- 1Institut Pasteur, Microbiology Department, Unité Biologie et Génétique de la Paroi Bactérienne, Paris, France
- 2CNRS, UMR 2001 Microbiologie intégrative et Moléculaire, Paris, France
- 3INSERM, Equipe Avenir, Paris, France
- 4Université de Paris, Sorbonne Paris Cité, Paris, France
- 5Laboratorio de Virus Animales, Instituto de Biotecnología y Biología Molecular, CONICET-Universidad Nacional de La Plata, La Plata, Argentina
- 6Departamento de Microbiologia, Universidade Federal de Minas Gerais (UFMG), Belo Horizonte, Brazil
The spirochetal bacteria Leptospira spp. are causative agents of leptospirosis, a globally neglected and reemerging zoonotic disease. Infection with these pathogens may lead to an acute and potentially fatal disease but also to chronic asymptomatic renal colonization. Both forms of disease demonstrate the ability of leptospires to evade the immune response of their hosts. In this review, we aim first to recapitulate the knowledge and explore the controversial data about the opsonization, recognition, intracellular survival, and killing of leptospires by scavenger cells, including platelets, neutrophils, macrophages, and dendritic cells. Second, we will summarize the known specificities of the recognition or escape of leptospire components (the so-called microbial-associated molecular patterns; MAMPs) by the pattern recognition receptors (PRRs) of the Toll-like and NOD-like families. These PRRs are expressed by phagocytes, and their stimulation by MAMPs triggers pro-inflammatory cytokine and chemokine production and bactericidal responses, such as antimicrobial peptide secretion and reactive oxygen species production. Finally, we will highlight recent studies suggesting that boosting or restoring phagocytic functions by treatments using agonists of the Toll-like or NOD receptors represents a novel prophylactic strategy and describe other potential therapeutic or vaccine strategies to combat leptospirosis.
Introduction
Leptospires are diderm bacteria belonging to the phylum Spirochetes and are classified as extracellular pathogens. These bacteria are responsible for a zoonosis with a worldwide distribution with a higher incidence in poor countries and tropical humid areas. Some animals, including rats and mice, are chronic carriers of leptospires in their kidneys, particularly in the lumen of the proximal tubules. They excrete the bacteria in the urine and contaminate the environment. Leptospires are found in water and soil and can infect all vertebrates, including mammals. Transmission occurs through transdermal or mucosal penetration of the bacteria, which first strongly adhere to skin and mucosal surfaces. Then, the bacteria reach the blood circulation and disseminate to all organs.
In terms of the symptoms and severity of the diseases caused by Leptospira spp., most Leptospira infections are asymptomatic. Leptospira interrogans are responsible for the most severe forms of leptospirosis in both humans and animals (1). In humans, the symptoms vary from a flu-like disease with fever, headaches, and muscular pains to more severe forms with icterus, hemorrhages, pulmonary or kidney insufficiency, requiring hospitalization. It was estimated in 2015 that in 5% of cases, leptospirosis led to multiorgan failure and accounted for 60,000 fatalities (2).
Compared to the saprophytic L. biflexa Patoc strain, which grows rapidly and is amenable to genetic manipulation, L. interrogans are difficult bacteria to study because of their extended generation time (approximately 18 h), the difficulty of obtaining mutant strains, and the fact that in vitro passaging quickly leads to the loss of virulence. In addition, more than 350 serovars have been described based on the immunogenicity of lipopolysaccharide (LPS), the major antigen of leptospires. Serovar diversity complicates diagnostics and constitutes one of the main barriers to obtaining a universal vaccine against leptospirosis (1).
One of the first lines of defense of the innate immune system is comprised of antibacterial components present in the serum. The complement system is a complex set of proteolytic cascades and opsonins that aim to directly destroy pathogens or target them for destruction by immune cells, such as macrophages (MΦ). This system is considered a nonspecific innate mechanism (3, 4). In addition, preimmunized hosts have a repertoire of antibodies that specifically target a pathogen for elimination and destruction. Therefore, both antibodies and other opsonins are of special importance for destroying pathogens through neutralization and engulfment by professional phagocytes, such as MΦ and neutrophils. The phagocytic function is mediated by several membrane-associated receptors on the cell surface, such as scavenger receptors (5, 6) and Fc receptors, which are exclusively dedicated to the recognition of the fragment crystallizable (Fc) regions of antibodies (6, 7). In addition, upon infection, phagocytes produce reactive oxygen species (ROS), such as nitric oxide (NO), and other potent antimicrobial compounds that participate in pathogen elimination.
Pattern recognition receptors (PRRs) recognize microbial-associated molecular patterns (MAMPs). They are essential, evolutionarily conserved structures shared among microbes but are not found in the host and include viral or bacterial nucleic acids and lipopolysaccharide. PRRs also recognize endogenous molecules associated with cellular damage (DAMPs) that are produced upon microbial infection, for example, (8). PRRs are expressed on both immune cells and nonimmune cells and include members of the membrane Toll-like receptor (TLR) and the cytosolic NOD-like receptor (NLR) families (9). MAMP recognition by a PRR triggers a signaling cascade leading to activation of transcription factors such as NF-κB and IRF3 involved in the production of cytokines, chemokines, and antimicrobial peptides, which leads to the activation and recruitment of phagocytes, such as neutrophils, MΦs, and dendritic cells (DCs), at the site of infection. The resulting inflammation not only may lead to pathogen destruction but also, if uncontrolled, may be deleterious for the host, such as the “cytokine storm” observed in septic patients. PRR activation also results in the expression of costimulatory molecules at the surface of MΦ and DCs that are important for antigen presentation to naive T cells and the onset of adaptive immunity.
Several studies have explored the role of phagocytes during leptospiral infection. In part I of this review, we will address the cellular biology of leptospire infection in vitro and ex vivo with a focus on the role of opsonization, the intracellular localization of leptospires, and cell death. We will also highlight in vivo studies suggesting the limited role of phagocytes in leptospires. In part II, we will recapitulate what has been published about leptospire recognition by or escape from TLR and NLR proteins. In part III, we will present recent studies suggesting that boosting TLR or NLR responses may help the host combat leptospirosis.
Part I—Phagocytes; Poor Foes for Leptospira
In Vitro and Ex Vivo Studies of the Role of Phagocytes and Scavenger Receptors
Macrophages (MΦ)
This section will review the literature regarding the antibacterial effect of serum, the effect of complement antibody opsonization on the internalization of leptospires by MΦ (Table 1 and Figure 1), the fate of leptospires in MΦ (Table 2 and Figure 2A), and the complex data about the effect of Leptospira spp. on cell death (Table 3 and Figure 2B).
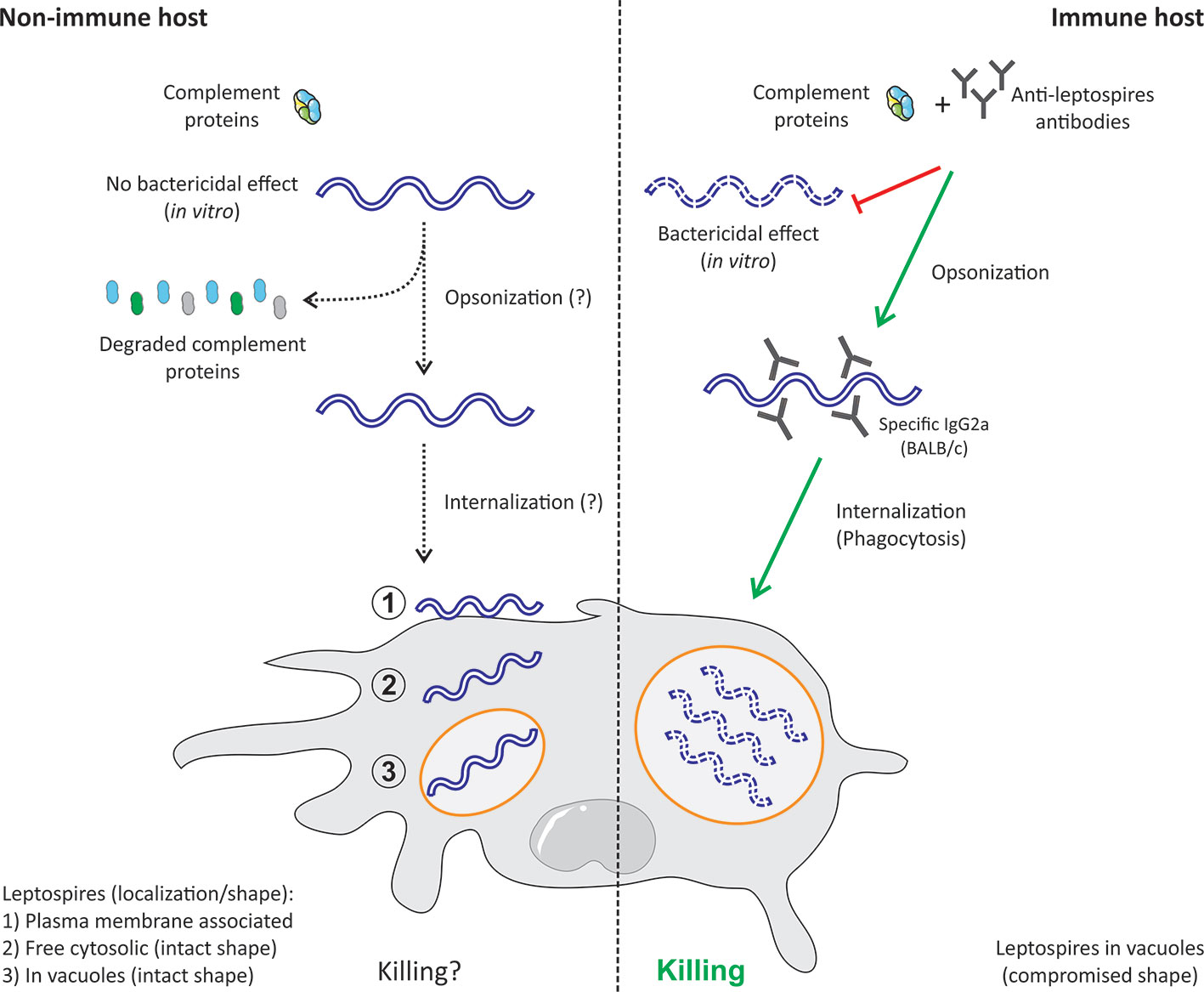
Figure 1 Effects of opsonization on leptospire survival in macrophages. Leptospira interrogans are resistant to nonimmune serum and have evolved diverse mechanisms to avoid the complement system. In contrast, immune serum exerts a bactericidal effect on leptospires. Preincubation of leptospires with immune serum containing anti-leptospires antibodies (Abs) leads to rapid internalization and killing of leptospires by macrophages. In this case, leptospires are exclusively found in vacuoles, in which they have compromised shapes. When leptospires are incubated with naive serum, their internalization seems to be slower, and several populations can be identified inside and outside macrophages: (1) plasma membrane-associated, (2) free in the cytosol, and (3) or in vacuoles. In this case, vacuolar leptospires do not seem to have a compromised shape (12, 17).
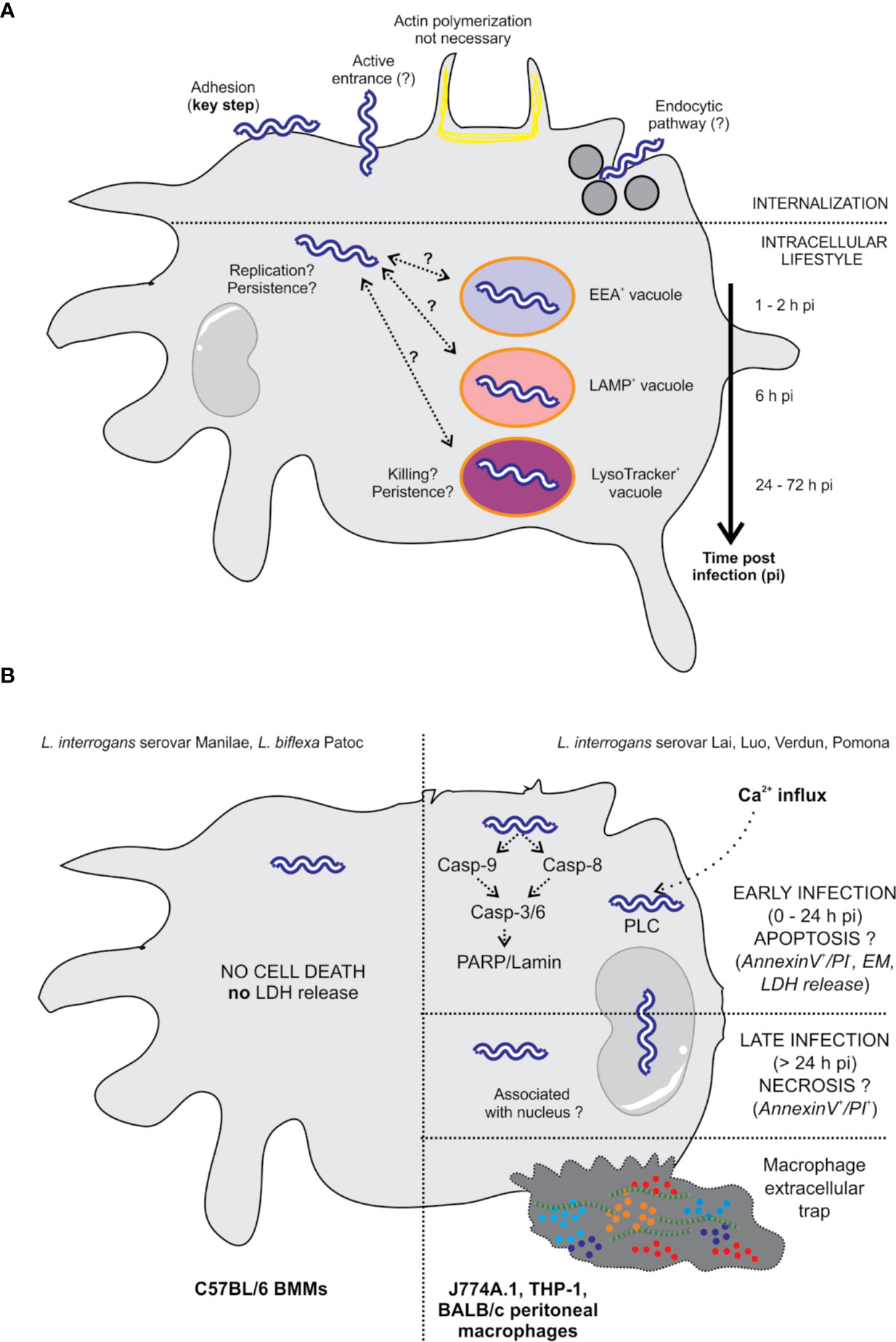
Figure 2 Intracellular localization of L. interrogans and effect on cell death. (A) The internalization of leptospires is highly dependent on adhesion to the cell surface (25). The entrance mechanism is not clearly established since it does not seem to involve actin polymerization, suggesting an entrance mechanism other than phagocytosis (18, 25, 26). Viability seems to be essential since formalin-fixed bacteria do not enter the cells (18). The endocytic pathway could potentially be involved in the internalization of leptospires since inhibition of this pathway in macrophages drastically reduced the number of intracellular bacteria (18). Once inside the cells, leptospires are found free in the cytosol in human and murine macrophages. In addition, some authors describe that leptospires are found in EEA1-, LAMP-, and LysoTracker-positive compartments using colocalization (20, 24). Colocalization seems to be extended over time, which may suggest the arrest of potential phagosomes/autophagosomes at several stages. In human cells, leptospires appear to be replicative (20). However, in murine cells, the overall number of leptospires seems to be constant or diminished over time (20, 24), with the unique observation that live bacteria are still found at 72 hpi, suggesting their persistence. (B) L. interrogans serovar Manilae does not induce macrophage cell death upon infection of BMMs obtained from a C57BL/6 background (24). L. interrogans serovars Lai, Luo, and Verdun induce cell death by apoptosis/necrosis upon infection of J774A.1, THP-1, and BALB/c peritoneal macrophages (18, 21–23). However, not all the studies have completely correlating results, and some controversies are revealed in the literature. Additionally, some studies report that leptospires are associated with the nucleus (18, 23).
Role of Opsonization in Leptospire Survival and Interaction With MΦ
Early studies performed from 1960 to the mid-1980s showed that in vitro incubation with nonimmune serum does not exert bactericidal activity against pathogenic leptospires (11, 13, 28, 29). These studies described that pathogenic, but not saprophytic, leptospires were resistant to complement-induced destruction (11, 28, 29). More recently, other studies have confirmed this observation and shown that leptospires are resistant to complement (30–32). Nevertheless, in vitro incubation with immune serum exerts bactericidal activity on saprophytic and pathogenic leptospires (13, 28, 29). Given the importance of serum components and antibodies in MΦ function, several studies have addressed their role in phagocyte function. When leptospires were opsonized with immunoglobulin G (IgG), guinea pig peritoneal MΦ‘ bactericidal activity was enhanced, and leptospires were found in membrane compartments of MΦ with a compromised shape (11). In line with this study, Cinco et al. showed that elicited peritoneal MΦ from guinea pigs had no antibactericidal activity if the eliciting leptospires were not opsonized (10). Free nonopsonized leptospires were found in the cytosol or in membrane compartments. In contrast, opsonized leptospires were found in membrane compartments with compromised shapes. Furthermore, Wang et al. reported that human monocytes and MΦ only take up leptospires if they are opsonized with immune but not with normal serum (13). Opsonized leptospires were also found inside vesicles with altered shapes (13). Using peritoneal MΦ from BALB/c mice, Tu et al. reported that phagocytosis is only observed when leptospires are pretreated with rat anti-leptospiral serum (12). In contrast, Isogai et al. showed that murine MΦ do not need opsonization to take up leptospires, although opsonization drastically increases the uptake efficiency (14). Uptake seems to be mediated by IgG2a rather than IgG2b, and opsonization increases the rate of uptake of membrane-associated leptospires (14). The data presented in this section are recapitulated in Figure 1 and Table 1.
Interestingly, in studies that did not use opsonizing conditions that resemble the physiology of a nonimmune host, it was found that motility and the endocytic pathway but not actin remodeling were involved in the internalization of pathogenic leptospires (18, 25, 26), suggesting their escape from phagocytic internalization. Interestingly, motility has been shown to alter phagocytosis of other pathogens, such as Pseudomonas aeruginosa, in both murine and human MΦ (33). Since motility constitutes one of the few virulence factors of leptospires [reviewed in (34)], this would represent a potent evasion mechanism that deserves further investigation.
Intracellular Localization and Fate of Leptospires
Phagocytosis requires the engagement of different receptors that trigger the engulfment of the pathogen (5). It is an actin-mediated process (35) that delivers the engulfed cargo into the lysosomal compartment for degradation. In addition, phagocytized particles can be used for antigen presentation. The phagocytic pathway is complex and involves many partners. Several players (36) such as Rab GTPases (37, 38) and phosphatidylinositol kinases (PIK) (39) are essential to the formation and maturation of the phagosome and for antigen presentation (40). Numerous proteins serve as hallmarks of key steps. For example, early phagosomes are characterized by the presence of early endosome antigen 1 (EEA1) and Rab5 (38, 41, 42). Rab5 then further recruits Rab7 in the so-called late phagosome, which is also characterized by the presence of lysosomal-associated membrane proteins (LAMPs). The latter are required for lysosomal fusion and acidification (43). Lysosomal acidification to a pH of 4.5–5 is mediated by a V-type H+ ATPase (44), allowing for the optimal functioning of lysosomal enzymes such as cathepsins and proteases. Many pathogens have evolved strategies to block or inhibit phagocytic killing (45, 46). For example, Rab proteins are selectively targeted to the benefit of the pathogen by two intracellular pathogens, Mycobacterium tuberculosis (47) and Salmonella enterica (48). Whether leptospires subvert MΦ killing functions is still unknown.
However, many studies have studied the fate of leptospires when they are incubated with MΦ or epithelial cells. In both cell types, virulent leptospires are rapidly internalized. In contrast, avirulent (high culture passage) and saprophytic strains were adherent to the cell surface and less likely to be internalized. The endocytic pathway and viability but not actin polymerization were shown to be involved in the internalization of leptospires (18). In contrast, other studies found that virulent (serovar Lai) and avirulent (serovar Luo) L. interrogans but not L. biflexa rapidly adhere to MΦ and epithelial cell surfaces (23). Both serovars were observed inside MΦ by electron microscopy (EM) in membrane-associated compartments (23). Furthermore, both serovars were found to adhere to and be internalized by murine and human MΦ (cell lines and primary cells) (20). In human cells, leptospires appear in a replicative form, are free in the cytosol, and conserve their helical shape (20). However, leptospires partially colocalize with the lysosomal marker LAMP-1 after infection (20). On the other hand, in murine cells, leptospires are killed, and they are found in membrane compartments with compromised round shapes (20). In murine cells, leptospires are delivered into LAMP-positive compartments over time with a plateau at 48 h postinfection (hpi) (20). Although leptospires seem to be killed by murine MΦ, MΦ still contain live and viable leptospires 72 hpi (20). Surprisingly, the same research group showed 3 years later using the same settings and strains that upon infection of human cells, leptospires are located in phagosomes (21), which suggests that the careful interpretation of both articles is necessary (Table 2).
In a set of different studies, Toma et al. used bone marrow-derived MΦ from a C57BL/6 background and infected them with virulent (low passage) and avirulent (high passage) L. interrogans serovar Manilae or with saprophytic L. biflexa serovar Patoc (24, 25). Both pathogenic and saprophytic leptospires were found to be intracellular (24). Infection was shown to be highly dependent on virulence and adhesion through at least 2 proteins, LigB and Lmb216, which is a putative adhesin present only in pathogenic species (25). Notably, this study confirmed that actin polymerization does not play a role in the internalization process (25).
Interestingly, L. biflexa are killed and show a compromised shape, whereas pathogenic bacteria conserve their shape and are not killed (24, 25). Both EEA-1 and LAMP were found to colocalize with leptospires after infection (24). However, the phagosomal protease cathepsin D showed delayed recruitment kinetics toward the pathogenic strain (24). Furthermore, only the saprophytic strain was found to colocalize almost completely with the lysosomal marker LysoTracker (24). In contrast, pathogenic leptospires showed a decreasing level of colocalization with lysosomal markers (24). This set of observations seems to indicate the arrest of the maturation of the lysosome after internalization of pathogenic leptospires. To support these observations, confocal microscopy images of intracellular leptospires were obtained, and EMJH cultures were performed to assess viability. Not surprisingly, saprophytic but not pathogenic leptospires were killed at 24 hpi (24). Moreover, live pathogenic leptospires were observed for at least 48 hpi (24). More recently, using MΦ of bovine origin, Nagel et al. observed that leptospires are intracellular and that their internalization is dependent on virulence and is independent of actin polymerization (26). Intracellular leptospires were found in LAMP- and LysoTracker-positive compartments (26). This set of observations suggests that leptospires are not internalized via actin-dependent phagocytosis and that the arrest of the maturation of lysosomes could take place upon infection with pathogenic leptospires.
Interestingly, opsonized leptospires are found in vacuoles in MΦ from resistant (murine) or sensitive (human and guinea pig) hosts, revealing the central role of MΦ in peritoneally immunized hosts regardless of their sensitivity to the disease (10–14). Once they are inside MΦ, leptospires colocalize with phagosomal/lysosomal pathway markers (13, 26, 36). This could indicate that leptospires are located in the phagosomal compartment; however, the persistent colocalization of these markers may also indicate the arrest of the phagocytic pathways or the presence of persistent forms. Such is the case for the intracellular pathogen Listeria monocytogenes, which switches to a vascular lifestyle once it is inside host cells (49). In this state, L. monocytogenes persists in the lysosomal environment, which favors both survival and asymptomatic carriage of this pathogen (49). This can have an impact on intracellular survival, as shown by Li et al. (20) and Toma et al. (24), which showed that the overall number of intracellular leptospires seems to be either decreased or constant. In any case, it seems that there are a few persistent bacteria inside cells that are not cleared even 72 hpi.
In conclusion, there are several questions about aspects of the literature about MΦ and leptospires that are still difficult to interpret or should be considered with caution due to the lack of controls, experimental caveats, or internally contradictory results (Tables 2, 3). Once they are inside of murine MΦ, leptospires are localized in membrane-associated compartments (20, 24, 26). However, in human cells, the localization of leptospires is not clear since conflicting results indicate that leptospires are either free in the cytosol (20) or found in phagosomes (21) (Table 2). This difference could be due to the culture conditions of leptospires or the state of the infected MΦ, allowing leptospires to be internalized inside vacuoles or to remain in the cytosol. Another possibility to explain the discrepant results could be that experiments performed at different time points could reflect that leptospires are either trapped in a vacuole or have had time to escape from a vacuole. Whether leptospires modulate intracellular vacuole trafficking to benefit their survival during an infection, similar to what is observed for other pathogens such as Staphylococcus aureus (50), remains to be studied.
Apoptosis and Cell Death
Programmed cell death (PCD) is a crucial physiological and homeostatic process that can be triggered under different stress conditions (51). It occurs through different mechanisms, including apoptosis, necroptosis, and pyroptosis. Each of these mechanisms has its own signaling pathway and physiological role (51). Apoptosis is an immunologically silent form of cell death in which cells undergo a noninflammatory type of programmed cell death (PCD). Apoptotic cells are engulfed by phagocytes, and any threat is hence eliminated. On the other hand, although they are mechanistically different, both necroptosis and pyroptosis are inflammatory types of PCD. In fine, PCD can be considered a host defense mechanism against pathogens that may use the host as a reservoir. Therefore, it is not surprising that during infection, pathogens modulate cell death pathways to their advantage (52, 53). This section aims to present studies that describe MΦ cell death during infection with pathogenic leptospires. The main findings are summarized in Table 3 and Figure 2.
Some studies reported that leptospires were associated with the nucleus in some cells, suggesting a particular killing mechanism (18, 23). Li et al. showed that both live and dead leptospires trigger apoptosis/necrosis, which is surprising to some extent (23). Furthermore, L. interrogans serovar Lai triggers apoptosis early upon infection, although necrosis is the most prevalent form of cell death in MΦ at late stages postinfection (20). In addition, infection seems to trigger the nuclear accumulation of p53 and DNA damage (21). A mechanism was proposed in murine MΦ by which L. interrogans serovar Lai but not L. biflexa serovar Patoc activates caspase-3- and caspase-8-mediated PCD (27). This type of PCD seems to be mediated by a leptospiral phospholipase involved in the accumulation of intracellular Ca2+ that is associated with apoptotic cell death (22). Unfortunately, some of these results are conflicting (see Tables 1, 4), making their interpretation complex. On the other hand, infection with L. interrogans serovar Manilae does not induce LDH release (24), suggesting that this strain does not induce cell death.
More recently, infection with bovine MΦ was shown to trigger the formation of macrophage-extracellular traps (METs) (26), which have also been observed during neutrophil infection (63). This effect seems to be independent of the virulence status of leptospires since a low or high number of passages lead to similar formation of METs (26). Although this phenomenon is considered a particular form of PCD, some people have reported that after the release in NETS, neutrophils remain alive (65).
In fact, there seems to be a clear difference between the Lai and Icterohaemorrhagiae serovars compared with the Manilae strain in terms of the induction of PCD upon MΦ infection. However, the referenced studies did not use the same infection model (66, 67). This could be the basis of the major difference between strains, as in the case of M. tuberculosis, in which virulent strains only escape apoptosis mediated by infected MΦ (68). The use of MΦ of different origins (cell lines, primary bone marrow-derived, peritoneal) and from different hosts (guinea pig, mouse, rat and human) as well as the different species/serovar/strains of leptospires makes it very difficult to formulate a global interpretation. Furthermore, currently, it is widely accepted that even though they constitute a valuable resource for research, cell lines are also a major source of variability and reproducibility issues (69). In addition, most studies did not perform gentamicin assays (Table 2), which is a caveat when studying the internalization of bacteria and leads to the questioning of the interpretation of some data, which are to be considered with caution (Table 2). Therefore, additional work is required to better understand whether different leptospire strains may behave differently and/or whether species-specific aspects of the intracellular environment may explain some of the controversial data (Table 2 and Figure 2).
Neutrophils Recognize Leptospires but Are Poor Phagocytes
Neutrophils are key cells that act against extracellular pathogens through three major mechanisms: (i) phagocytosis, which usually requires opsonization beforehand; (ii) degranulation, which involves the release of an arsenal of cytotoxic molecules stored in granules; and (iii) the release of extracellular traps (ETs, or NETs, in this case). Although neutrophils are short-lived, their life span is significantly extended under infectious and inflammatory conditions (70). Moreover, neutrophils can produce ROS through NADPH activation and the release of pro-inflammatory cytokines involved in the activation, regulation, and effector functions of innate and adaptive immune cells (71).
Early in vitro studies of Leptospira phagocytosis by neutrophils showed some partially contradictory results (Table 4 and Figure 3 neutrophils). However, phagocytosis by human polymorphonuclear leucocytes (PMN) of both virulent and avirulent Leptospira spp. required the presence of specific immune serum and complement components (72), while other studies showed that only saprophytic leptospires were phagocytized in the absence of serum, and pathogenic leptospires were not phagocytized by human PMN even in the presence of serum (13). Interestingly, it was also reported that avirulent leptospires showed increased phagocytosis by human PMN compared to virulent leptospires, and phagocytosis was markedly enhanced after opsonization (54).
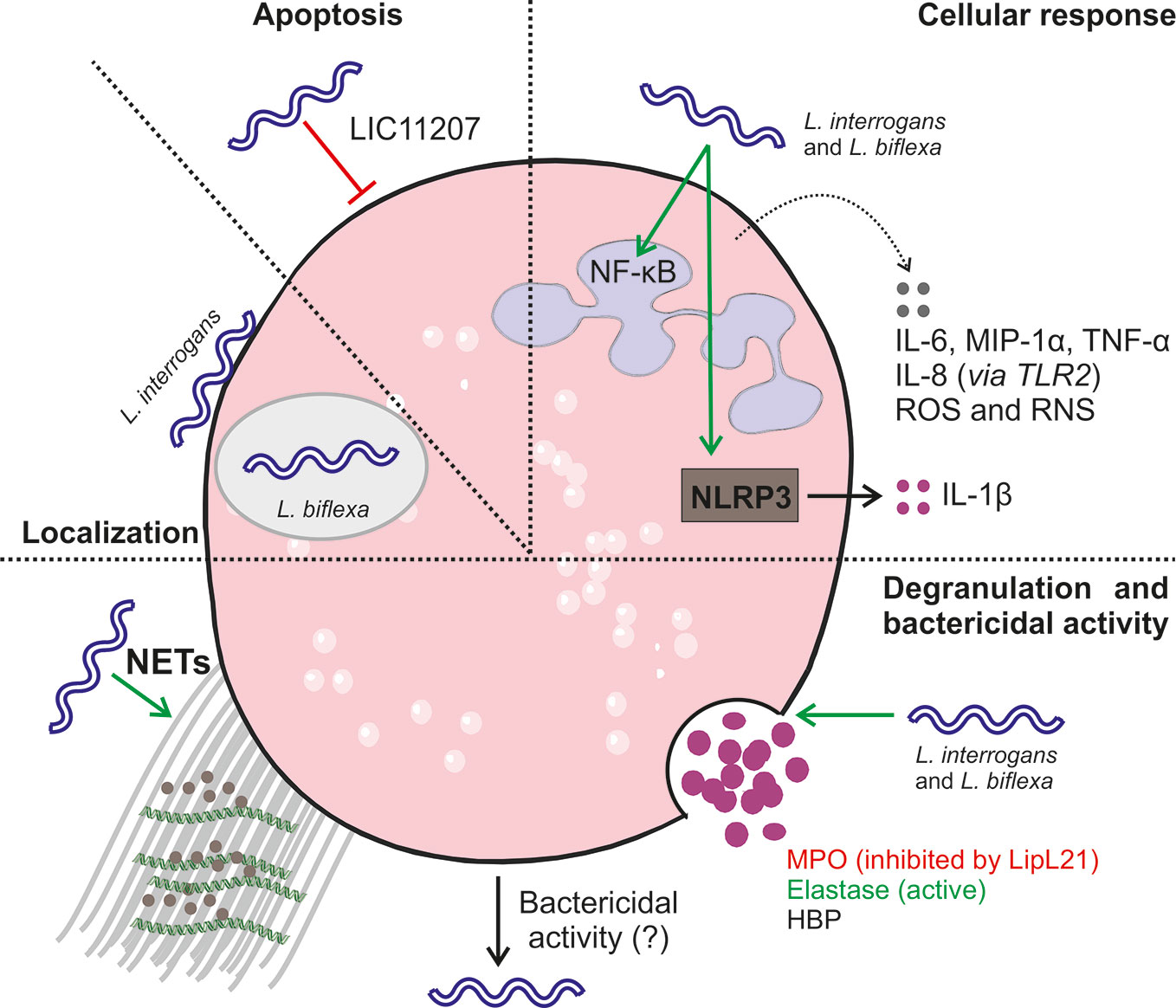
Figure 3 Interaction of L. interrogans and neutrophils. Upon infection, leptospires are observed at the neutrophil surface or intracellularly in vacuoles. Live pathogenic leptospires seem to be resistant to internalization unless they are opsonized. In contrast, saprophytic leptospires are rapidly internalized and killed (13, 54, 55). Leptospires do not trigger apoptosis (58, 59), and they have an anti-apoptotic effect on infected cells (55). Intracellular uptake of leptospires triggers cellular responses characterized by the production of antibacterial components such as reactive oxygen species (ROS) and reactive nitrogen species (RNS) (54, 55, 57, 58, 61, 62, 64). In addition, infection triggers TLR2-mediated production of IL-8 and NLRP3-dependent production of IL-1β (55). Moreover, infection triggers the production of other antibacterial mediators, such as cytokines and chemokines (55, 57), but does not induce or inhibit neutrophil myeloperoxidase (MPO) activity (55, 60). Cellular responses tend to be more robust for live leptospires than heat- or formalin-inactivated leptospires (54, 55, 60, 63). Moreover, infection triggers the production of neutrophil extracellular traps (NETSs) (57). The bactericidal activity of neutrophils has not always been observed, and it seems to be dependent on the sources of cells, the serovar used, and the use of opsonizing antibodies (13, 55, 57, 60).
In a recent EM analysis (55), most pathogenic leptospires were found on the neutrophil surface and were not phagocytized. In contrast, saprophytic leptospires were taken up. Intracellular ROS levels correlated with leptospire uptake. Altogether, it seems that pathogenic leptospires can avoid or significantly reduce their uptake by human neutrophils, but the precise mechanisms and in vivo relevance involved are unknown.
Regarding degranulation, it has been shown that both virulent and avirulent Leptospira spp. are killed by both primary and secondary granule contents. The primary (azurophilic) granules, which contain myeloperoxidase (MPO), heparin-binding protein (HBP), defensins, and other antimicrobial peptides (AMPs), showed the highest microbicidal activity (61). Remarkably, it has been recently shown that the L. interrogans outer membrane protein LipL21 is a potent inhibitor of neutrophil MPO (60). This heme-containing peroxidase enzyme, which is mainly expressed in neutrophils, catalyzes the formation of ROS intermediates in the presence of hydrogen peroxide and halides, including hypochlorous acid (HOCl), a major effector of microbial killing by neutrophils. Interestingly, MPO deficiency results in exaggerated inflammatory responses and affects neutrophil functions, including cytokine production (73). In addition, the leptospiral proteins Lsa63 and LipL45 can induce release of the HBP protein into the extracellular space (58), which in turn can induce endothelial cell cytoskeletal rearrangements, leading to disruption of cell barriers and increased vascular permeability (58, 74). The role of MPO and HBP in the pathogenesis of leptospirosis would be further clarified by using animal models deficient in each neutrophil component.
Concerning the formation of NETs, it was demonstrated that Leptospira spp. were able to induce NET release in human neutrophils and that the bacterial number, pathogenicity, and viability were relevant factors for NET release induction, whereas bacterial motility was not (63). Interestingly, although NETs reduced leptospire viability, pathogenic but not saprophytic Leptospira spp. exerted nuclease activity and degraded DNA, suggesting that pathogenic leptospires may counteract this microbicidal mechanism (63). The formation of NETs was also observed when Leptospira spp. were incubated with bovine neutrophils, although the amounts were lower than those induced by E. coli (57), suggesting that the in vivo role of NETs in Leptospira dissemination may be less important than that of other bacteria. More recently, it was reported that both avirulent and virulent Leptospira spp. triggered neutrophil responses involved in migration, including the upregulation of CD11b expression and adhesion to collagen. In addition, both species activated the NF-κB and inflammasome pathways (see part II-4) and increased the levels and release of the pro-inflammatory chemokine IL-8 and the cytokines IL-6 and IL-1β. As expected with PMN activation, leptospires delayed neutrophil apoptosis (55).
Platelets
Platelets play a well-recognized role in hemostasis and thrombosis, and it is now broadly accepted that platelets also have an important role in inflammation and immune responses (75). Platelet immunological functions include the secretion of functional mediators such as cytokines and chemokines (76) as well as direct interactions with immune cells (77). In addition, platelets may function as direct scavengers in bacterial infections (78, 79). To accomplish such diverse functions, in addition to all the components that are critical to guarantee hemostasis, platelets express several PRRs (80), namely, TLRs, complement, and Fc-γ receptors (81). Human platelets express 10 members of the TLR family, which are functional PRRs that not only sense microbes but also trigger platelet effector responses that modulate the innate immune response (82). In addition to TLRs, platelet cell adhesion molecules, such as glycoprotein Ib (GPIb), P-selectin, and CD40L, allow intimate contact with inflammatory neutrophils and monocytes through binding to their counterreceptors αMβ2, PSGL-1, and CD40 (83). Moreover, platelets also interact (touch-and-go interactions) with leukocytes, including DCs, T cells, and B cells (84, 85). These associations favor crosstalk between platelets and neutrophils, resulting in bidirectional activation of both cell types and amplification of the inflammatory response (65).
Early studies reported that LPS from pathogenic Leptospira spp. leads to aggregation, degranulation, and lysis of rabbit platelets in vitro (86). In contrast, it was reported that the pathogenic leptospiral immunoglobulin-like protein B (LigB) binds to the C-terminus of the fibrinogen αC domain, inhibiting fibrin clot formation and human platelet adhesion and aggregation (87), while leptospiral proteins containing the von Willebrand factor type A domain induce hemorrhage in leptospirosis by competitive inhibition of vWF/GPIb-mediated platelet aggregation (88, 89). Curiously, in human platelets, aggregation seems to occur only when platelets are in a mixture with PMN previously primed with leptospiral LPS but not when they are in platelet suspensions alone (64). In this regard, it has been shown by flow cytometry that Leptospira spp. induce the formation of neutrophil-platelet mixed aggregates (55), a fact that partially explains the thrombocytopenia observed in patients. It is clear that more studies about the effects of Leptospira on platelets are needed, including bacterial uptake, activation and viability (90).
Dendritic Cells
DCs are professional antigen-presenting cells, and their activation links the innate immune recognition of pathogens to the triggering of adaptive immunity. DCs express many different PRRs, including the carbohydrate receptor DC-SIGN of the C-type lectin family, which recognizes high-mannose glycans. DC-SIGN binds intercellular adhesion molecule-3 (ICAM-3) and ICAM-2 on T cells, promoting the adhesion of DCs to naive T cells.
One study showed that live virulent or attenuated strains of L. interrogans serovar Pyrogenes and Autumnalis induced the maturation of monocyte-derived DCs obtained from healthy blood donors in vitro. Leptospiral infection also triggered TNF and IL-12 cytokine production, both of which are important for T cell activation (91). The authors demonstrated that leptospires bind in vitro and in vivo to DC-SIGN through surface carbohydrates (91). However, DC-SIGN requires other PRRs to trigger cytokine production. Which PRRs are involved in cytokine production induced by Leptospira infection and whether leptospires are killed by DCs and properly processed for antigen presentation have yet to be investigated in both human and murine cells.
In Vivo Role of Macrophages, Neutrophils, and Platelets During Leptospirosis
In Vivo Studies of Macrophages
One approach to studying the role of MΦ in vivo involves the depletion of these cells and the observation of the outcome of infection. Silica depletion via the intravenous (iv) route enhanced the susceptibility of mice to leptospiral infection independently of preimmunization with leptospires (14). Furthermore, intravenous (iv) immunization of undepleted mice with heat-killed leptospires or leptospiral LPS was found to have a positive effect on blood clearance, highlighting the role of phagocytes (14). More recently, using the zebrafish embryo model, Davis et al. showed that iv infection triggers active and rapid migration of MΦ to the site of infection, where they internalize leptospires (16). Interestingly, at this stage of development, there are no antibodies present in the embryo, which suggests that no opsonization or complement opsonization is required for internalization of the leptospires in these embryos (16). More recently, using clodronate depletion of MΦ, our groups showed that MΦ partially controls leptospires in mice, with effects observed at both the acute phase of uncontrolled initial infection (17) and at the chronic phase (92). In addition, in a mouse model, it was recently observed that MΦ but not neutrophils appeared to be the major infiltrating anti-Leptospira phagocytes in the kidneys during leptospirosis (62). Therefore, although they play a limited role in nonimmunized hosts, MΦ are important cellular components of the immune response required to control leptospires.
In Vivo Studies of Neutrophils
It is accepted that human leptospirosis causes neutrophilia, which correlates with severity (93–96), and the pathogenic serovar is involved (97). Moreover, some neutrophil molecules, such as neutrophil gelatinase-associated lipocalin (NGAL) and HBP, have been proposed as early markers of acute leptospirosis (58, 98). In leptospirosis patients, enhanced expression levels of TLR2, CD15 (94, 99), and TLR4 (99) were found on neutrophils. In contrast, low expression levels of the AMP LL37 cathelicidin (active on leptospires) were found in leptospirosis patients (95). Curiously, CD62L and CD11b levels were found to be similar to those of controls (94, 99). The discrepancies between the abovementioned in vitro studies (55) may be explained by several factors, including the number of circulating bacteria. In a mouse model, it was observed that although neutrophil depletion significantly increases the early leptospiral loads in blood (63), depletion did not modify the overall course of the disease (62, 63, 100). Unfortunately, mice and humans have important differences regarding neutrophils (as well as platelets) (101). Therefore, the real importance of neutrophils and the mechanisms of leptospiral escape remain to be determined in patients with leptospirosis.
In Vivo Studies of Platelets During Leptospirosis
Platelet activation and dysfunction have been reported in leptospirosis patients (102, 103). In addition, thrombocytopenia is a frequent clinical finding, particularly in severe leptospirosis cases, and is associated with a worse prognosis and occurrence of hemorrhages (80, 104–106). There is no consensus about the underlying mechanisms of the decrease in platelet numbers during leptospirosis. The hypotheses include (i) disseminated intravascular coagulation (DIC), (ii) toxin-mediated platelet death, (iii) impaired production of platelets, (iv) platelet overactivation and consumption, and (v) autoimmune platelet clearance. While some studies reported negative results for DIC in leptospirosis patients (107) as well as in guinea pig studies (108, 109), other studies found evidence of DIC in some leptospirosis patients (103). Thrombocytopenia in human leptospirosis is apparently not immune-mediated (110), and some studies suggest that it might result from activation (102, 110). Therefore, a comprehensive investigation is needed to determine the mechanisms of thrombocytopenia in the disease.
Part II—Leptospires: Stealthy Pathogens That Escape Several PRR-Mediated Innate Responses
The high motility of leptospires is conferred by two atypical “endoflagella” that are present at each pole of the bacterium and extend within the periplasm. Their spiral shape is due to the peptidoglycan mesh, forming a thin layer close to the inner membrane. The membranes are rich in lipoproteins (111). In contrast with that of other spirochetes, the leptospiral outer membrane is covered in lipopolysaccharide (LPS) (112). LPS, lipoproteins, and peptidoglycan are conserved components of bacteria but are absent from the host and are recognized by the innate system through PRRs from the TLR and NLR families (9). Here, we aim to recapitulate the more striking features of leptospiral MAMP recognition by PRRs expressed by phagocytes from different hosts (Figure 4). For a more extensive review of this topic, see (112).
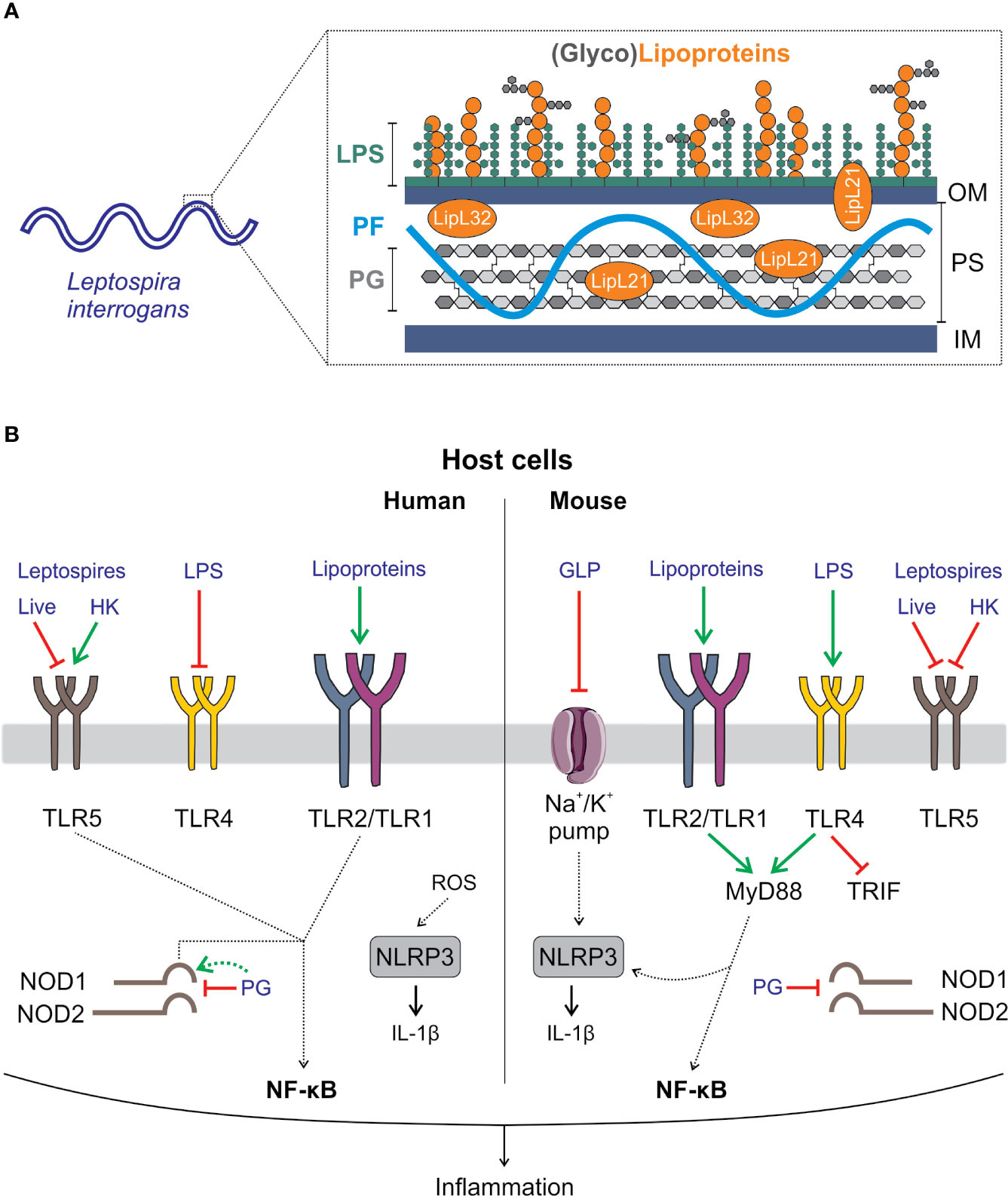
Figure 4 Interaction of Leptospira interrogans with the innate immune system. (A) L. interrogans are diderm bacteria. Their cell wall is composed of an inner membrane (IM) and an outer membrane (OM) containing lipopolysaccharide (LPS). In between the membranes, the periplasmic space (PS) contains the peptidoglycan (PG) layer, the periplasmic flagella (PF) that extends from each pole of the cell inside the PS and lipoproteins such as LipL32. Notably, leptospires contain in their OM a broad repertoire of lipoproteins and a glycolipoprotein (GLP) (111). (B) Host cells have a variety of mechanisms to detect microbes. One of them involves a subset of receptors collectively known as pattern recognition receptors (PRRs) that sense conserved and ubiquitously expressed microbial components. The latter are known as microbial-associated molecular patterns (MAMPs). Recognition of MAMPs by PRRs triggers the immune response. In blue, leptospiral MAMPs and MAMP sensing in human (left side panel) and mouse (right side panel) cells are shown. TLR2 is the main receptor for sensing leptospires, which is common in humans and mice. TLR2 heterodimerizes with TLR1 and detects triacylated lipoproteins. A major difference between sensitive humans and resistant mice is the specific sensing of the leptospiral LPS (113), a major leptospiral MAMP and virulence factor (34). Mouse TLR4 but not human TLR4 is able to detect it (114–116). However, the stimulation of mouse TLR4 by leptospiral LPS is only partial, since only the MyD88 pathway but not the TRIF pathway is activated. This results in only minimal production of antimicrobial components such as nitric oxide (117). Due to the presence of PF in leptospires, live leptospires also escape TLR5 recognition. However, once leptospires are killed by antimicrobial peptides that expose PF, leptospiral flagellins are recognized by human (and bovine) TLR5 but not by mouse TLR5 (118). Leptospires have been described to induce activation of the NLRP3 inflammasome in both humans and mice. In mice, activation of the inflammasome requires 2-step signaling involving LPS and lipoprotein signaling through TLR4 and TLR2, respectively, in combination with the downregulation of a Na/K pump (a danger signal) by GLP (119). In humans, NLRP3 activation is mediated by bacteria-induced ROS (120). Finally, PG from leptospires escapes host recognition by NOD1 and NOD2 due to the lipoprotein LipL21, which is tightly attached to the PG. In addition, human but not murine NOD1 can detect leptospiral PG in the absence of LipL21 (121).
TLR2 Recognition of Lipoproteins
The most striking feature of innate recognition of leptospires is their potent lipoprotein-induced TLR2 activation (112, 114, 122–124). It was first shown that LipL32, the major lipoprotein of leptospires, is a TLR2 agonist in human macrophages and hamster ovary cells (114) and in mouse renal tubular proximal cells (125). We also determined that the recognition of leptospires occurred through dimers of TLR2 and TLR1 (115) specific for triacylated lipoproteins that are present in leptospires (111). More recently, it was shown that leptospires also stimulate pig and bovine fibroblasts through TLR2 (122, 123). The abundant lipoproteins present in the membranes of leptospires easily explain their potent TLR2 activity, at least in vitro. However, the regulation of their expression and posttranslational modifications may lead to immune evasion in vivo (111). Hence, despite being responsible for cellular activation and inflammation in vitro, TLR2 triggering is not essential to mouse defense. Indeed, in contrast with TLR4 knockout (KO) mice, TLR2KO mice do not die from experimental leptospirosis (116, 126). However, activation of TLR2 in addition to TLR4 contributes to host protection in mice by triggering cytokine secretion and leptospire-specific IgG, NO, and IFNγ secretion (116). In addition, we recently showed that the inflammatory response of PMNs infected with L. interrogans was dependent on TLR2 in human neutrophils (55).
Notably, we have also highlighted the potential protective role of TLR2 in the human defense against Leptospira. Indeed, single nucleotide polymorphisms (SNPs) in TLR2 and TLR1, which are known to modulate susceptibility to infectious diseases, have been linked to increased susceptibility to developing severe leptospirosis in Argentina (124). However, the Argentinian cohort of patients was small, and the SNP found in TLR2 has not been associated with increased susceptibility to leptospirosis in a population from the Azores Islands (127).
TLR4 Recognition of LPS
LPS is a macromolecule composed of three sections: (i) a carbohydrate part responsible for the antigenic properties of LPS, (ii) a central core and KDO region, and (iii) lipid A, consisting of a disaccharide moiety linked to lipid chains that anchor LPS in the outer membrane. Lipid A, also known as “endotoxin,” is recognized by the TLR4/MD2 complex and is responsible for the toxic properties of LPS.
We first showed that the LPS of leptospires is not recognized through TLR4, as expected in human MΦ (114). However, it is recognized by murine TLR4 (115). The structure of leptospiral lipid A has been deciphered by the Raetz group and showed several modifications, including a methylated phosphate group and four amide-linked acyl chains, compared to the structure of classic lipid A from Enterobacteria, and these modifications result in the lack of reactive phosphate groups on each side of the disaccharide (113). These modifications potentially explain the lack of human TLR4 recognition, which is more stringent than its murine counterpart. Using deficient mice, it was shown that TLR4 is a major PRR involved in the control of leptospires (116, 128). The species specificity of TLR4 recognition most likely explains the differences in the severity of leptospirosis, which can be fatal in humans but asymptomatic in mice that are considered chronic carriers of Leptospira (34).
In addition, we recently showed that in mouse macrophages, TLR4 recognition of leptospiral LPS is not complete (117). Only one arm of the signaling pathway is triggered. Indeed, TLR4 has two adaptors: MyD88 at the cell surface and TRIF in the endosome. MyD88 signaling results in cytokine and costimulatory molecule expression, whereas TRIF leads to production of type I interferons and nitric oxide (NO) (117). Leptospiral LPS only stimulates the MyD88 pathway but does not stimulate the TRIF pathway, and as a consequence, whole bacteria induce only minimal antimicrobial NO and IFN-ß production. This mechanism may participate in bacterial evasion and potentially explains why LPS is a virulence factor (129) and why pathogenic leptospires succeed in reaching the mouse kidney despite TLR4 recognition. We showed that the escape mechanism is linked to the presence of a complete O antigen in LPS and tightly bound lipoproteins, which together impair the interaction of LPS with CD14, an important coreceptor required for TLR4 internalization and TRIF signaling (117). Indeed, purification protocols for leptospiral LPS (130) always lead to copurification of TLR2 agonists, suggesting the close structural association of LPS and lipoproteins (114). Moreover, we showed that the protein portion of lipoproteins but not the lipidic portion that signals through TLR2 is important for TRIF escape (117). In line with these data, a recent study showed that one strain of L. interrogans serovar Autumnalis responsible for the self-resolution of infection in a murine model possesses an LPS devoid of TLR2 activity (131).
TLR5 Recognition of Flagellin
TLR5 is the receptor of bacterial flagellins, composing the filamentous part of flagella. We previously showed that live leptospires triggered production of equivalent amounts of IL1ß and IL6 cytokines in wild-type and TLR5KO bone marrow–derived MΦ, suggesting that murine TLR5 does not recognize leptospires (132). However, it has also been shown in human whole blood infected or stimulated with inactivated leptospires that a neutralizing TLR5 antibody decreased the cytokine response, suggesting that human TLR5 may recognize leptospires (133). Both observations were confirmed and explained by our recent study showing that live leptospires escape TLR5 recognition in both human and mouse macrophages, but leptospires that are either degraded by heating or killed by antimicrobial peptides such as the cathelicidins LL37 or bMap28 are sensed by human and bovine TLR5, although they are not detected or only barely detected by mouse TLR5. These results confirmed the hypothesis that the periplasmic localization of the endoflagella participates in immune evasion. From a therapeutic perspective, since Enterobacteria flagellin is a potent adjuvant (134), one may also hypothesize that escape from TLR5 could potentially limit the adaptive immune response to Leptospira. Moreover, we also highlighted that the structure of leptospiral FlaB flagellins, which are agonists of TLR5, is devoid of the variable portion that confers the antigenicity of flagellins, suggesting that leptospiral flagellins would not be efficiently recognized by antibodies; this adds a second layer of immune evasion.
NOD1 and NOD2 Escape
NOD1 and NOD2 are cytosolic PRRs that recognize bacterial fragments of peptidoglycan called muropeptides, which are constantly released by the bacteria upon remodeling and synthesis of the cell wall (9). NOD1 and NOD2 are important for controlling invasive and extracellular bacteria (9). Activation of NOD receptors is also important for immune functions and the onset of adaptive immune responses (112). Indeed, in mice, NOD1 activation by the microbiota has been shown to be crucial for PMN killing of bacteria and fungi (135). In addition, we showed that NOD1 activation plays an important role in murine renal defense using a model of retro-urethral infection with uropathogenic Escherichia coli (136).
We showed that leptospires escape NOD1 and NOD2 recognition. We demonstrated that the lipoprotein LipL21 is tightly bound to peptidoglycan and impairs the release of muropeptides, therefore blocking NOD1/2 sensing (121). Moreover, we found a species-specific mechanism of NOD1 escape. Human and mouse NOD1 do not recognize exactly the same agonists (9). We showed that the peptidoglycan of L. interrogans is devoid of the murine NOD1 agonist but possesses fair amounts of the human NOD1 agonist, suggesting that bacteria in which LipL21 would be degraded or missing could also escape NOD1 sensing in mice but would still signal in human cells. This hypothesis has been confirmed using humanized NOD1 mice infected with the L. interrogans Manilae L495 and lipL21 mutants (121).
The other role of LipL21, its potent inhibition of MPO activity in PMNs (60), is probably not linked to PG binding and NOD1 escape, since experiments with recombinant LipL21 led to similar observations as experiments with membrane fractions of whole bacteria (60).
Therefore, we hypothesize that LipL21 blocks muropeptide release (121) and, along with LipL45, inhibits MPO (60), most likely contributing to neutrophil escape and immune evasion. Because the leptospiral genome encodes more than 140 lipoproteins, most of them with unknown functions (111), we may speculate that other lipoproteins participate in immune evasion.
NLRP3 Inflammasome Activation
The inflammasome is a platform for cytosolic proteins composed of NOD-like protein (NLRP), one or several adaptors such as the protein ASC, and pro-caspase 1. Inflammasome activation results in the secretion of pro-inflammatory IL1β. This cytokine is central to inflammation and is tightly regulated. In mice, two signals are required; the first signal leads to NF-κB activation and mRNA expression of pro-IL1ß and the components of the inflammasome, and the second signal activates the inflammasome through oligomerization of the NLRP proteins and recruitment of the ASC adaptor and pro-caspase 1, which itself is cleaved and activated to convert pro-IL1ß into mature IL1ß (9).
We have shown in murine bone marrow-derived MΦ that leptospires trigger the NLRP3 inflammasome through LPS and lipoprotein activation of TLR2 and TLR4 and downregulation of the potassium pump by glycolipoprotein (GLP) (119). We also showed that leptospiral activation of the inflammasome was mostly limited to NLRP3 and excluded other potential inflammasomes, such as NLRC4/NAIP5, which recognize flagellin. NLRP3-dependent IL1β secretion was confirmed in a recent study showing that doxycyclin reduced IL1β secretion in J774 murine MΦ cells infected with leptospires (137). Subsequently, it was shown that leptospires also trigger the NLRP3 inflammasome in the THP1 human MΦ cell line (120). The mechanism of activation was not investigated but revealed the contribution of ROS and cathepsin B, which was not found in mice.
Species Specificity of PRR/Leptospire Recognition
One key point to consider is the species specificity of the innate immune responses to leptospiral MAMPs. Several studies presented in this review highlighted differences in the recognition of leptospires between different hosts that could contribute to species-specific sensitivity to the disease (Figure 4). For example, the intracellular fate of leptospires in phagocytes (20) as well as TLR4, TLR5 and NOD1 recognition (115, 117, 118, 121) differ between humans and mice. Recent studies on neutrophil and platelet activation by leptospires also suggest differences between humans and rats or rabbits (54–56, 64). In addition, we also highlighted a difference in TLR5 sensing in mice, in which TLR5 does not sense leptospiral flagellins, and cows, in which TLR5 can sense them, as in humans (118). Recognition of PRRs of leptospires by TLRs involved in nucleic sensing, including TLR3, TLR7, TLR8, TLR9, and those recognizing microbial RNAs and DNA, as well as other cytosolic sensors should also be considered.
Part III—TLR/NLR Agonists Boost Phagocytic Responses Against Leptospires
Considering the previous section and the different PRRs involved in leptospire escape, the use of TLR and NLR agonists to boost or restore deficient leptospire responses is attractive. A few studies have shown that their use is effective in combating leptospirosis (Figure 5).
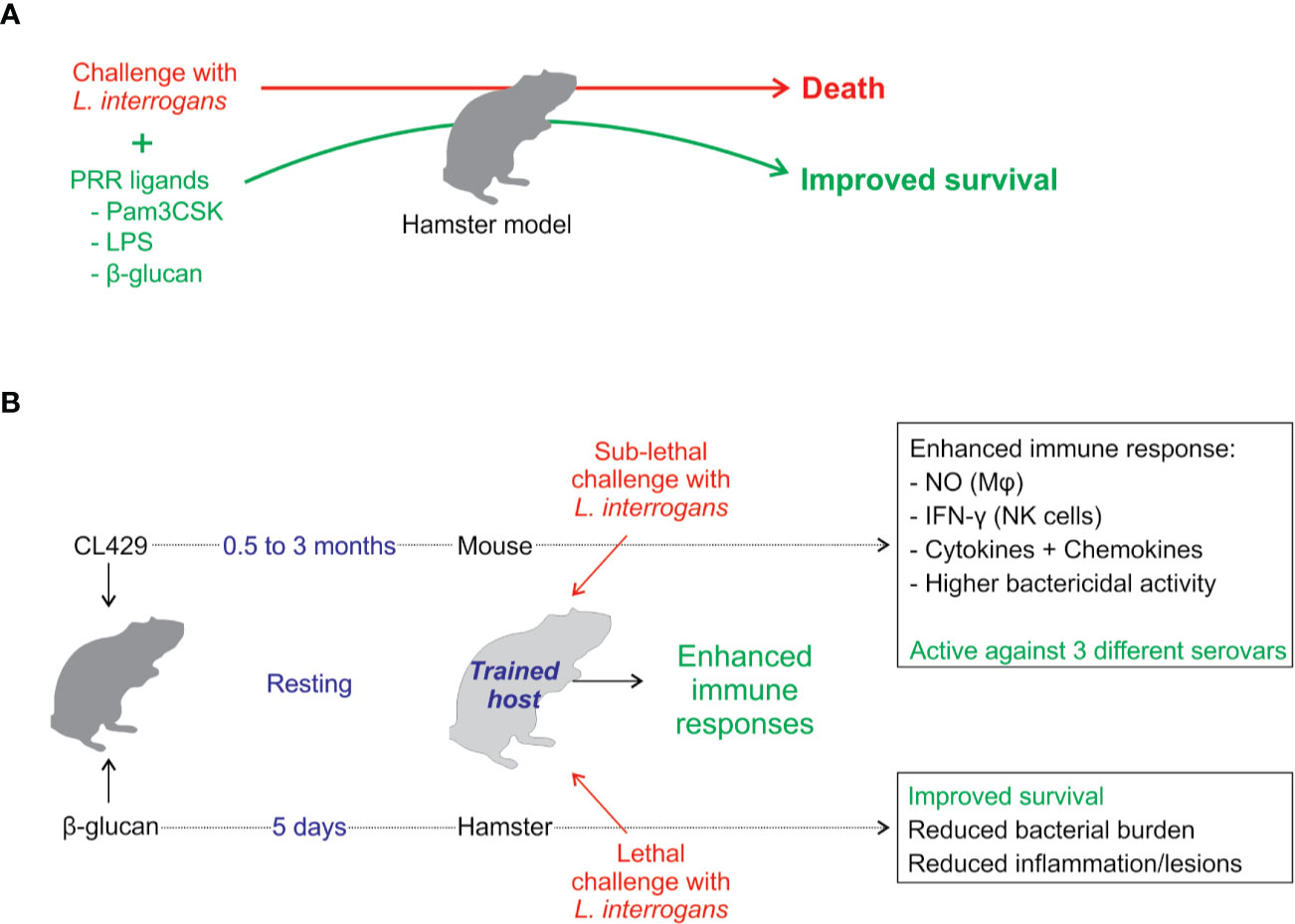
Figure 5 Enhancement of the immune system to better control leptospires. (A) Coinjection at the time of leptospiral infection of PRR agonists such as Pam3CSK (a TLR2 agonist) (138), β-glucan (a Dectin-1 agonist that potentially synergizes with TLR2) (139), or crude Escherichia coli LPS (a TLR2/TLR4 agonist) (126) leads to enhancement of hamster immune responses along with improved survival or delayed lethality and reductions in the number of survivors in tissue lesions, bacterial loads, and inflammation. (B) Recently, innate immune memory or trained immunity has been explored as a therapeutic strategy against leptospires. The TLR2/NOD2 agonist CL429 was used to treat mice before infection (17). After CL429 treatment, the animals were challenged with L. interrogans, and the treated group showed improved resolution of the acute phase of infection. Interestingly, CL429 treatment leads to enhanced antibacterial activity in macrophages characterized by enhanced production of antimicrobial compounds such as nitric oxide (NO). Moreover, the treatment has systemic effects that enhance the cellular response in the bone marrow and in spleen NK cells. Overall, this effect lasts for at least 3 months posttreatment, and ex vivo data suggest that it could be used to alleviate human leptospirosis. Another study used β-glucan (139), a main component of the fungal cell wall, to treat hamsters 5 days before infection. β-glucan pretreatment leads to reduced mortality and decreased bacterial burden in blood and target organs as well as a reduction in tissue lesions and inflammation (139).
Coinjection of TLR or PRR Agonists
Several recent studies from the Cao group used PRR agonists at the time of infection or 1 day prior to the infection, which showed some protective effects in hamsters, a sensitive animal model of leptospirosis (34).
First, it was shown that early expression of TLR2 (but not TLR4) was observed upon leptospiral infection in resistant BALB/c mice, whereas delayed expression was observed in sensitive hamsters (138). This confirmed a previous study from Matsui et al., suggesting that the early triggering of inflammatory mediators in mice infected with leptospires was protective, whereas the delayed response in hamsters could participate in a cytokine storm (140). Interestingly, anti-inflammatory IL-10 cytokine secretion was shown to be dependent on TLR2 in mice (140). The coinjection of hamsters with L. interrogans serovar Autumnalis and Pam3cysSK4, a synthetic agonist of TLR2, alleviated acute leptospirosis and improved the survival of hamsters, which showed reduced leptospiral loads and histopathological lesions in organs 3 weeks pi (138).
More recently, the same group investigated the effect of crude E. coli LPS administered in hamsters after infection with leptospires and showed an improved outcome in hamsters, which was correlated with increased inflammation levels (126). However, the use of nonrepurified crude E. coli LPS, which is known to activate both TLR2 and TLR4 (126, 141), made the interpretation of the relative contributions of TLR4 and TLR2 difficult. Nonetheless, an interesting observation is that upon leptospiral infection, cotreatment with E. coli LPS dramatically enhanced both NO secretion and MPO activity. However, in vivo pharmacological inhibition of NO did not impact the outcome due to LPS (119), suggesting that the enhanced or restored MPO activity (or activity of other mediators) could be responsible for protection. Interestingly, coinjections starting 1 day prior to the time of leptospiral infection with ß-glucan, a fungal cell wall component agonist of the Dectin-1 receptor known to synergize with TLR2, also increased early inflammation and improved survival (139).
Prophylactic Use of TLR and NLR Agonists
By attempting to use Lactobacillus plantarum, a generally recognized as safe (GRAS) organism, as a platform to express leptospiral proteins to immunize sensitive C3H/HeJ mice through the oral route, we observed unanticipated protective effects. Indeed, after a 6-week regimen of intermittent oral gavage, it was shown that treatment with the parental L. plantarum strain protected mice from acute leptospirosis in the sensitive C3H/HeJ TLR4-deficient model (142). The symptoms of leptospirosis were alleviated, and 15 days postleptospiral challenge, the effect of L. plantarum was associated with the recruitment of macrophage-like cells in lymphoid organs and kidneys (142). L. plantarum did not prevent renal colonization, but the treatment reduced inflammation and renal fibrosis. Because these features were reminiscent of the newly introduced concept of “innate immune memory”, also known as “trained immunity,” during which a first infection or MAMP stimulation triggers metabolic and epigenetic reprogramming of MΦ and NK cells to cause them to more robustly respond upon a second challenge, we hypothesized that L. plantarum triggered such a mechanism. We tested the effect of two intraperitoneal injections of CL429, a dual TLR2-NOD2 agonist that was shown by others to recapitulate the protective and anti-inflammatory effects of oral L. plantarum in mice in a model of viral pulmonary infection (143). We demonstrated that CL429 pretreatment indeed induced an innate memory effect independent of B and T cells in MΦ from the peritoneal cavity but also in distant sites, such as the bone marrow and NK cells from spleen (17). CL429 pretreatment enhances the MΦ secretion of cytokines and NO and IFNγ production by NK cells. Notably, the CL429 effect lasted for at least 3 months posttreatment and was effective against the 3 L. interrogans serovars tested (17) (Figure 5). In addition, CL429 also triggered a trained immunity effect in human MΦ derived from monocytes of healthy blood donors (17). A recent study showed that ß-glucan, the prototypic MAMP used to induce trained immunity, was consistently effective in preventing severe leptospirosis in hamsters when administered 5 days prior to infection with L. interrogans serovar Lai strain 56601 (139) (Figure 5). ß-glucan protected 37.5% of hamsters from death and alleviated lesions in the liver, lungs, and kidneys (139).
Conclusions: Current Gaps and Future Directions
Phagocytosis plays an important role in linking innate and adaptive immune responses (144). PRR activation is intricately connected to different phagocytic and platelet functions (65, 145, 146). The consequences of leptospiral PRR escape or recognition may directly influence phagocytic functions, which in turn may influence the shaping of adaptive responses.
MΦ and neutrophils barely control pathogenic leptospires, which largely escape phagocytosis. Strikingly, these responses vary between phagocytes and hosts. Indeed, although neutrophils are the primary professional bactericidal cells, they are unexpectedly even less active than MΦ in controlling Leptospira in naive hosts (62, 63, 94, 99). Bacterial adhesion and motility are key steps in the infection process, and pathogenic leptospires adhere to neutrophils (55, 56) and MΦ but only enter MΦ (18, 20–25). In contrast, it has been established that opsonization with immune serum significantly enhances bacterial uptake and killing in human and mouse MΦ (11–15, 147) but not in human PMN (55, 56), although this could be different for rat PMN (54). The effective response upon opsonization is an interesting point highlighting the fact that leptospires may avoid but do not deactivate phagocyte functions. Nevertheless, considering the newly acquired knowledge of trained immunity and the effect of priming phagocytes, the fact that many opsonization and internalization experiments have been performed with preactivation of cells with casein/NaCl, starch or thioglycolate (148) (Tables 1, 4), which are all known to elicit increases in cells in the peritoneal cavity and to boost MΦ function, brings into question the physiological relevance of the corresponding data. Hence, a gap exists in the knowledge of the in vitro features of PRR escape in leptospires and the physiological consequences of phagocyte function for leptospires. The precise mechanisms and the consequences for adaptive responses remain to be studied. In addition, considering the host specificity of PRR recognition in leptospires, additional infection experiments using different animal models and primary cells are mandatory to assess the functions of phagocytes during leptospirosis.
Although many studies have been performed on MΦ/neutrophils, almost nothing is known about DC recognition of leptospires. Since the skin and mucosa are the first organs to be infected by leptospires, Langerhans DCs are the first antigen presenting cells to be in contact with leptospires. DCs should be crucial in alerting the host to the presence of invading leptospires through PRR sensing and in digesting the bacteria and presenting antigens to naive T cells. Since the adaptive immune response to leptospires is believed to mostly rely on a T cell–independent strong humoral response against LPS that is not durable (112), we may speculate that leptospires may somehow overcome the DC response. The process of DC recognition and antigen presentation of leptospires in different hosts is currently under investigation. Hopefully, this will also increase our understanding of the poor immune responses towards leptospires that allow for chronic renal carriage and shedding of leptospires. Whether leptospires trigger memory B and T cell immunity in relation with PRR recognition or escape is an important question that should be addressed in different hosts and, if possible, with primary cells.
Hopefully, an improved understanding of the immunobiology and function of phagocytes in the context of leptospirosis may help to exploit the ability of phagocytes to fight leptospires and aid in the development of novel therapeutics. Recent results presented here suggest that the use of PRR agonists targeting phagocytes could constitute a novel strategy to fight leptospirosis. Trained immunity using some PRR agonists seems to be a promising prophylactic tool to alleviate acute leptospirosis, but it remains to be tested in animals other than mice and hamsters, such as cattle. In addition, it also remains to be understood whether such strategies may be beneficial to shape a more robust adaptive cellular immunological response against Leptospira. In addition to prophylaxis, it will also be important to study whether such strategies could be used to treat active infections either at the acute phase during leptospiral dissemination in blood or at the chronic phase of renal colonization. These PRR-based strategies may also promote the design of novel and efficient vaccines to fight leptospirosis, a neglected re-emerging zoonotic disease for which only a few serovar-specific and short-lived vaccines are currently available.
Data Availability Statement
All datasets presented in this study are included in the article/supplementary material.
Author Contributions
IS, FF, MV, and RG contributed to the writing of part I. IS conceived the tables and did the figures. CW wrote the original draft. All authors contributed to the article and approved the submitted version.
Funding
This work was supported by the Institut Pasteur grant PTR2017-66 (CW). This is part of the Ph.D. thesis of IS, a fellow from the PPU program with funding from Institut Carnot Pasteur Microbes et Santé. IS also received a 4th year Ph.D salary from the Fondation pour la Recherche Médicale (FDT201805005258). This work was supported by grants PICT 2016-1740 (RG) and PICT 2016-2608 (MF) from the Agencia Nacional de Promoción Científica y Tecnológica (ANPCyT) and PPID X037 (MF) from UNLP, Argentina. The funders had no role in study design, data collection and interpretation, or the decision to submit the work for publication.
Conflict of Interest
The authors declare that the research was conducted in the absence of any commercial or financial relationships that could be construed as a potential conflict of interest.
The handling editor declared a past co-authorship with several of the authors RG, MF.
References
1. Ko AI, Goarant C, Picardeau M. Leptospira: the dawn of the molecular genetics era for an emerging zoonotic pathogen. Nat Rev Microbiol (2009) 7:736–47. doi: 10.1038/nrmicro2208
2. Costa F, Hagan JE, Calcagno J, Kane M, Torgerson P, Martinez-Silveira MS, et al. Global Morbidity and Mortality of Leptospirosis: A Systematic Review. PloS Negl Trop Dis (2015) 9:e0003898. doi: 10.1371/journal.pntd.0003898
3. Merle NS, Church SE, Fremeaux-Bacchi V, Roumenina LT. Complement System Part I - Molecular Mechanisms of Activation and Regulation. Front Immunol (2015) 6:262. doi: 10.3389/fimmu.2015.00262
4. Merle NS, Noe R, Halbwachs-Mecarelli L, Fremeaux-Bacchi V, Roumenina LT, Complement System Part II. Role in Immunity. Front Immunol (2015) 6:257. doi: 10.3389/fimmu.2015.00257
5. Gordon S. Phagocytosis: An Immunobiologic Process. Immunity (2016) 44:463–75. doi: 10.1016/j.immuni.2016.02.026
6. Stuart LM, Ezekowitz RA. Phagocytosis: elegant complexity. Immunity (2005) 22:539–50. doi: 10.1016/j.immuni.2005.05.002
7. Hirayama D, Iida T, Nakase H. The Phagocytic Function of Macrophage-Enforcing Innate Immunity and Tissue Homeostasis. Int J Mol Sci (2017) 19. doi: 10.3390/ijms19010092
8. Takeuchi O, Akira S. Pattern recognition receptors and inflammation. Cell (2010) 140:805–20. doi: 10.1016/j.cell.2010.01.022
9. Werts C, Girardin SE, Philpott DJ. TIR, CARD and PYRIN: three domains for an antimicrobial triad. Cell Death Differ (2006) 13:798–815. doi: 10.1038/sj.cdd.4401890
10. Cinco M, Banfi E, Soranzo MR. Studies on the interaction between macrophages and leptospires. J Gen Microbiol (1981) 124:409–13. doi: 10.1099/00221287-124-2-409
11. Banfi E, Cinco M, Bellini M, Soranzo MR. The role of antibodies and serum complement in the interaction between macrophages and leptospires. J Gen Microbiol (1982) 128:813–6. doi: 10.1099/00221287-128-4-813
12. Tu V, Adler B, Faine S. The role of macrophages in the protection of mice against leptospirosis: in vitro and in vivo studies. Pathology (1982) 14:463–8. doi: 10.3109/00313028209092128
13. Wang B, Sullivan JA, Sullivan GW, Mandell GL. Role of specific antibody in interaction of leptospires with human monocytes and monocyte-derived macrophages. Infect Immun (1984) 46:809–13. doi: 10.1128/IAI.46.3.809-813.1984
14. Isogai E, Kitagawa H, Isogai H, Kurebayashi Y, Ito N. Phagocytosis as a defense mechanism against infection with Leptospira. Zentralbl Bakteriol Mikrobiol Hyg A (1986) 261:65–74. doi: 10.1016/S0176-6724(86)80063-3
15. Isogai E, Isogai H, Fujii N, Oguma K. Macrophage activation by leptospiral lipopolysaccharide. Zentralbl Bakteriol (1990) 273:200–8:. doi: 10.1016/S0934-8840(11)80250-1
16. Davis JM, Haake DA, Ramakrishnan L. Leptospira interrogans stably infects zebrafish embryos, altering phagocyte behavior and homing to specific tissues. PloS Negl Trop Dis (2009) 3:e463. doi: 10.1371/journal.pntd.0000463
17. Santecchia I, Vernel-Pauillac F, Rasid O, Quintin J, Gomes-Solecki M, Boneca IG, et al. Innate immune memory through TLR2 and NOD2 contributes to the control of Leptospira interrogans infection. PloS Pathog (2019) 15:e1007811. doi: 10.1371/journal.ppat.1007811
18. Merien F, Baranton G, Perolat P. Invasion of Vero cells and induction of apoptosis in macrophages by pathogenic Leptospira interrogans are correlated with virulence. Infect Immun (1997) 65:729–38. doi: 10.1128/IAI.65.2.729-738.1997
19. Liu Y, Zheng W, Li L, Mao Y, Yan J. Pathogenesis of leptospirosis: interaction of Leptospira interrogans with in vitro cultured mammalian cells. Med Microbiol Immunol (2007) 196:233–9. doi: 10.1007/s00430-007-0047-0
20. Li S, Ojcius DM, Liao S, Li L, Xue F, Dong H, et al. Replication or death: distinct fates of pathogenic Leptospira strain Lai within macrophages of human or mouse origin. Innate Immun (2010) 16:80–92. doi: 10.1177/1753425909105580
21. Hu W, Ge Y, Ojcius DM, Sun D, Dong H, Yang XF, et al. p53 signalling controls cell cycle arrest and caspase-independent apoptosis in macrophages infected with pathogenic Leptospira species. Cell Microbiol (2013) 15:1642–59. doi: 10.1111/cmi.12141
22. Zhao JF, Chen HH, Ojcius DM, Zhao X, Sun D, Ge YM, et al. Identification of Leptospira interrogans phospholipase C as a novel virulence factor responsible for intracellular free calcium ion elevation during macrophage death. PloS One (2013) 8:e75652. doi: 10.1371/journal.pone.0075652
23. Li L, Ojcius DM, Yan J. Comparison of invasion of fibroblasts and macrophages by high- and low-virulence Leptospira strains: colonization of the host-cell nucleus and induction of necrosis by the virulent strain. Arch Microbiol (2007) 188:591–8. doi: 10.1007/s00203-007-0280-3
24. Toma C, Okura N, Takayama C, Suzuki T. Characteristic features of intracellular pathogenic Leptospira in infected murine macrophages. Cell Microbiol (2011) 13:1783–92. doi: 10.1111/j.1462-5822.2011.01660.x
25. Toma C, Murray GL, Nohara T, Mizuyama M, Koizumi N, Adler B, et al. Leptospiral outer membrane protein LMB216 is involved in enhancement of phagocytic uptake by macrophages. Cell Microbiol (2014) 16:1366–77. doi: 10.1111/cmi.12296
26. Nagel A, Vazquez CL, Etulain J, Blanco FC, Gravisaco MJ, Gomez RM, et al. Bovine macrophages responses to the infection with virulent and attenuated Leptospira interrogans serovar Pomona. Vet Microbiol (2019) 233:124–32. doi: 10.1016/j.vetmic.2019.04.033
27. Jin D, Ojcius DM, Sun D, Dong H, Luo Y, Mao Y, et al. Leptospira interrogans induces apoptosis in macrophages via caspase-8- and caspase-3-dependent pathways. Infect Immun (2009) 77:799–809. doi: 10.1128/IAI.00914-08
28. Johnson RC, Muschel LH. Antileptospiral activity of serum. I. Normal and immune serum. J Bacteriol (1966) 91:1403–9. doi: 10.1128/JB.91.4.1403-1409.1966
29. Stephens J, Lewin E. Serum Enzyme Variations and Histological Abnormalities in the Carrier State in Duchenne Dystrophy. J Neurol Neurosurg Psychiatry (1965) 28:104–8. doi: 10.1136/jnnp.28.2.104
30. Fraga TR, Barbosa AS, Isaac L. Leptospirosis: aspects of innate immunity, immunopathogenesis and immune evasion from the complement system. Scand J Immunol (2011) 73:408–19. doi: 10.1111/j.1365-3083.2010.02505.x
31. Fraga TR, Isaac L, Barbosa AS. Complement Evasion by Pathogenic Leptospira. Front Immunol (2016) 7:623. doi: 10.3389/fimmu.2016.00623
32. Barbosa AS, Isaac L. Complement Immune Evasion by Spirochetes. Curr Top Microbiol Immunol (2018) 415:215–38. doi: 10.1007/82_2017_47
33. Lovewell RR, Patankar YR, Berwin B. Mechanisms of phagocytosis and host clearance of Pseudomonas aeruginosa. Am J Physiol Lung Cell Mol Physiol (2014) 306:L591–603. doi: 10.1152/ajplung.00335.2013
34. Gomes-Solecki M, Santecchia I, Werts C. Animal Models of Leptospirosis: Of Mice and Hamsters. Front Immunol (2017) 8:58. doi: 10.3389/fimmu.2017.00058
36. Li Q, Jagannath C, Rao PK, Singh CR, Lostumbo G. Analysis of phagosomal proteomes: from latex-bead to bacterial phagosomes. Proteomics (2010) 10:4098–116. doi: 10.1002/pmic.201000210
37. Stenmark H. Rab GTPases as coordinators of vesicle traffic. Nat Rev Mol Cell Biol (2009) 10:513–25. doi: 10.1038/nrm2728
38. Woodman PG. Biogenesis of the sorting endosome: the role of Rab5. Traffic (2000) 1:695–701. doi: 10.1034/j.1600-0854.2000.010902.x
39. Gillooly DJ, Simonsen A, Stenmark H. Phosphoinositides and phagocytosis. J Cell Biol (2001) 155:15–7. doi: 10.1083/jcb.200109001
40. Gutierrez MG. Functional role(s) of phagosomal Rab GTPases. Small GTPases (2013) 4:148–58. doi: 10.4161/sgtp.25604
41. Simonsen A, Lippe R, Christoforidis S, Gaullier JM, Brech A, Callaghan J, et al. EEA1 links PI(3)K function to Rab5 regulation of endosome fusion. Nature (1998) 394:494–8. doi: 10.1038/28879
42. Christoforidis S, McBride HM, Burgoyne RD, Zerial M. The Rab5 effector EEA1 is a core component of endosome docking. Nature (1999) 397:621–5. doi: 10.1038/17618
43. Huynh KK, Eskelinen EL, Scott CC, Malevanets A, Saftig P, Grinstein S. LAMP proteins are required for fusion of lysosomes with phagosomes. EMBO J (2007) 26:313–24. doi: 10.1038/sj.emboj.7601511
44. Mindell JA. Lysosomal acidification mechanisms. Annu Rev Physiol (2012) 74:69–86. doi: 10.1146/annurev-physiol-012110-142317
45. Pryor PR, Raines SA. Manipulation of the host by pathogens to survive the lysosome. Biochem Soc Trans (2010) 38:1417–9. doi: 10.1042/BST0381417
46. Flannagan RS, Cosio G, Grinstein S. Antimicrobial mechanisms of phagocytes and bacterial evasion strategies. Nat Rev Microbiol (2009) 7:355–66. doi: 10.1038/nrmicro2128
47. Seto S, Tsujimura K, Koide Y. Rab GTPases regulating phagosome maturation are differentially recruited to mycobacterial phagosomes. Traffic (2011) 12:407–20. doi: 10.1111/j.1600-0854.2011.01165.x
48. Smith AC, Heo WD, Braun V, Jiang X, Macrae C, Casanova JE, et al. A network of Rab GTPases controls phagosome maturation and is modulated by Salmonella enterica serovar Typhimurium. J Cell Biol (2007) 176:263–8. doi: 10.1083/jcb.200611056
49. Kortebi M, Milohanic E, Mitchell G, Pechoux C, Prevost MC, Cossart P, et al. Listeria monocytogenes switches from dissemination to persistence by adopting a vacuolar lifestyle in epithelial cells. PloS Pathog (2017) 13:e1006734. doi: 10.1371/journal.ppat.1006734
50. Moldovan A, Fraunholz MJ. In or out: Phagosomal escape of Staphylococcus aureus. Cell Microbiol (2019) 21:e12997. doi: 10.1111/cmi.12997
51. Duprez L, Wirawan E, Vanden Berghe T, Vandenabeele P. Major cell death pathways at a glance. Microbes Infect (2009) 11:1050–62. doi: 10.1016/j.micinf.2009.08.013
52. Navarre WW, Zychlinsky A. Pathogen-induced apoptosis of macrophages: a common end for different pathogenic strategies. Cell Microbiol (2000) 2:265–73. doi: 10.1046/j.1462-5822.2000.00056.x
53. Chow SH, Deo P, Naderer T. Macrophage cell death in microbial infections. Cell Microbiol (2016) 18:466–74. doi: 10.1111/cmi.12573
54. Isogai E, Isogai H, Wakizaka H, Miura H, Kurebayashi Y. Chemiluminescence and phagocytic responses of rat polymorphonuclear neutrophils to leptospires. Zentralbl Bakteriol (1989) 272:36–46. doi: 10.1016/S0934-8840(89)80090-8
55. Charo N, Scharrig E, Ferrer MF, Sanjuan N, Carrera Silva EA, Schattner M, et al. Leptospira species promote a pro-inflammatory phenotype in human neutrophils. Cell Microbiol (2019) 21:e12990. doi: 10.1111/cmi.12990
56. Wang B, Sullivan J, Sullivan GW, Mandell GL. Interaction of leptospires with human polymorphonuclear neutrophils. Infect Immun (1984) 44:459–64. doi: 10.1128/IAI.44.2.459-464.1984
57. Wilson-Welder JH, Frank AT, Hornsby RL, Olsen SC, Alt DP. Interaction of Bovine Peripheral Blood Polymorphonuclear Cells and Leptospira Species; Innate Responses in the Natural Bovine Reservoir Host. Front Microbiol (2016) 7:1110. doi: 10.3389/fmicb.2016.01110
58. Vieira ML, Persson S, Lopes-Ferreira M, Romero EC, Kirchgatter K, Nascimento A, et al. Heparin-Binding Protein Release Is Strongly Induced by Leptospira Species and Is a Candidate for an Early Diagnostic Marker of Human Leptospirosis. J Infect Dis (2019) 219:996–1006. doi: 10.1093/infdis/jiy589
59. Pretre G, Lapponi MJ, Atzingen MV, Schattner M, Nascimento AL, Gomez RM. Characterization of LIC11207, a novel leptospiral protein that is recognized by human convalescent sera and prevents apoptosis of polymorphonuclear leukocytes. Microb Pathog (2013) 56:21–8. doi: 10.1016/j.micpath.2012.10.002
60. Vieira ML, Teixeira AF, Pidde G, Ching ATC, Tambourgi DV, Nascimento A, et al. Leptospira interrogans outer membrane protein LipL21 is a potent inhibitor of neutrophil myeloperoxidase. Virulence (2018) 9:414–25. doi: 10.1080/21505594.2017.1407484
61. Murgia R, Garcia R, Cinco M. Leptospires are killed in vitro by both oxygen-dependent and -independent reactions. Infect Immun (2002) 70:7172–5. doi: 10.1128/iai.70.12.7172-7175.2002
62. Chen X, Li SJ, Ojcius DM, Sun AH, Hu WL, Lin X, et al. Mononuclear-macrophages but not neutrophils act as major infiltrating anti-leptospiral phagocytes during leptospirosis. PloS One (2017) 12:e0181014. doi: 10.1371/journal.pone.0181014
63. Scharrig E, Carestia A, Ferrer MF, Cedola M, Pretre G, Drut R, et al. Neutrophil Extracellular Traps are Involved in the Innate Immune Response to Infection with Leptospira. PloS Negl Trop Dis (2015) 9:e0003927. doi: 10.1371/journal.pntd.0003927
64. Isogai E, Hirose K, Kimura K, Hayashi S, Kubota T, Fujii N, et al. Role of platelet-activating-factor (PAF) on cellular responses after stimulation with leptospire lipopolysaccharide. Microbiol Immunol (1997) 41:271–5. doi: 10.1111/j.1348-0421.1997.tb01200.x
65. Gómez RM, López Ortiz AO, Schattner M. Platelets and Extracellular Traps in Infections. Platelets (2020) 1–9. doi: 10.1080/09537104.2020.1718631
66. Santos JL, Andrade AA, Dias AA, Bonjardim CA, Reis LF, Teixeira SM, et al. Differential sensitivity of C57BL/6 (M-1) and BALB/c (M-2) macrophages to the stimuli of IFN-gamma/LPS for the production of NO: correlation with iNOS mRNA and protein expression. J Interferon Cytokine Res (2006) 26:682–8. doi: 10.1089/jir.2006.26.682
67. Depke M, Breitbach K, Dinh Hoang Dang K, Brinkmann L, Salazar MG, Dhople VM, et al. Bone marrow-derived macrophages from BALB/c and C57BL/6 mice fundamentally differ in their respiratory chain complex proteins, lysosomal enzymes and components of antioxidant stress systems. J Proteomics (2014) 103:72–86. doi: 10.1016/j.jprot.2014.03.027
68. Keane J, Remold HG, Kornfeld H. Virulent Mycobacterium tuberculosis strains evade apoptosis of infected alveolar macrophages. J Immunol (2000) 164:2016–20. doi: 10.4049/jimmunol.164.4.2016
69. Liu Y, Mi Y, Mueller T, Kreibich S, Williams EG, Van Drogen A, et al. Multi-omic measurements of heterogeneity in HeLa cells across laboratories. Nat Biotechnol (2019) 37:314–22. doi: 10.1038/s41587-019-0037-y
70. Ley K, Hoffman HM, Kubes P, Cassatella MA, Zychlinsky A, Hedrick CC, et al. Neutrophils: New insights and open questions. Sci Immunol (2018) 3:eaat4579. doi: 10.1126/sciimmunol.aat4579
71. Mantovani A, Cassatella MA, Costantini C, Jaillon S. Neutrophils in the activation and regulation of innate and adaptive immunity. Nat Rev Immunol (2011) 11:519–31. doi: 10.1038/nri3024
72. McGrath H, Adler B, Vinh T, Faine S. Phagocytosis of virulent and avirulent leptospires by guinea-pig and human polymorphonuclear leukocytes in vitro. Pathology (1984) 16:243–9. doi: 10.3109/00313028409068531
73. Aratani Y. Myeloperoxidase: Its role for host defense, inflammation, and neutrophil function. Arch Biochem Biophys (2018) 640:47–52. doi: 10.1016/j.abb.2018.01.004
74. Gautam N, Olofsson AM, Herwald H, Iversen LF, Lundgren-Akerlund E, Hedqvist P, et al. Heparin-binding protein (HBP/CAP37): a missing link in neutrophil-evoked alteration of vascular permeability. Nat Med (2001) 7:1123–7. doi: 10.1038/nm1001-1123
75. Cognasse F, Laradi S, Berthelot P, Bourlet T, Marotte H, Mismetti P, et al. Platelet Inflammatory Response to Stress. Front Immunol (2019) 10, 1478. doi: 10.3389/fimmu.2019.01478
76. van der Meijden PEJ, Heemskerk JWM. Platelet biology and functions: new concepts and clinical perspectives. Nat Rev Cardiol (2019) 16:166–79. doi: 10.1038/s41569-018-0110-0
77. Hillion S, Arleevskaya MI, Blanco P, Bordron A, Brooks WH, Cesbron JY, et al. The Innate Part of the Adaptive Immune System. Clin Rev Allergy Immunol (2020) 58(2):151–4. doi: 10.1007/s12016-019-08740-1
78. Gaertner F, Ahmad Z, Rosenberger G, Fan S, Nicolai L, Busch B, et al. Migrating Platelets Are Mechano-scavengers that Collect and Bundle Bacteria. Cell (2017) 171:1368–82. doi: 10.1016/j.cell.2017.11.001
79. Hoylaerts MF, Vanassche T, Verhamme P. Bacterial killing by platelets: making sense of (H)IT. J Thromb Haemost (2018) 16:1182–6. doi: 10.1111/jth.14012
80. D’ Atri LP, Schattner M. Platelet toll-like receptors in thromboinflammation. Front Biosci (Landmark Ed) (2017) 22:1867–83. doi: 10.2741/4576
81. Claushuis TAM, Van Der Veen AIP, Horn J, Schultz MJ, Houtkooper RH, Van Der Poll T. Platelet Toll-like receptor expression and activation induced by lipopolysaccharide and sepsis. Platelets (2019) 30:296–304. doi: 10.1080/09537104.2018.1445841
82. Schattner M. Platelet TLR4 at the crossroads of thrombosis and the innate immune response. J Leukoc Biol (2019) 105:873–80. doi: 10.1002/JLB.MR0618-213R
83. Swystun LL, Liaw PC. The role of leukocytes in thrombosis. Blood (2016) 128:753–62. doi: 10.1182/blood-2016-05-718114
84. Wong CH, Jenne CN, Petri B, Chrobok NL, Kubes P. Nucleation of platelets with blood-borne pathogens on Kupffer cells precedes other innate immunity and contributes to bacterial clearance. Nat Immunol (2013) 14:785–92. doi: 10.1038/ni.2631
85. Li C, Li J, Li Y, Lang S, Yougbare I, Zhu G, et al. Crosstalk between Platelets and the Immune System: Old Systems with New Discoveries. Adv Hematol (2012) 2012:384685. doi: 10.1155/2012/384685
86. Isogai E, Kitagawa H, Isogai H, Matsuzawa T, Shimizu T, Yanagihara Y, et al. Effects of leptospiral lipopolysaccharide on rabbit platelets. Zentralbl Bakteriol (1989) 271:186–96. doi: 10.1016/S0934-8840(89)80072-6
87. Lin YP, McDonough SP, Sharma Y, Chang YF. Leptospira immunoglobulin-like protein B (LigB) binding to the C-terminal fibrinogen alphaC domain inhibits fibrin clot formation, platelet adhesion and aggregation. Mol Microbiol (2011) 79:1063–76. doi: 10.1111/j.1365-2958.2010.07510.x
88. Fang JQ, Imran M, Hu WL, Ojcius DM, Li Y, Ge YM, et al. vWA proteins of Leptospira interrogans induce hemorrhage in leptospirosis by competitive inhibition of vWF/GPIb-mediated platelet aggregation. EBioMedicine (2018) 37:428–41. doi: 10.1016/j.ebiom.2018.10.033
89. Passalia FJ, Heinemann MB, de Andrade SA, Nascimento A, Vieira ML. Leptospira interrogans Bat proteins impair host hemostasis by fibrinogen cleavage and platelet aggregation inhibition. Med Microbiol Immunol (2020) 209:201–13. doi: 10.1007/s00430-020-00664-4
90. Vieira ML, Herwald H, Nascimento A. The interplay between host haemostatic systems and Leptospira spp. Infect Crit Rev Microbiol (2020) 46:121–35. doi: 10.1080/1040841X.2020.1735299
91. Gaudart N, Ekpo P, Pattanapanyasat K, van Kooyk Y, Engering A. Leptospira interrogans is recognized through DC-SIGN and induces maturation and cytokine production by human dendritic cells. FEMS Immunol Med Microbiol (2008) 53:359–67. doi: 10.1111/j.1574-695X.2008.00437.x
92. Ferrer MF, Scharrig E, Charo N, Ripodas AL, Drut R, Carrera Silva EA, et al. Macrophages and Galectin 3 Control Bacterial Burden in Acute and Subacute Murine Leptospirosis That Determines Chronic Kidney Fibrosis. Front Cell Infect Microbiol (2018) 8:384. doi: 10.3389/fcimb.2018.00384
93. Craig SB, Graham GC, Burns MA, Dohnt MF, Smythe LD, McKay DB. Haematological and clinical-chemistry markers in patients presenting with leptospirosis: a comparison of the findings from uncomplicated cases with those seen in the severe disease. Ann Trop Med Parasitol (2009) 103:333–41. doi: 10.1179/136485909X435058
94. Raffray L, Giry C, Vandroux D, Kuli B, Randrianjohany A, Pequin AM, et al. Major Neutrophilia Observed in Acute Phase of Human Leptospirosis Is Not Associated with Increased Expression of Granulocyte Cell Activation Markers. PloS One (2016) 11:e0165716. doi: 10.1371/journal.pone.0165716
95. Lindow JC, Wunder EA Jr., Popper SJ, Min JN, Mannam P, Srivastava A, et al. Cathelicidin Insufficiency in Patients with Fatal Leptospirosis. PloS Pathog (2016) 12:e1005943. doi: 10.1371/journal.ppat.1005943
96. De Silva NL, Niloofa M, Fernando N, Karunanayake L, Rodrigo C, De Silva HJ, et al. Changes in full blood count parameters in leptospirosis: a prospective study. Int Arch Med (2014) 7:31. doi: 10.1186/1755-7682-7-31
97. Craig SB, Collet TA, Wynwood SJ, Smythe LD, Weier SL, McKay DB. Neutrophil counts in leptospirosis patients infected with different serovars. Trop BioMed (2013) 30:579–83.
98. Srisawat N, Praditpornsilpa K, Patarakul K, Techapornrung M, Daraswang T, Sukmark T, et al. Neutrophil Gelatinase Associated Lipocalin (NGAL) in Leptospirosis Acute Kidney Injury: A Multicenter Study in Thailand. PloS One (2015) 10:e0143367. doi: 10.1371/journal.pone.0143367
99. Lindow JC, Tsay AJ, Montgomery RR, Reis EAG, Wunder EA, Araujo G, et al. Elevated Activation of Neutrophil Toll-Like Receptors in Patients with Acute Severe Leptospirosis: An Observational Study. Am J Trop Med Hyg (2019) 101:585–9. doi: 10.4269/ajtmh.19-0160
101. Mestas J, Hughes CC. Of mice and not men: differences between mouse and human immunology. J Immunol (2004) 172:2731–8. doi: 10.4049/jimmunol.172.5.2731
102. Tunjungputri RN, Gasem MH, van der Does W, Sasongko PH, Isbandrio B, Urbanus RT, et al. Platelet dysfunction contributes to bleeding complications in patients with probable leptospirosis. PloS Negl Trop Dis (2017) 11:e0005915. doi: 10.1371/journal.pntd.0005915
103. Chierakul W, Tientadakul P, Suputtamongkol Y, Wuthiekanun V, Phimda K, Limpaiboon R, et al. Activation of the coagulation cascade in patients with leptospirosis. Clin Infect Dis (2008) 46:254–60. doi: 10.1086/524664
104. Daher EF, Silva GB, Silveira CO, Falcao FS, Alves MP, Mota JA, et al. Factors associated with thrombocytopenia in severe leptospirosis (Weil’s disease). Clinics (Sao Paulo) (2014) 69:106–10. doi: 10.6061/clinics/2014(02)06
105. Wagenaar JF, Goris MG, Sakundarno MS, Gasem MH, Mairuhu AT, de Kruif MD, et al. What role do coagulation disorders play in the pathogenesis of leptospirosis? Trop Med Int Health (2007) 12:111–22. doi: 10.1111/j.1365-3156.2006.01792.x
106. Wagenaar JF, Goris MG, Partiningrum DL, Isbandrio B, Hartskeerl RA, Brandjes DP, et al. Coagulation disorders in patients with severe leptospirosis are associated with severe bleeding and mortality. Trop Med Int Health (2010) 15:152–9. doi: 10.1111/j.1365-3156.2009.02434.x
107. Edwards CN, Nicholson GD, Hassell TA, Everard CO, Callender J. Thrombocytopenia in leptospirosis: the absence of evidence for disseminated intravascular coagulation. Am J Trop Med Hyg (1986) 35:352–4. doi: 10.4269/ajtmh.1986.35.352
108. Yang HL, Jiang XC, Zhang XY, Li WJ, Hu BY, Zhao GP, et al. Thrombocytopenia in the experimental leptospirosis of guinea pig is not related to disseminated intravascular coagulation. BMC Infect Dis (2006) 6:19. doi: 10.1186/1471-2334-6-19
109. Nally JE, Chantranuwat C, Wu XY, Fishbein MC, Pereira MM, Da Silva JJ, et al. Alveolar septal deposition of immunoglobulin and complement parallels pulmonary hemorrhage in a guinea pig model of severe pulmonary leptospirosis. Am J Pathol (2004) 164:1115–27. doi: 10.1016/S0002-9440(10)63198-7
110. Nicodemo AC, Del Negro G, Amato Neto V. Thrombocytopenia and leptospirosis. Rev Inst Med Trop Sao Paulo (1990) 32:252–9. doi: 10.1590/s0036-46651990000400004
111. Haake DA, Zuckert WR. Spirochetal Lipoproteins in Pathogenesis and Immunity. Curr Top Microbiol Immunol (2018) 415:239–71. doi: 10.1007/82_2017_78
112. Werts C. Interaction of Leptospira with the Innate Immune System. Curr Top Microbiol Immunol (2018) 415:163–87. doi: 10.1007/82_2017_46
113. Que-Gewirth NL, Ribeiro AA, Kalb SR, Cotter RJ, Bulach DM, Adler B, et al. A methylated phosphate group and four amide-linked acyl chains in Leptospira interrogans lipid A. The membrane anchor of an unusual lipopolysaccharide that activates TLR2. J Biol Chem (2004) 279:25420–9. doi: 10.1074/jbc.M400598200
114. Werts C, Tapping RI, Mathison JC, Chuang TH, Kravchenko V, Saint Girons I, et al. Leptospiral lipopolysaccharide activates cells through a TLR2-dependent mechanism. Nat Immunol (2001) 2:346–52. doi: 10.1038/86354
115. Nahori MA, Fournie-Amazouz E, Que-Gewirth NS, Balloy V, Chignard M, Raetz CR, et al. Differential TLR recognition of leptospiral lipid A and lipopolysaccharide in murine and human cells. J Immunol (2005) 175:6022–31. doi: 10.4049/jimmunol.175.9.6022
116. Chassin C, Picardeau M, Goujon JM, Bourhy P, Quellard N, Darche S, et al. TLR4- and TLR2-mediated B cell responses control the clearance of the bacterial pathogen, Leptospira interrogans. J Immunol (2009) 183:2669–77. doi: 10.4049/jimmunol.0900506
117. Bonhomme D, Santecchia I, Vernel-Pauillac F, Caroff M, Germon P, Murray G, et al. Leptospiral LPS escapes mouse TLR4 internalization and TRIF-associated antimicrobial responses through O antigen and associated lipoproteins. PloS Pathog (2020) 16:e1008639. doi: 10.1371/journal.ppat.1008639
118. Holzapfel M, Bonhomme D, Cagliero J, Vernel-Pauillac F, Fanton d’Andon M, Bortolussi S, et al. Escape of TLR5 Recognition by Leptospira spp.: A Rationale for Atypical Endoflagella. Front Immunol (2020) 11, 2007. doi: 10.3389/fimmu.2020.02007
119. Lacroix-Lamande S, d’Andon MF, Michel E, Ratet G, Philpott DJ, Girardin SE, et al. Downregulation of the Na/K-ATPase pump by leptospiral glycolipoprotein activates the NLRP3 inflammasome. J Immunol (2012) 188:2805–14. doi: 10.4049/jimmunol.1101987
120. Li S, Wang M, Ojcius DM, Zhou B, Hu W, Liu Y, et al. Leptospira interrogans infection leads to IL-1beta and IL-18 secretion from a human macrophage cell line through reactive oxygen species and cathepsin B mediated-NLRP3 inflammasome activation. Microbes Infect (2018) 20:254–60. doi: 10.1016/j.micinf.2018.01.010
121. Ratet G, Santecchia I, Fanton d’Andon M, Vernel-Pauillac F, Wheeler R, Lenormand P, et al. LipL21 lipoprotein binding to peptidoglycan enables Leptospira interrogans to escape NOD1 and NOD2 recognition. PloS Pathog (2017) 13:e1006725. doi: 10.1371/journal.ppat.1006725
122. Guo Y, Fukuda T, Donai K, Kuroda K, Masuda M, Nakamura S, et al. Leptospiral lipopolysaccharide stimulates the expression of toll-like receptor 2 and cytokines in pig fibroblasts. Anim Sci J (2015) 86:238–44. doi: 10.1111/asj.12254
123. Guo Y, Fukuda T, Nakamura S, Bai L, Xu J, Kuroda K, et al. Interaction between Leptospiral Lipopolysaccharide and Toll-like Receptor 2 in Pig Fibroblast Cell Line, and Inhibitory Effect of Antibody against Leptospiral Lipopolysaccharide on Interaction. Asian-Australas J Anim Sci (2015) 28:273–9. doi: 10.5713/ajas.14.0440
124. Cedola M, Chiani Y, Pretre G, Alberdi L, Vanasco B, Gomez RM. Association of Toll-like receptor 2 Arg753Gln and Toll-like receptor 1 Ile602Ser single-nucleotide polymorphisms with leptospirosis in an Argentine population. Acta Trop (2015) 146:73–80. doi: 10.1016/j.actatropica.2015.03.007
125. Yang CW, Hung CC, Wu MS, Tian YC, Chang CT, Pan MJ, et al. Toll-like receptor 2 mediates early inflammation by leptospiral outer membrane proteins in proximal tubule cells. Kidney Int (2006) 69:815–22. doi: 10.1038/sj.ki.5000119
126. Zhang W, Xie X, Wang J, Song N, Lv T, Wu D, et al. Increased inflammation with crude E. coli LPS protects against acute leptospirosis in hamsters. Emerg Microbes Infect (2020) 9:140–7. doi: 10.1080/22221751.2019.1710435
127. Esteves LM, Bulhoes SM, Branco CC, Mota FM, Paiva C, Cabral R, et al. Human leptospirosis: seroreactivity and genetic susceptibility in the population of Sao Miguel Island (Azores, Portugal). PloS One (2014) 9:e108534. doi: 10.1371/journal.pone.0108534
128. Viriyakosol S, Matthias MA, Swancutt MA, Kirkland TN, Vinetz JM. Toll-like receptor 4 protects against lethal Leptospira interrogans serovar icterohaemorrhagiae infection and contributes to in vivo control of leptospiral burden. Infect Immun (2006) 74:887–95. doi: 10.1128/IAI.74.2.887-895.2006
129. Murray GL, Srikram A, Henry R, Hartskeerl RA, Sermswan RW, Adler B. Mutations affecting Leptospira interrogans lipopolysaccharide attenuate virulence. Mol Microbiol (2010) 78:701–9. doi: 10.1111/j.1365-2958.2010.07360.x
130. Bonhomme D, Werts C. Purification of lipopolysaccharide (LPS) from Leptospira. In: Koizumi N, Picardeau M, editors. Methods in Molecular Biology: Leptospira spp.- Methods and Protocols. Springer-Verlag New York Inc. ISBN: 9781071604588 (2020). p. 2134.
131. Xia B, Sun L, Fan X, Xiao H, Zhu Y, Qin J, et al. A new model of self-resolving leptospirosis in mice infected with a strain of Leptospira interrogans serovar Autumnalis harboring LPS signaling only through TLR4. Emerg Microbes Infect (2017) 6:e36. doi: 10.1038/emi.2017.16
132. Fanton d’Andon M, Quellard N, Fernandez B, Ratet G, Lacroix-Lamande S, Vandewalle A, et al. Leptospira interrogans induces fibrosis in the mouse kidney through Inos-dependent, TLR- and NLR-independent signaling pathways. PloS Negl Trop Dis (2014) 8:e2664. doi: 10.1371/journal.pntd.0002664
133. Goris MGA, Wagenaar JFP, Hartskeerl RA, van Gorp ECM, Schuller S, Monahan AM, et al. Potent innate immune response to pathogenic Leptospira in human whole blood. PloS One (2011) 6:e18279. doi: 10.1371/journal.pone.0018279
134. Biedma ME, Cayet D, Tabareau J, Rossi AH, Ivicak-Kocjan K, Moreno G, et al. Recombinant flagellins with deletions in domains D1, D2, and D3: Characterization as novel immunoadjuvants. Vaccine (2019) 37:652–63. doi: 10.1016/j.vaccine.2018.12.009
135. Clarke TB, Davis KM, Lysenko ES, Zhou AY, Yu Y, Weiser JN. Recognition of peptidoglycan from the microbiota by Nod1 enhances systemic innate immunity. Nat Med (2010) 16:228–31. doi: 10.1038/nm.2087
136. Tourneur E, Ben Mkaddem S, Chassin C, Bens M, Goujon JM, Charles N, et al. Cyclosporine A impairs nucleotide binding oligomerization domain (Nod1)-mediated innate antibacterial renal defenses in mice and human transplant recipients. PloS Pathog (2013) 9:e1003152. doi: 10.1371/journal.ppat.1003152
137. Zhang W, Xie X, Wu D, Jin X, Liu R, Hu X, et al. Doxycycline Attenuates Leptospira-Induced IL-1beta by Suppressing NLRP3 Inflammasome Priming. Front Immunol (2017) 8:857. doi: 10.3389/fimmu.2017.00857
138. Zhang W, Zhang N, Xie X, Guo J, Jin X, Xue F, et al. Toll-Like Receptor 2 Agonist Pam3CSK4 Alleviates the Pathology of Leptospirosis in Hamster. Infect Immun (2016) 84:3350–7. doi: 10.1128/IAI.00708-16
139. Wang J, Jin Z, Zhang W, Xie X, Song N, Lv T, et al. The preventable efficacy of beta-glucan against leptospirosis. PloS Negl Trop Dis (2019) 13:e0007789. doi: 10.1371/journal.pntd.0007789
140. Matsui M, Rouleau V, Bruyere-Ostells L, Goarant C. Gene expression profiles of immune mediators and histopathological findings in animal models of leptospirosis: comparison between susceptible hamsters and resistant mice. Infect Immun (2011) 79:4480–92. doi: 10.1128/IAI.05727-11
141. Tirsoaga A, Novikov A, Adib-Conquy M, Werts C, Fitting C, Cavaillon JM, et al. Simple method for repurification of endotoxins for biological use. Appl Environ Microbiol (2007) 73:1803–8. doi: 10.1128/AEM.02452-06
142. Potula HH, Richer L, Werts C, Gomes-Solecki M. Pre-treatment with Lactobacillus plantarum prevents severe pathogenesis in mice infected with Leptospira interrogans and may be associated with recruitment of myeloid cells. PloS Negl Trop Dis (2017) 11:e0005870. doi: 10.1371/journal.pntd.0005870
143. Rice TA, Brenner TA, Percopo CM, Ma M, Keicher JD, Domachowske JB, et al. Signaling via pattern recognition receptors NOD2 and TLR2 contributes to immunomodulatory control of lethal pneumovirus infection. Antiviral Res (2016) 132:131–40. doi: 10.1016/j.antiviral.2016.06.002
144. Jutras I, Desjardins M. Phagocytosis: at the crossroads of innate and adaptive immunity. Annu Rev Cell Dev Biol (2005) 21:511–27. doi: 10.1146/annurev.cellbio.20.010403.102755
145. Moretti J, Blander JM. Insights into phagocytosis-coupled activation of pattern recognition receptors and inflammasomes. Curr Opin Immunol (2014) 26:100–10. doi: 10.1016/j.coi.2013.11.003
146. Travassos LH, Carneiro LA, Girardin S, Philpott DJ. Nod proteins link bacterial sensing and autophagy. Autophagy (2010) 6:409–11. doi: 10.4161/auto.6.3.11305
147. Cinco M, Banfi E, Soranzo MR. Leptospires macrophage interactions. Adv Exp Med Biol (1982) 141:167–74. doi: 10.1007/978-1-4684-8088-7_17
Keywords: leptospires, phagocytes, macrophages, neutrophils, platelets, TLR—toll-like receptor, NLR (NOD-like receptor), zoonosis
Citation: Santecchia I, Ferrer MF, Vieira ML, Gómez RM and Werts C (2020) Phagocyte Escape of Leptospira: The Role of TLRs and NLRs. Front. Immunol. 11:571816. doi: 10.3389/fimmu.2020.571816
Received: 11 June 2020; Accepted: 16 September 2020;
Published: 06 October 2020.
Edited by:
Jarlath E. Nally, United States Department of Agriculture, United StatesReviewed by:
Arup Sarkar, Trident Academy of Creative Technology, IndiaFelix Ngosa Toka, Warsaw University of Life Sciences, Poland
Copyright © 2020 Santecchia, Ferrer, Vieira, Gómez and Werts. This is an open-access article distributed under the terms of the Creative Commons Attribution License (CC BY). The use, distribution or reproduction in other forums is permitted, provided the original author(s) and the copyright owner(s) are credited and that the original publication in this journal is cited, in accordance with accepted academic practice. No use, distribution or reproduction is permitted which does not comply with these terms.
*Correspondence: Catherine Werts, Y3dlcnRzQHBhc3RldXIuZnI=