCs (Figure 4C). This suggests that membrane-associated factors presented by the transduced SK-MEL-147 cells are responsible for most of their dendritic cell activation effect.
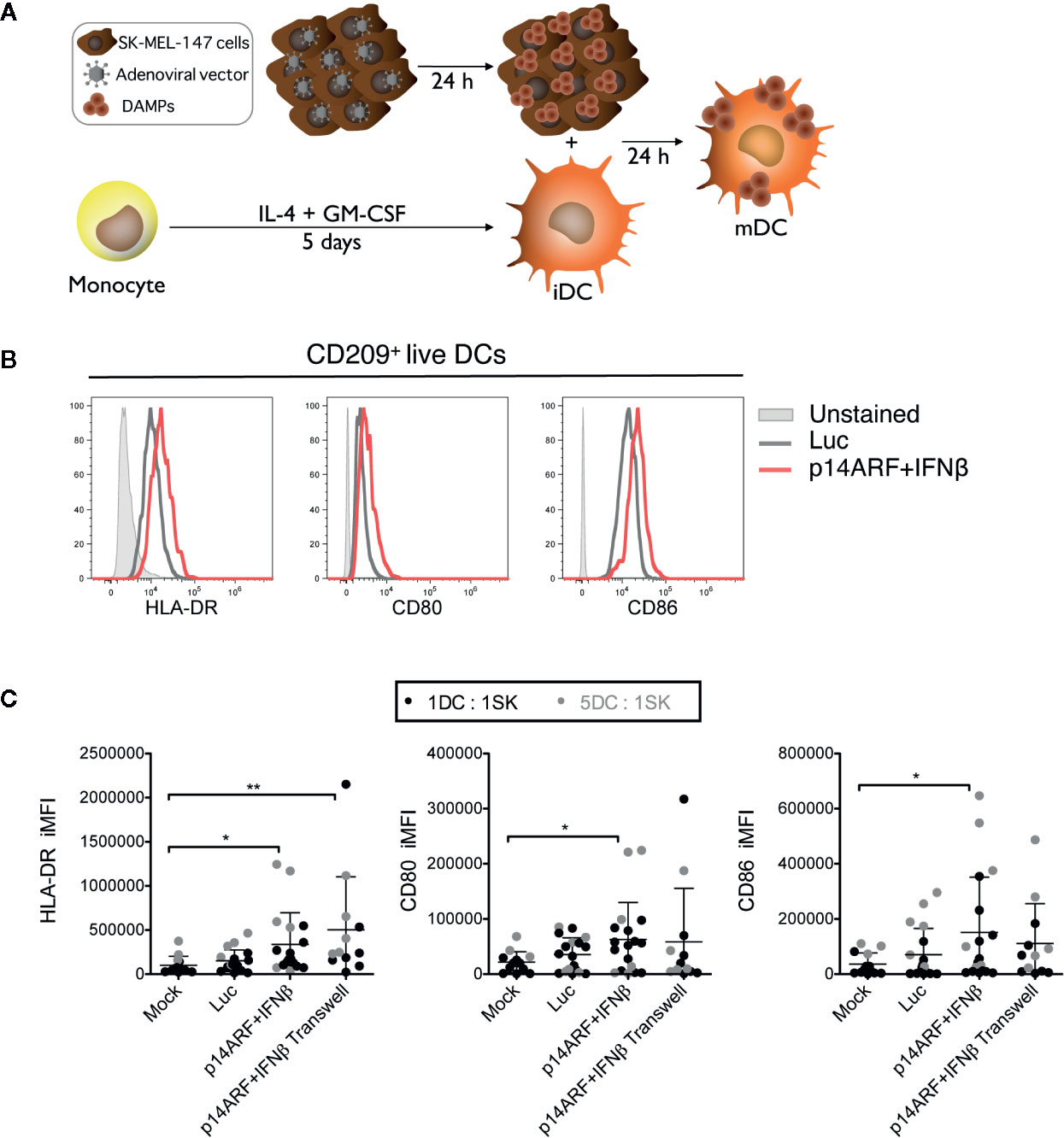
Figure 4 SK-MEL-147 cells transduced with p14ARF+IFNβ adenoviral vectors induced activation of monocyte-derived dendritic cells. (A) Schematic representation of the co-culture assay where SK-MEL-147 cells without treatment (Mock) or transduced with AdRGD-PG-Luc2 (Luc) or the combination AdRGD-Luc2-PG-p14ARF + AdRGD-PG-Luc2-IFNβ (p14ARF + IFNβ) were used to activate immature monocyte-derived dendritic cells (iDC). (B) Representative histograms and (C) frequency multiplied by MFI (iMFI) of HLA-DR, CD80 and CD86 in iDCs co-cultured in direct contact with transduced SK-MEL-147 cells or through a transwell membrane (0.4-μm pore size). Statistical analyses were performed using one-way ANOVA followed by the Bonferroni post-test. *p < 0.05, **p < 0.005.
iDCs Activated With p14ARF+IFNβ-Transduced SK-MEL-147 Cells Modulate the Priming and Function of T Cells
Next, we explored whether iDCs exposed to transduced SK-MEL-147 cells were able to prime T cells and polarize cytokine production (Figure 5A). Using an autologous system supported by IL-15, IL-7, and IL-2, we found that iDCs activated with Luc and p14ARF+IFNβ-transduced SK-MEL-147 cells were able to switch the CD4/CD8 ratio, favoring the proliferation of CD8+ T cells rather than CD4+ (Figure 5B). Compared to unstimulated CD8+ T cells, iDCs activated with mock-transduced, Luc-transduced and p14ARF+IFNβ-transduced SK-MEL-147 cells favored a non-significant upregulation of the checkpoint molecules LAG-3, PD-1, and TIM-3 among the CD8+ T cell population (Figure 5C). To characterize the cytokine milieu induced during antigen presentation and co-stimulation, we co-cultured activated DCs with allogeneic T cells to avoid the interference of external cytokines needed in the autologous system. We found that iDCs activated with p14ARF+IFNβ-transduced SK-MEL-147 cells potently induced the production of IFN-γ by T cells, compared to iDCs activated with Luc-transduced SK-MEL-147 cells; TNF-α and IL-10 were also significantly increased, however at lower concentrations than IFN-γ. In turn, IL-6 was upregulated in T cells co-cultures with both, iDCs activated with Luc and p14ARF+IFNβ-transduced SK-MEL-147 cells. When iDCs were activated with p14ARF+IFNβ-transduced SK-MEL-147 cells separated through a transwell membrane, they lost the ability to induce cytokine production by T cells. No significant differences were found when iDCs were activated at a 1:1 (black) or 5:1 (gray) ratio with p14ARF+IFNβ-transduced SK-MEL-147 cells (Figure 5D).
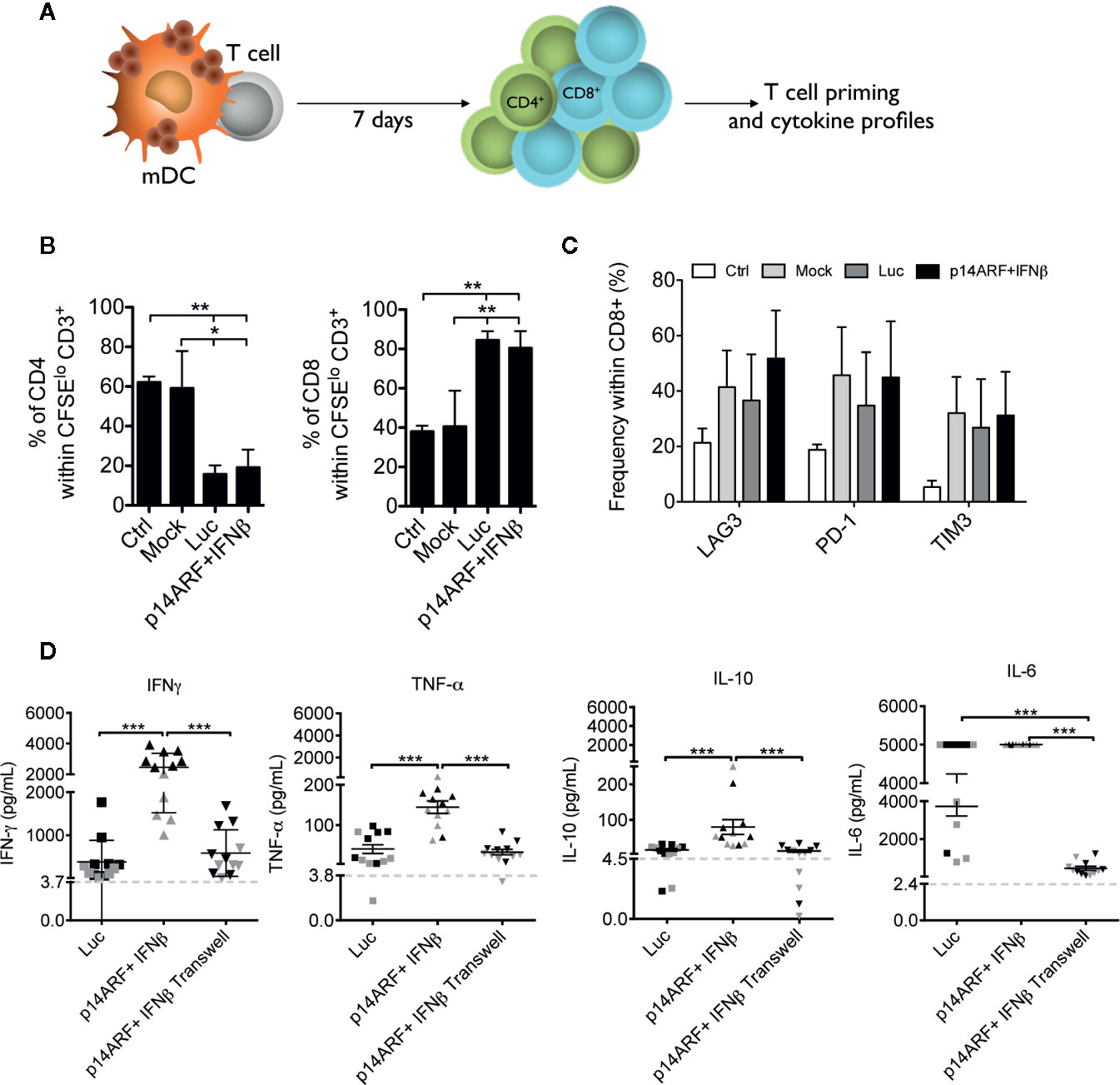
Figure 5 iDCs activated with p14ARF+IFNβ-transduced SK-MEL-147 cells modulate the priming and function of T cells. (A) Schematic representation of the DC-mediated priming of T cells. DCs (pre-activated with transduced SK-MEL-147 cells) were co-cultured with autologous T cells in the presence of IL-15, IL-7 and IL-2 to determine T cell proliferation and phenotypic profile. (B) Frequency of CD4+ and CD8+ T cells within CD3+ CFSElo (proliferating) T cells, primed by DCs previously activated with transduced SK-MEL-147 cells. Graphs show the mean and standard deviation from three independent assays. (C) Expression profile of checkpoint receptors in primed T cells. Graphs show the mean and standard deviation from three independent assays. (D) To determine the polarized cytokine profile, DCs were co-cultured with allogeneic T cells from two different donors (black and gray), to avoid the interference of external cytokines used in the autologous system. Cell-free supernatants were assessed for IFN-γ, TNF-α, IL-10, and IL-6 secretion by cytometric bead array. Gray line indicates the detection limit of each cytokine. Statistical analyses were performed using one-way ANOVA test followed by the Bonferroni post-test. *p < 0.05, **p < 0.005, and ***p < 0.0005.
T Cells Primed by iDCs Activated With p14ARF+IFNβ-Transduced SK-MEL-147 Cells Kill Fresh Non-Transduced SK-MEL-147 Cells
Finally, to explore whether iDCs activated with adenovirus-transduced SK-MEL-147 cells were able to induce a specific cytotoxic population, autologous primed T cells from two healthy donors were challenged with fresh non-transduced SK-MEL-147 cells. After 48 h, we assessed the abundance of T cells in samples and the viability of CD3neg cells within the SK-MEL-147 gate (higher FSC). We observed that within the lymphocyte gate, more than 90% of the cells were CD3+ (Figure 6A). For both donors, we found higher viability loss among fresh SK-MEL-147 cells when challenged with T cells primed by iDCs activated with p14ARF+IFNβ-transduced SK-MEL-147 cells, compared to all controls (Figure 6B). Based on these findings, we propose in Figure 6C the mechanism of immune activation in response to treatment of SK-MEL-147 cells with the combination of p14ARF+IFNβ gene transfer.
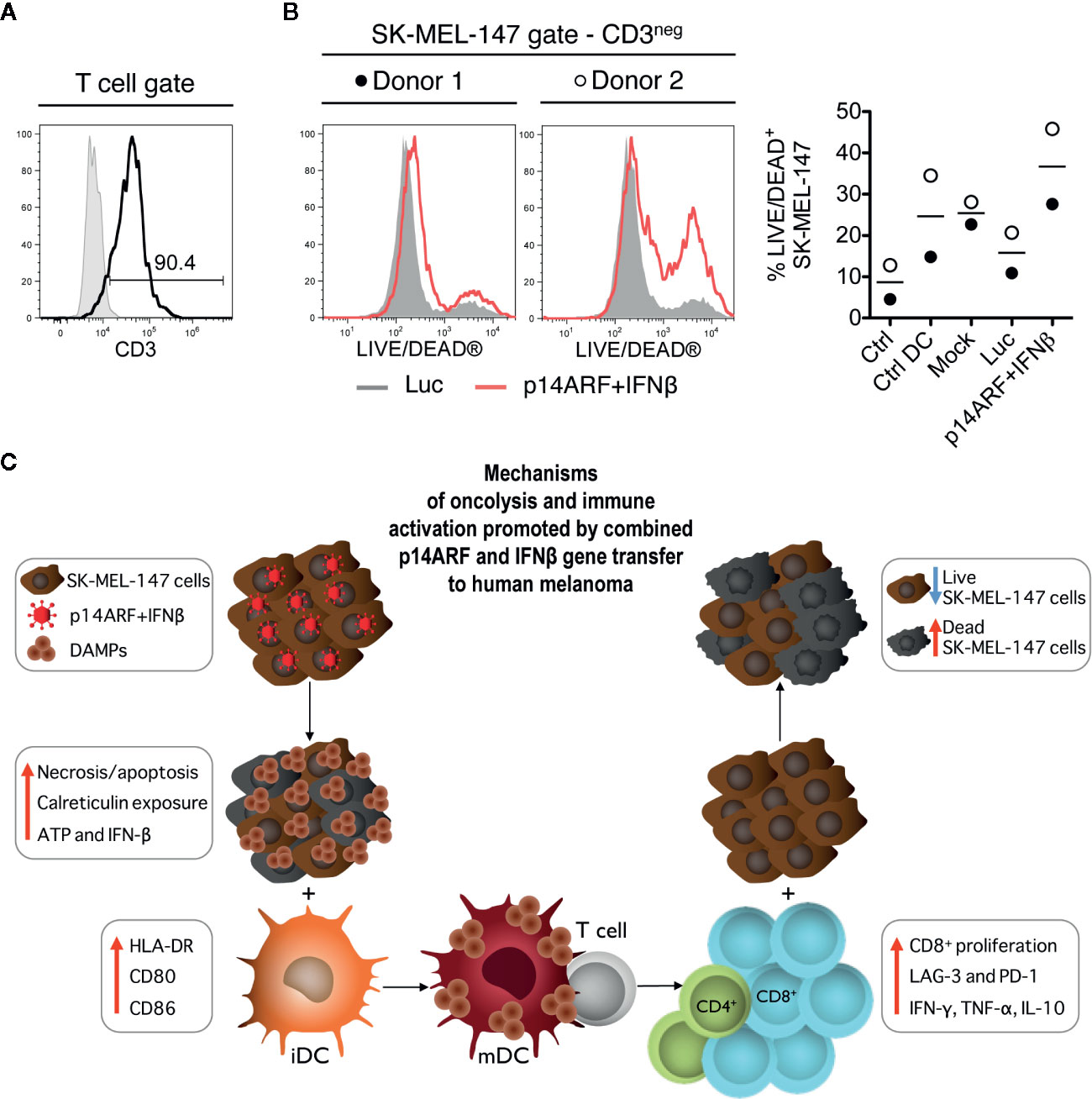
Figure 6 T cells primed by iDCs activated with p14ARF+IFNβ-transduced SK-MEL-147 cells induced viability loss of fresh non-transduced SK-MEL-147 cells. T cells primed with autologous DCs (previously activated with transduced SK-MEL-147 cells) from two healthy donors were challenged with fresh SK-MEL-147 cells to assess viability 48 h later. (A) Representative histogram of the frequency of CD3+ T cells, which comprised over 90% of the cell population among the lymphocyte gate (FSClo). (B) Histograms showing the viability of SK-MEL-147 (CD3neg FSChi) after challenge with previously primed T cells. For both donors, there was an increase in dead cells triggered by the p14ARF + IFNβ combination group compared to the controls. (C) Schematic representation of the mechanisms of oncolysis and immune activation triggered by p14ARF + IFNβ gene therapy of melanoma cells.
Discussion
The classic conception of cancer therapy involves the use of chemotherapy and radiotherapy to induce cell death preferentially in tumor populations (44, 45). Frequently, these agents induce apoptosis, a well-known mechanism of cell death that involves the activation of caspases 3 and 7, fragmentation of DNA and packaging of cell content within portions of integral cell membrane (46–49). The maintenance of membrane integrity is an important feature of programmed cell death since this does not alarm the immune system (50). However, apoptosis may activate the proliferation of the remaining cells and thus reestablish the tumor mass (46, 51); therefore, alternative approaches that induce tumor cell death are needed.
In this paper, we constructed novel adenoviral vectors expressing p14ARF and IFNβ and used them individually or in combination for the transduction of SK-MEL-147 cells. Interestingly, our results show that the combination of p14ARF and IFNβ adenoviruses induced cell death independent of caspases3/7, unlike the IFNβ vector when used alone, corroborating other findings of our group when using mouse (21) or human cell lines (SM, OC, and BS, unpublished data).
The use of animal models to test in situ cancer gene therapy is an approach that allows us to make valuable inferences. Our group has accumulated evidence from previous studies that show adenoviral vectors, when injected intratumorally, are well tolerated by animals (20, 22, 52). Serum levels of ALT and AST did not show significant changes in a model of in situ gene therapy where only the IFNβ vector was administered, thus hepatotoxicity did not occur (53).
In this work, we investigated the potential antitumor effects of AdRGD-PG-IFNβ and AdRGD-PG-p14ARF in a model of in situ gene therapy using the human melanoma cell line SK-MEL-147 engrafted in Nod-Scid mice. Our results indicate that IFNβ gene transfer alone or when combined with p14ARF conferred potent inhibition of tumor progression, which directly reflects on the survival of these animals. In agreement with the in vitro cell death assays, p14ARF alone was less effective. The in vivo performance of our gene transfer approach corroborates with previous work (20, 22, 52), but nevertheless, it is unprecedented since this is the first demonstration of the use of a p53-responsive adenoviral vector for the transfer of p14ARF+IFNβ to human melanoma cells. Although the results were encouraging, the use of human cells in animal models brings an important limitation, the use of immunocompromised animals. Our previous data using the B16 cell line in immunocompetent mice pointed out that the contribution of the immune system to the control of tumors treated with the mouse transgenes (p19Arf + mIFNβ) is crucial (18, 20, 52).
One of our main motivations for the use of gene transfer to promote oncolysis was precisely the potential to modulate the tumor microenvironment, release tumor antigens, and DAMPs to stimulate the immune response. To this end, we investigated the occurrence of ICD. In this work, we focus on the exposure of calreticulin (54) and secretion of ATP (55) in response to gene transfer. Considered an early event of ICD, exposure of calreticulin on the cell surface acts as an “eat-me” signal for phagocytosis by macrophages, neutrophils, and DCs and is necessary for subsequent antigen cross-presentation to cytotoxic T cells (54, 56). Our data indicate that combined p14ARF+IFNβ gene transfer was the strongest inducer of ICD as measured by the release of DAMPS and motivated us to focus only on this group to continue the work. There are an increasing number of markers for characterizing ICD, including type I interferon (9, 10). Although we did not focus on expanding the number of ICD markers, we consider that the production of IFNβ encoded by the adenoviral vectors contribute to the ICD process.
Next, we characterized the immunogenic response itself, through assays with DCs and T cells. Our data suggest that the DAMPs and tumor antigens produced during the ICD of SK-MEL-147 cells upon transduction with p14ARF+IFNβ were able to activate iDCs, which in turn primed T cells to produce IFN-γ, TNF-α, and IL-10, and could also induce a specific cytotoxic population recognizing and killing SK-MEL-147 cells. In iDCs exposed to the factors induced by p14ARF+IFNβ, soluble factors, such as ATP and IFNβ, may be sufficient for the upregulation of HLA-DR, whereas calreticulin exposure and other contact-dependent factors are needed in addition to soluble factors to significantly increase the expression of the co-stimulatory molecules CD80 and CD86. Extracellular ATP released by dying cells guides the recruitment of antigen-presenting cells and promote debris uptake and clearance (54, 55, 57, 58) that in an IFN-β milieu, will increase the expression of interferon-stimulated genes, sustain MHC class II synthesis, antigen-processing and presenting capacity (59). The effect of calreticulin exposure, in turn, relies on the contact between iDCs and dying cells and the blockage of this pathway abolishes the immunogenicity of cell death (54, 60), perhaps by modulating the expression of co-stimulatory molecules in activated DCs as we observed in our transwell experiments. Indeed, the lack of contact between dying cells and iDCs (a.k.a. calreticulin-exposure signaling) also affects the subsequent polarization of T cells, which fail to improve their cytokine-producing capacity.
We explored antigen dosage by varying the number of transduced-SK-MEL-147 cells cultured with iDCs. While dosage had no significant impact on HLA-DR, CD80, and CD86 expression or the ability to induce cytokine-production by T cells, it appears that less antigen was better for the activation of DCs, particularly relevant when it comes to the induction of viable memory antigen-specific CD8+ T cells without exhausted phenotype (61, 62), that in our system remains to be investigated. Preliminary evidence obtained from four healthy donors show that iDCs activated with both Luc and p14ARF+IFNβ-transduced SK-MEL-147 cells were biased to induce the proliferation of CD8+ T cells, due to the adenoviral-transduction itself and not necessarily by p14ARF+IFNβ. However, only p14ARF+IFNβ was able to polarize the cytokine milieu toward a Th1 profile, suggesting that our combination could effectively trigger a desirable cytokine setting for an antitumor response (63).
The upregulation of checkpoint molecules in CD8+ T cells has been proposed to distinguish tumor-specific clones that had recent TCR activation (64). The transient invigoration of exhausted CD8+ T cells to potent tumor-reactive cells has been achieved through the combination of anti-PD-1 therapy with PEGylated IL-10, leading to the expansion of a rare population of LAG-3+ PD-1+ CD8+ T cells that positively correlates with clinical response (65). Intriguingly, iDCs activated with p14ARF+IFNβ-transduced SK-MEL-147 cells favored the upregulation of LAG-3 and PD-1 in CD8+ T cells simultaneously with IL-10 production; the significance of this phenomena to our strategy is unknown but noteworthy since one of the biggest challenges of modern immunotherapy strategies is the induction of potent antitumor response without the adverse events arising from an uncontrolled immune response. Future studies of our strategy will address the kinetics of cytotoxic and exhausted CD8+ T cell populations and the significance of the simultaneous checkpoint molecule and IL-10 upregulation.
Beyond the phenotype and function of the CD209+ monocyte-derived DCs characterized here, DAMPs and ICD triggered by p14ARF+IFNβ could also influence other circulating DC populations potentially present in the adherent and non-adherent fractions, sources of monocytes and T cells. Of note, plasmacytoid DCs (pDC) with its remarkable antiviral capacity and production of type I interferons, slanDCs the major source of IL-12 that favor Th1 responses and cDC1 CD141+, specialists in antigen cross-presentation to CD8+ T cells (66, 67). Each subpopulation, that substantially differ in terms of function, might also have important differences in their capacity to internalize calreticulin-exposing dying cells, the repertoire of pattern recognition receptors (PRRs) recognizing p14ARF+IFNβ-induced DAMPs, as well as signaling toward inflammatory and anti-inflammatory cytokines and ability to polarize adaptive responses (68, 69). Also, by our methods, we could not rule out the participation of other immune cells, such as NK cells; previous in vivo work from our group points to the participation of NK cells in our strategy and poorer antitumor effect after in situ IFNβ-vector treatment of SK-MEL-147 tumors in Nod-Scid mice versus nude (OC and BS, manuscript submitted). In a mouse model, we have observed activation of ULBP1, IL-15, Killer/DR5 and Fas/Apo1 in B16 tumor cells specifically in response to combined p19Arf+mIFNβ gene transfer (52). Additional studies are needed to understand the participation of other lymphocyte populations, such as CD4+ helper T cells and NK cells in the mechanisms described here.
In summary, we present evidence that p14ARF+IFNβ-transduction of human melanoma cells leads to ICD that activates iDCs, which in turn, induce and polarize the adaptive immune response toward a Th1 profile. Data from our experiments with T cells suggest that the expansion of antigen-specific CD8+ T cells would be expected to participate in controlling tumor progression in vivo. Based on our in vitro proof of concept data, further exploration of immune cell activation using healthy donors or, ideally, from melanoma patients is warranted.
Data Availability Statement
The raw data supporting the conclusions of this article will be made available by the authors, without undue reservation.
Ethics Statement
The studies involving human participants were reviewed and approved by Comite de Etica em Pesquisa, Pró-Sangue, Faculdade de Medicina, Universidade de Sao Paulo. Written informed consent for participation was obtained for this study in accordance with the national legislation and the institutional requirements. The animal study was reviewed and approved by Comite de Etica em Uso de Animais (CEUA), Faculdade de Medicina, Universidade de Sao Paulo.
Author Contributions
OC: experimental design, coordinated assays, in situ therapy in animal models, in vitro co-culture of tumor and immune cells experiments, western-blot, immunofluorescence, microscopy, data analysis, and manuscript writing. MC-S: design of immunological assays, co-culture of tumor and immune cells experiments, ELISA, flow cytometry, data analysis, and manuscript writing. EC: co-culture of tumor and immune cells experiments, CBA, flow cytometry, data analysis, and manuscript writing. TC: hypodiploid assays, flow cytometry analysis, and manuscript writing. SM: adenovirus construction and production. JB: design of immunological assays and data interpretation. BS: coordinated supporting grants, experimental design, data interpretation, manuscript writing and editing. All authors contributed to the article and approved the submitted version.
Funding
This work was supported by the Sao Paulo Research Foundation (FAPESP) grant 2015/26580-9 (BS), post-doctoral fellowships 2017/23068-0 (OC) and 2017/13686-9 (MC-S), and Conselho Nacional de Desenvolvimento Científico e Tecnológico (CNPq) fellowship 302888/2017-9 (BS).
Conflict of Interest
The authors declare that the research was conducted in the absence of any commercial or financial relationships that could be construed as a potential conflict of interest.
Acknowledgments
The authors thank Roger Chammas (ICESP-FMUSP) for unwavering support, Mara Junqueira (Centro de Medicina Nuclear-FMUSP) for assistance with the in vivo assays, and Carolina C. Salomón for scientific illustration.
Supplementary Material
The Supplementary Material for this article can be found online at: https://www.frontiersin.org/articles/10.3389/fimmu.2020.576658/full#supplementary-material
References
1. Hanahan D, Weinberg RA. Hallmarks of cancer: the next generation. Cell (2011) 144(5):646–74. doi: 10.1016/j.cell.2011.02.013
2. Schreiber RD, Old LJ, Smyth MJ. Cancer immunoediting: integrating immunity’s roles in cancer suppression and promotion. Science (2011) 331(6024):1565–70. doi: 10.1126/science.1203486
3. Oleinika K, Nibbs RJ, Graham GJ, Fraser AR. Suppression, subversion and escape: the role of regulatory T cells in cancer progression. Clin Exp Immunol (2013) 171(1):36–45. doi: 10.1111/j.1365-2249.2012.04657.x
4. Choi AH, O’Leary MP, Fong Y, Chen NG. From benchtop to bedside: a review of oncolytic virotherapy. Biomedicines (2016) 4(3):18. doi: 10.3390/biomedicines4030018
5. Filley AC, Dey M. Immune system, friend or foe of oncolytic virotherapy? Front Oncol (2017) 7:106. doi: 10.3389/fonc.2017.00106
6. Kaufman HL, Kohlhapp FJ, Zloza A. Oncolytic viruses: a new class of immunotherapy drugs. Nat Rev Drug Discovery (2015) 14(9):642–62. doi: 10.1038/nrd4663
7. Vähä-Koskela MJV, Heikkilä JE, Hinkkanen AE. Oncolytic viruses in cancer therapy. Cancer Lett (2007) 254(2):178–216. doi: 10.1016/j.canlet.2007.02.002
8. Davola ME, Mossman KL. Oncolytic viruses: how “lytic” must they be for therapeutic efficacy? Oncoimmunology (2019) 8(6):e1581528. doi: 10.1080/2162402X.2019.1596006
9. Galluzzi L, Vitale I, Aaronson SA, Abrams JM, Adam D, Agostinis P, et al. Molecular mechanisms of cell death: recommendations of the Nomenclature Committee on Cell Death 2018. Cell Death Different (2018) 25(3):486–541. doi: 10.1038/s41418-017-0012-4
10. Galluzzi L, Vitale I, Warren S, Adjemian S, Agostinis P, Martinez AB, et al. Consensus guidelines for the definition, detection and interpretation of immunogenic cell death. J Immunother Cancer (2020) 8(1):e000337. doi: 10.1136/jitc-2019-000337corr1
11. Kroemer G, Galluzzi L, Kepp O, Zitvogel L. Immunogenic Cell Death in Cancer Therapy. Annu Rev Immunol (2013) 31(1):51–72. doi: 10.1146/annurev-immunol-032712-100008
12. Marelli G, Howells A, Lemoine NR, Wang Y. Oncolytic viral therapy and the immune system: a double-edged sword against cancer. Front Immunol (2018) 9:866. doi: 10.3389/fimmu.2018.00866
13. Gujar S, Pol JG, Kim Y, Lee PW, Kroemer G. Antitumor benefits of antiviral immunity: an underappreciated aspect of oncolytic virotherapies. Trends Immunol (2018) 39(3):209–21. doi: 10.1016/j.it.2017.11.006
14. Lemos de Matos A, Franco LS, McFadden G. Oncolytic Viruses and the Immune System: The Dynamic Duo. Mol Ther Methods Clin Dev (2020) 17:349–58. doi: 10.1016/j.omtm.2020.01.001
15. Haanen JB. Converting cold into hot tumors by combining immunotherapies. Cell (2017) 170(6):1055–6. doi: 10.1016/j.cell.2017.08.031
16. Fukuhara H, Ino Y, Todo T. Oncolytic virus therapy: A new era of cancer treatment at dawn. Cancer Sci (2016) 107(10):1373–9. doi: 10.1111/cas.13027
17. Shirley JL, de Jong YP, Terhorst C, Herzog RW. Immune Responses to Viral Gene Therapy Vectors. Mol Ther J Am Soc Gene Ther (2020) 28(3):709–22. doi: 10.1016/j.ymthe.2020.01.001
18. Strauss BE, Silva GRO, de Luna Vieira I, Cerqueira OLD, Del Valle PR, Medrano RFV, et al. Perspectives for cancer immunotherapy mediated by p19Arf plus interferon-beta gene transfer. Clinics (Sao Paulo Brazil) (2018) 73(suppl 1):e479s. doi: 10.6061/clinics/2018/e479s
19. Medrano RFV, Hunger A, Mendonca SA, Barbuto JAM, Strauss BE. Immunomodulatory and antitumor effects of type I interferons and their application in cancer therapy. Oncotarget (2017) 8(41):71249–84. doi: 10.18632/oncotarget.19531
20. Hunger A, Medrano RF, Strauss BE. Harnessing combined p19Arf and interferon-beta gene transfer as an inducer of immunogenic cell death and mediator of cancer immunotherapy. Cell Death Dis (2017) 8(5):e2784. doi: 10.1038/cddis.2017.201
21. Hunger A, Medrano RF, Zanatta DB, Del Valle PR, Merkel CA, Salles TA, et al. Reestablishment of p53/Arf and interferon-beta pathways mediated by a novel adenoviral vector potentiates antiviral response and immunogenic cell death. Cell Death Discov (2017) 3:17017. doi: 10.1038/cddiscovery.2017.17
22. Catani JP, Medrano RF, Hunger A, Del Valle P, Adjemian S, Zanatta DB, et al. Intratumoral Immunization by p19Arf and Interferon-beta Gene Transfer in a Heterotopic Mouse Model of Lung Carcinoma. Transl Oncol (2016) 9(6):565–74. doi: 10.1016/j.tranon.2016.09.011
23. Qin XQ, Beckham C, Brown JL, Lukashev M, Barsoum J. Human and mouse IFN-beta gene therapy exhibits different anti-tumor mechanisms in mouse models. Mol Ther J Am Soc Gene Ther (2001) 4(4):356–64. doi: 10.1006/mthe.2001.0464
24. Lomas A, Leonardi-Bee J, Bath-Hextall F. A systematic review of worldwide incidence of nonmelanoma skin cancer. Br J Dermatol (2012) 166(5):1069–80. doi: 10.1111/j.1365-2133.2012.10830.x
25. Balch CM, Buzaid AC, Soong S-J, Atkins MB, Cascinelli N, Coit DG, et al. Final version of the American Joint Committee on Cancer staging system for cutaneous melanoma. J Clin Oncol (2001) 19(16):3635–48. doi: 10.1200/JCO.2001.19.16.3635
26. Giglia-Mari G, Sarasin A. TP53 mutations in human skin cancers. Hum Mutat (2003) 21(3):217–28. doi: 10.1002/humu.10179
27. Merkel CA, da Silva Soares RB, de Carvalho ACV, Zanatta DB, Bajgelman MC, Fratini P, et al. Activation of endogenous p53 by combined p19Arf gene transfer and nutlin-3 drug treatment modalities in the murine cell lines B16 and C6. BMC Cancer (2010) 10(1):316. doi: 10.1186/1471-2407-10-316
28. Sandoval R, Xue J, Pilkinton M, Salvi D, Kiyokawa H, Colamonici OR. Different requirements for the cytostatic and apoptotic effects of type I interferons. Induction of apoptosis requires ARF but not p53 in osteosarcoma cell lines. J Biol Chem (2004) 279(31):32275–80. doi: 10.1074/jbc.M313830200
29. Takaoka A, Hayakawa S, Yanai H, Stoiber D, Negishi H, Kikuchi H, et al. Integration of interferon-alpha/beta signalling to p53 responses in tumour suppression and antiviral defence. Nature (2003) 424(6948):516–23. doi: 10.1038/nature01850
30. Sharpless E, Chin L. The INK4a/ARF locus and melanoma. Oncogene (2003) 22(20):3092–8. doi: 10.1038/sj.onc.1206461
31. James CD, He J, Carlbom E, Nordenskjold M, Cavenee WK, Collins VP. Chromosome 9 deletion mapping reveals interferon alpha and interferon beta-1 gene deletions in human glial tumors. Cancer Res (1991) 51(6):1684–8.
32. Miyakoshi J, Dobler KD, Allalunis-Turner J, McKean JD, Petruk K, Allen PB, et al. Absence of IFNA and IFNB genes from human malignant glioma cell lines and lack of correlation with cellular sensitivity to interferons. Cancer Res (1990) 50(2):278–83.
33. Fountain JW, Karayiorgou M, Ernstoff MS, Kirkwood JM, Vlock DR, Titus-Ernstoff L, et al. Homozygous deletions within human chromosome band 9p21 in melanoma. Proc Natl Acad Sci (1992) 89(21):10557–61. doi: 10.1073/pnas.89.21.10557
34. Mizuguchi H, Koizumi N, Hosono T, Utoguchi N, Watanabe Y, Kay MA, et al. A simplified system for constructing recombinant adenoviral vectors containing heterologous peptides in the HI loop of their fiber knob. Gene Ther (2001) 8(9):730–5. doi: 10.1038/sj.gt.3301453
35. Peng HH, Wu S, Davis JJ, Wang L, Roth JA, Marini III FC, et al. A rapid and efficient method for purification of recombinant adenovirus with arginine–glycine–aspartic acid-modified fibers. Analyt Biochem (2006) 354(1):140–7. doi: 10.1016/j.ab.2006.04.032
36. Tamura RE, Hunger A, Fernandes DC, Laurindo FR, Costanzi-Strauss E, Strauss BE. Induction of Oxidants Distinguishes Susceptibility of Prostate Carcinoma Cell Lines to p53 Gene Transfer Mediated by an Improved Adenoviral Vector. Hum Gene Ther (2017) 28(8):639–53. doi: 10.1089/hum.2016.139
37. Cerqueira OL, Truesdell P, Baldassarre T, Vilella-Arias SA, Watt K, Meens J, et al. CIP4 promotes metastasis in triple-negative breast cancer and is associated with poor patient prognosis. Oncotarget (2015) 6(11):9397–408. doi: 10.18632/oncotarget.3351
38. Merkel CA, Medrano RF, Barauna VG, Strauss BE. Combined p19Arf and interferon-beta gene transfer enhances cell death of B16 melanoma in vitro and in vivo. Cancer Gene Ther (2013) 20(5):317–25. doi: 10.1038/cgt.2013.23
39. Tamura RE, Lana MG, Costanzi-Strauss E, Strauss BE. Combination of cabazitaxel and p53 gene therapy abolishes prostate carcinoma tumor growth. Gene Ther (2020) 27:15–26. doi: 10.1038/s41434-019-0071-x
40. Tomayko MM, Reynolds CP. Determination of subcutaneous tumor size in athymic (nude) mice. Cancer Chemother Pharmacol (1989) 24(3):148–54. doi: 10.1007/BF00300234
41. Ito Y, Shinomiya K. A new continuous-flow cell separation method based on cell density: principle, apparatus, and preliminary application to separation of human buffy coat. J Clin Apher (2001) 16(4):186-91. doi: 10.1002/jca.1032
42. Sallusto F, Lanzavecchia A. Efficient presentation of soluble antigen by cultured human dendritic cells is maintained by granulocyte/macrophage colony-stimulating factor plus interleukin 4 and downregulated by tumor necrosis factor alpha. J Exp Med (1994) 179(4):1109–18. doi: 10.1084/jem.179.4.1109
43. CECHIM G, J. A. B. CHIES. In vitro generation of human monocyte-derived dendritic cells methodological aspects in a comprehensive review. An Acad Bras Cienc (2019) 91(4):e20190310. doi: 10.1590/0001-3765201920190310
44. Strebhardt K, Ullrich A. Paul Ehrlich’s magic bullet concept: 100 years of progress. Nat Rev Cancer (2008) 8(6):473–80. doi: 10.1038/nrc2394
45. Sánchez NS, Mills GB, Mills Shaw KR. Precision oncology: neither a silver bullet nor a dream. Pharmacogenomics (2017) 18(16):1525–39. doi: 10.2217/pgs-2017-0094
46. Huang Q, Li F, Liu X, Li W, Shi W, Liu F-F, et al. Caspase 3–mediated stimulation of tumor cell repopulation during cancer radiotherapy. Nat Med (2011) 17(7):860. doi: 10.1038/nm.2385
47. Kaufmann SH, Earnshaw WC. Induction of apoptosis by cancer chemotherapy. Exp Cell Res (2000) 256(1):42–9. doi: 10.1006/excr.2000.4838
48. Ricci MS, Zong W-X. Chemotherapeutic approaches for targeting cell death pathways. Oncol (2006) 11(4):342. doi: 10.1634/theoncologist.11-4-342
49. Alison MR, Sarraf CE. Apoptosis: a gene-directed programme of cell death. J R Coll Physicians London (1992) 26(1):25–35.
50. Green D, Oguin T, Martinez J. The clearance of dying cells: table for two. Cell Death Different (2016) 23(6):915–26. doi: 10.1038/cdd.2015.172
51. Li F, Huang Q, Chen J, Peng Y, Roop DR, Bedford JS, et al. Apoptotic cells activate the “phoenix rising” pathway to promote wound healing and tissue regeneration. Sci Signal (2010) 3(110):ra13–ra. doi: 10.1126/scisignal.2000634
52. Medrano RF, Catani JP, Ribeiro AH, Tomaz SL, Merkel CA, Costanzi-Strauss E, et al. Vaccination using melanoma cells treated with p19arf and interferon beta gene transfer in a mouse model: a novel combination for cancer immunotherapy. Cancer Immunol Immunother (2016) 65(4):371–82. doi: 10.1007/s00262-016-1807-8
53. David TIP, Cerqueira OLD, Lana MG, Medrano RVF, Hunger A, Strauss BE, et al. Response of human melanoma cell lines to interferon-beta genetransfer mediated by a modified adenoviral vector. Sci Rep (2020). doi: 10.1038/s41598-020-74826-y
54. Obeid M, Tesniere A, Ghiringhelli F, Fimia GM, Apetoh L, Perfettini JL, et al. Calreticulin exposure dictates the immunogenicity of cancer cell death. Nat Med (2007) 13(1):54–61. doi: 10.1038/nm1523
55. Elliott MR, Chekeni FB, Trampont PC, Lazarowski ER, Kadl A, Walk SF, et al. Nucleotides released by apoptotic cells act as a find-me signal to promote phagocytic clearance. Nature (2009) 461(7261):282–6. doi: 10.1038/nature08296
56. Panaretakis T, Kepp O, Brockmeier U, Tesniere A, Bjorklund AC, Chapman DC, et al. Mechanisms of pre-apoptotic calreticulin exposure in immunogenic cell death. EMBO J (2009) 28(5):578–90. doi: 10.1038/emboj.2009.1
57. Idzko M, Dichmann S, Ferrari D, Di Virgilio F, la Sala A, Girolomoni G, et al. Nucleotides induce chemotaxis and actin polymerization in immature but not mature human dendritic cells via activation of pertussis toxin-sensitive P2y receptors. Blood (2002) 100(3):925–32. doi: 10.1182/blood.V100.3.925
58. Ma Y, Adjemian S, Mattarollo SR, Yamazaki T, Aymeric L, Yang H, et al. Anticancer chemotherapy-induced intratumoral recruitment and differentiation of antigen-presenting cells. Immunity (2013) 38(4):729–41. doi: 10.1016/j.immuni.2013.03.003
59. Simmons DP, Wearsch PA, Canaday DH, Meyerson HJ, Liu YC, Wang Y, et al. Type I IFN drives a distinctive dendritic cell maturation phenotype that allows continued class II MHC synthesis and antigen processing. J Immunol (Baltimore Md 1950) (2012) 188(7):3116–26. doi: 10.4049/jimmunol.1101313
60. Zitvogel L, Kepp O, Senovilla L, Menger L, Chaput N, Kroemer G. Immunogenic tumor cell death for optimal anticancer therapy: the calreticulin exposure pathway. Clin Cancer Res Off J Am Assoc Cancer Res (2010) 16(12):3100–4. doi: 10.1158/1078-0432.CCR-09-2891
61. Dieckmann D, Schultz ES, Ring B, Chames P, Held G, Hoogenboom HR, et al. Optimizing the exogenous antigen loading of monocyte-derived dendritic cells. Int Immunol (2005) 17(5):621–35. doi: 10.1093/intimm/dxh243
62. Steffensen MA, Holst PJ, Steengaard SS, Jensen BAH, Bartholdy C, Stryhn A, et al. Qualitative and Quantitative Analysis of Adenovirus Type 5 Vector-Induced Memory CD8 T Cells: Not as Bad as Their Reputation. J Virol (2013) 87(11):6283–95. doi: 10.1128/JVI.00465-13
63. Kemp RA, Ronchese F. Tumor-specific Tc1, but not Tc2, cells deliver protective antitumor immunity. J Immunol (Baltimore Md 1950) (2001) 167(11):6497–502. doi: 10.4049/jimmunol.167.11.6497
64. Gros A, Parkhurst MR, Tran E, Pasetto A, Robbins PF, Ilyas S, et al. Prospective identification of neoantigen-specific lymphocytes in the peripheral blood of melanoma patients. Nat Med (2016) 22(4):433–8. doi: 10.1038/nm.4051
65. Naing A, Infante JR, Papadopoulos KP, Chan IH, Shen C, Ratti NP, et al. PEGylated IL-10 (Pegilodecakin) Induces Systemic Immune Activation, CD8(+) T Cell Invigoration and Polyclonal T Cell Expansion in Cancer Patients. Cancer Cell (2018) 34(5):775–91.e3. doi: 10.1016/j.ccell.2018.10.007
66. Villani A-C, Satija R, Reynolds G, Sarkizova S, Shekhar K, Fletcher J, et al. Single-cell RNA-seq reveals new types of human blood dendritic cells, monocytes, and progenitors. Science (2017) 356(6335):eaah4573. doi: 10.1126/science.aah4573
67. Hansel A, Gunther C, Ingwersen J, Starke J, Schmitz M, Bachmann M, et al. Human slan (6-sulfo LacNAc) dendritic cells are inflammatory dermal dendritic cells in psoriasis and drive strong TH17/TH1 T-cell responses. J Allergy Clin Immunol (2011) 127(3):787–94.e1-9. doi: 10.1016/j.jaci.2010.12.009
68. Wemeau M, Kepp O, Tesnière A, Panaretakis T, Flament C, De Botton S, et al. Calreticulin exposure on malignant blasts predicts a cellular anticancer immune response in patients with acute myeloid leukemia. Cell Death Dis (2010) 1(12):e104–e. doi: 10.1038/cddis.2010.82
Keywords: melanoma, adenovirus (Ad) vector, oncolysis, immunogenic cell death (ICD), immunotherapy
Citation: Cerqueira OLD, Clavijo-Salomon MA, Cardoso EC, Citrangulo Tortelli Junior T, Mendonça SA, Barbuto JAM and Strauss BE (2020) Combined p14ARF and Interferon-β Gene Transfer to the Human Melanoma Cell Line SK-MEL-147 Promotes Oncolysis and Immune Activation. Front. Immunol. 11:576658. doi: 10.3389/fimmu.2020.576658
Received: 26 June 2020; Accepted: 25 September 2020;
Published: 22 October 2020.
Edited by:
Peter Brossart, University of Bonn, GermanyReviewed by:
Guilan Shi, University of South Florida, United StatesRamon Kaneno, São Paulo State University, Brazil
Copyright © 2020 Cerqueira, Clavijo-Salomon, Cardoso, Citrangulo Tortelli Junior, Mendonça, Barbuto and Strauss. This is an open-access article distributed under the terms of the Creative Commons Attribution License (CC BY). The use, distribution or reproduction in other forums is permitted, provided the original author(s) and the copyright owner(s) are credited and that the original publication in this journal is cited, in accordance with accepted academic practice. No use, distribution or reproduction is permitted which does not comply with these terms.
*Correspondence: Bryan E. Strauss, YnN0cmF1c3NAdXNwLmJy; YnJ5YW4uc3RyYXVzc0BoYy5mbS51c3AuYnI=
†Present address: Samir Andrade Mendonça, Department of Radiation Oncology, Washington University School of Medicine in St. Louis, MO, United States
‡These authors have contributed equally to this work