- Laboratory of Host–Microbe Interactions and Cell Dynamics, Institute of Microbiology and Immunology, National Yang-Ming University, Taipei, Taiwan
Listeria monocytogenes is a Gram-positive foodborne bacterial pathogen capable of interacting and crossing the intestinal barrier, blood–brain barrier, and placental barrier to cause deadly infection with high mortality. L. monocytogenes is an intracellular pathogen characterized by its ability to enter non-phagocytic cells. Expression of the cytolysin listeriolysin O has been shown to be the main virulence determinant in vitro and in vivo in mouse models. L. monocytogenes can also perform cell-to-cell spreading using actin-rich membrane protrusions to infect neighboring cells, which also constitutes an important strategy for infection. These events including entry into host cells, interaction between listeriolysin O and host plasma membrane, and bacterial cell-to-cell spreading have been demonstrated to implicate the cholesterol-rich lipid rafts or molecules in these microdomains in the host plasma membrane in vitro with tissue culture models. Here we review the contribution of lipid rafts on plasma membrane to L. monocytogenes infection.
Introduction
Human listeriosis is a foodborne disease caused by the intracellular pathogen Listeria monocytogenes. Upon ingestion of contaminated food by the host, L. monocytogenes interacts and traverses the intestinal epithelium to reach the lamina propria, followed by dissemination into lymph and bloodstream toward the liver and spleen, where the bacteria replicate. L. monocytogenes can further cross the blood–brain barrier to induce meningoencephalitis and invade the placenta and result in fetal infection, stillbirth, abortion, and neonatal infection (1, 2). L. monocytogenes has been used as a model microorganism in studying host–microbe interactions since the late 1980s. Efforts have been made to unveil how this facultative intracellular pathogen enters into cultured non-phagocytic epithelial cells, escapes from the internalization vacuole, and spreads from the infected cell to another (3). Interaction of L. monocytogenes surface protein InlA and InlB with corresponding host receptors human E-cadherin (hEcad) and human c-Met at the plasma membrane leads to host actin polymerization and septin assembly, followed by internalization of bacteria in a vacuole (4–7). The L. monocytogenes-containing vacuole, especially in phagocytic cells, is subsequently lysed by the bacterial pore-forming toxin listeriolysin O (LLO), resulting in bacterial escape from the vacuole (8). Within the host cell cytosol, the bacterial surface protein ActA contributes to L. monocytogenes intracellular motility via host actin polymerization and actin comet tail formation, followed by the induction of membrane protrusions and infection of neighboring cells (9). In the neighboring cells, L. monocytogenes will then be located in a double-membrane vacuole, in which bacterial phospholipases PlcA and PlcB, together with LLO, are required for vacuole lysis and bacterial escape into cytosol for infection propagation (10). The membrane damaging property of LLO is also involved in multiple modulation activities during infection, such as Arp2/3-dependent F-actin remodeling that promotes bacterial internalization, changes in histone modification and thus modulation of host gene expression, desumoylation of host proteins, induction of mitochondrial fission, increase of endoplasmic reticulum (ER) stress, and lysosomal permeabilization (11–19).
Plasma membranes have been described as a fluid mosaic interface containing various lipid species in two asymmetric leaflets with plenty of floating proteins (20, 21). The observations that cell membranes can be separated into detergent-sensitive and detergent-resistant fractions suggested the presence of distinct membrane sub-compartments in cell membranes. The clusters of lipids in a more ordered state with relatively saturated lipids and glycosylated lipids are referred to as lipid rafts, as compared to the disordered membrane domains with unsaturated lipids (20). Lipid rafts are cholesterol- and sphingolipid-enriched microdomains with ordered assemblies of proteins and lipids in cell membranes (22). These rafts are heterogeneous and dynamic, and have the potential to form large domains (>300 nm) upon clustering induced by protein–protein and protein–lipid interactions (20). The organization of lipid rafts can also be regulated and mobilized by cortical actin filaments via specific interactions between actin and membrane adaptor proteins (23, 24). Lipid rafts have been suggested to be implicated in membrane protein signaling, membrane trafficking, and host–microbe interactions (25). Compartmentalization of cellular signaling in membrane domains is important to regulate maturation of immune cells. It was demonstrated that T cell receptors and B cell receptors were found in detergent-sensitive membrane in resting stage, but shifted to detergent-resistant fractions upon receptor activation (26–29). This suggests that the translocation of receptors involved in antigen presentation to lipid rafts is associated with active signaling in these immune cells. Lipid rafts are also enriched in caveolae, which are flask-shaped pits with a size of 50–80 nm in the cell membrane. Caveolae are associated with expression of caveolin, which is responsible for stabilization of caveolar structure and the internalization of extracellular materials into caveolae (25). While host plasma membranes are the first barrier for the invasion of intracellular pathogens, the observation that host receptors are clustered in the lipid rafts and enrichment of cholesterol on microbe-containing vacuoles highlights the importance of lipid rafts in pathogen–host interactions (30). Here we review and discuss the implication of lipid rafts in the interaction between L. monocytogenes and host cells at different interfaces (Figure 1).
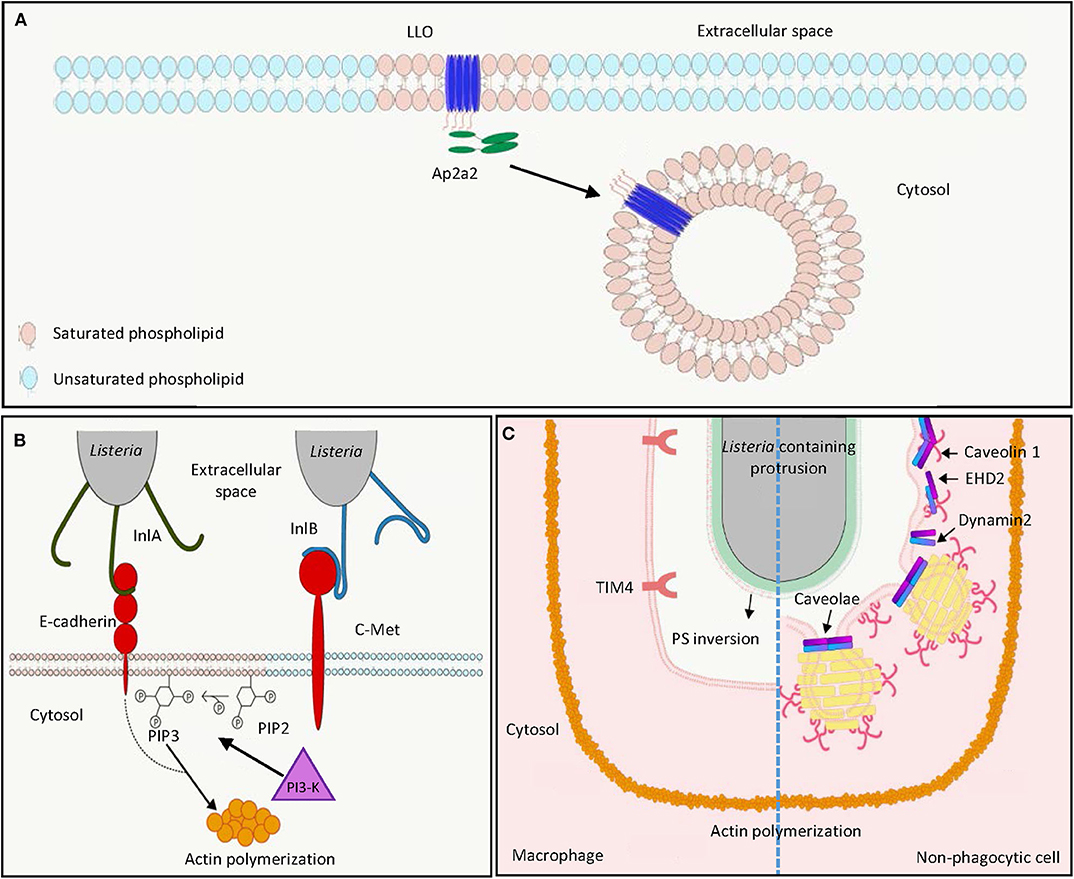
Figure 1. L. monocytogenes harnesses host lipid rafts for infection. (A) Extracellular L. monocytogenes secretes the cytolysin LLO, which binds cholesterol and inserts into host cell membrane in lipid raft domains. The interaction between the endocytosis adaptor protein Ap2a2 and inserted LLO results in endocytosis of LLO to prevent LLO-induced plasma membrane damage. (B) During bacterial entry, the L. monocytogenes surface protein InlA acts as an adhesin binding to host E-cadherin in lipid raft domains. Binding of L. monocytogenes InlB to host c-Met triggers PI3-K activation, which catalyzes the production of PIP3 from PIP2 in lipid raft domains, leading to actin polymerization and internalization of E-cadherin-bound bacteria. (C) In the process of cell-to-cell spreading, LLO damages the plasma membrane of the infected cells to induce PS inversion in the lipid rafts. Neighboring macrophages engulf PS-positive protrusion structures by the PS receptor TIM4. Neighboring non-phagocytic cells internalize L. monocytogenes membrane protrusions by caveolin-dependent endocytosis in lipid rafts. LLO, listeriolysin O; PIP2, phosphatidylinositol-4,5-bisphosphate; PIP3, phosphatidylinositol-3,4,5-triphosphate; PI3-K, phosphoinositide 3-kinase; PS, phosphatidylserine.
LLO, a Multifunctional Cytolysin Targeting Lipid Rafts
LLO is crucial for full virulence of L. monocytogenes in both in vitro tissue culture systems and in vivo animal models (31–35). LLO is a secreted protein of 56 kDa molecular weight belonging to the family of cholesterol-dependent cytolysins (CDCs), which represent the largest family of pore-forming toxins that form large pores (up to 35 nm) produced by different bacterial species (36–40). Preincubation of LLO with cholesterol abolished cytolytic activity, suggesting the importance of cholesterol binding in lipid rafts for cytolysis (41). Differing from other CDC members, LLO exhibits optimal binding to cholesterol-containing membranes at pH 5.5, and this binding decreases at neutral and basic pH. Nevertheless, high cholesterol levels, corresponding to the concentration range of cholesterol found in lipid rafts, can restore LLO binding to membranes at suboptimal pH (42). This is explained by the presence of an acidic triad in the transmembrane domain, which functions as a pH sensor and triggers premature denaturation of LLO at neutral pH at 37°C, thereby allowing pore formation to occur mainly at acidic pH (43–45). The pH dependence of LLO limits cytolytic activity to acidic vesicles and prevents damage in the host cytosol, which is a niche for L. monocytogenes replication. Accordingly, replacement of LLO by pH-insensitive CDCs such as perfringolysin O from Clostridium perfringens allowed phagosomal escape of L. monocytogenes, but led to decreased infection efficiency in vitro in a plaque assay (46). Cholesterol in the lipid rafts not only provides an initial binding site for LLO, in vitro studies with high-speed atomic force microscopy (HS-AFM) further demonstrated that in acidic environments LLO can produce arc pores in the membrane as a lineactant, and therefore creates large-scale defects for bacterial escape from phagocytic vacuole (47).
In addition to targeting of cholesterol-rich domains to damage the host cell membrane, the PEST-like sequence at the N-terminus of LLO interacts with the endocytosis adaptor Ap2a2, a lipid-raft associated protein (Figure 1A) (48, 49). This interaction facilitates clathrin-dependent endocytosis of plasma membrane-associated LLO and removes these pore-forming toxins from the plasma membrane, thereby preventing its cytotoxicity to the infected cell and enhancing L. monocytogenes virulence during infection in vivo (48, 50). However, this clathrin-dependent endocytosis of LLO-associated membrane may not contribute to membrane repair after pore formation as endocytic proteins are not recruited to the membrane damage sites (51). Instead, LLO-induced membrane damage can result in influx of intracellular calcium, which subsequently activates TMEM16F lipid scramblase, leading to membrane blebbing and extracellular vesicle release to repair plasma membrane damage (52). Although less characterized, LLO was demonstrated to induce clustering of GPI-anchored proteins CD14 and CD24 on the surface of murine macrophages J774, while the non-lipid-raft marker transferrin receptor was not affected (53).
L. monocytogenes Harnesses Lipid Rafts Proteins for Entry Into Host Cells
L. monocytogenes uses its surface internalin proteins InlA and InlB to bind hEcad and human c-Met, respectively. These interactions result in cytoskeleton rearrangement, thereby bacterial entry into non-phagocytic cells through a zipper mechanism (Figure 1B) (4, 7, 54). Treatment of phosphoinositide 3-kinase (PI3-K) inhibitors wortmannin and LY294002, respectively, abolished InlA- and InlB-dependent invasion into different host cells, showing the importance of PI3-K activity in internalin-mediated entry (Figure 1B) (55, 56). Depletion of cholesterol by methyl-β-cyclodextrin reduced InlA- and InlB-dependent L. monocytogenes internalization into non-phagocytic cells, indicating the involvement of lipid rafts in internalin-mediated internalization (57). This is further supported by the observation that multiple lipid raft markers, such as glycosylphosphatidylinositol-linked proteins, a myristoylated and palmitoylated peptide, and the ganglioside GM1 were recruited at the bacterial entry site (57). While InlA–Ecad interaction and Ecad recruitment at the entry site were cholesterol-dependent, cholesterol depletion did not affect InlB interaction with c-Met, the recruitment of c-Met at the entry site, and c-Met downstream signaling (57). Nevertheless, cholesterol depletion abrogates InlB-mediated actin polymerization (57). The implication of lipid rafts in InlA-mediated entry was further demonstrated by the observation that caveolin was recruited to the bacterial entry sites and was required for the internalization in an InlA-dependent manner (58). While InlB is dispensable for bacterial entry into LS174T intestinal epithelial cells that show constitutively activated PI3-K activity, this protein is necessary for InlA-dependent entry into cells that do not exhibit constitutive PI3-K activity (55). Together, while InlB-induced c-Met phosphorylation does not depend on cholesterol and lipid rafts, subsequent PI3-K activation and Rac1-induced actin polymerization at the bacterial entry site occur within lipid rafts and require their integrity (57, 59). InlA acts as an adhesion molecule to Ecad in the detergent-resistant lipid rafts, thereby triggering internalization of L. monocytogenes dependent on PI3-K activation and caveolin-mediated endocytosis (Figure 1B) (57–60).
The Role of Lipid Rafts in L. monocytogenes Cell-to-Cell Spreading
Following lysis of the phagocytic vacuole in host cells, the L. monocytogenes surface protein ActA can polymerize actin in cytosol, which allows formation of bacteria-containing membrane protrusions, the structures that are later internalized by neighboring cells to result in cell-to-cell spreading of L. monocytogenes (Figure 1C) (61). Internalization of L. monocytogenes membrane protrusions was demonstrated to be exploited by efferocytosis, by which the apoptotic cells are removed by macrophages (Figure 1C, left) (62). In both phagocytic and non-phagocytic cells, ActA-mediated actin-based motility was proposed to allow close apposition of bacteria to the cell membrane, where secreted LLO may damage cell membrane and induce externalization of phosphatidylserine (PS), a hallmark of apoptotic cells at cell membrane (62). The PS-positive protrusion structures containing L. monocytogenes are subsequently recognized by the PS-binding receptor TIM-4 on macrophages to facilitate phagocytic uptake and cell–cell spreading (62). While methyl-β-cyclodextrin-mediated cholesterol depletion was demonstrated to reduce PS externalization and phagocytosis of apoptotic cells, this suggests that lipid raft integrity could be important for efferocytosis-mediated L. monocytogenes spreading (63–65). L. monocytogenes cell-to-cell spreading is not limited to transfer to phagocytic cells (61). Dhanda et al. further investigated the membrane invagination in neighboring non-phagocytic cells (Figure 1C, right) (66). While caveolin-based and lipid raft-dependent endocytosis is supposed to be a process that internalizes extracellular material into bulb-shaped caveolae no larger than 100 nm, L. monocytogenes membrane protrusions triggered the recruitment of caveolar proteins and PS in a neighboring cell (66). Knock-down of caveolin-1 reduced invagination length in the neighboring cells and L. monocytogenes cell-to-cell spreading without detectable effect on the length of the actin comet tail and protrusion in initial infected cells (66). This suggests that caveolin can mediate engulfment of large materials, such as L. monocytogenes-containing membrane protrusions, which was not supposed to be achieved based on the size of caveolae. Collectively, due to the importance of lipid rafts in both efferocytosis and caveolae structure, efficient L. monocytogenes cell-to-cell spreading may require lipid raft integrity (63, 67). Further studies disrupting these membrane microdomains in L. monocytogenes cell-to-cell spreading are needed to directly address the role of lipid rafts in this process.
Concluding Remarks
Our understanding of lipid rafts has been improved by the development of biochemical and microscopy tools (20, 21, 25). Depletion of membrane cholesterol by methyl-β-cyclodextrin constitutes the most common approach to disrupt membrane lipid rafts to investigate their biological function (68). However, methyl-β-cyclodextrin exhibits pleiotropic effects beyond lipid raft disruption such as inhibition of clathrin-mediated endocytosis (69–71). The models where lipid raft components are genetically deficient may be applied to more specifically address the function of lipid rafts (72–74). On the other hand, advance in microscopy techniques allows for visualizing the lipid rafts from model membranes to living cells in vitro (25). Recent advances suggest that pathogens may behave differently and adopt a distinct strategy to interact with the host between in vitro cell culture and in vivo animal models. For example, while L. monocytogenes interacts with enterocytes and access to the cytosol in cell culture systems, it targets goblet cells in the small intestine and performs transcytosis to cross intestinal epithelium followed by systemic infection in humanized mouse models (55, 75, 76). Conditional genetic knock-out mouse models, where lipid rafts are disrupted in villin-expressing intestinal epithelium, may provide a clue regarding the role of lipid rafts in L. monocytogenes crossing of intestinal barrier (77). Stem cell-derived organoids have been shown to recapitulate the complexity of a local tissue in vitro in culture systems (78). While L. monocytogenes was demonstrated to successfully infect intestinal organoids, coupling of super-resolution optical microscopy methods may allow visualizing the organization of lipid rafts at the interface between L. monocytogenes and host cells relevant to the in vivo environment (79, 80). Together, future studies addressing the role of lipid rafts in vivo and visualizing these nanoscale membrane domains at the interface between L. monocytogenes and host in tissues will provide insight into how lipid rafts are implicated in the pathophysiology of L. monocytogenes infection.
Author Contributions
Y-HT: conceived and designed the study, and wrote and edited the paper. W-LC: reviewed the literature and edited the paper. All authors contributed to the article and approved the submitted version.
Funding
This work was supported by a grant from the Ministry of Science and Technology, Taiwan (MOST106-2321-B-182-004-MY3) to Y-HT. The funder of the study had no role in study design, data collection, data analysis, data interpretation, or writing of the paper.
Conflict of Interest
The authors declare that the research was conducted in the absence of any commercial or financial relationships that could be construed as a potential conflict of interest.
Acknowledgments
We would like to thank all the members of the Laboratory of Host–Microbe Interactions and Cell Dynamics.
References
1. Lecuit M. Human listeriosis and animal models. Microbes Infect. (2007) 9:1216–25. doi: 10.1016/j.micinf.2007.05.009
2. Charlier C, Perrodeau E, Leclercq A, Cazenave B, Pilmis B, Henry B, et al. Clinical features and prognostic factors of listeriosis: the MONALISA national prospective cohort study. Lancet Infect Dis. (2017) 17:510–9. doi: 10.1016/S1473-3099(16)30521-7
3. Pizarro-Cerda J, Cossart P. Listeria monocytogenes membrane trafficking and lifestyle: the exception or the rule? Annu Rev Cell Dev Biol. (2009) 25:649–70. doi: 10.1146/annurev.cellbio.042308.113331
4. Mengaud J, Ohayon H, Gounon P, Mège R-M, Cossart P. E-Cadherin is the receptor for internalin, a surface protein required for entry of L. monocytogenes into Epithelial Cells. Cell. (1996) 84:923–32. doi: 10.1016/S0092-8674(00)81070-3
5. Mostowy S, Danckaert A, Tham TN, Machu C, Guadagnini S, Pizarro-Cerda J, et al. Septin 11 restricts InlB-mediated invasion by Listeria. J Biol Chem. (2009) 284:11613–21. doi: 10.1074/jbc.M900231200
6. Mostowy S, Nam Tham T, Danckaert A, Guadagnini S, Boisson-Dupuis S, Pizarro-Cerda J, et al. Septins regulate bacterial entry into host cells. PLoS ONE. (2009) 4:e4196. doi: 10.1371/journal.pone.0004196
7. Shen Y, Naujokas M, Park M, Ireton K. InIB-dependent internalization of Listeria is mediated by the Met receptor tyrosine kinase. Cell. (2000) 103:501–10. doi: 10.1016/S0092-8674(00)00141-0
8. Schnupf P, Portnoy DA. Listeriolysin O: a phagosome-specific lysin. Microbes Infect. (2007) 9:1176–87. doi: 10.1016/j.micinf.2007.05.005
9. Kocks C, Gouin E, Tabouret M, Berche P, Ohayon H, Cossart P. L. monocytogenes-induced actin assembly requires the actA gene product, a surface protein. Cell. (1992) 68:521–31. doi: 10.1016/0092-8674(92)90188-I
10. Vazquez-Boland JA, Kocks C, Dramsi S, Ohayon H, Geoffroy C, Mengaud J, et al. Nucleotide sequence of the lecithinase operon of Listeria monocytogenes and possible role of lecithinase in cell-to-cell spread. Infect Immun. (1992) 60:219–30. doi: 10.1128/IAI.60.1.219-230.1992
11. Birmingham CL, Canadien V, Kaniuk NA, Steinberg BE, Higgins DE, Brumell JH. Listeriolysin O allows Listeria monocytogenes replication in macrophage vacuoles. Nature. (2008) 451:350–4. doi: 10.1038/nature06479
12. Dramsi S, Cossart P. Listeriolysin O-mediated calcium influx potentiates entry of Listeria monocytogenes into the human Hep-2 epithelial cell line. Infect Immun. (2003) 71:3614–8. doi: 10.1128/IAI.71.6.3614-3618.2003
13. Hamon MA, Batsche E, Regnault B, Tham TN, Seveau S, Muchardt C, et al. Histone modifications induced by a family of bacterial toxins. Proc Natl Acad Sci USA. (2007) 104:13467–72. doi: 10.1073/pnas.0702729104
14. Hamon MA, Cossart P. K+ efflux is required for histone H3 dephosphorylation by Listeria monocytogenes listeriolysin O and other pore-forming toxins. Infect Immun. (2011) 79:2839–46. doi: 10.1128/IAI.01243-10
15. Lam JGT, Vadia S, Pathak-Sharma S, McLaughlin E, Zhang X, Swanson J, et al. Host cell perforation by listeriolysin O (LLO) activates a Ca(2+)-dependent cPKC/Rac1/Arp2/3 signaling pathway that promotes Listeria monocytogenes internalization independently of membrane resealing. Mol Biol Cell. (2018) 29:270–84. doi: 10.1091/mbc.E17-09-0561
16. Pillich H, Loose M, Zimmer KP, Chakraborty T. Activation of the unfolded protein response by Listeria monocytogenes. Cell Microbiol. (2012) 14:949–64. doi: 10.1111/j.1462-5822.2012.01769.x
17. Ribet D, Hamon M, Gouin E, Nahori MA, Impens F, Neyret-Kahn H, et al. Listeria monocytogenes impairs SUMOylation for efficient infection. Nature. (2010) 464:1192–5. doi: 10.1038/nature08963
18. Stavru F, Palmer AE, Wang C, Youle RJ, Cossart P. Atypical mitochondrial fission upon bacterial infection. Proc Natl Acad Sci USA. (2013) 110:16003–8. doi: 10.1073/pnas.1315784110
19. Vadia S, Arnett E, Haghighat AC, Wilson-Kubalek EM, Tweten RK, Seveau S. The pore-forming toxin listeriolysin O mediates a novel entry pathway of L. monocytogenes into human hepatocytes. PLoS Pathog. (2011) 7:e1002356. doi: 10.1371/journal.ppat.1002356
20. Sezgin E, Levental I, Mayor S, Eggeling C. The mystery of membrane organization: composition, regulation and roles of lipid rafts. Nat Rev Mol Cell Biol. (2017) 18:361–74. doi: 10.1038/nrm.2017.16
21. Levental I, Levental KR, Heberle FA. Lipid rafts: controversies resolved, mysteries remain. Trends Cell Biol. (2020) 30:341–53. doi: 10.1016/j.tcb.2020.01.009
22. Lingwood D, Simons K. Lipid rafts as a membrane-organizing principle. Science. (2010) 327:46–50. doi: 10.1126/science.1174621
23. Gowrishankar K, Ghosh S, Saha S, Rumamol C, Mayor S, Rao M. Active remodeling of cortical actin regulates spatiotemporal organization of cell surface molecules. Cell. (2012) 149:1353–67. doi: 10.1016/j.cell.2012.05.008
24. Fritzsche M, Li D, Colin-York H, Chang VT, Moeendarbary E, Felce JH, et al. Self-organizing actin patterns shape membrane architecture but not cell mechanics. Nat Commun. (2017) 8:14347. doi: 10.1038/ncomms14347
25. Simons K, Gerl MJ. Revitalizing membrane rafts: new tools and insights. Nat Rev Mol Cell Biol. (2010) 11:688–99. doi: 10.1038/nrm2977
26. Beck-Garcia K, Beck-Garcia E, Bohler S, Zorzin C, Sezgin E, Levental I, et al. Nanoclusters of the resting T cell antigen receptor (TCR) localize to non-raft domains. Biochim Biophys Acta. (2015) 1853:802–9. doi: 10.1016/j.bbamcr.2014.12.017
27. Sproul TW, Malapati S, Kim J, Pierce SK. Cutting edge: B cell antigen receptor signaling occurs outside lipid rafts in immature B cells. J Immunol. (2000) 165:6020–3. doi: 10.4049/jimmunol.165.11.6020
28. Stone MB, Shelby SA, Nunez MF, Wisser K, Veatch SL. Protein sorting by lipid phase-like domains supports emergent signaling function in B lymphocyte plasma membranes. Elife. (2017) 6:e19891. doi: 10.7554/eLife.19891
29. Dinic J, Riehl A, Adler J, Parmryd I. The T cell receptor resides in ordered plasma membrane nanodomains that aggregate upon patching of the receptor. Sci Rep. (2015) 5:10082. doi: 10.1038/srep10082
30. Toledo A, Benach JL. Hijacking and use of host lipids by intracellular pathogens. Microbiol Spectr. (2015) 3. doi: 10.1128/microbiolspec.VMBF-0001-2014
31. Portnoy DA, Jacks PS, Hinrichs DJ. Role of hemolysin for the intracellular growth of Listeria monocytogenes. J Exp Med. (1988) 167:1459–71. doi: 10.1084/jem.167.4.1459
32. Gaillard JL, Berche P, Sansonetti P. Transposon mutagenesis as a tool to study the role of hemolysin in the virulence of Listeria monocytogenes. Infect Immun. (1986) 52:50–5. doi: 10.1128/IAI.52.1.50-55.1986
33. McKay DB, Lu CY. Listeriolysin as a virulence factor in Listeria monocytogenes infection of neonatal mice and murine decidual tissue. Infect Immun. (1991) 59:4286–90. doi: 10.1128/IAI.59.11.4286-4290.1991
34. Kathariou S, Metz P, Hof H, Goebel W. Tn916-induced mutations in the hemolysin determinant affecting virulence of Listeria monocytogenes. J Bacteriol. (1987) 169:1291–7. doi: 10.1128/JB.169.3.1291-1297.1987
35. Cossart P, Vicente MF, Mengaud J, Baquero F, Perez-Diaz JC, Berche P. Listeriolysin O is essential for virulence of Listeria monocytogenes: direct evidence obtained by gene complementation. Infect Immun. (1989) 57:3629–36. doi: 10.1128/IAI.57.11.3629-3636.1989
36. Nguyen BN, Peterson BN, Portnoy DA. Listeriolysin O: a phagosome-specific cytolysin revisited. Cell Microbiol. (2019) 21:e12988. doi: 10.1111/cmi.12988
37. Savinov SN, Heuck AP. Interaction of cholesterol with perfringolysin O: what have we learned from functional analysis? Toxins. (2017) 9:381. doi: 10.3390/toxins9120381
38. Christie MP, Johnstone BA, Tweten RK, Parker MW, Morton CJ. Cholesterol-dependent cytolysins: from water-soluble state to membrane pore. Biophys Rev. (2018) 10:1337–48. doi: 10.1007/s12551-018-0448-x
39. Vogele M, Bhaskara RM, Mulvihill E, van Pee K, Yildiz O, Kuhlbrandt W, et al. Membrane perforation by the pore-forming toxin pneumolysin. Proc Natl Acad Sci USA. (2019) 116:13352–7. doi: 10.1073/pnas.1904304116
40. Pleckaityte M. Cholesterol-dependent cytolysins produced by vaginal bacteria: certainties and controversies. Front Cell Infect Microbiol. (2019) 9:452. doi: 10.3389/fcimb.2019.00452
41. Jacobs T, Darji A, Frahm N, Rohde M, Wehland J, Chakraborty T, et al. Listeriolysin O: cholesterol inhibits cytolysis but not binding to cellular membranes. Mol Microbiol. (1998) 28:1081–9. doi: 10.1046/j.1365-2958.1998.00858.x
42. Bavdek A, Gekara NO, Priselac D, Gutierrez Aguirre I, Darji A, Chakraborty T, et al. Sterol and pH interdependence in the binding, oligomerization, and pore formation of Listeriolysin O. Biochemistry. (2007) 46:4425–37. doi: 10.1021/bi602497g
43. Schuerch DW, Wilson-Kubalek EM, Tweten RK. Molecular basis of listeriolysin O pH dependence. Proc Natl Acad Sci USA. (2005) 102:12537–42. doi: 10.1073/pnas.0500558102
44. Bavdek A, Kostanjsek R, Antonini V, Lakey JH, Dalla Serra M, Gilbert RJ, et al. pH dependence of listeriolysin O aggregation and pore-forming ability. FEBS J. (2012) 279:126–41. doi: 10.1111/j.1742-4658.2011.08405.x
45. Glomski IJ, Gedde MM, Tsang AW, Swanson JA, Portnoy DA. The Listeria monocytogenes hemolysin has an acidic pH optimum to compartmentalize activity and prevent damage to infected host cells. J Cell Biol. (2002) 156:1029–38. doi: 10.1083/jcb.200201081
46. Jones S, Portnoy DA. Characterization of Listeria monocytogenes pathogenesis in a strain expressing perfringolysin O in place of listeriolysin O. Infect Immun. (1994) 62:5608–13. doi: 10.1128/IAI.62.12.5608-5613.1994
47. Ruan Y, Rezelj S, Bedina Zavec A, Anderluh G, Scheuring S. Listeriolysin O membrane damaging activity involves arc formation and lineaction – implication for Listeria monocytogenes escape from phagocytic vacuole. PLoS Pathog. (2016) 12:e1005597. doi: 10.1371/journal.ppat.1005597
48. Chen C, Nguyen BN, Mitchell G, Margolis SR, Ma D, Portnoy DA. The Listeriolysin O PEST-like sequence Co-opts AP-2-mediated endocytosis to prevent plasma membrane damage during Listeria infection. Cell Host Microbe. (2018) 23:786–95 e785. doi: 10.1016/j.chom.2018.05.006
49. Rollason R, Korolchuk V, Hamilton C, Schu P, Banting G. Clathrin-mediated endocytosis of a lipid-raft-associated protein is mediated through a dual tyrosine motif. J Cell Sci. (2007) 120(Pt 21):3850–8. doi: 10.1242/jcs.003343
50. McMahon HT, Boucrot E. Molecular mechanism and physiological functions of clathrin-mediated endocytosis. Nat Rev Mol Cell Biol. (2011) 12:517–33. doi: 10.1038/nrm3151
51. Nygard Skalman L, Holst MR, Larsson E, Lundmark R. Plasma membrane damage caused by listeriolysin O is not repaired through endocytosis of the membrane pore. Biol Open. (2018) 7:bio.035287. doi: 10.1242/bio.035287
52. Wu N, Cernysiov V, Davidson D, Song H, Tang J, Luo S, et al. Critical role of lipid scramblase TMEM16F in phosphatidylserine exposure and repair of plasma mem brane after pore formation. Cell Rep. (2020) 30:1129–40 e1125. doi: 10.1016/j.celrep.2019.12.066
53. Gekara NO, Weiss S. Lipid rafts clustering and signalling by listeriolysin O. Biochem Soc Trans. (2004) 32(Pt 5):712–4. doi: 10.1042/BST0320712
54. Cossart P, Sansonetti PJ. Bacterial invasion: the paradigms of enteroinvasive pathogens. Science. (2004) 304:242–8. doi: 10.1126/science.1090124
55. Gessain G, Tsai YH, Travier L, Bonazzi M, Grayo S, Cossart P, et al. PI3-kinase activation is critical for host barrier permissiveness to Listeria monocytogenes. J Exp Med. (2015) 212:165–83. doi: 10.1084/jem.20141406
56. Ireton K, Payrastre B, Chap H, Ogawa W, Sakaue H, Kasuga M, et al. A role for phosphoinositide 3-kinase in bacterial invasion. Science. (1996) 274:780–2. doi: 10.1126/science.274.5288.780
57. Seveau S, Bierne H, Giroux S, Prevost MC, Cossart P. Role of lipid rafts in E-cadherin– and HGF-R/Met–mediated entry of Listeria monocytogenes into host cells. J Cell Biol. (2004) 166:743–53. doi: 10.1083/jcb.200406078
58. Bonazzi M, Veiga E, Pizarro-Cerda J, Cossart P. Successive post-translational modifications of E-cadherin are required for InlA-mediated internalization of Listeria monocytogenes. Cell Microbiol. (2008) 10:2208–22. doi: 10.1111/j.1462-5822.2008.01200.x
59. Seveau S, Tham TN, Payrastre B, Hoppe AD, Swanson JA, Cossart P. A FRET analysis to unravel the role of cholesterol in Rac1 and PI 3-kinase activation in the InlB/Met signalling pathway. Cell Microbiol. (2007) 9:790–803. doi: 10.1111/j.1462-5822.2006.00832.x
60. Ortega FE, Rengarajan M, Chavez N, Radhakrishnan P, Gloerich M, Bianchini J, et al. Adhesion to the host cell surface is sufficient to mediate Listeria monocytogenes entry into epithelial cells. Mol Biol Cell. (2017) 28:2945–57. doi: 10.1091/mbc.e16-12-0851
61. Lambrechts A, Gevaert K, Cossart P, Vandekerckhove J, Van Troys M. Listeria comet tails: the actin-based motility machinery at work. Trends Cell Biol. (2008) 18:220–7. doi: 10.1016/j.tcb.2008.03.001
62. Czuczman MA, Fattouh R, van Rijn JM, Canadien V, Osborne S, Muise AM, et al. Listeria monocytogenes exploits efferocytosis to promote cell-to-cell spread. Nature. (2014) 509:230–4. doi: 10.1038/nature13168
63. Ferracini M, Rios FJ, Pecenin M, Jancar S. Clearance of apoptotic cells by macrophages induces regulatory phenotype and involves stimulation of CD36 and platelet-activating factor receptor. Mediators Inflamm. (2013) 2013:950273. doi: 10.1155/2013/950273
64. Ishii H, Mori T, Shiratsuchi A, Nakai Y, Shimada Y, Ohno-Iwashita Y, et al. Distinct localization of lipid rafts and externalized phosphatidylserine at the surface of apoptotic cells. Biochem Biophys Res Commun. (2005) 327:94–9. doi: 10.1016/j.bbrc.2004.11.135
65. Kunzelmann-Marche C, Freyssinet JM, Martinez MC. Loss of plasma membrane phospholipid asymmetry requires raft integrity. Role of transient receptor potential channels and ERK pathway. J Biol Chem. (2002) 277:19876–81. doi: 10.1074/jbc.M200324200
66. Dhanda AS, Yu C, Lulic KT, Vogl AW, Rausch V, Yang D, et al. Listeria monocytogenes exploits host caveolin for cell-to-cell spreading. mBio. (2020) 11:e02857-19. doi: 10.1128/mBio.02857-19
67. Lajoie P, Nabi IR. Lipid rafts, caveolae, and their endocytosis. Int Rev Cell Mol Biol. (2010) 282:135–63. doi: 10.1016/S1937-6448(10)82003-9
68. Mahammad S, Parmryd I. Cholesterol depletion using methyl-beta-cyclodextrin. Methods Mol Biol. (2015) 1232:91–102. doi: 10.1007/978-1-4939-1752-5_8
69. Klein U, Gimpl G, Fahrenholz F. Alteration of the myometrial plasma membrane cholesterol content with beta-cyclodextrin modulates the binding affinity of the oxytocin receptor. Biochemistry. (1995) 34:13784–93. doi: 10.1021/bi00042a009
70. Subtil A, Gaidarov I, Kobylarz K, Lampson MA, Keen JH, McGraw TE. Acute cholesterol depletion inhibits clathrin-coated pit budding. Proc Natl Acad Sci USA. (1999) 96:6775–80. doi: 10.1073/pnas.96.12.6775
71. Rodal SK, Skretting G, Garred O, Vilhardt F, van Deurs B, Sandvig K. Extraction of cholesterol with methyl-beta-cyclodextrin perturbs formation of clathrin-coated endocytic vesicles. Mol Biol Cell. (1999) 10:961–74. doi: 10.1091/mbc.10.4.961
72. Razani B, Lisanti MP. Caveolin-deficient mice: insights into caveolar function human disease. J Clin Invest. (2001) 108:1553–61. doi: 10.1172/JCI200114611
73. Williams TM, Lisanti MP. The Caveolin genes: from cell biology to medicine. Ann Med. (2004) 36:584–95. doi: 10.1080/07853890410018899
74. Cohen AW, Hnasko R, Schubert W, Lisanti MP. Role of caveolae and caveolins in health and disease. Physiol Rev. (2004) 84:1341–79. doi: 10.1152/physrev.00046.2003
75. Nikitas G, Deschamps C, Disson O, Niault T, Cossart P, Lecuit M. Transcytosis of Listeria monocytogenes across the intestinal barrier upon specific targeting of goblet cell accessible E-cadherin. J Exp Med. (2011) 208:2263–77. doi: 10.1084/jem.20110560
76. Tsai YH, Disson O, Bierne H, Lecuit M. Murinization of internalin extends its receptor repertoire, altering Listeria monocytogenes cell tropism and host responses. PLoS Pathog. (2013) 9:e1003381. doi: 10.1371/journal.ppat.1003381
77. el Marjou F, Janssen KP, Chang BH, Li M, Hindie V, Chan L, et al. Tissue-specific and inducible Cre-mediated recombination in the gut epithelium. Genesis. (2004) 39:186–93. doi: 10.1002/gene.20042
78. Dutta D, Heo I, Clevers H. Disease modeling in stem cell-derived 3D organoid systems. Trends Mol Med. (2017) 23:393–410. doi: 10.1016/j.molmed.2017.02.007
79. Dekkers JF, Alieva M, Wellens LM, Ariese HCR, Jamieson PR, Vonk AM, et al. High-resolution 3D imaging of fixed and cleared organoids. Nat Protoc. (2019) 14:1756–71. doi: 10.1038/s41596-019-0160-8
Keywords: Listeria monocytogenes, listeriosis, lipid rafts, intracellular bacteria, listeriolysin O, internalin, cell-to-cell spreading
Citation: Tsai Y-H and Chen W-L (2020) Host Lipid Rafts as the Gates for Listeria monocytogenes Infection: A Mini-Review. Front. Immunol. 11:1666. doi: 10.3389/fimmu.2020.01666
Received: 25 April 2020; Accepted: 22 June 2020;
Published: 11 August 2020.
Edited by:
Chih-Ho Lai, Chang Gung University, College of Medicine, TaiwanReviewed by:
Fabrizia Stavru, CNRS UMR2001 Microbiologie Intégrative et Moléculaire, Institut Pasteur, FranceStephanie M. Seveau, The Ohio State University, United States
Copyright © 2020 Tsai and Chen. This is an open-access article distributed under the terms of the Creative Commons Attribution License (CC BY). The use, distribution or reproduction in other forums is permitted, provided the original author(s) and the copyright owner(s) are credited and that the original publication in this journal is cited, in accordance with accepted academic practice. No use, distribution or reproduction is permitted which does not comply with these terms.
*Correspondence: Yu-Huan Tsai, eXVodWFuLnRzYWkmI3gwMDA0MDt5bS5lZHUudHc=