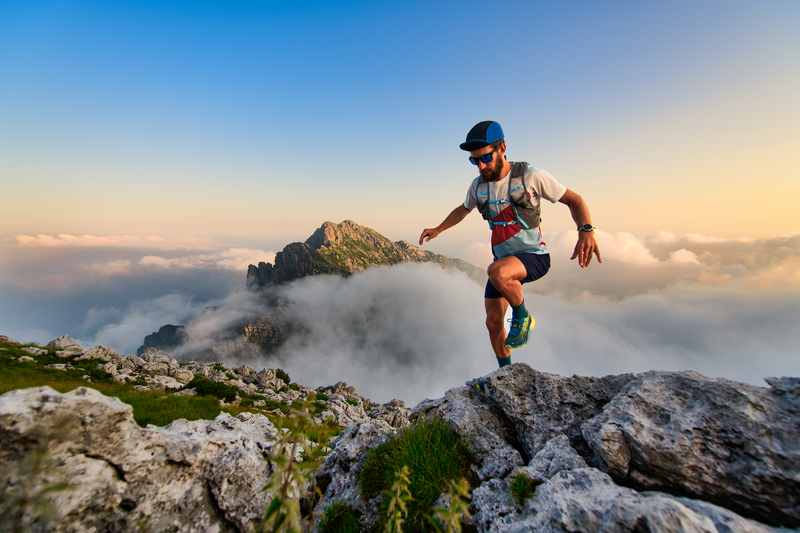
95% of researchers rate our articles as excellent or good
Learn more about the work of our research integrity team to safeguard the quality of each article we publish.
Find out more
OPINION article
Front. Immunol. , 14 July 2020
Sec. Inflammation
Volume 11 - 2020 | https://doi.org/10.3389/fimmu.2020.01762
The novel severe acute respiratory syndrome coronavirus 2 (SARS-CoV-2) pandemic infected (as of June 27, 2020) more than 10 million people and claimed 500,000 lives (1). Besides a rapidly rising death toll, this largely unknown disease is accompanied by fear and uncertainty, inflicting worldwide economic consequences, and unpopular lockdown measures. Clinical features of Coronavirus disease 2019 (COVID-19) range from mild respiratory and gastrointestinal symptoms (fever, dry cough, headaches, fatigue, chills, and diarrhea) to severe frank pneumonia and acute respiratory distress syndrome, disseminated intravascular coagulation, and death (2). Besides flu-like symptoms, COVID-19 patients exhibit less common, specific symptoms like loss of smell and taste, chilblain-like lesions on toes, mostly encountered in children (Covid toes), rashes, and skin discolorations (3). Moreover, after initial reports that children develop a low-incidence, asymptomatic infection, with extremely low mortality rate, recent reports described a Kawasaki-like disease with rashes, morbilliform exanthema, pityriasis-like discolorations, fever, reddened tongue, and enlarged coronary arteries (4).
The sudden onset of the COVID-19 pandemic motivated the medical community to decipher the pathophysiological mechanisms underlying severe outcomes rapidly. Developing safe, efficient therapeutic, and preventive strategies against SARS-CoV-2 became a global biomedical priority.
Cellular entry of SARS-CoV-2 is based on high-affinity binding of the spike protein to a cellular receptor named ACE2 (5). Angiotensin-converting enzyme 2 (ACE2) is found on epithelial cells in lungs, arteries, heart, kidney, and intestines. ACE2 is a key player in the Renin Angiotensin System (RAS), which lowers blood pressure by hydrolyzation of vasopressor angiotensin II into vasodilator angiotensin (1–7). A possible physiopathological explanation of the deleterious effects of SARS-CoV-2 is reduced ACE2 function after viral infection, low activation due to massive viral binding and internalization of the receptors, and then cellular pyroptosis. Dysregulation of RAS pathways promotes inflammation and abnormally increases blood pressure and vascular airway permeability (6, 7). Evading cellular defenses, the virus enters a phase of rapid, polymerase-based replication followed by apoptotic cell death, which releases damage-associated molecular patterns like nucleic acids, and ASC protein oligomers (8). In turn, these pyroptotic by-products activate pro-inflammatory alveolar macrophages, triggering the release of a vast array of cytokines and chemokines like Interleukin (IL) 6, IL-7, IL-2, IL-1-β, Tumor necrotic factor (TNF) α, Macrophage Inflammatory Protein (MIP) 1α and MIP-1β, Monocyte Chemoattractant Protein (MCP) 1, and Toll-Like Receptor (TLR) 4 activator protein High Mobility Group Box (HMGB)1 (8, 9). These soluble factors serve as chemoattractants for monocytes, macrophages, and T cells, triggering a self-sustaining, unabated, inflammatory feedback loop. The subsequent hypercytokinemia, followed by vascular epithelium permeabilization, leads to extracellular red blood cell leakage, thromboembolisms, and multiple organ failure. The abnormal elevation of cytokine levels, termed “cytokine storm,” sustains the observation that COVID-19 is an immune-based disease. Development of a widely available vaccine, safe for vulnerable categories (immuno-deficient patients, cancer patients, pregnant, and nursing women, etc.) paralleled with immuno-regulatory therapeutic regimens is the two-pronged approach used to harness the pandemic (9–11).
Early observations revealed that population groups are differentially affected by COVID-19. While the young showed very low prevalence and mortality, the elderly developed severe disease. Also, the death rate is higher among men (2.8%) than in women (1.7%) (12). While age-related effects were hypothetically explained by immunological features, sex-related disparity was speculatively linked to genetic reasons. The ACE2 gene location on the X chromosome may lead to allelic variants with higher protection of women against COVID-19 (13). Disease progression to Europe showed staggering disparities in the number of deaths per million (Mil) between Western and Eastern countries. While in Italy, France, UK, Spain, Ireland, Belgium, Netherlands, and Sweden, the death rate ranges 350–850/1 Mil (June 27, 2020) in Romania, Slovenia, Serbia, Croatia, Bulgaria, Albania, and Greece the rate is up to 10 fold lower: 22–83/1 Mil (14). The lower death rates (75–150/1 Mil) in Germany, Portugal, and Austria were attributed to early lockdown (Portugal) and to heavy testing in the initial stages and better social distancing compliance and intensive care capacities (Germany and Austria). While a few theories emerged, such as early imposition of quarantine and social distancing measures in Eastern countries, higher life expectancy leading to a greater number of elders and multigenerational living arrangements, discontinuation of BCG vaccine, higher air pollution levels, lower antigenic contact during life, and different social dynamics and population density in Western countries, it is hard to explain a tenfold or higher mortality rate in countries with better-prepared health care systems (15).
A recent study hypothesized that variability in the D/I genotype of the angiotensin-converting 1 (ACE1) enzyme distribution might partly elucidate the variable prevalence of COVID-19 infection amongst continental European countries. The enzyme is characterized by a genetic deletion/insertion (D/I) polymorphism in intron 16 that affects circulating and tissue concentrations of ACE2. High-affinity binding of SARS-CoV-2 to ACE-2 receptors works as a cellular doorway, facilitating viral entry and, subsequently, a higher viral load in the target tissues. According to this study, the D allele, which has higher expression in countries with lower mortality rate, is associated with reduced expression of ACE2, leading to decreased viral entry and less intense disease (16). Also, a high fat, high sugar, and low fiber diet, vitamin D intake, and geographical intestinal microbiota variations were cited as possible protective factors (17, 18). Recent data from the USA and UK underlined a disproportionate effect of COVID-19 on ethnic minorities. The UK's Intensive Care Research Centre reported (April 30) that of 6,574 patients with COVID-19 in intensive care, 1/3 were from non-white backgrounds. In the UK, ethnic minorities account for only 13% of the population. In the US, a similar study published on June 10 confirms the race disparity of COVID-19 victims, with African Americans having a 2, 5-fold higher death risk than Caucasians. Higher incidence of heart disease, diabetes, and asthma, correlated with social circumstances such as lack of medical insurance, poor diet, and higher virus exposure in the line of work, are cited as possible underlying causes of this dire reality.
Besides age, sex, and ethnicity, the presence of comorbidities was a key predictive factor for the outcome of severe COVID-19 cases. A meta-analysis conducted on 1,576 Chinese patients indicated the most prevalent comorbidities of COVID-19 patients: hypertension (21.1%, 95% CI: 13.0–27.2%), diabetes (9.7%, 95% CI: 7.2–12.2%), cardiovascular disease (8.4%, 95% CI: 3.8–13.8%), and respiratory disease (1.5%, 95% CI: 0.9–2.1%). A comparison between severe and non-severe patients resulted in pooled odds ratio (OR) of 2.36 (95% CI: 1.46–3.83), 2.46 (95% CI: 1.76–3.44), and 3.42 (95% CI: 1.88–6.22), respectively, for hypertension, respiratory system disease, and cardiovascular disease (19). Another US study done on 5,350 hospitalized patients between March 1, 2020, and April 4, 2020, in New York City area reported hypertension (56.6 %), obesity (41.7%), and diabetes (33.8%) as main comorbidities. Overall, the median age of patients was 63 years old, and 60.3% were male (20).
Low-grade inflammation (LGI) is the common feature that encompasses all the high-risk categories for developing severe COVID-19. LGI is characterized by a chronic increase of inflammatory cytokines like IL-6, TNF-α, and IL-1-β. While hyperglycemia, obesity, and hypertension (features of metabolic syndrome, MetS, increasing cardiovascular disease/T2DM risk) are accompanied by LGI, elderly persons develop 3, and inflamm-aging (21–23). Infiltrating pro-inflammatory macrophages in SARS-CoV-2 target tissues (lungs, brain, gut, kidney), and lymphocytes also contribute to the hypercytokinemia that accompanies LGI in MetS patients (22). A recent study revealed that the risk of respiratory failure for patients with IL-6 levels of ≥ 80 pg/ml was 22 times higher than for patients with lower IL-6 levels (24). In high-risk patients with chronic LGI, SARS-CoV-2 infection will elicit a cellular immune response mediated mainly by Th-1, Th-17, and proinflammatory macrophages (10, 11, 25). Even a “normal” pro-inflammatory response may increase cytokine levels further above those of the underlying LGI, hence substantially increasing the risk of developing life-threatening forms of COVID-19. We think that the “cytokine storm” term extensively used to refer to COVID-19-induced hypercytokinemia may be better suited for conditions like toxic shock or sepsis. In the case of SARS-Cov-2 infection, a “normal” anti-viral immune response combined with LGI may trigger deleterious effects. However, severe effects of COVID-19 in children and healthy adults without any apparent underlying, LGI-inducing/high-risk conditions still remain unexplained. In addition, scientists are studying the genome of individuals who never became infected with SARS-CoV-2 despite extended exposure. Genome sequencing studies are currently underway in 30 genome sequencing hubs around the world.
Beside drug-based approaches, mitigation of disease severity in high-risk categories must include prevention as a first line of defense against COVID-19. Reducing the intensity of LGI in high-risk categories is a realistic goal in diabetics. In 2019, the International Diabetes Federation warned that half of diabetics are still undiagnosed (26). A better effort to diagnose diabetes and hypertension can increase awareness about a higher risk of developing severe COVID-19.
A prospective cohort study, done on 40 newly diagnosed diabetics (aged 45–65) starting prescribed insulin therapy, compared TNF-α and IL-6 levels pre-and post-insulin treatment for 24 and 48 weeks. Volunteers were split into equal cohorts: obese diabetic and non-obese diabetic. Also, 10 age- and sex-matched healthy volunteers were used as a control group. Healthy controls had low levels of TNF-α (4.46 pg/ml) and IL-6 (4.98 pg/ml). Pre-insulin samples of age-matched controls showed an ~20-fold (87.8 pg/ml) increase in TNF-α levels and ~7-fold (34.9 pg/ml) augmentation in IL-6 levels of pre-insulin non-obese diabetics compared to healthy controls (Table 1). The effect of insulin treatment in the same groups was assessed after 24 and 48 weeks of insulin administration. A significant decrease (~1.28 fold in obese and ~1.44 fold in non-obese) was recorded in TNF-α and IL-6 levels after 24 weeks, while after 48 weeks, the downregulation was ~3.63-fold in non-obese and 2.82-fold (Table 1) in obese patients (27). Starting insulin therapy in undiagnosed diabetics can markedly reduce LGI intensity. A retrospective, multi-centered study done in 7,337 COVID-19 cases, among which 952 had pre-existing T2DM, showed that the well-controlled blood glucose (BG) levels group (<10 mmol/L) had a significantly lower in-hospital death rate (1.1 vs. 11.0%) relative to the poorly controlled BG group (28).
Table 1. IL-6 and TNF-α levels in obese and non-obese diabetics before and after insulin therapy for 24 and 48 weeks (27).
The Firmicutes to Bacteroidetes (F/B) ratio is recognized as a key indicator of dysbiotic states. The F/B ratio changes dramatically in favor of Bacteroidetes in obese individuals. Larsen et al. demonstrated that in T2DM patients, Firmicutes were significantly higher than Bacteroidetes, while in healthy subjects, the number of Bacteroidetes remained slightly increased or unchanged. The F/B ratio was significantly and positively correlated with reduced glucose tolerance (29–31). Probiotic and synbiotic supplementation had beneficent effects in T2DM by improving glycated hemoglobin (HbA1c), fasting insulin levels, Homeostasis model of assessment-insulin resistance (HOMA-IR), and the quantitative insulin sensitivity check index (QUICKI) (32, 33). Probiotics play immunomodulatory roles by downregulating LGI levels, skewing pro-inflammatory macrophages toward a pro-restorative phenotype, increasing the Treg/Th17 ratio, and promoting interferon (IFN) γ-producing Th-1 lymphocytes [reviewed in (33, 34)].
A meta-analysis done on 49 articles pooled the known effects of probiotics, resveratrol, Vitamin D, and other molecules on elderly patients with LGI. The study showed that IL-6 levels were downregulated by probiotics (−0.68 pg/ml), Angiotensin Receptor Blockers (−0.37 pg/ml), and omega-3 fatty acids (−0.19 pg/ml) while resveratrol and vitamin D treatments had no significant effects (35).
Besides insulin therapy and probiotic supplementation, other interventional measures like whole-body vibration, increased exercise, and nutritional changes, which aimed to decrease insulin resistance (IR), improve BG control, and restore intestinal eubiosis, were shown to significantly downregulate LGI by decreasing IL-6, TNF-α, and IL-1-β levels (36–40). Although there is no direct evidence certifying a protective effect of supplements like Vitamin C, Vitamin D, Zinc, probiotics, and synbiotics on COVID-19 outcomes, we are hypothesizing that preventive measures targeting LGI can lower the propensity to develop severe disease (41–44). Unlike drug-based therapies, the side effects of supplements are extremely mild (diarrhea, bloating, headaches, antibiotic resistance); however, further studies are necessary to confirm safe usage. These non-drugs based, preventive measures, aiming to downregulate LGI in high-risk patients, must be correlated with risk-minimizing behavior like mask-wearing, social distancing, hand washing, and cough covering. In SARS-CoV2 infection, these supplements may be used only as adjuvant measures recommended by doctors and under no circumstances can replace prescribed drug regimens. A better understanding of the pathophysiology of COVID-19 will enable biomedical scientists to find efficient preventive and therapeutic strategies.
The author confirms being the sole contributor of this work and has approved it for publication.
The author declares that the research was conducted in the absence of any commercial or financial relationships that could be construed as a potential conflict of interest.
1. Zhu N, Zhang D, Wang WX, Li B, Yang J, Song, et al. A novel coronavirus from patients with pneumonia in China, 2019. N Engl J Med. (2020) 382:727–33. doi: 10.1056/NEJMoa2001017
2. Pascarella G, Strumia A, Piliego C, Bruno F, Del Buono R, et al. COVID-19 diagnosis and management: a comprehensive review. J Intern Med. (2020). doi: 10.1111/joim.13091. [Epub ahead of print].
3. CDC. Symptoms of coronavirus (2020). Available online at: https://www.cdc.gov/coronavirus/2019-ncov/symptoms-testing/symptoms.html (accessed July 5, 2020).
4. CNN (2018). Available online at: https://edition.cnn.com/2020/05/07/health/new-york-children-coronavirus-kawasaki/index.html (accessed June 27, 2020).
5. Yuki K, Fujiogi M, Koutsogiannaki S. COVID-19 pathophysiology: a review. Clin Immunol. (2020) 215:108427. doi: 10.1016/j.clim.2020.108427
6. Busse LW, Chow JH, McCurdy MT, Khanna AK. COVID-19 and the RAAS-a potential role for angiotensin II? Crit Care. (2020) 24:136. doi: 10.1186/s13054-020-02862-1
7. Cheng ZJ, Vapaatalo H, Mervaala E. Angiotensin II and vascular inflammation. Med Sci Monit. (2005) 11:RA194–205.
8. Tay MZ, Poh CM, Rénia L, Mac Ari PA, Ng LFP. The trinity of COVID-19: immunity inflammation and intervention. Nat Rev Immunol. (2020) 20:363–74. doi: 10.1038/s41577-020-0311-8
9. Jose RJ, Manuel A. COVID-19 cytokine storm: the interplay between inflammation and coagulation. Lancet. (2020) 8:e46–7. doi: 10.1016/S2213-2600(20)30216-2
10. Ye Q, Wang B, Mao J. The pathogenesis and treatment of the ‘Cytokine Storm' in COVID-19 (preprint). J Infect. (2020) 80:607–13. doi: 10.1016/j.jinf.2020.03.037
11. Lipworth B, Chan R, Lipworth S, RuiWen Kuo C. Weathering the cytokine storm in susceptible patients with severe SARS-CoV-2 infection (preprint). J Allergy Clin Immunol Pract. (2020) 8:1798–801. doi: 10.1016/j.jaip.2020.04.014
12. Zhonghua L, Xue XB, Zhi Z. Novel coronavirus pneumonia emergency response epidemiology team. Epidemiol Team. (2020) 41:145–51. doi: 10.3760/cma.j.issn.0254-6450.2020.02.003
13. Conti P, Younes A. Coronavirus COV-19/SARS-CoV-2 affects women less than men: clinical response to viral infection. J Biol Regul Homeost Agents. (2020). doi: 10.23812/Editorial-Conti-3. [Epub ahead of print].
14. Worldometer (2020). Available online at: https://www.worldometers.info/coronavirus (accessed July 5, 2020).
15. The Guardian (2020). Available online at: https://www.theguardian.com/world/2020/may/05/why-has-eastern-europe-suffered-less-from-coronavirus-than-the-west (accessed July 5, 2020).
16. Delanghe JR, Speeckaert MM, De Buyzere ML. The host's angiotensin-converting enzyme polymorphism may explain epidemiological findings in COVID-19 infections. Clin Chim Acta. (2020) 505:192–3. doi: 10.1016/j.cca.2020.03.031
17. Khunti, K, Singh A K, Pareek M, Hanif W. Is ethnicity linked to incidence or outcomes of covid-19? BMJ. (2020) 369:m1548. doi: 10.1136/bmj.m1548
18. Baud D, Dimopoulou Agri V, Gibson GR, Reid G, Giannoni E. Using probiotics to flatten the curve of Coronavirus Disease COVID-2019 pandemic. Front Public Health. (2020) 8:186. doi: 10.3389/fpubh.2020.00186
19. Yang J, Zheng Y, Gou X, Pu k, Chen Z, Guo Q, et al. Prevalence of comorbidities and its effects in coronavirus disease 2019 patients: a systematic review and meta-analysis (preprint). Int J Infect Dis. (2020) 94:91–5. doi: 10.1016/j.ijid.2020.03.017
20. Richardson S, Hirsch JS, Narasimhan M. Presenting characteristics, comorbidities, and outcomes among 5700 patients hospitalized with COVID-19 in the New York City area. JAMA. (2020) 22:2020. doi: 10.1001/jama.2020.6775
21. Fang L, Karakiulakis G, Roth M. Are patients with hypertension and diabetes mellitus at increased risk for COVID-19 infection? Lancet Respir Med. (2020) 8:e21. doi: 10.1016/S2213-2600(20)30116-8
22. Pereira SS, Alvarez-Leite JI. Low-grade inflammation, obesity, and diabetes. Curr Obes Rep. (2014) 3:422–31. doi: 10.1007/s13679-014-0124-9
23. Ferrucci L, Fabbri E. Inflammageing: chronic inflammation in ageing, cardiovascular disease, and frailty. Nat Rev Cardiol. (2018) 15:505–22. doi: 10.1038/s41569-018-0064-2
24. Herold T, Jurinovic V, Arnreich CJC, Hellmuth von Bergwelt-Baildon M, Klein M, et al. Elevated levels of IL-6 predicts respiratory failure in hospitalized symptomatic COVID-19 patients. J Allergy Clin Immunol. (2020) 146:128–36. doi: 10.1016/j.jaci.2020.05.008
25. Wu D, Yang XO. TH17 responses in cytokine storm of COVID-19: an emerging target of JAK2 inhibitor Fedratinib (preprint). J Microbiol Immunol Infect. (2020) 53:368–70. doi: 10.1016/j.jmii.2020.03.005
26. IDF Diabetes Atlas (2020). Available online at: https://www.diabetesatlas.org/en/ (accessed June 27, 2020).
27. Goyal R, Faizy AF, Siddiqui SS, Singhai M. Evaluation of TNF-α and IL-6 Levels in Obese and Non-obese Diabetics: Pre- and Postinsulin Effects. N Am J Med Sci. (2012) 4:180–4. doi: 10.4103/1947-2714.94944
28. Lihua Z, Zhi-Gang S, Xu C, Juan-Juan Q, Xiao-Jing Z, Cai J. et al. Association of blood glucose control and outcomes in patients with COVID-19 and Pre-existing type 2 diabetes. Cell Metab. (2020) 31:1068–77.e3. doi: 10.1016/j.cmet.2020.04.021
29. Crommen S, Simon MC. Microbial regulation of glucose metabolism and insulin resistance. Genes (Basel). (2017) 9:10. doi: 10.3390/genes9010010
30. Larsen N, Vogensen FK, van den Berg FW, Nielsen DS, Andreasen AS, Pedersen BK, et al. Gut microbiota in human adults with type 2 diabetes differs from non-diabetic adults. PLoS ONE. (2010) 5:e9085. doi: 10.1371/journal.pone.0009085
31. Qin J, Li Y, Cai Z, Zhu J, Zhang F., Liang, S., et al. A metagenome-wide association study of gut microbiota in type 2 diabetes. Nature. (2012) 490:55–60. doi: 10.1038/nature11450
32. Kesika P, Sivamaruthi BS, Chaiyasut C. Do probiotics improve the health status of individuals with diabetes mellitus? A review on outcomes of clinical trials. Biomed Res Int. (2019) 2019:1531567. doi: 10.1155/2019/1531567
33. Plaza-Díaz J, Ruiz-Ojeda FJ, Vilchez-Padial LM, Gil A. Evidence of the anti-inflammatory effects of probiotics and synbiotics in intestinal chronic diseases. Nutrients. (2017) 9:555. doi: 10.3390/nu9060555
34. Azad MA, Sarker M, Wan D. Immunomodulatory effects of probiotics on cytokine profiles. BioMed Res Int. (2018) 2018:10. doi: 10.1155/2018/8063647
35. Custodero C, Mankowski RT, Lee SA, Chen Z, Wu S, Manini TM, et al. Evidence-based nutritional and pharmacological interventions targeting chronic low-grade inflammation in middle-age and older adults: a systematic review and meta-analysis. Ageing Res Rev. (2018) 46:42–59. doi: 10.1016/j.arr.2018.05.004
36. Yin H, Berdel HO, Moore D, Davis F, Liu J, Mozaffari M, et al. Whole body vibration therapy: a novel potential treatment for type 2 diabetes mellitus. SpringerPlus. (2015) 4:578. doi: 10.1186/s40064-015-1373-0
37. Karstoft K, Pedersen BK. Exercise and type 2 diabetes: focus on metabolism and inflammation. Immunol Cell Biol. (2016) 94:146–50. doi: 10.1038/icb.2015.101
38. Casas R, Sacanella E, Estruch R. The immune protective effect of the Mediterranean diet against chronic low-grade inflammatory diseases. Endocr Metab Immune Disord Drug Targets. (2014) 14:245–54. doi: 10.2174/1871530314666140922153350
39. Minihane AM, Vinoy S, Russell WR, Baka A, Roche HM, Touhy CM, et al. Low-grade inflammation, diet composition and health: current research evidence and its translation. Br J Nutr. (2015) 114:999–1012. doi: 10.1017/S0007114515002093
40. Blanks AM, Rodriguez-Miguelez P, Looney J, Tucker MA, Jeong J, Thomas J, et al. Whole body vibration elicits differential immune and metabolic responses in obese and normal weight individuals. Brain Behav Immunity-Health. (2020) 1:100011. doi: 10.1016/j.bbih.2019.100011
41. Ilie PC, Stefanescu S, Smith L. The role of Vitamin D in the prevention of Coronavirus Disease 2019 infection and mortality. Aging Clin Exp Res. (2020) 32:1195–8. doi: 10.1007/s40520-020-01570-8
42. Malaguarnera L. Vitamin D and microbiota: Two sides of the same coin in the immunomodulatory aspects. Int Immunopharmacol. (2020) 79:106112. doi: 10.1016/j.intimp.2019.106112
43. Read SA, Obeid S, Ahlenstiel C, Ahlenstiel G. The role of zinc in antiviral immunity. Adv Nutr. (2019) 10:696–710. doi: 10.1093/advances/nmz013
Keywords: COVID-19, SARS-CoV-2, Coronavirus, probiotics, low-grade inflammation, type 2 diabetes, obesity, cytokine storm
Citation: Ciornei RT (2020) Prevention of Severe Coronavirus Disease 2019 Outcomes by Reducing Low-Grade Inflammation in High-Risk Categories. Front. Immunol. 11:1762. doi: 10.3389/fimmu.2020.01762
Received: 11 May 2020; Accepted: 30 June 2020;
Published: 14 July 2020.
Edited by:
Stefano Caserta, University of Hull, United KingdomReviewed by:
Yongwen Chen, Third Military Medical University, ChinaCopyright © 2020 Ciornei. This is an open-access article distributed under the terms of the Creative Commons Attribution License (CC BY). The use, distribution or reproduction in other forums is permitted, provided the original author(s) and the copyright owner(s) are credited and that the original publication in this journal is cited, in accordance with accepted academic practice. No use, distribution or reproduction is permitted which does not comply with these terms.
*Correspondence: Radu Tudor Ciornei, cmFkdXR1ZG9yY2lvcm5laUBnbWFpbC5jb20=
Disclaimer: All claims expressed in this article are solely those of the authors and do not necessarily represent those of their affiliated organizations, or those of the publisher, the editors and the reviewers. Any product that may be evaluated in this article or claim that may be made by its manufacturer is not guaranteed or endorsed by the publisher.
Research integrity at Frontiers
Learn more about the work of our research integrity team to safeguard the quality of each article we publish.