- 1Department of Dermatology, Second Xiangya Hospital, Central South University, Hunan Key Laboratory of Medical Epigenomics, Changsha, China
- 2Clinical Immunology Research Center, Central South University, Changsha, China
- 3Research Unit of Key Technologies of Diagnosis and Treatment for Immune-related Skin Diseases, Chinese Academy of Medical Sciences (2019RU027), Changsha, China
In the last two decades, extracellular vesicles (EVs) have aroused wide interest among researchers in basic and clinical research. EVs, small membrane vesicles are released by almost all kinds of cells into the extracellular environment. According to many recent studies, EVs participate in immunomodulation and play an important role in the pathogenesis of autoimmune diseases. In addition, EVs have great potential in the diagnosis and therapy of autoimmune diseases. Here, we reviewed the latest research advances on the functions and mechanisms of EVs and their roles in the pathogenesis, diagnosis, and treatment of rheumatoid arthritis and systemic lupus erythematosus.
Introduction
Rheumatoid arthritis (RA) and systemic lupus erythematosus (SLE) are both autoimmune diseases that can involve multiple organs. Their etiologies and pathogenesis are complex, and epigenetic and environmental factors are shown to be associated with the onset of the disease (1, 2). Glucocorticoids, immunosuppressants, and biological agents are commonly used in the treatment of RA and SLE, but problems such as toxic side effects and non-response to treatment remain (3–5). EVs are phospholipid bilayer-enclosed vesicles secreted from all cell types. The classification of EVs includes exosomes (<150 nm), microvesicles (150–1,000 nm) (6), and apoptotic bodies (1,000–5,000 nm), depending on size and biogenesis (7). EVs play an important role in cellular communication processes. In the past, intercellular communication was thought to have two modes, direct contact between cells and secretion of cellular molecules (8). The relationship between EVs and cellular communication has attracted more attention and has become the third mechanism of intercellular communication (9). EVs began to be isolated and studied from additional cell types, such as immune cells, nerve cells and tumor cells (10). It is demonstrated that EVs are involved as carriers in intercellular communication by transporting lipids, proteins, and other components (11). In 1996, Raposo et al. first showed that EVs could stimulate adaptive immune responses (12). EVs can also carry mitochondria to regulate immunity and alter the phenotype of macrophages (13).
EVs are secreted by almost every functional cell type and have the characteristics of easy detection and stability. Body fluids, such as urine and peripheral blood contain EVs, which present promising prospects as biomarkers for tumors, infectious diseases, and autoimmune diseases. Furthermore, the biological characteristics of EVs that can transport multiple cellular components also make it possible to use them in therapeutic approaches for diseases. To date, there have been some studies of EV treatment for RA and SLE, which have made certain achievements (14, 15). Here, we summarize the functions of EVs on immune cells and their applications in the pathogenesis, diagnosis and treatment of RA and SLE.
The Biogenesis and Composition of EVs
Different types of EVs have slightly different biological origins, and their biological functions are determined by their respective intercellular components. Exosomes are EVs with a diameter of no more than 150 nm. The limiting membrane of late endosomes generates exosomes by invagination and budding (16). Then, exosomes are covered by endosomal multivesicular bodies (MVBs) and form intraluminal vesicles (ILVs), which fuse with the plasma membrane and are exocrine. The endosomal sorting complex required for transport (ESCRT) is also involved in exosome generation, which is formed by approximately twenty proteins (17). The action of ESCRT is mainly carried out by four proteins following specific steps. First, ESCRT-0 recognizes ubiquitinated proteins in the endosomal membrane and isolates them individually. Second, both ESCRT-I and ESCRT-II mediate the transformation and assembly of the membrane. Third, ESCRT-III leads to the scission (18). Exosomes contain proteins, nucleic acids, lipids, and organelles such as mitochondria (19).
Microvesicles (MVs) are formed as the plasma membrane germinates outward directly. Although the diameter-based classification of exosomes and MVs is somewhat controversial, the fundamental distinction is apparent based on their biogenesis. Their formation is related to changes in the symmetry of phospholipids in cell membranes, and their release is associated with lipid rafts on the cell membrane (20). Proteins and phospholipids are unevenly distributed on the plasma membrane by the regulation of aminophospholipid translocases. The transfer of phosphatidylserine and the change in protein structure create a dynamic equilibrium, contributing to the formation of MVs (21). MVs are composed similarly to exosomes.
Apoptotic bodies are the products of apoptosis, while exosomes and MVs are secreted by living cells. The contents of the cell after apoptosis decompose into membrane-bound vesicles. In terms of composition, apoptotic bodies are characterized by the inclusion of organelles and smaller vesicles (21). Apoptotic bodies also contain ribosomal RNA which are almost undetectable in exosomes and MVs (22). They work primarily as garbage carriers of cells containing cellular wastes (23).
The Role and Mechanism of EVs in The Immune System
Research on EVs began in 1983 when exosomes were first identified in reticulocytes from sheep (24). However, it was not until 1996 that B cells were shown to release exosomes with the major histocompatibility complex class II (MHC II), which indicated the relationship between immune cell regulation and exosomes (12). Other immunocytes, such as T cells, natural killer (NK) cells, and dendritic cells (DCs), have been proven to be associated with EVs in recent publications (25–27). Since EVs, especially exosomes, can carry MHC II, it is possible for EVs to participate in antigen presentation. Tian et al. summarized three mechanisms by which EVs are involved in antigen presentation (7). First, loading antigen proteins inside exosomes improves the efficiency of antigen presentation, and then APCs costimulating molecules act on the activation of T cells (28). Second, when peptide/MHC complexes are formed, exosomes with antigens can be captured by APCs and then are exposed to the cell membrane to activate T cells. Third, EVs directly activate T cells without the participation of APCs (29). Interestingly, reverse transport of miRNA by exosomes, which is antigen-driven, has been proven to regulate the gene expression of APCs (30).
DCs are one of the most effective immunocytes in presenting antigens and are critical to both innate and adaptive immunity. Some DCs can establish immune tolerance by reducing the T cell activity level, while the other DCs can activate T cells to enhance the immune response. With the expression of a high level of MHC I/peptide complexes as well as B7 and ICAM-1, exosomes from DCs are able to directly activate CD8+ T cells without the participation of normal APCs (31). Not only the mutual effect between DCs and T cells but also the intercellular communication between DCs play crucial roles in the process of DCs regulating innate immune responses (32). Angela Montecalvo et al. found that the miRNA components of exosomes released by DCs at different stages of maturation were different. Mature DC-derived exosomes show a stronger T-cell stimulatory ability than immature DC-derived exosomes because of higher expression of CD86 and CD54 (32). DC-derived exosomes (Dexs) containing MHC/peptide complexes can boost T cell-dependent tumor rejection. And NK cells can be activated by both IL-15Ra and NKG2D ligands in Dexs and secrete IFN-γ (33).
Similar to other APCs, B cells have a cellular structure called the endocytic compartment MIIC (major histocompatibility complex [MHC] class II-enriched compartment), which participates in the activation of antigen-specific MHC II-restricted T cell responses (12). Activated B cells infected with EBV can also excrete exosomes with EBV-miRNAs, which accumulate in neighboring primary immature monocyte-derived DCs (MoDCs) without infection (34). Furthermore, exosomes from activated B cells with EBV infection harbor the viral latent membrane protein 1 (LMP1), which imitates CD40 signaling, resulting in the propagation of B cells as well as T cell-independent class-switch recombination (35).
NK cells are important immunocytes in innate immunity with a variety of biological functions, including recognizing and killing viral infections and tumor cells, and producing cytokines such as interferon (IFN)-γ, involved in immune regulation (36). After being activated by Dexs, NK cells secrete exosomes containing CD63, fibronectin, perforin, granulysin, and granzyme A/B, which indicates that NK-derived EVs contain the killing function of NK cells (26). The EV interaction between APCs and T cells as well as NK cells is shown in Figure 1.
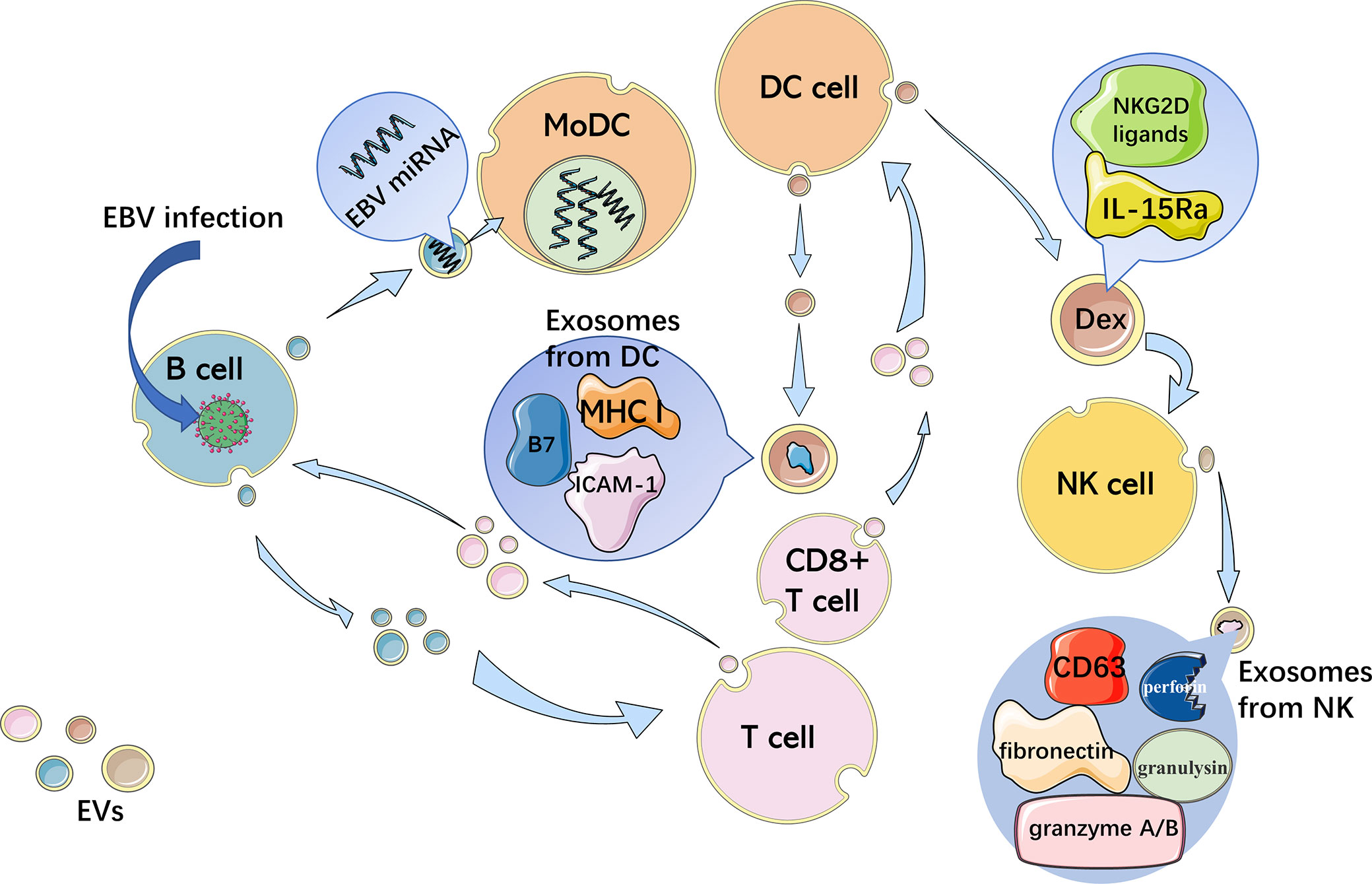
Figure 1 Interaction between immune cells through EVs. APCs activate T cells with EVs and reverse transport of miRNA by exosomes, which is antigen-driven, regulating the gene expression of APCs. B cells infected with EBV excrete exosomes with EBV-miRNAs, accumulating in neighboring MoDCs. Exosomes with MHC I/peptide complexes, B7 and ICAM-1 from DCs, directly activate CD8+ T cells. Dexs containing IL-15Ra and NKG2D ligands activate NK cells, which then secrete exosomes with CD63, fibronectin, perforin, granulysin, and granzyme A/B.
Other non-immune cells can also produce EVs and participate in the regulation of immune responses. EB virus (EBV) infected cells can secrete exosomes containing EBV-microRNAs (miRNA) to mediate gene silencing in immune cells (37). EVs derived from tumor cells and stem cells have also been shown to regulate immune function (38). miRNAs in tumor exosomes may induce immune tolerance (39). While EVs derived from stem cells have been shown to regulate immunity and reduce inflammatory responses (40, 41). Lipid-filled vesicles, derived by adipocytes, can modulate tissue macrophage to participate in immune regulation (42).
The Role of EVs in RA
EVs Are Involved in the Pathogenesis of RA
RA is an autoimmune disease with a high incidence that damages multiple joints throughout the body and can cause progressive disability. RA is characterized by synovial inflammation and cartilage destruction (43). In comparison with those from normal controls, EVs showed a high density in the synovial fluid of RA samples, which was associated with disease progression (44). EVs are mainly involved in antigen presentation, inflammatory cytokine and miRNA transmission, and activation of fibroblast-like synoviocytes (FLSs) in the pathological process of RA. It has also been shown that FLSs-derived EVs contained hexosaminidase D activities in the samples of RA patients (45). Additionally, the level of N-acetyl-beta-D-hexosaminidase (NAHase) in destructive RA is higher than that in inflammatory RA, indicating that glycosaminoglycan-degrading glycosidases may cause joint damage in RA (46). Citrullinated proteins can be detected in synovial exosomes, which can enhance T cell activity with fibronectin (47). In addition, antibodies to citrullinated protein antigens (ACPAs) are crucial in the pathological process of RA and are expected to work as biomarkers with the highest predictive value (48, 49).
FLS-derived microparticles (MPs) contain B cell stimulation factors in the synovial fluid of the joints of RA patients (50). There are microparticle-containing immune complexes (mpICs) in synovial fluid with CD41 highly expression, indicating platelet-derived. These mpICs could induce neutrophils to release leukotrienes, which proves that platelet mpICs are proinflammatory and highly reactive (51). Also, platelet-derived microparticles (MPs) seem to release IL-1β, which promotes joint inflammation by increasing the levels of IL-6 and IL-8 in fibroblasts from RA patients (52). Tumor necrosis factor-α (TNF-α) is crucial to the pathogenesis of RA. TNF-α binding membranes were detected in FLSs-derived EVs from RA patients. EVs containing TNF-α activated AKT and NF-κB and rendered these activated T cells resistant to apoptosis (53). Additionally, T cell-derived MPs treated with TNF-α could upregulate prostaglandin E2 (PGE2), microsomal prostaglandin E synthase 1 (mPGES-1) and cyclooxygenase 2 (COX-2) (54). Then COX2 caused pain and inflammation in patients. Coinhibitory T cell receptors can be expressed in cells from RA joints, including PD-1 and TIM-3. EVs from synovial fluid and T cells after cocultivation could express PD-1. Carrying the PD-1 receptor and inhibitive miRNAs, EVs may induce T cell exhaustion (55).
The transmission of miRNAs is crucial in the RA pathological process. Of all the miRNAs associated with RA, miR-155 and miR-146a have attracted most attention. A study proved that exosomal miR-146a and miR-155 are expressed at high levels in RA synovial tissue (56). Furthermore, miR-155 can be upregulated by stimulation with proinflammatory mediators, including Toll-like receptor (TLR) ligands, TNFα and IL-1β. Overexpression of miR-155 in RA synovial fibroblasts (RASFs) can downregulate matrix metalloproteinase 3 (MMP-3) and MMP-1 (57). MMP-3 is involved in the generation of severe cartilage damage (58). All these components in EVs contribute to the onset and development of RA (Table 1).
The Role of EVs in Diagnosis and Treatment of RA
Existing studies implied that EVs have potential as biomarkers for RA. RA patients with IgM-rheumatoid factor (RF) EVs showed high-level C-reactive protein (CRP) and Erythrocyte sedimentation rate (ESR) levels compared with those of RA patients without IgM-RF in EVs (63). Thus, EVs with IgM-RF can be used to distinguish between active and inactive RA. RA patients express high levels of MPs in the circulatory system compared with those in healthy controls (64). Moreover, as the role of miRNA in the pathology of RA disease has been revealed, exosomal miRNAs, including miR-155 and miR-146a, can be used for the early diagnosis of RA (57). Potential biomarkers for RA in EVs are summarized in Table 2.
Mesenchymal stem cells (MSCs) have anti-fibrosis and anti-inflammatory immune regulatory effects. The transplantation of MSCs has been used as a new technique for RA therapy. When collagen-induced arthritis (CIA) rats were treated with human umbilical cord MSCs (hUCMSCs), the results showed that hUCMSCs can reduce T lymphocyte activity and function, as well as inhibit Th17 cells and induce Treg cells to alleviate the disease (78). The same immunomodulatory function between hUCMSCs and hUCMSC-derived EVs has been demonstrated in vitro, which indicates the potential of hUCMSC-derived EVs as a new treatment for RA (78). hUCMSC-derived EVs also can inhibit the expression of IL-17 by downregulating Th17 cells and increasing the proportion of Treg cells in a dose-dependent manner. Moreover, it was demonstrated that periarticular injection of exosomes containing IL-10 or exosomes from bone marrow-derived DCs could relieve arthritis by anti-inflammatory action since DC-derived exosomes showed strong anti-inflammatory and immunosuppressive activity through the class II-dependent pathway. In addition, as exosomes are phenotypically stable after purification in vitro (79), EVs could potentially be a drug carrier for precise therapy for RA. Louise et al. used the human neutrophil-derived EVs as scaffolds, which have the function of immune regulation and cartilage penetration. Anti-reactive oxygen species-collagen type II (Anti-ROS-CII) is an antibody targeting impaired arthritic cartilage. Combining this antibody with EVs allows the complex to penetrate the cartilage into the articular cavity and still maintain antibody activity, suggesting the potential of EVs as a targeted carrier for drug delivery (80).
The Role of EVs in SLE
EVs Are Involved in the Pathogenesis of SLE
SLE is a complex heterogenous autoimmune disease that involves damage to multiple organs throughout the body and can cause death in severe cases. Patients with SLE are characterized by T and B lymphocyte dysfunction, accumulation of autoantibodies, and deposition of immune complexes (81). However, the pathogenesis of SLE remains unclear. The role of EVs in the pathogenesis of SLE is of interest to researchers.
Exosomal miRNAs in exosomes play an important role in the development of SLE. The level of miR-146a contained within exosomes in the urine of lupus patients was significantly higher than that outside of exosomes. In contrast, miR-146a levels in serum exosomes were significantly lower in SLE patients than in HCs (59). Of all miRNAs, miR-146a can significantly distinguish active LN from inactive LN and is related to inflammation and fibrosis of the kidney (74). In addition, miR-146a may be upregulated by chemokines as well as proinflammatory cytokines and leads to anemia in SLE patients (82). MSCs can internalize exosomes with miR-146a and target TRAF6/NF-κB signaling, leading to the senescence of MSCs (59). The senescence of MSCs may be related to the disease activity and pathological process of SLE (83, 84). Another important exosomal miRNA is miR-21 contained in EVs, facilitating estrogen-regulated STAT1 activation and Toll-like receptor (TLR) 8 expression in SLE. miRNAs can be endogenous ligands of human TLR7, which is the single-stranded RNA (ssRNA) receptor expressed by plasmacytoid dendritic cells (pDCs). miR-21 can replace viral ssRNA to combine with TLR8 to stimulate innate immune responses (60). Interferon (IFN)-α plays a major role in SLE (85). It was proven that miRNAs in exosomes, such as miR-574, upregulated type I IFNs secreted by pDCs in SLE (61). MVs from apoptosis in SLE serum can activate cyclic guanosine monophosphate (GMP)-AMP synthase (cGAS), which stimulates the stimulator of interferon genes (STING) pathway and upregulates the type I IFN production (62). The EVs involved in the pathogenesis of SLE were shown in Table 1.
The Role of EVs in Diagnosis and Treatment of SLE
EVs can be used to measure disease activity and differential diagnosis in patients with LE. Damage to glomerular podocytes is crucial in renal injury in SLE. Urinary podocyte-derived MPs can be used for the prediction of disease activity. They are positively correlated with clinical indicators of SLE, including erythrocyte sedimentation rate, proteinuria, and SLE Disease Activity Index (SLEDAI) score (75). Urinary HMGB1 in MPs is expressed at a significantly high level in active LN, which can distinguish between active and non-active LN (77). And identification of MPs with different surface proteins in SLE patients can predict disease activity and vascular damage (86). It was reported in another study that high plasma expression of monocytic CD 14+ MP has a positive correlation with the disease activity of SLE (65). Compared with healthy controls and systemic sclerosis (SSc) patients, SLE patients presented a higher expression of endothelial cell MP (EMP), suggesting that EMP has potential as a biomarker for SLE vascular lesions (76). Potential biomarkers for SLE in EVs are summarized in Table 2.
EVs have also received further attention in the treatment of SLE. In LN, MP surface proteins, especially G3BP, play a key role in the deposition of ICs. Therefore, targeting MPs may be a new approach for treating LN (87). MSC-derived MVs have anti-inflammatory and immunomodulatory effects (88). Although the use of MSCs in the treatment of SLE is mature and has been used clinically (89–93), Juhi et al. found that MSC-derived EVs can replace MSCs in the treatment of SLE, with the following advantages. First, there is no evidence that EVs are carcinogenic. Second, compared with MSCs, EVs are more stable and easier to preserve in the long term. Third, EVs do not cause an immune response that harms the host. EVs can bypass the blood–brain barrier, which makes it possible for EVs to be used in the treatment of lupus encephalopathy (94). In addition, EVs are easier to prepare on a large scale and at a low cost for clinical therapy. However, the effect of EVs is closely related to the dose, and the appropriate therapeutic dose needs to be explored.
Conclusion and Outlook
Although EVs were discovered in 1983, research on EVs has grown rapidly only in the current century. The role of EVs in cellular communication and immune regulation is being gradually explained. EVs secreted by immune cells are involved in antigen presentation and regulation of immunity. Cytokines or miRNAs contained in EVs and MSC-derived EVs play important roles in autoimmune diseases. Technology for isolating and purifying EVs is growing (95–98). New technologies, such as nanoscale flow cytometry (NanoFCM) and microfluidic platforms with 100,000 pillars, have been used for more efficient isolation of EVs (99, 100). A microfluidic cell culture platform using a 3D-printed microfluidic chip has also been used in the preparation of EVs (101). The research development of EVs is helpful to understand the pathogenesis of autoimmune diseases and provide new ideas for diagnosis and treatment. At the same time, we should also pay attention to the role of EVs in the onset and development of diseases and emphasize the dose and safety in the treatment to avoid potential side effects.
Author Contributions
BZ wrote the manuscript. MZ and QL conceptualized and revised the manuscript. All authors contributed to the article and approved the submitted version.
Funding
The present research was supported by the National Natural Science Foundation of China (No. 81874243, No. 81861138016, No. 81830097), CAMS Innovation Fund for Medical Sciences (CIFMS) (2019-I2M-5-033), the Key project for international and regional cooperation in science and technology innovation of Hunan province (2019WK2081), and the Project for leading talents in science and technology in Hunan province (2019RS3003).
Conflict of Interest
The authors declare that the research was conducted in the absence of any commercial or financial relationships that could be construed as a potential conflict of interest.
References
1. van der Woude D, van der Helm-van Mil AH. Update on the epidemiology, risk factors, and disease outcomes of rheumatoid arthritis. Best Pract Res Clin Rheumatol (2018) 32:174–87. doi: 10.1016/j.berh.2018.10.005
2. Wu H, Chang C, Lu Q. The Epigenetics of Lupus Erythematosus. Adv Exp Med Biol (2020) 1253:185–207. doi: 10.1007/978-981-15-3449-2_7
3. Luo S, Long H, Lu Q. Recent advances in understanding pathogenesis and therapeutic strategies of Systemic Lupus Erythematosus. Int Immunopharmacol (2020) 89:107028–36. doi: 10.1016/j.intimp.2020.107028
4. Gomides APM, de Albuquerque CP, Santos ABV, Bértolo MB, Júnior PL, Giorgi RDN, et al. Real-life data of survival and reasons for discontinuation of biological disease-modifying drugs ‘in’ rheumatoid arthritis. Int J Clin Pharm (2020) 10:1–6. doi: 10.1007/s11096-020-01171-5
5. Porta S, Danza A, Arias Saavedra M, Carlomagno A, Goizueta MC, Vivero F, et al. Glucocorticoids in Systemic Lupus Erythematosus. Ten Questions and Some Issues. J Clin Med (2020) 9:2709–22. doi: 10.3390/jcm9092709
6. Shah R, Patel T, Freedman JE. Circulating Extracellular Vesicles in Human Disease. N Engl J Med (2018) 379:958–66. doi: 10.1056/NEJMra1704286
7. Tian J, Casella G, Zhang Y, Rostami A, Li X. Potential roles of extracellular vesicles in the pathophysiology, diagnosis, and treatment of autoimmune diseases. Int J Biol Sci (2020) 16:620–32. doi: 10.7150/ijbs.39629
8. Raposo G, Stoorvogel W. Extracellular vesicles: exosomes, microvesicles, and friends. J Cell Biol (2013) 200:373–83. doi: 10.1083/jcb.201211138
9. Maas SLN, Breakefield XO, Weaver AM. Extracellular Vesicles: Unique Intercellular Delivery Vehicles. Trends Cell Biol (2017) 27:172–88. doi: 10.1016/j.tcb.2016.11.003
10. Andaloussi S EL, Mager I, Breakefield XO, Wood MJ. Extracellular vesicles: biology and emerging therapeutic opportunities. Nat Rev Drug Discov (2013) 12:347–57. doi: 10.1038/nrd3978
11. Barile L, Vassalli G. Exosomes: Therapy delivery tools and biomarkers of diseases. Pharmacol Ther (2017) 174:63–78. doi: 10.1016/j.pharmthera.2017.02.020
12. Raposo G, Nijman HW, Stoorvogel W, Liejendekker R, Harding CV, Melief CJ, et al. B lymphocytes secrete antigen-presenting vesicles. J Exp Med (1996) 183:1161–72. doi: 10.1084/jem.183.3.1161
13. Morrison TJ, Jackson MV, Cunningham EK, Kissenpfennig A, McAuley DF, O’Kane CM, et al. Mesenchymal Stromal Cells Modulate Macrophages in Clinically Relevant Lung Injury Models by Extracellular Vesicle Mitochondrial Transfer. Am J Respir Crit Care Med (2017) 196:1275–86. doi: 10.1164/rccm.201701-0170OC
14. Kim IK, Kim SH, Choi SM, Youn BS, Kim HS. Extracellular Vesicles as Drug Delivery Vehicles for Rheumatoid Arthritis. Curr Stem Cell Res Ther (2016) 11:329–42. doi: 10.2174/1574888x11666151203223251
15. Perez-Hernandez J, Redon J, Cortes R. Extracellular Vesicles as Therapeutic Agents in Systemic Lupus Erythematosus. Int J Mol Sci (2017) 18:10.3390/ijms18040717. doi: 10.3390/ijms18040717
16. Théry C, Zitvogel L, Amigorena S. Exosomes: composition, biogenesis and function. Nat Rev Immunol (2002) 2:569–79. doi: 10.1038/nri855
17. Hessvik NP, Llorente A. Current knowledge on exosome biogenesis and release. Cell Mol Life Sci (2018) 75:193–208. doi: 10.1007/s00018-017-2595-9
18. Colombo M, Moita C, van Niel G, Kowal J, Vigneron J, Benaroch P, et al. Analysis of ESCRT functions in exosome biogenesis, composition and secretion highlights the heterogeneity of extracellular vesicles. J Cell Sci (2013) 126:5553–65. doi: 10.1242/jcs.128868
19. Hough KP, Trevor JL, Strenkowski JG, Wang Y, Chacko BK, Tousif S, et al. Exosomal transfer of mitochondria from airway myeloid-derived regulatory cells to T cells. Redox Biol (2018) 18:54–64. doi: 10.1016/j.redox.2018.06.009
20. Lorent JH, Levental I. Structural determinants of protein partitioning into ordered membrane domains and lipid rafts. Chem Phys Lipids (2015) 192:23–32. doi: 10.1016/j.chemphyslip.2015.07.022
21. Akers JC, Gonda D, Kim R, Carter BS, Chen CC. Biogenesis of extracellular vesicles (EV): exosomes, microvesicles, retrovirus-like vesicles, and apoptotic bodies. J Neurooncol (2013) 113:1–11. doi: 10.1007/s11060-013-1084-8
22. Crescitelli R, Lässer C, Szabó TG, Kittel A, Eldh M, Dianzani I, et al. Distinct RNA profiles in subpopulations of extracellular vesicles: apoptotic bodies, microvesicles and exosomes. J Extracell Vesicles (2013) 2:20677–86. doi: 10.3402/jev.v2i0.20677
23. Kalra H, Drummen GP, Mathivanan S. Focus on Extracellular Vesicles: Introducing the Next Small Big Thing. Int J Mol Sci (2016) 17:170. doi: 10.3390/ijms17020170
24. Johnstone RM, Adam M, Hammond JR, Orr L, Turbide C. Vesicle formation during reticulocyte maturation. Association of plasma membrane activities with released vesicles (exosomes). J Biol Chem (1987) 262:9412–20. doi: 10.1557/PROC-0928-GG08-04
25. Gutierrez-Vazquez C, Villarroya-Beltri C, Mittelbrunn M, Sanchez-Madrid F. Transfer of extracellular vesicles during immune cell-cell interactions. Immunol Rev (2013) 251:125–42. doi: 10.1111/imr.12013
26. Jong AY, Wu CH, Li J, Sun J, Fabbri M, Wayne AS, et al. Large-scale isolation and cytotoxicity of extracellular vesicles derived from activated human natural killer cells. J Extracell Vesicles (2017) 6:1294368. doi: 10.1080/20013078.2017.1294368
27. Reis M, Mavin E, Nicholson L, Green K, Dickinson AM, Wang XN. Mesenchymal Stromal Cell-Derived Extracellular Vesicles Attenuate Dendritic Cell Maturation and Function. Front Immunol (2018) 9:2538. doi: 10.3389/fimmu.2018.02538
28. Arima Y, Liu W, Takahashi Y, Nishikawa M, Takakura Y. Effects of Localization of Antigen Proteins in Antigen-Loaded Exosomes on Efficiency of Antigen Presentation. Mol Pharm (2019) 16:2309–14. doi: 10.1021/acs.molpharmaceut.8b01093
29. Li Q, Wang H, Peng H, Huyan T, Cacalano NA. Exosomes: Versatile Nano Mediators of Immune Regulation. Cancers (Basel) (2019) 11:1557–77. doi: 10.3390/cancers11101557
30. Mittelbrunn M, Gutierrez-Vazquez C, Villarroya-Beltri C, Gonzalez S, Sanchez-Cabo F, Gonzalez MA, et al. Unidirectional transfer of microRNA-loaded exosomes from T cells to antigen-presenting cells. Nat Commun (2011) 2282:282–289. doi: 10.1038/ncomms1285
31. Sprent J. Direct stimulation of naïve T cells by antigen-presenting cell vesicles. Blood Cells Mol Dis (2005) 35:17–20. doi: 10.1016/j.bcmd.2005.04.004
32. Montecalvo A, Larregina AT, Shufesky WJ, Stolz DB, Sullivan ML, Karlsson JM, et al. Mechanism of transfer of functional microRNAs between mouse dendritic cells via exosomes. Blood (2012) 119:756–66. doi: 10.1182/blood-2011-02-338004
33. Viaud S, Terme M, Flament C, Taieb J, Andre F, Novault S, et al. Dendritic cell-derived exosomes promote natural killer cell activation and proliferation: a role for NKG2D ligands and IL-15Ralpha. PloS One (2009) 4:e4942. doi: 10.1371/journal.pone.0004942
34. Pegtel DM, Cosmopoulos K, Thorley-Lawson DA, van Eijndhoven MA, Hopmans ES, Lindenberg JL, et al. Functional delivery of viral miRNAs via exosomes. Proc Natl Acad Sci USA (2010) 107:6328–33. doi: 10.1073/pnas.0914843107
35. Gutzeit C, Nagy N, Gentile M, Lyberg K, Gumz J, Vallhov H, et al. Exosomes derived from Burkitt’s lymphoma cell lines induce proliferation, differentiation, and class-switch recombination in B cells. J Immunol (2014) 192:5852–62. doi: 10.4049/jimmunol.1302068
36. Mandal A, Viswanathan C. Natural killer cells: In health and disease. Hematol Oncol Stem Cell Ther (2015) 8:47–55. doi: 10.1016/j.hemonc.2014.11.006
37. Hurwitz SN, Nkosi D, Conlon MM, York SB, Liu X, Tremblay DC, et al. CD63 Regulates Epstein-Barr Virus LMP1 Exosomal Packaging, Enhancement of Vesicle Production, and Noncanonical NF-κB Signaling. J Virol (2017) 91. doi: 10.1128/jvi.02251-16
38. Ning Y, Shen K, Wu Q, Sun X, Bai Y, Xie Y, et al. Tumor exosomes block dendritic cells maturation to decrease the T cell immune response. Immunol Lett (2018) 199:36–43. doi: 10.1016/j.imlet.2018.05.002
39. Que RS, Lin C, Ding GP, Wu ZR, Cao LP. Increasing the immune activity of exosomes: the effect of miRNA-depleted exosome proteins on activating dendritic cell/cytokine-induced killer cells against pancreatic cancer. J Zhejiang Univ Sci B (2016) 17:352–60. doi: 10.1631/jzus.B1500305
40. Riazifar M, Mohammadi MR, Pone EJ, Yeri A, Lässer C, Segaliny AI, et al. Stem Cell-Derived Exosomes as Nanotherapeutics for Autoimmune and Neurodegenerative Disorders. ACS Nano (2019) 13:6670–88. doi: 10.1021/acsnano.9b01004
41. Park KS, Bandeira E, Shelke GV, Lässer C, Lötvall J. Enhancement of therapeutic potential of mesenchymal stem cell-derived extracellular vesicles. Stem Cell Res Ther (2019) 10:288. doi: 10.1186/s13287-019-1398-3
42. Flaherty SE, Grijalva A, Xu X, Ables E, Nomani A, Ferrante AW. A lipase-independent pathway of lipid release and immune modulation by adipocytes. Science (2019) 363:989–93. doi: 10.1126/science.aaw2586
43. Alam J, Jantan I, Bukhari SNA. Rheumatoid arthritis: Recent advances on its etiology, role of cytokines and pharmacotherapy. Biomed Pharmacother (2017) 92:615–33. doi: 10.1016/j.biopha.2017.05.055
44. Burbano C, Rojas M, Munoz-Vahos C, Vanegas-Garcia A, Correa LA, Vasquez G, et al. Extracellular vesicles are associated with the systemic inflammation of patients with seropositive rheumatoid arthritis. Sci Rep (2018) 8:17917. doi: 10.1038/s41598-018-36335-x
45. Pásztói M, Sódar B, Misják P, Pálóczi K, Kittel Á, Tóth K, et al. The recently identified hexosaminidase D enzyme substantially contributes to the elevated hexosaminidase activity in rheumatoid arthritis. Immunol Lett (2013) 149:71–6. doi: 10.1016/j.imlet.2012.10.012
46. Berenbaum F, Le Gars L, Toussirot E, Sanon A, Bories C, Kaplan G, et al. Marked elevation of serum N-acetyl-beta-D-hexosaminidase activity in rheumatoid rheumatoid arthritis. Clin Exp Rheumatol (2000) 18:63–6. doi: 10.1186/ar132
47. Skriner K, Adolph K, Jungblut PR, Burmester GR. Association of citrullinated proteins with synovial exosomes. Arthritis Rheum (2006) 54:3809–14. doi: 10.1002/art.22276
48. Rantapää-Dahlqvist S, de Jong BA, Berglin E, Hallmans G, Wadell G, Stenlund H, et al. Antibodies against cyclic citrullinated peptide and IgA rheumatoid factor predict the development of rheumatoid arthritis. Arthritis Rheum (2003) 48:2741–9. doi: 10.1002/art.11223
49. Khandpur R, Carmona-Rivera C, Vivekanandan-Giri A, Gizinski A, Yalavarthi S, Knight JS, et al. NETs are a source of citrullinated autoantigens and stimulate inflammatory responses in rheumatoid arthritis. Sci Trans Med (2013) 5:178ra40. doi: 10.1126/scitranslmed.3005580
50. Messer L, Alsaleh G, Freyssinet JM, Zobairi F, Leray I, Gottenberg JE, et al. Microparticle-induced release of B-lymphocyte regulators by rheumatoid synoviocytes. Arthritis Res Ther (2009) 11:R40. doi: 10.1186/ar2648
51. Cloutier N, Tan S, Boudreau LH, Cramb C, Subbaiah R, Lahey L, et al. The exposure of autoantigens by microparticles underlies the formation of potent inflammatory components: the microparticle-associated immune complexes. EMBO Mol Med (2013) 5:235–49. doi: 10.1002/emmm.201201846
52. Boilard E, Nigrovic PA, Larabee K, Watts GF, Coblyn JS, Weinblatt ME, et al. Platelets amplify inflammation in arthritis via collagen-dependent microparticle production. Science (2010) 327:580–3. doi: 10.1126/science.1181928
53. Zhang HG, Liu C, Su K, Su K, Yu S, Zhang L, et al. A membrane form of TNF-alpha presented by exosomes delays T cell activation-induced cell death. J Immunol (2006) 176:7385–93. doi: 10.4049/jimmunol.176.12.7385
54. Jüngel A, Distler O, Schulze-Horsel U, Huber LC, Ha HR, Simmen B, et al. Microparticles stimulate the synthesis of prostaglandin E(2) via induction of cyclooxygenase 2 and microsomal prostaglandin E synthase 1. Arthritis Rheum (2007) 56:3564–74. doi: 10.1002/art.22980
55. Greisen SR, Yan Y, Hansen AS, Venø MT, Nyengaard JR, Moestrup SK, et al. Extracellular Vesicles Transfer the Receptor Programmed Death-1 in Rheumatoid Arthritis. Front Immunol (2017) 8851:851. doi: 10.3389/fimmu.2017.00851
56. Takamura Y, Aoki W, Satomura A, Shibasaki S, Ueda M. Small RNAs detected in exosomes derived from the MH7A synovial fibroblast cell line with TNF-α stimulation. PloS One (2018) 13:e0201851. doi: 10.1371/journal.pone.0201851
57. Stanczyk J, Pedrioli DM, Brentano F, Sanchez-Pernaute O, Kolling C, Gay RE, et al. Altered expression of MicroRNA in synovial fibroblasts and synovial tissue in rheumatoid arthritis. Arthritis Rheum (2008) 58:1001–9. doi: 10.1002/art.23386
58. Blom AB, van Lent PL, Libregts S, Holthuysen AE, van der Kraan PM, van Rooijen N, et al. Crucial role of macrophages in matrix metalloproteinase-mediated cartilage destruction during experimental osteoarthritis: involvement of matrix metalloproteinase 3. Arthritis Rheum (2007) 56:147–57. doi: 10.1002/art.22337
59. Dong C, Zhou Q, Fu T, Zhao R, Yang J, Kong X, et al. Circulating Exosomes Derived-miR-146a from Systemic Lupus Erythematosus Patients Regulates Senescence of Mesenchymal Stem Cells. BioMed Res Int (2019) 2019:6071308. doi: 10.1155/2019/6071308
60. Young NA, Valiente GR, Hampton JM, Wu LC, Burd CJ, Willis WL, et al. Estrogen-regulated STAT1 activation promotes TLR8 expression to facilitate signaling via microRNA-21 in systemic lupus erythematosus. Clin Immunol (2017) 176:12–22. doi: 10.1016/j.clim.2016.12.005
61. Salvi V, Gianello V, Busatto S, Bergese P, Andreoli L, D’Oro U, et al. Exosome-delivered microRNAs promote IFN-α secretion by human plasmacytoid DCs via TLR7. JCI Insight (2018) 3. doi: 10.1172/jci.insight.98204
62. Kato Y, Park J, Takamatsu H, Konaka H, Aoki W, Aburaya S, et al. Apoptosis-derived membrane vesicles drive the cGAS-STING pathway and enhance type I IFN production in systemic lupus erythematosus. Ann Rheum Dis (2018) 77:1507–15. doi: 10.1136/annrheumdis-2018-212988
63. Arntz OJ, Pieters BCH, Thurlings RM, Wenink MH, van Lent PLEM, Koenders MI, et al. Rheumatoid Arthritis Patients With Circulating Extracellular Vesicles Positive for IgM Rheumatoid Factor Have Higher Disease Activity. Front Immunol (2018) 9:2388. doi: 10.3389/fimmu.2018.02388
64. Sellam J, Proulle V, Jüngel A, Ittah M, Miceli Richard C, Gottenberg JE, et al. Increased levels of circulating microparticles in primary Sjögren’s syndrome, systemic lupus erythematosus and rheumatoid arthritis and relation with disease activity. Arthritis Res Ther (2009) 11:R156. doi: 10.1186/ar2833
65. Viñuela-Berni V, Doníz-Padilla L, Figueroa-Vega N, Portillo-Salazar H, Abud-Mendoza C, Baranda L, et al. Proportions of several types of plasma and urine microparticles are increased in patients with rheumatoid arthritis with active disease. Clin Exp Immunol (2015) 180:442–51. doi: 10.1111/cei.12598
66. György B, Szabó TG, Turiák L, Wright M, Herczeg P, Lédeczi Z, et al. Improved flow cytometric assessment reveals distinct microvesicle (cell-derived microparticle) signatures in joint diseases. PloS One (2012) 7:e49726. doi: 10.1371/journal.pone.0049726
67. Song J, Kim D, Han J, Kim Y, Lee M, Jin EJ. PBMC and exosome-derived Hotair is a critical regulator and potent marker for rheumatoid arthritis. Clin Exp Med (2015) 15:121–6. doi: 10.1007/s10238-013-0271-4
68. Liu D, Fang Y, Rao Y, Tan W, Zhou W, Wu X, et al. Synovial fibroblast-derived exosomal microRNA-106b suppresses chondrocyte proliferation and migration in rheumatoid arthritis via down-regulation of PDK4. J Mol Med (2020) 98:409–23. doi: 10.1007/s00109-020-01882-2
69. Xu D, Song M, Chai C, Wang J, Jin C, Wang X, et al. Exosome-encapsulated miR-6089 regulates inflammatory response via targeting TLR4. J Cell Physiol (2019) 234:1502–11. doi: 10.1002/jcp.27014
70. Wang Y, Zheng F, Gao G, Yan S, Zhang L, Wang L, et al. MiR-548a-3p regulates inflammatory response via TLR4/NF-κB signaling pathway in rheumatoid arthritis. J Cell Biochem (2018) 120:1133–40. doi: 10.1002/jcb.26659
71. Murata K, Yoshitomi H, Tanida S, Ishikawa M, Nishitani K, Ito H, et al. Plasma and synovial fluid microRNAs as potential biomarkers of rheumatoid arthritis and osteoarthritis. Arthritis Res Ther (2010) 12:R86. doi: 10.1186/ar3013
72. Garcia-Vives E, Solé C, Moliné T, Vidal M, Agraz I, Ordi-Ros J, et al. The Urinary Exosomal miRNA Expression Profile is Predictive of Clinical Response in Lupus Nephritis. Int J Mol Sci (2020) 21:1372–89. doi: 10.3390/ijms21041372
73. Solé C, Moliné T, Vidal M, Ordi-Ros J, Cortés-Hernández J. An Exosomal Urinary miRNA Signature for Early Diagnosis of Renal Fibrosis in Lupus Nephritis. Cells (2019) 8:773–89. doi: 10.3390/cells8080773
74. Perez-Hernandez J, Forner MJ, Pinto C, Chaves FJ, Cortes R, Redon J. Increased Urinary Exosomal MicroRNAs in Patients with Systemic Lupus Erythematosus. PloS One (2015) 10:e0138618. doi: 10.1371/journal.pone.0138618
75. Lu J, Hu ZB, Chen PP, Lu CC, Zhang JX, Li XQ, et al. Urinary podocyte microparticles are associated with disease activity and renal injury in systemic lupus erythematosus. BMC Nephrol (2019) 20:303. doi: 10.1186/s12882-019-1482-z
76. McCarthy EM, Moreno-Martinez D, Wilkinson FL, McHugh NJ, Bruce IN, Pauling JD, et al. Microparticle subpopulations are potential markers of disease progression and vascular dysfunction across a spectrum of connective tissue disease. BBA Clin (2017) 7:16–22. doi: 10.1016/j.bbacli.2016.11.003
77. Burbano C, Gómez-Puerta JA, Muñoz-Vahos C, Vanegas-García A, Rojas M, Vásquez G, et al. HMGB1 microparticles present in urine are hallmarks of nephritis in patients with systemic lupus erythematosus. Eur J Immunol (2019) 49:323–35. doi: 10.1002/eji.201847747
78. Ma D, Xu K, Zhang G, Liu Y, Gao J, Tian M, et al. Immunomodulatory effect of human umbilical cord mesenchymal stem cells on T lymphocytes in rheumatoid arthritis. Int Immunopharmacol (2019) 74:105687. doi: 10.1016/j.intimp.2019.105687
79. Kim SH, Lechman ER, Bianco N, Menon R, Keravala A, Nash J, et al. Exosomes derived from IL-10-treated dendritic cells can suppress inflammation and collagen-induced arthritis. J Immunol (2005) 174:6440–8. doi: 10.4049/jimmunol.174.10.6440
80. Topping LM, Thomas BL, Rhys HI, Tremoleda JL, Foster M, Seed M, et al. Targeting Extracellular Vesicles to the Arthritic Joint Using a Damaged Cartilage-Specific Antibody. Front Immunol (2020) 11:10. doi: 10.10.3389/fimmu.2020.00010
81. Cui Y, Sheng Y, Zhang X. Genetic susceptibility to SLE: recent progress from GWAS. J Autoimmun (2013) 41:25–33. doi: 10.1016/j.jaut.2013.01.008
82. Dominguez-Gutierrez PR, Ceribelli A, Satoh M, Sobel ES, Reeves WH, Chan EK. Positive correlation of STAT1 and miR-146a with anemia in patients with systemic lupus erythematosus. J Clin Immunol (2014) 34:171–80. doi: 10.1007/s10875-013-9973-3
83. Nie Y, Lau C, Lie A, Chan G, Mok M. Defective phenotype of mesenchymal stem cells in patients with systemic lupus erythematosus. Lupus (2010) 19:850–9. doi: 10.1177/0961203309361482
84. Gao L, Bird AK, Meednu N, Dauenhauer K, Liesveld J, Anolik J, et al. Bone Marrow-Derived Mesenchymal Stem Cells From Patients With Systemic Lupus Erythematosus Have a Senescence-Associated Secretory Phenotype Mediated by a Mitochondrial Antiviral Signaling Protein-Interferon-β Feedback Loop. Arthritis Rheumatol (2017) 69:1623–35. doi: 10.1002/art.40142
85. Niewold TB, Hua J, Lehman TJ, Harley JB, Crow MK. High serum IFN-alpha activity is a heritable risk factor for systemic lupus erythematosus. Genes Immun (2007) 8:492–502. doi: 10.1038/sj.gene.6364408
86. Fortin PR, Cloutier N, Bissonnette V, Aghdassi E, Eder L, Simonyan D, et al. Distinct Subtypes of Microparticle-containing Immune Complexes Are Associated with Disease Activity, Damage, and Carotid Intima-media Thickness in Systemic Lupus Erythematosus. J Rheumatol (2016) 43:2019–25. doi: 10.3899/jrheum.160050
87. Nielsen CT, Rasmussen NS, Heegaard NH, Jacobsen S. “Kill” the messenger: Targeting of cell-derived microparticles in lupus nephritis. Autoimmun Rev (2016) 15:719–25. doi: 10.1016/j.autrev.2016.03.009
88. Mokarizadeh A, Delirezh N, Morshedi A, Mosayebi G, Farshid AA, Mardani K. Microvesicles derived from mesenchymal stem cells: potent organelles for induction of tolerogenic signaling. Immunol Lett (2012) 147:47–54. doi: 10.1016/j.imlet.2012.06.001
89. Sun L, Wang D, Liang J, Zhang H, Feng X, Wang H, et al. Umbilical cord mesenchymal stem cell transplantation in severe and refractory systemic lupus erythematosus. Arthritis Rheum (2010) 62:2467–75. doi: 10.1002/art.27548
90. Malgieri A, Kantzari E, Patrizi MP, Gambardella S. Bone marrow and umbilical cord blood human mesenchymal stem cells: state of the art. Int J Clin Exp Med (2010) 3:248–69. doi: 10.1007/978-1-4419-5635-4_11
91. Wang D, Zhang H, Liang J, Li X, Feng X, Wang H, et al. Allogeneic mesenchymal stem cell transplantation in severe and refractory systemic lupus erythematosus: 4 years of experience. Cell Transplant (2013) 22:2267–77. doi: 10.3727/096368911X582769c
92. Wang D, Li J, Zhang Y, Zhang M, Chen J, Li X, et al. Umbilical cord mesenchymal stem cell transplantation in active and refractory systemic lupus erythematosus: a multicenter clinical study. Arthritis Res Ther (2014) 16:R79. doi: 10.1186/ar4520
93. Carrion F, Nova E, Ruiz C, Diaz F, Inostroza C, Rojo D, et al. Autologous mesenchymal stem cell treatment increased T regulatory cells with no effect on disease activity in two systemic lupus erythematosus patients. Lupus (2010) 19:317–22. doi: 10.1177/0961203309348983
94. Sharma J, Hampton JM, Valiente GR, Wada T, Steigelman H, Young MC, et al. Therapeutic Development of Mesenchymal Stem Cells or Their Extracellular Vesicles to Inhibit Autoimmune-Mediated Inflammatory Processes in Systemic Lupus Erythematosus. Front Immunol (2017) 8:526. doi: 10.3389/fimmu.2017.00526
95. Konoshenko MY, Lekchnov EA, Vlassov AV, Laktionov PP. Isolation of Extracellular Vesicles: General Methodologies and Latest Trends. BioMed Res Int (2018) 2018:8545347. doi: 10.1155/2018/8545347
96. Nordin JZ, Lee Y, Vader P, Mäger I, Johansson HJ, Heusermann W, et al. Ultrafiltration with size-exclusion liquid chromatography for high yield isolation of extracellular vesicles preserving intact biophysical and functional properties. Nanomedicine (2015) 11:879–83. doi: 10.1016/j.nano.2015.01.003
97. Dehghani M, Gulvin SM, Flax J, Gaborski TR. Systematic Evaluation of PKH Labelling on Extracellular Vesicle Size by Nanoparticle Tracking Analysis. Sci Rep (2020) 10:9533. doi: 10.1038/s41598-020-66434-7
98. Benedikter BJ, Bouwman FG, Vajen T, Heinzmann ACA, Grauls G, Mariman EC, et al. Ultrafiltration combined with size exclusion chromatography efficiently isolates extracellular vesicles from cell culture media for compositional and functional studies. Sci Rep (2017) 7:15297. doi: 10.1038/s41598-017-15717-7
99. Zieren RC, Dong L, Pierorazio PM, Pienta KJ, de Reijke TM, Amend SR. Extracellular vesicle isolation from human renal cancer tissue. Med Oncol (2020) 37:28. doi: 10.1007/s12032-020-1346-1
100. Kamyabi N, Abbasgholizadeh R, Maitra A, Ardekani A, Biswal SL, Grande-Allen KJ. Isolation and mutational assessment of pancreatic cancer extracellular vesicles using a microfluidic platform. Biomed Microdevices (2020) 22:23. doi: 10.1007/s10544-020-00483-7
Keywords: extracellular vesicles, exosome, systemic lupus erythematosus, microRNA, rheumatoid arthritis
Citation: Zhang B, Zhao M and Lu Q (2021) Extracellular Vesicles in Rheumatoid Arthritis and Systemic Lupus Erythematosus: Functions and Applications. Front. Immunol. 11:575712. doi: 10.3389/fimmu.2020.575712
Received: 24 June 2020; Accepted: 27 November 2020;
Published: 14 January 2021.
Edited by:
Winston Patrick Kuo, Harvard University, United StatesReviewed by:
Hai-Feng Pan, Anhui Medical University, ChinaGeorge C. Tsokos, Harvard Medical School, United States
Copyright © 2021 Zhang, Zhao and Lu. This is an open-access article distributed under the terms of the Creative Commons Attribution License (CC BY). The use, distribution or reproduction in other forums is permitted, provided the original author(s) and the copyright owner(s) are credited and that the original publication in this journal is cited, in accordance with accepted academic practice. No use, distribution or reproduction is permitted which does not comply with these terms.
*Correspondence: Ming Zhao, emhhb21pbmczMDdAY3N1LmVkdS5jbg==; Qianjin Lu, cWlhbmx1NTg2MEBjc3UuZWR1LmNu