- 1I. Department of Medicine, University Medical Center Hamburg-Eppendorf, Hamburg, Germany
- 2Martin Zeitz Centre for Rare Diseases, University Medical Center Hamburg-Eppendorf, Hamburg, Germany
The immune system responds differently in women and in men. Generally speaking, adult females show stronger innate and adaptive immune responses than males. This results in lower risk of developing most of the infectious diseases and a better ability to clear viral infection in women (1–5). On the other hand, women are at increased risk of developing autoimmune diseases (AID) such as rheumatoid arthritis, multiple sclerosis (MS), systemic lupus erythematosus (SLE), Sjögren's syndrome, and the autoimmune liver diseases autoimmune hepatitis (AIH) and primary biliary cholangitis (PBC) (6). Factors contributing to the female sex bias in autoimmune diseases include environmental exposure, e.g., microbiome, behavior, and genetics including X chromosomal inactivation of genes. Several lines of evidence and clinical observations clearly indicate that sex hormones contribute significantly to disease pathogenesis, and the role of estrogen in autoimmune diseases has been extensively studied. In many of these diseases, including the autoimmune liver diseases, T cells are thought to play an important pathogenetic role. We will use this mini-review to focus on the effects of androgens on T cells and how the two major androgens, testosterone and dihydrotestosterone, potentially contribute to the pathogenesis of autoimmune liver diseases (AILD).
Androgens in Steady State
The androgenic steroid hormones, testosterone, dihydrotestosterone (DHT), androstenedione, and dehydroepiandrostenone (DHEA) are generated from cholesterol (7). In men, the majority of testosterone precursors (>95%) are produced by Leydig cells in the testes and, to a lesser degree, by the adrenal glands. In women, testosterone precursors are produced by the adrenal glands, the thecal cells of the ovaries, and, during pregnancy, by the placenta (7–10). Metabolism of androgens is complex with testosterone generated from androstenedione in peripheral tissues and the conversion of testosterone into estrogen mediated by the enzyme aromatase in a context and tissue specific manner. Conversion of testosterone into DHT mainly occurs in the liver by the action of 5α-reductase, and DHT cannot be further metabolized to estrogen (11). Sixty-five to 70% of testosterone in blood is bound to sex hormone-binding globulin (SHGB) and 30–35% to albumin, which transport the hormone to target tissues. Only around 0.5–3% of testosterone is found freely in blood (9). Concentrations of bioavailable testosterone can be estimated with total testosterone, SHGB, and albumin serum levels (12).
Interestingly, women show blood androgen levels that are higher than the levels of estrogen. This is due to DHEA produced by the adrenal glands which is subsequently converted to testosterone via androstenedione (8). The levels of total testosterone in women range from 0.35 to 2.94 nmol/l, and there are no significant changes during daytime in testosterone and free testosterone levels (9, 13). In premenopausal women testosterone and free testosterone slightly peak midcycle, but DHT levels do not seem to change during the menstrual cycle (14, 15). With age and after menopause, testosterone levels in women decline, leading to significantly lower levels of testosterone, free testosterone, DHT, and SHGB (13).
In men, testosterone helps to regulate a variety of physiological processes including muscle mass and strength, bone mass, fat distribution, libido, and the production of sperm, red blood cells, and immune cells (11). Due to the complex metabolism of androgens and their tissue and context dependent conversion into estrogen, it is difficult to delineate the action of specific androgens within a given tissue in humans in vivo. For example, studies suggest that the effect of testosterone on male bone mass occurs mainly through its conversion to estrogen (16, 17). Serum testosterone levels are significantly higher in men than in women and typically range from 6.2 to 32.1 nmol/l (18). During daytime, a slight decrease in testosterone levels toward the afternoon can be observed (18). Testosterone production in men typically decreases with age to approximately the lower end of the mean levels observed in middle-aged adult men (12, 18–20).
Androgens Signal Through Cytosolic Androgen Receptor (AR) and Non-Classical Membrane Bound Receptors (mAR)
Cytosolic Androgen Receptor (AR)
Androgens, including testosterone and DHT, reach their target cells and signal through androgen receptors. In addition to the classical cytoplasmic androgen receptor (AR), androgens can also bind and activate membrane androgen receptors (mAR) (21). DHT binds the AR with a higher affinity and lower dissociation rate than testosterone, while testosterone probably has a higher affinity to the mAR (11). The expression of androgen receptors has been reported in many different tissues, in epithelial and endothelial cells, and in a variety of innate and adaptive immune cells, including human and mouse T cells (22–24).
The classical cytoplasmic AR is a member of the nuclear receptor superfamily and can act as a ligand-dependent transcription factor (25, 26). The human AR gene consists of 8 exons and is located on the X chromosome (27). It has a ligand-binding domain (LBD), a DNA-binding domain (DBD), and an N-terminal domain (NTD) (27). In an unbound state, the AR is residing in the cytoplasm in a complex with chaperons, heat-shock proteins, and cytoskeletal proteins (27, 28). The binding of ligands leads to a conformational change, receptor dimerization, and translocation to the nucleus (29). The NTD affects the transcriptional activity and the DBD permits the binding and recognition of androgen response elements (ARE) on target genes (27). The complex finally disassociates and returns to the cytoplasm (27). AR can also be post-translationally modified through phosphorylation, methylation, or ubiquitination, allowing for ligand-independent modulation of signaling (27, 29, 30).
Next to the regulation of gene transcription, AR interacts with PI3K (phosphoinositide-3-kinase), Src family kinase, and RAS GTPase (27). This interaction affects MAPK/ERK signaling and ERK translocates into the nucleus to affect transcriptional factors leading to adjustment of gene expression involved, e.g., in cell proliferation and survival (27, 28). In a complex but not yet fully elucidated process, mTOR, FOXO1, FOXO3a, HDAC3, STAT3, EGFR, and AKT were shown to be involved in non-genomic AR signaling (27, 28, 31–35).
Membrane Bound Androgen Receptors (mAR)
The zinc transporter ZIP9 (SLC39A9) has been identified as a membrane bound androgen receptor (mAR), interacting with several kinase pathways such as ERK1/2 and others (36–39). In human prostate cancer cells with overexpressed ZIP9 (PC-3-ZIP9) and breast cancer cells (MDA-MB-468), stimulation with testosterone leads to G proteins being activated, second messenger pathways, and elevation of intracellular free zinc, resulting in initiation of apoptosis and upregulation of pro-apoptotic genes such as BAX, p53, and Caspase-3 (36, 40). In the spermatogenic cell line GC-2, testosterone was shown to induce activation of ERK1/2 and the transcription factors ATF-1 and CREB through Zip9, which interacted with G-protein Gnα11 (38, 39).
The G-protein coupled receptor GPRC6A was suggested as another mAR, which has not yet been reported in a broad range of tissues. In vitro, GPRC6A phosphorylates ERK after testosterone stimulation in prostate cancer and bone marrow stromal cells (11, 41). One group showed the involvement of GPRC6A in testosterone production in Leydig cells (42). To our knowledge, however, the expression of GPRC6A in T cells is unknown, reflecting the general lack of knowledge on the role of membrane bound androgen receptors in the immune system.
Androgen Independent Receptor Signaling
AR signaling can also be induced independently from androgen binding. In prostate cancer cells, IL-6 dependent interplay with AR interferes with the PKA/PKC/MAPK pathway and IL-8 has been shown to promote their AR dependent growth and activation independent of androgens (11, 28, 42–44). Furthermore, IGF-1 stimulated AR phosphorylation, translocation to the nucleus, and upregulation of AR gene expression in myoblast C2C12 cell line (45, 46). These data suggest that inflammation associated changes in the cytokine milieu in an organ affected by autoimmune injury may significantly alter AR signaling. The liver is the central organ of androgen conversion, but so far, the effects of liver inflammation on testosterone metabolism and AR signaling have not yet been explored.
Moreover, the length of the CAG repeat region in exon 1 of the AR gene influences its signaling activity (47–49). Studies in men and women with systemic lupus erythematosus (SLE) and rheumatoid arthritis (RA) demonstrated variable and sex dependent effects of this heritable trait on disease severity and phenotype (50–53).
Overall, the activation and signaling of AR and mAR is complex, and crosstalk between AR transcriptional activity and non-genomic modification of AR- or mAR induced signaling cascades can lead to highly context dependent modification of androgen responses (27).
Androgens and Human T Cells
AR expression was identified in the majority of innate and adaptive immune cells suggesting that androgens directly modulate the function and development of immune cells. Already in the 1980s, AR expression was reported for human thymocytes (54). Thereafter, AR was found to be expressed on various human and mouse cells of the innate immune system, such as monocytes and macrophages from different tissues, ILC2 progenitors, neutrophils, and mast cells (55–61). In adaptive immunity, AR-expression was shown in human T cells, including CD8+ T cells and CD4+ and splenic CD4+ CD25+ T cells (55, 56, 62–64). In addition to AR, CD4+ and CD8+ T cells were shown to express mAR (65).
The effects of androgens on T cells were studied in vitro and by comparing male and female T cells ex vivo. It was found that Foxp3 expression, the Treg master transcription factor, was increased in human T cells after DHT treatment in vitro, and increased Treg frequencies were reported in men compared to women, and in boys already at the age of eight (66–68). Therefore, androgens may already influence the frequencies of T cells in vivo early in life. In adult men, there is a recent report of a negative correlation between CD3+, CD8+, and CD4+ T cells residing in adipose tissue and serum testosterone levels (69). Moreover, upon stimulation of healthy human PBMC with TLR8/9 ligands, secretion of IL-10 in male PBMC was higher than in female PBMC. Upon TLR7 stimulation, IFNα was lower in male PBMC. The amount of IL-10 upon TLR9 stimulation correlated to dehydroepiandrosterone sulfate levels in males, but this study cannot conclude whether these are direct or indirect effects on T cells via dendritic cells (70). Microarray analysis of restimulated T cells showed a higher expression of “pro-inflammatory” genes, such as IFNγ, IL12Rß2, LTß, GNLY, and GZMA in female T cells, while male T cells had a higher expression of IL10, IL5, and IL17A (71). Moreover, healthy male human naïve CD4 cells produced lower levels of IFNγ and had a trend of higher levels of IL-17A upon CD3/CD28 stimulation, possibly through upregulated PPARα and downregulated PPARγ1, and similar results were observed in mice (72–74).
Analysis of men under hormone replacement therapy could give new insight into the effects of androgens in vivo, although it is impossible to delineate these in vivo effects to single immune cell types such as T cells. Thus, in hypogonadal men a reduction in serum IL1ß and TNF, as well as an increase in IL-10, has been described following testosterone replacement treatment. Whether part of these observed differences related to changes in T cell subpopulations remained speculation (75). In a single case study with one hypogonadal man, an increase in naïve CD4+ CD45Ra+ cells could be observed that could be reverted upon androgen treatment (76). In prostate tissue of BPH (benign prostatic hyperplasia) patients undergoing 5α-reductase type II inhibitor treatment with finasteride leading to reduced intraprostatic DHT levels, a stronger infiltration of CD8+ T cells and higher CCL5 expression was observed (77). Moreover, in a follow-up study, the authors showed in vitro that in conditions of low androgen concentrations, CD8+ T cells were able to promote prostate epithelial cell proliferation, possibly through the CCL5/JAK-STAT5/CCND1 pathway (78). After androgen deprivation therapy (ADT) of prostate cancer patients, Wang et al. found enrichment of CD4low HLA-G+ T cells in peripheral blood, besides generally increased CD4+ T cell frequencies (79). In detail, these CD4low HLA-G+ T cells expressed IL-4, IL-17A, and RORγt, indicating an enrichment of IL-4 producing TH17 cells after ADT (79).
Testosterone therapy in transgender individuals offers further possibilities to study the effects of androgens on immune cells in vivo. Giltay et al. reported an increase in the IFNγ/IL-4 ratio and TNF production of PBMCs isolated from women undergoing hormone replacement therapy with testosterone. Cells were stimulated with PHA for 36 h and the results indicated increased TH1 differentiation (80). However, as these results contrast some of the above-mentioned studies, they should be further validated and it should be investigated in detail which cell type produced these cytokines.
Taken together, these results provide evidence that androgens influence T cell function and phenotype either directly or indirectly. However, in-depth and comprehensive analyses of direct and context dependent androgen effects on human T cells are lacking.
Effects of Testosterone on T Cells in Animal Models
Animal models have added to the knowledge on the effects of testosterone on immune cells. Olsen et al. observed a reduced thymus size within 2–4 h after testosterone injection of castrated male mice already in 1998. Mechanistically, increased apoptosis was induced in in vitro thymus tissue culture through the AR and reduced percentages of CD4+ CD8+ double positive thymocyte were detected in testosterone treated mice (81, 82). However, several other studies found no direct in vitro effect of testosterone on apoptosis of isolated thymocytes (82, 83). A potential explanation for this discrepancy could be that the thymic effects of androgens are mediated by AR expression on thymic epithelial cells (TEC) which are crucial for the negative selection of immature T cells (22, 84, 85). Reduction of androgen levels through castration of mice led to increased numbers of immature triple negative T cells and early T lineage progenitors and a decrease in mature CD4+ and CD8+ single positive cells in the thymus (86). More recently, thymic expression of AIRE (autoimmune regulator) in medullary TECs, which is involved in the thymic selection of T cells by clonal deletion of autoreactive T cells, has been reported to be higher in male human and mouse thymus, possibly induced by the effects of androgens through AR (87). However, ADT by castration of adult male mice did not change TCR diversity but increased the numbers of “naïve” CD44low CD4+ and CD8+ T cells within lymph nodes (88). Additionally, these mice recovered their T and B cells quicker than non-castrated controls after chemotherapy-induced lymphocyte depletion, and these androgen-deprived T cells were more prone to proliferate in vitro (88). Taken together, these data suggest that androgens affect T cell maturation and selection within the thymus either directly or indirectly via epithelial cells.
Regarding peripheral mature T cells, it has been shown that DHT treatment in female mice resulted in decreased IL-12 and increased IL-10 production compared to cells from untreated mice following aCD3 stimulation in vitro, and this difference was primarily caused by CD4+ T cells (55). Microarray analysis of splenic CD4+ T cells from castrated or control mice showed genes of IFN-signaling and T-helper cell pathways skewed into TH1 differentiation, including upregulation of IFNy, T-bet, and IL-12R (89). Additionally, CXCR3 expression was increased in CD4+ T cells of the castrated group suggesting suppressive effects of androgens on chemokine receptor expression relevant for tissue homing. Along this line, after castration there was an increase in CD3+ cells within lung and prostate tissue. A direct suppressive effect of testosterone on T cells was confirmed by a decrease of IFNy and T-bet expression found in splenic derived CD4+ T cells after treatment with synthetic testosterone in vitro (89). Further in vitro assays showed a reduction of STAT4 phosphorylation in CD4+ T cells upon androgen and IL-12 stimulation (89).
Confirming direct effects of androgens on mouse T cells, female T cell lines selected in the presence of DHT produced less IFNy and more IL-10 than control cell lines selected without the addition of DHT (63). Splenic derived mouse CD4+ T cells cultured with testosterone-enriched Leydig-conditioned medium showed induction of IL-10 secretion and increased Foxp3 expression, suggesting not only suppression of TH1 cytokines but also an increase in suppressor function of T cells induced by androgens (59, 63). In contrast to some previous reports of a shift toward TH2 cells, Jia et al. found reduced frequencies of TH1 and TH17 cells after in vitro DHT and aCD3 stimulation of mouse lymph node cells with no shift toward TH2 cells, possibly through enhanced autophagy in these cells (90). Recently, a reduction of murine in vitro TH1 and TH17 differentiation has been demonstrated by aromatase inhibitor treatment in combination with testosterone (91). In addition, visceral adipose tissue (VAT) from male mice showed higher Treg (CD4+ FOXP3+) frequencies then female VAT. The isolated Tregs showed differences regarding phenotype, chromatin accessibility, and transcriptional landscape. In particular, the expression of CCR2 was higher in male VAT Tregs compared to female Tregs. Female mice treated with testosterone showed an increased VAT expression of CCL2, the ligand for CCR2, and IL-6 and IL1ß, which likely stem from innate immune cells (92). These data show that the microenvironment including crosstalk with epithelial and innate immune cells clearly contributes to sex dependent differences observed in T cells.
Taken together, current knowledge suggests that androgens directly or indirectly affect T cell maturation, proliferation, and also their differentiation and cytokine production in mice and adult males. However, little is known on the direct effects of the different androgens on T cells, and specifically on the context dependent cellular and molecular mechanisms involved. Overall, androgens seem to induce a shift from TH1 effector T cells to a more suppressive phenotype. They also seem to enhance regulatory T cells. Clearly, more studies are needed that take into account signaling via classical and non-classical androgen receptors and the context dependent modulation of androgen signaling by an inflammatory microenvironment within tissues.
Effects of Androgens on T Cells in Autoimmunity
Autoimmune diseases (AIDs) are disorders characterized by an aberrant immune response against self-antigens. There are more than 60 different autoimmune diseases, which pose a major medical and societal challenge. The pathophysiology of most AIDs is complex and includes environmental, genetic, and epigenetic components. Most AIDs present with a strong female predisposition. MS, SLE, and the autoimmune liver disease PBC are among the diseases with the strongest female predominance (Table 1). While many AIDs occur more frequently in women, the course of disease may be more severe in men, exemplified by the worse disease course of male patients with MS or PBC (93, 119). Male PBC patients respond less to treatment with ursodeoxycholic acid and are at increased risk of disease progression and hepatocellular carcinoma development (119, 120). The mechanisms behind these apparent sex differences in disease susceptibility and severity are largely unknown.
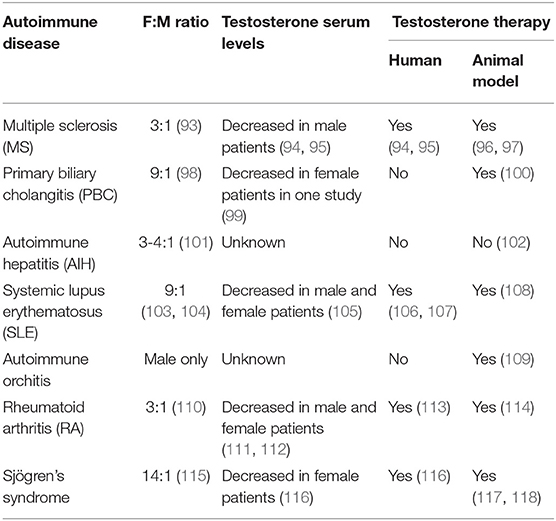
Table 1. Comparison of autoimmune diseases regarding female to male ratio, knowledge on testosterone serum levels, and therapeutic testosterone application.
PBC is a rare AID of the liver with a female to male ratio as high as 9:1 and characterized by the presence of anti-mitochondrial antibodies (AMAs), specific antinuclear antibodies (ANAs), and strong HLA associations (98, 121, 122). Immune responses directed against intrahepatic cholangiocytes, leading to the destruction and loss of small bile ducts (ductopenia) and portal inflammation with granuloma formation, are involved in the disease pathogenesis (121–123). In the other classical autoimmune liver disease, AIH, the female to male ratio is 3:1, and patients can present with elevated serum IgG-levels and/or hypergammaglobulinemia, elevated serum transaminase levels, and non-organ specific autoantibodies (101). The target cells of autoimmune attack in AIH are hepatocytes. The human leukocyte antigen alleles (HLA)-DRB1*03:01 and HLA-DRB1*04:01 are known risk factors for AIH and may also correlate with disease course, but they are not required for AIH development (124). Many lines of evidence support the involvement of CD4+ and CD8+ T cells in both diseases' pathogenesis (122, 125). Studies have investigated the effect of sex hormones on immune cells and how sex chromosomes including X chromosome inactivation affect the sex bias in AIDs (126–130). For example, in PBC an enhanced X monosomy rate within PBMC, possibly T and B cells, compared to healthy women was found, while XCI was random and similar to the controls (131–133). Both PBC and AIH have their age peak of manifestation around menopause, and both show disease modulation by pregnancy with greatly reduced AIH activity during pregnancy and frequent flares after delivery, strongly suggesting the involvement of sex hormones (134–137). Deciphering these mechanisms may lead to novel therapeutic strategies for many of these diseases. We will focus on the studies investigating androgens in the context of autoimmunity and T cells in mouse models and in the human autoimmune liver diseases, AIH and PBC.
There are few mouse models for autoimmune liver inflammation reflecting certain aspects of autoimmune liver diseases and in some of them, a female predominance is observed similar to human disease. In a mouse model of PBC (ARE-Del−/−), female mice showed increased serum levels of chemokines, such as MIG and IP-10, as well as increased cytokine levels including TNF, IL-10, and IL-13. They also showed increased expression of interferon Type I and II signaling in the liver compared to the male mice (138, 139). For chronic cholestatic liver inflammation and periductular fibrosis, the Mdr2−/− is a well-established mouse model. Already in 1997 Nieuwerk et al. described a more severe liver pathology in Mdr2−/− female mice compared to male mice which was associated with altered bile salt composition in bile (140). However, the impact of sex hormones on disease development in this model has not yet been investigated. We could recently identify an immunosuppressive effect of testosterone in an antigen dependent and T cell driven mouse model of experimental cholangitis. Cholangitis is induced by the transfer of antigen-specific CD8+ T cells (OT-1) which recognize their ovalbumine peptide antigen on cholangiocytes of recipient mice (100). This model shows a high female predominance. Furthermore, testosterone treatment completely suppressed liver inflammation in female mice and lack of testosterone rendered male mice susceptible to cholangitis development. Mechanistically, we could demonstrate that testosterone suppressed the expression of IL-17A by liver infiltrating lymphocytes and the hepatic expression of the lymphotropic chemokines CXCL-9 and CXCL-10 (100). Similar protective effects of testosterone were also shown in mouse models of MS and murine lupus (96, 97, 141–145). In these models, an influence of sex and androgens on the T cell expression of IFNγ and IL-10 was reported (63, 96, 97). The protective effect of testosterone on EAE development depended on androgen receptor expression and also on age, since older mice were not protected (146). In a mouse model of T cell mediated autoimmune diabetes (NOD mice), a higher in vitro CD4+ T cell production of IFNy was observed in female mice and of IL-4 in male mice, which was most prominent in young NOD mice (147). In experimental autoimmune orchitis (EAO), a rat model of a male AID called autoimmune orchitis, testosterone supplementation lead to a reduced incidence of EAO (109). Testosterone treatment decreased the frequencies/numbers of CD4+ T cells and macrophages in the testis, whereas frequencies of Treg populations increased. Furthermore, testosterone treatment resulted in reduced testicular expression of TNF, IL-6, and MCP-1 (CCL2) as well as in reduced secretion of IL-2 and IFNγ of ex vivo stimulated mononuclear testicular lymph node cells (109).
It has been difficult to establish mouse models for AIH and few truly represent features of human disease. In one model, xenoimmunization with human antigens (Cytochrome P450 2D6 and formiminotransferase-cyclodeaminase, which are type 2 AIH self-antigens) was used, based on the principle of molecular mimicry. This model showed a higher susceptibility in females compared to males (102, 148, 149). Adoptive transfer of ex vivo expanded CXCR3+ Tregs recovered peripheral tolerance and ameliorated disease, but neither castration nor estradiol treatment of these mice had any effect (102, 150). To our knowledge, supplementation with testosterone or DHT was not performed to investigate the suppressive effects androgens might exert.
In humans with autoimmune liver disease, increased serum levels of the proinflammatory cytokines, IFNγ and IL-17 in AIH and PBC patients were reported, while IL-10 was lower than in healthy controls (151). TNF was reduced in the sera of these patients compared to healthy controls, but a recent publication showed an enhanced production of TNF by liver and blood derived CD4+ T cells, with a majority of these cells identified as potentially pathogenic IFNγ co-producers (151). Furthermore, CD4+ T cells of PBC patients revealed increased expression and demethylation of CXCR3, which is the receptor for lymphotropic chemokines produced in inflamed liver (152). Although one older study showed reduced serum levels of testosterone in female PBC patients, it remains unclear whether altered sex hormone levels directly relate to some of the immunological alterations reported above (99).
Data from other AID suggest a role of testosterone in disease pathogenesis. Lower serum levels of testosterone were reported in men with MS compared to age matched healthy men, and testosterone levels seemed to correlate with disease severity (94). Another study suggested lower levels of testosterone in female MS patients compared to female age matched controls (94, 153). Of note, some pilot studies showed disease improvement upon testosterone treatment of male MS patients (94, 95, 154). Also for SLE, lower serum levels of testosterone were reported in affected women compared to age matched healthy women (105, 155, 156). The limitations of these and other studies, summarized in Table 1, are small cohort sizes, and they lack detailed clinical information and the use of now outdated analytical methods. Thus, studies regarding hormone levels in females with AIDs should be interpreted with caution.
Taken together, limited human data and studies using mouse models of autoimmune liver diseases hint to a higher production of proinflammatory cytokines by T cells, but a direct link to sex hormones and, specifically, androgen levels remains unclear. The novel finding of intestinal microbiota associated changes in testosterone serum levels in mice should spark interest in the role of the microbiome for sex differences in autoimmune liver diseases, which are clearly linked to an altered intestinal microbiota (157–159).
Concluding Remarks
The mechanisms behind the sex differences observed in the autoimmune liver diseases PBC and AIH, specifically the female predominance and worse disease course in male PBC patients, remain largely unknown. Emerging evidence mainly from murine studies suggests immunosuppressive effects of androgens on T cells (Figure 1). More studies are needed to decipher signaling pathways involved in T cells upon androgen stimulation including the classical and non-classical androgen receptors and their modulation by the local microenvironment. Understanding the effects of androgens on immune cells may pave the way for novel treatment strategies for autoimmune liver diseases.
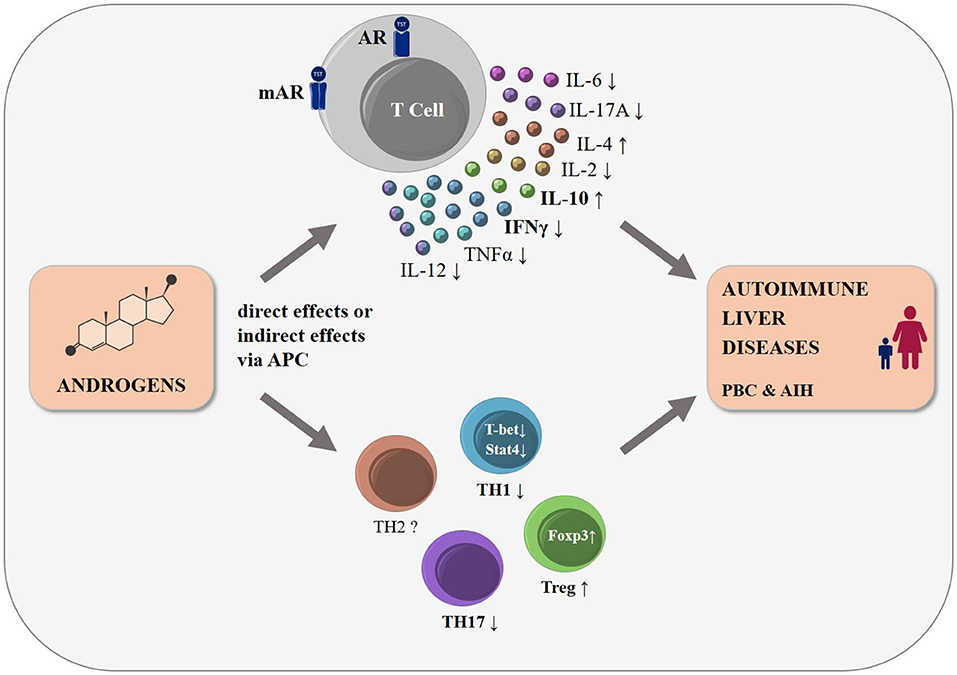
Figure 1. The influence of androgens on T cell function and differentiation: schematic representation. Many autoimmune diseases, including the autoimmune liver diseases PBC and AIH, show a strong female predominance. Androgens modulate T cell development already in the thymus, mainly by altering thymic epithelial cell function (not shown). Human and mouse T cells express cytosolic (AR) and membrane bound androgen receptors (mAR). Androgens lead to changes in cytokine expression in T cells either directly or indirectly via antigen presenting cells, with a shift to a decreased pro-inflammatory cytokine expression, such as IFNγ and TNF, and an increased secretion of anti-inflammatory cytokines such as IL-10 and IL-4. Androgens were reported to reduce TH1-, TH17-, and to increase Treg-differentiation, while changes in other T cell subpopulations (e.g., TH2 cells) remain less clear. We postulate that these androgen-induced effects may influence the incidence and disease course of the T cell driven autoimmune liver diseases, PBC and AIH.
Author Contributions
LH, DS, and CS designed and wrote the manuscript. All authors contributed to the article and approved the submitted version.
Funding
CS was supported by the Helmut and Hannelore Greve and YAEL Foundation, DFG (SFB841 and KFO306), CS, DS, and LH are supported by the Landesforschungsförderung Hamburg LFF-FV 45.
Conflict of Interest
The authors declare that the research was conducted in the absence of any commercial or financial relationships that could be construed as a potential conflict of interest.
Acknowledgments
We are grateful for critical reading by Elaine Hussey.
Abbreviations
AID, autoimmune diseases; SHGB, sex hormone-binding globulin; DHT, dihydrotestosterone; AR, androgen receptor; mAR, membrane bound androgen receptors; LBD, ligand-binding domain; DBD, DNA-binding domain; NTD, N-terminal domain; ARE, androgen response elements; PI3K, phosphoinositide-3-kinase; CREB, cAMP response element-binding protein; Treg, regulatory T cell; BPH, benign prostatic hyperplasia; ADT, androgen deprivation therapy; AIRE, autoimmune regulator; VAT, visceral adipose tissue; MS, Multiple sclerosis; PBC, primary biliary cholangitis; AIH, autoimmune hepatitis; SLE, systemic lupus erythematosus; EAE, autoimmune encephalomyelitis; AMAs, anti-mitochondrial antibodies; ANAs, antinuclear antibodies; EAO, experimental autoimmune orchitis.
References
1. Fish EN. The X-files in immunity: sex-based differences predispose immune responses. Nat Rev Immunol. (2008) 8:737–44. doi: 10.1038/nri2394
2. Bernin H, Lotter H. Sex bias in the outcome of human tropical infectious diseases: influence of steroid hormones. J Infect Dis. (2014) 209(Suppl. 3):S107–13. doi: 10.1093/infdis/jit610
3. Sellau J, Groneberg M, Lotter H. Androgen-dependent immune modulation in parasitic infection. Semin Immunopathol. (2019) 41:213–24. doi: 10.1007/s00281-018-0722-9
4. Guess TE, Rosen J, Castro-Lopez N, Wormley FL Jr, McClelland EE. An inherent T cell deficit in healthy males to C. neoformans infection may begin to explain the sex susceptibility in incidence of cryptococcosis. Biol Sex Differ. (2019) 10:44. doi: 10.1186/s13293-019-0258-2
5. Grebely J, Page K, Sacks-Davis R, van der Loeff MS, Rice TM, Bruneau J, et al. The effects of female sex, viral genotype, and IL28B genotype on spontaneous clearance of acute hepatitis C virus infection. Hepatology. (2014) 59:109–20. doi: 10.1002/hep.26639
6. Ngo ST, Steyn FJ, McCombe PA. Gender differences in autoimmune disease. Front Neuroendocrinol. (2014) 35:347–69. doi: 10.1016/j.yfrne.2014.04.004
7. Prough RA, Clark BJ, Klinge CM. Novel mechanisms for DHEA action. J Mol Endocrinol. (2016) 56:R139–55. doi: 10.1530/JME-16-0013
8. Burger HG. Androgen production in women. Fertil Steril. (2002) 77(Suppl. 4):S3–5. doi: 10.1016/S0015-0282(02)02985-0
9. Zimmerman Y, Eijkemans MJ, Coelingh Bennink HJ, Blankenstein MA, Fauser BC. The effect of combined oral contraception on testosterone levels in healthy women: a systematic review and meta-analysis. Hum Reprod Update. (2014) 20:76–105. doi: 10.1093/humupd/dmt038
10. Zouboulis CC, Degitz K. Androgen action on human skin – from basic research to clinical significance. Exp Dermatol. (2004) 13(Suppl. 4):5–10. doi: 10.1111/j.1600-0625.2004.00255.x
11. Davey RA, Grossmann M. Androgen receptor structure, function and biology: from bench to bedside. Clin Biochem Rev. (2016) 37:3–15.
12. Vermeulen A, Goemaere S, Kaufman JM. Testosterone, body composition and aging. J Endocrinol Invest. (1999) 22(5 Suppl):110–6.
13. Haring R, Hannemann A, John U, Radke D, Nauck M, Wallaschofski H, et al. Age-specific reference ranges for serum testosterone and androstenedione concentrations in women measured by liquid chromatography-tandem mass spectrometry. J Clin Endocrinol Metab. (2012) 97:408–15. doi: 10.1210/jc.2011-2134
14. Rothman MS, Carlson NE, Xu M, Wang C, Swerdloff R, Lee P, et al. Reexamination of testosterone, dihydrotestosterone, estradiol and estrone levels across the menstrual cycle and in postmenopausal women measured by liquid chromatography-tandem mass spectrometry. Steroids. (2011) 76:177–82. doi: 10.1016/j.steroids.2010.10.010
15. Swerdloff RS, Dudley RE, Page ST, Wang C, Salameh WA. Dihydrotestosterone: biochemistry, physiology, and clinical implications of elevated blood levels. Endocr Rev. (2017) 38:220–54. doi: 10.1210/er.2016-1067
16. Sinnesael M, Boonen S, Claessens F, Gielen E, Vanderschueren D. Testosterone and the male skeleton: a dual mode of action. J Osteoporos. (2011) 2011:240328. doi: 10.4061/2011/240328
17. Clarke BL, Khosla S. Androgens and bone. Steroids. (2009) 74:296–305. doi: 10.1016/j.steroids.2008.10.003
18. Friedrich N, Volzke H, Rosskopf D, Steveling A, Krebs A, Nauck M, et al. Reference ranges for serum dehydroepiandrosterone sulfate and testosterone in adult men. J Androl. (2008) 29:610–7. doi: 10.2164/jandrol.108.005561
19. Vermeulen A. Ageing, hormones, body composition, metabolic effects. World J Urol. (2002) 20:23–7. doi: 10.1007/s00345-002-0257-4
20. Tenover JS. Effects of testosterone supplementation in the aging male. J Clin Endocrinol Metab. (1992) 75:1092–8. doi: 10.1210/jc.75.4.1092
21. Estrada M, Espinosa A, Muller M, Jaimovich E. Testosterone stimulates intracellular calcium release and mitogen-activated protein kinases via a G protein-coupled receptor in skeletal muscle cells. Endocrinology. (2003) 144:3586–97. doi: 10.1210/en.2002-0164
22. Olsen NJ, Olson G, Viselli SM, Gu X, Kovacs WJ. Androgen receptors in thymic epithelium modulate thymus size and thymocyte development. Endocrinology. (2001) 142:1278–83. doi: 10.1210/endo.142.3.8032
23. Death AK, McGrath KC, Sader MA, Nakhla S, Jessup W, Handelsman DJ, et al. Dihydrotestosterone promotes vascular cell adhesion molecule-1 expression in male human endothelial cells via a nuclear factor-kappaB-dependent pathway. Endocrinology. (2004) 145:1889–97. doi: 10.1210/en.2003-0789
24. Huang CK, Pang H, Wang L, Niu Y, Luo J, Chang E, et al. New therapy via targeting androgen receptor in monocytes/macrophages to battle atherosclerosis. Hypertension. (2014) 63:1345–53. doi: 10.1161/HYPERTENSIONAHA.113.02804
25. Sakkiah S, Ng HW, Tong W, Hong H. Structures of androgen receptor bound with ligands: advancing understanding of biological functions and drug discovery. Expert Opin Ther Targets. (2016) 20:1267–82. doi: 10.1080/14728222.2016.1192131
26. Edelsztein NY, Rey RA. Importance of the androgen receptor signaling in gene transactivation and transrepression for pubertal maturation of the testis. Cells. (2019) 8:861. doi: 10.3390/cells8080861
27. Pietri E, Conteduca V, Andreis D, Massa I, Melegari E, Sarti S, et al. Androgen receptor signaling pathways as a target for breast cancer treatment. Endocr Relat Cancer. (2016) 23:R485–98. doi: 10.1530/ERC-16-0190
28. Leung JK, Sadar MD. Non-genomic actions of the androgen receptor in prostate cancer. Front Endocrinol. (2017) 8:2. doi: 10.3389/fendo.2017.00002
29. Bennett NC, Gardiner RA, Hooper JD, Johnson DW, Gobe GC. Molecular cell biology of androgen receptor signalling. Int J Biochem Cell Biol. (2010) 42:813–27. doi: 10.1016/j.biocel.2009.11.013
30. Koryakina Y, Ta HQ, Gioeli D. Androgen receptor phosphorylation: biological context and functional consequences. Endocr Relat Cancer. (2014) 21:T131–45. doi: 10.1530/ERC-13-0472
31. Liu X, Qing S, Che K, Li L, Liao X. Androgen receptor promotes oral squamous cell carcinoma cell migration by increasing EGFR phosphorylation. Onco Targets Ther. (2019) 12:4245–52. doi: 10.2147/OTT.S200718
32. Turkson J, Bowman T, Garcia R, Caldenhoven E, De Groot RP, Jove R. Stat3 activation by Src induces specific gene regulation and is required for cell transformation. Mol Cell Biol. (1998) 18:2545–52. doi: 10.1128/MCB.18.5.2545
33. Garcia R, Bowman TL, Niu G, Yu H, Minton S, Muro-Cacho CA, et al. Constitutive activation of Stat3 by the Src and JAK tyrosine kinases participates in growth regulation of human breast carcinoma cells. Oncogene. (2001) 20:2499–513. doi: 10.1038/sj.onc.1204349
34. Yang L, Xie S, Jamaluddin MS, Altuwaijri S, Ni J, Kim E, et al. Induction of androgen receptor expression by phosphatidylinositol 3-kinase/Akt downstream substrate, FOXO3a, and their roles in apoptosis of LNCaP prostate cancer cells. J Biol Chem. (2005) 280:33558–65. doi: 10.1074/jbc.M504461200
35. Liu P, Li S, Gan L, Kao TP, Huang H. A transcription-independent function of FOXO1 in inhibition of androgen-independent activation of the androgen receptor in prostate cancer cells. Cancer Res. (2008) 68:10290–9. doi: 10.1158/0008-5472.CAN-08-2038
36. Thomas P, Pang Y, Dong J, Berg AH. Identification and characterization of membrane androgen receptors in the ZIP9 zinc transporter subfamily: II. Role of human ZIP9 in testosterone-induced prostate and breast cancer cell apoptosis. Endocrinology. (2014) 155:4250–65. doi: 10.1210/en.2014-1201
37. Converse A, Zhang C, Thomas P. Membrane androgen receptor zip9 induces croaker ovarian cell apoptosis via stimulatory G protein α subunit and MAP kinase signaling. Endocrinology. (2017) 158:3015–29. doi: 10.1210/en.2017-00087
38. Shihan M, Bulldan A, Scheiner-Bobis G. Non-classical testosterone signaling is mediated by a G-protein-coupled receptor interacting with Gnα11. Biochim Biophys Acta. (2014) 1843:1172–81. doi: 10.1016/j.bbamcr.2014.03.002
39. Shihan M, Chan KH, Konrad L, Scheiner-Bobis G. Non-classical testosterone signaling in spermatogenic GC-2 cells is mediated through ZIP9 interacting with Gnalpha11. Cell Signal. (2015) 27:2077–86. doi: 10.1016/j.cellsig.2015.07.013
40. Thomas P, Pang Y, Dong J. Membrane androgen receptor characteristics of human ZIP9 (SLC39A) zinc transporter in prostate cancer cells: androgen-specific activation and involvement of an inhibitory G protein in zinc and MAP kinase signaling. Mol Cell Endocrinol. (2017) 447:23–34. doi: 10.1016/j.mce.2017.02.025
41. Pi M, Parrill AL, Quarles LD. GPRC6A mediates the non-genomic effects of steroids. J Biol Chem. (2010) 285:39953–64. doi: 10.1074/jbc.M110.158063
42. Bagchi G, Wu J, French J, Kim J, Moniri NH, Daaka Y. Androgens transduce the G alphas-mediated activation of protein kinase A in prostate cells. Cancer Res. (2008) 68:3225–31. doi: 10.1158/0008-5472.CAN-07-5026
43. Ueda T, Bruchovsky N, Sadar MD. Activation of the androgen receptor N-terminal domain by interleukin-6 via MAPK and STAT3 signal transduction pathways. J Biol Chem. (2002) 277:7076–85. doi: 10.1074/jbc.M108255200
44. Lee LF, Louie MC, Desai SJ, Yang J, Chen HW, Evans CP, et al. Interleukin-8 confers androgen-independent growth and migration of LNCaP: differential effects of tyrosine kinases Src and FAK. Oncogene. (2004) 23:2197–205. doi: 10.1038/sj.onc.1207344
45. Kim HJ, Lee WJ. Ligand-independent activation of the androgen receptor by insulin-like growth factor-I and the role of the MAPK pathway in skeletal muscle cells. Mol Cells. (2009) 28:589–93. doi: 10.1007/s10059-009-0167-z
46. Kim HJ, Lee WJ. Insulin-like growth factor-I induces androgen receptor activation in differentiating C2C12 skeletal muscle cells. Mol Cells. (2009) 28:189–94. doi: 10.1007/s10059-009-0118-8
47. Zitzmann M. The role of the CAG repeat androgen receptor polymorphism in andrology. Front Horm Res. (2009) 37:52–61. doi: 10.1159/000175843
48. Zinn AR, Ramos P, Elder FF, Kowal K, Samango-Sprouse C, Ross JL. Androgen receptor CAGn repeat length influences phenotype of 47,XXY (Klinefelter) syndrome. J Clin Endocrinol Metab. (2005) 90:5041–6. doi: 10.1210/jc.2005-0432
49. Beilin J, Ball EM, Favaloro JM, Zajac JD. Effect of the androgen receptor CAG repeat polymorphism on transcriptional activity: specificity in prostate and non-prostate cell lines. J Mol Endocrinol. (2000) 25:85–96. doi: 10.1677/jme.0.0250085
50. Tessnow AH, Olsen NJ, Kovacs WJ. Expression of humoral autoimmunity is related to androgen receptor CAG repeat length in men with systemic lupus erythematosus. J Clin Immunol. (2011) 31:567–73. doi: 10.1007/s10875-011-9519-5
51. Doukas C, Saltiki K, Mantzou A, Cimponeriu A, Terzidis K, Sarika L, et al. Hormonal parameters and sex hormone receptor gene polymorphisms in men with autoimmune diseases. Rheumatol Int. (2013) 33:575–82. doi: 10.1007/s00296-012-2386-4
52. Dziedziejko V, Kurzawski M, Safranow K, Ossowski A, Piatek J, Parafiniuk M, et al. CAG repeat polymorphism in the androgen receptor gene in women with rheumatoid arthritis. J Rheumatol. (2012) 39:10–7. doi: 10.3899/jrheum.110894
53. Olsen NJ, Benko AL, Kovacs WJ. Variation in the androgen receptor gene exon 1 CAG repeat correlates with manifestations of autoimmunity in women with lupus. Endocr Connect. (2014) 3:99–109. doi: 10.1530/EC-14-0039
55. Liva SM, Voskuhl RR. Testosterone acts directly on CD4+ T lymphocytes to increase IL-10 production. J Immunol. (2001) 167:2060–7. doi: 10.4049/jimmunol.167.4.2060
56. McCrohon JA, Death AK, Nakhla S, Jessup W, Handelsman DJ, Stanley KK, et al. Androgen receptor expression is greater in macrophages from male than from female donors. A sex difference with implications for atherogenesis. Circulation. (2000) 101:224–6. doi: 10.1161/01.CIR.101.3.224
57. Cutolo M, Accardo S, Villaggio B, Barone A, Sulli A, Coviello DA, et al. Androgen and estrogen receptors are present in primary cultures of human synovial macrophages. J Clin Endocrinol Metab. (1996) 81:820–7. doi: 10.1210/jcem.81.2.8636310
58. Laffont S, Blanquart E, Savignac M, Cenac C, Laverny G, Metzger D, et al. Androgen signaling negatively controls group 2 innate lymphoid cells. J Exp Med. (2017) 214:1581–92. doi: 10.1084/jem.20161807
59. Fijak M, Damm LJ, Wenzel JP, Aslani F, Walecki M, Wahle E, et al. Influence of testosterone on inflammatory response in testicular cells and expression of transcription factor Foxp3 in T cells. Am J Reprod Immunol. (2015) 74:12–25. doi: 10.1111/aji.12363
60. Chen W, Beck I, Schober W, Brockow K, Effner R, Buters JT, et al. Human mast cells express androgen receptors but treatment with testosterone exerts no influence on IgE-independent mast cell degranulation elicited by neuromuscular blocking agents. Exp Dermatol. (2010) 19:302–4. doi: 10.1111/j.1600-0625.2009.00969.x
61. Mantalaris A, Panoskaltsis N, Sakai Y, Bourne P, Chang C, Messing EM, et al. Localization of androgen receptor expression in human bone marrow. J Pathol. (2001) 193:361–6. doi: 10.1002/1096-9896(0000)9999:9999<::AID-PATH803>3.0.CO;2-W
62. Viselli SM, Olsen NJ, Shults K, Steizer G, Kovacs WJ. Immunochemical and flow cytometric analysis of androgen receptor expression in thymocytes. Mol Cell Endocrinol. (1995) 109:19–26. doi: 10.1016/0303-7207(95)03479-Q
63. Bebo BF Jr, Schuster JC, Vandenbark AA, Offner H. Androgens alter the cytokine profile and reduce encephalitogenicity of myelin-reactive T cells. J Immunol. (1999) 162:35–40.
64. Russi AE, Ebel ME, Yang Y, Brown MA. Male-specific IL-33 expression regulates sex-dimorphic EAE susceptibility. Proc Natl Acad Sci USA. (2018) 115:E1520–9. doi: 10.1073/pnas.1710401115
65. Benten WP, Lieberherr M, Giese G, Wrehlke C, Stamm O, Sekeris CE, et al. Functional testosterone receptors in plasma membranes of T cells. FASEB J. (1999) 13:123–33. doi: 10.1096/fasebj.13.1.123
66. Afshan G, Afzal N, Qureshi S. CD4+CD25(hi) regulatory T cells in healthy males and females mediate gender difference in the prevalence of autoimmune diseases. Clin Lab. (2012) 58:567–71.
67. Walecki M, Eisel F, Klug J, Baal N, Paradowska-Dogan A, Wahle E, et al. Androgen receptor modulates Foxp3 expression in CD4+CD25+Foxp3+ regulatory T-cells. Mol Biol Cell. (2015) 26:2845–57. doi: 10.1091/mbc.E14-08-1323
68. Lundell AC, Nordstrom I, Andersson K, Strombeck A, Ohlsson C, Tivesten A, et al. Dihydrotestosterone levels at birth associate positively with higher proportions of circulating immature/naive CD5(+) B cells in boys. Sci Rep. (2017) 7:15503. doi: 10.1038/s41598-017-15836-1
69. Rubinow KB, Chao JH, Hagman D, Kratz M, van Yserloo B, Gaikwad NW, et al. Circulating sex steroids coregulate adipose tissue immune cell populations in healthy men. Am J Physiol Endocrinol Metab. (2017) 313:E528–39. doi: 10.1152/ajpendo.00075.2017
70. Torcia MG, Nencioni L, Clemente AM, Civitelli L, Celestino I, Limongi D, et al. Sex differences in the response to viral infections: TLR8 and TLR9 ligand stimulation induce higher IL10 production in males. PLoS ONE. (2012) 7:e39853. doi: 10.1371/journal.pone.0039853
71. Hewagama A, Patel D, Yarlagadda S, Strickland FM, Richardson BC. Stronger inflammatory/cytotoxic T-cell response in women identified by microarray analysis. Genes Immun. (2009) 10:509–16. doi: 10.1038/gene.2009.12
72. Zhang MA, Rego D, Moshkova M, Kebir H, Chruscinski A, Nguyen H, et al. Peroxisome proliferator-activated receptor (PPAR)α and -γ regulate IFNγ and IL-17A production by human T cells in a sex-specific way. Proc Natl Acad Sci USA. (2012) 109:9505–10. doi: 10.1073/pnas.1118458109
73. Zhang MA, Ahn JJ, Zhao FL, Selvanantham T, Mallevaey T, Stock N, et al. Antagonizing peroxisome proliferator-activated receptor alpha activity selectively enhances th1 immunity in male mice. J Immunol. (2015) 195:5189–202. doi: 10.4049/jimmunol.1500449
74. Dunn SE, Ousman SS, Sobel RA, Zuniga L, Baranzini SE, Youssef S, et al. Peroxisome proliferator-activated receptor (PPAR)alpha expression in T cells mediates gender differences in development of T cell-mediated autoimmunity. J Exp Med. (2007) 204:321–30. doi: 10.1084/jem.20061839
75. Malkin CJ, Pugh PJ, Jones RD, Kapoor D, Channer KS, Jones TH. The effect of testosterone replacement on endogenous inflammatory cytokines and lipid profiles in hypogonadal men. J Clin Endocrinol Metab. (2004) 89:3313–8. doi: 10.1210/jc.2003-031069
76. Olsen NJ, Kovacs WJ. Evidence that androgens modulate human thymic T cell output. J Investig Med. (2011) 59:32–5. doi: 10.2310/JIM.0b013e318200dc98
77. Fan Y, Hu S, Liu J, Xiao F, Li X, Yu W, et al. Low intraprostatic DHT promotes the infiltration of CD8+ T cells in BPH tissues via modulation of CCL5 secretion. Mediators Inflamm. (2014) 2014:397815. doi: 10.1155/2014/397815
78. Yang Y, Hu S, Liu J, Cui Y, Fan Y, Lv T, et al. CD8+ T cells promote proliferation of benign prostatic hyperplasia epithelial cells under low androgen level via modulation of CCL5/STAT5/CCND1 signaling pathway. Sci Rep. (2017) 7:42893. doi: 10.1038/srep42893
79. Wang C, Chen J, Zhang Q, Li W, Zhang S, Xu Y, et al. Elimination of CD4(low)HLA-G(+) T cells overcomes castration-resistance in prostate cancer therapy. Cell Res. (2018) 28:1103–17. doi: 10.1038/s41422-018-0089-4
80. Giltay EJ, Fonk JC, von Blomberg BM, Drexhage HA, Schalkwijk C, Gooren LJ. In vivo effects of sex steroids on lymphocyte responsiveness and immunoglobulin levels in humans. J Clin Endocrinol Metab. (2000) 85:1648–57. doi: 10.1210/jcem.85.4.6562
81. Olsen NJ, Viselli SM, Fan J, Kovacs WJ. Androgens accelerate thymocyte apoptosis. Endocrinology. (1998) 139:748–52. doi: 10.1210/endo.139.2.5729
82. Dulos GJ, Bagchus WM. Androgens indirectly accelerate thymocyte apoptosis. Int Immunopharmacol. (2001) 1:321–8. doi: 10.1016/S1567-5769(00)00029-1
83. Olsen NJ, Watson MB, Henderson GS, Kovacs WJ. Androgen deprivation induces phenotypic and functional changes in the thymus of adult male mice. Endocrinology. (1991) 129:2471–6. doi: 10.1210/endo-129-5-2471
84. Velardi E, Tsai JJ, Holland AM, Wertheimer T, Yu VW, Zakrzewski JL, et al. Sex steroid blockade enhances thymopoiesis by modulating Notch signaling. J Exp Med. (2014) 211:2341–9. doi: 10.1084/jem.20131289
85. Dumont-Lagace M, St-Pierre C, Perreault C. Sex hormones have pervasive effects on thymic epithelial cells. Sci Rep. (2015) 5:12895. doi: 10.1038/srep12895
86. Heng TS, Goldberg GL, Gray DH, Sutherland JS, Chidgey AP, Boyd RL. Effects of castration on thymocyte development in two different models of thymic involution. J Immunol. (2005) 175:2982–93. doi: 10.4049/jimmunol.175.5.2982
87. Zhu ML, Bakhru P, Conley B, Nelson JS, Free M, Martin A, et al. Sex bias in CNS autoimmune disease mediated by androgen control of autoimmune regulator. Nat Commun. (2016) 7:11350. doi: 10.1038/ncomms11350
88. Roden AC, Moser MT, Tri SD, Mercader M, Kuntz SM, Dong H, et al. Augmentation of T cell levels and responses induced by androgen deprivation. J Immunol. (2004) 173:6098–108. doi: 10.4049/jimmunol.173.10.6098
89. Kissick HT, Sanda MG, Dunn LK, Pellegrini KL, On ST, Noel JK, et al. Androgens alter T-cell immunity by inhibiting T-helper 1 differentiation. Proc Natl Acad Sci USA. (2014) 111:9887–92. doi: 10.1073/pnas.1402468111
90. Jia T, Anandhan A, Massilamany C, Rajasekaran RA, Franco R, Reddy J. Association of autophagy in the cell death mediated by dihydrotestosterone in autoreactive t cells independent of antigenic stimulation. J Neuroimmune Pharmacol. (2015) 10:620–34. doi: 10.1007/s11481-015-9633-x
91. Massa MG, David C, Jorg S, Berg J, Gisevius B, Hirschberg S, et al. Testosterone differentially affects T cells and neurons in murine and human models of neuroinflammation and neurodegeneration. Am J Pathol. (2017) 187:1613–22. doi: 10.1016/j.ajpath.2017.03.006
92. Vasanthakumar A, Chisanga D, Blume J, Gloury R, Britt K, Henstridge DC, et al. Sex-specific adipose tissue imprinting of regulatory T cells. Nature. (2020) 579:581–5. doi: 10.1038/s41586-020-2040-3
93. Bove R, Chitnis T. The role of gender and sex hormones in determining the onset and outcome of multiple sclerosis. Mult Scler. (2014) 20:520–6. doi: 10.1177/1352458513519181
94. Chitnis T. The role of testosterone in MS risk and course. Mult Scler. (2018) 24:36–41. doi: 10.1177/1352458517737395
95. Sicotte NL, Giesser BS, Tandon V, Klutch R, Steiner B, Drain AE, et al. Testosterone treatment in multiple sclerosis: a pilot study. Arch Neurol. (2007) 64:683–8. doi: 10.1001/archneur.64.5.683
96. Wekerle H, Kojima K, Lannes-Vieira J, Lassmann H, Linington C. Animal models. Ann Neurol. (1994) 36:S47–53. doi: 10.1002/ana.410360714
97. Dalal M, Kim S, Voskuhl RR. Testosterone therapy ameliorates experimental autoimmune encephalomyelitis and induces a T helper 2 bias in the autoantigen-specific T lymphocyte response. J Immunol. (1997) 159:3–6.
98. Carbone M, Neuberger JM. Autoimmune liver disease, autoimmunity and liver transplantation. J Hepatol. (2014) 60:210–23. doi: 10.1016/j.jhep.2013.09.020
99. Floreani A, Paternoster D, Mega A, Farinati F, Plebani M, Baldo V, et al. Sex hormone profile and endometrial cancer risk in primary biliary cirrhosis: a case-control study. Eur J Obstet Gynecol Reprod Biol. (2002) 103:154–7. doi: 10.1016/S0301-2115(02)00046-5
100. Schwinge D, Carambia A, Quaas A, Krech T, Wegscheid C, Tiegs G, et al. Testosterone suppresses hepatic inflammation by the downregulation of IL-17, CXCL-9, and CXCL-10 in a mouse model of experimental acute cholangitis. J Immunol. (2015) 194:2522–30. doi: 10.4049/jimmunol.1400076
101. European Association for the Study of the L. EASL clinical practice guidelines: autoimmune hepatitis. J Hepatol. (2015) 63:971–1004. doi: 10.1016/j.jhep.2015.06.030
102. Lapierre P, Beland K, Martin C, Alvarez F Jr, Alvarez F. Forkhead box p3+ regulatory T cell underlies male resistance to experimental type 2 autoimmune hepatitis. Hepatology. (2010) 51:1789–98. doi: 10.1002/hep.23536
103. Murphy G, Isenberg D. Effect of gender on clinical presentation in systemic lupus erythematosus. Rheumatology. (2013) 52:2108–15. doi: 10.1093/rheumatology/ket160
104. Danchenko N, Satia JA, Anthony MS. Epidemiology of systemic lupus erythematosus: a comparison of worldwide disease burden. Lupus. (2006) 15:308–18. doi: 10.1191/0961203306lu2305xx
105. Lahita RG, Bradlow HL, Ginzler E, Pang S, New M. Low plasma androgens in women with systemic lupus erythematosus. Arthritis Rheum. (1987) 30:241–8. doi: 10.1002/art.1780300301
106. Olsen NJ, Kovacs WJ. Case report: testosterone treatment of systemic lupus erythematosus in a patient with Klinefelter's syndrome. Am J Med Sci. (1995) 310:158–60. doi: 10.1097/00000441-199510000-00006
107. Sasaki N, Yamauchi K, Sato R, Masuda T, Sawai T, Inoue H. Klinefelter's syndrome associated with systemic lupus erythematosus and autoimmune hepatitis. Mod Rheumatol. (2006) 16:305–8. doi: 10.3109/s10165-006-0511-5
108. Gubbels Bupp MR, Jorgensen TN, Kotzin BL. Identification of candidate genes that influence sex hormone-dependent disease phenotypes in mouse lupus. Genes Immun. (2008) 9:47–56. doi: 10.1038/sj.gene.6364447
109. Fijak M, Schneider E, Klug J, Bhushan S, Hackstein H, Schuler G, et al. Testosterone replacement effectively inhibits the development of experimental autoimmune orchitis in rats: evidence for a direct role of testosterone on regulatory T cell expansion. J Immunol. (2011) 186:5162–72. doi: 10.4049/jimmunol.1001958
110. Wolfe AM, Kellgren JH, Masi AT. The epidemiology of rheumatoid arthritis: a review. II. Incidence and diagnostic criteria. Bull Rheum Dis. (1968) 19:524–9.
111. Spector TD, Ollier W, Perry LA, Silman AJ, Thompson PW, Edwards A. Free and serum testosterone levels in 276 males: a comparative study of rheumatoid arthritis, ankylosing spondylitis and healthy controls. Clin Rheumatol. (1989) 8:37–41. doi: 10.1007/BF02031066
112. Masi AT. Sex hormones and rheumatoid arthritis: cause or effect relationships in a complex pathophysiology? Clin Exp Rheumatol. (1995) 13:227–40.
113. Hall GM, Larbre JP, Spector TD, Perry LA, Da Silva JA. A randomized trial of testosterone therapy in males with rheumatoid arthritis. Br J Rheumatol. (1996) 35:568–73. doi: 10.1093/rheumatology/35.6.568
114. Keith RC, Sokolove J, Edelman BL, Lahey L, Redente EF, Holers VM, et al. Testosterone is protective in the sexually dimorphic development of arthritis and lung disease in SKG mice. Arthritis Rheum. (2013) 65:1487–93. doi: 10.1002/art.37943
115. Mavragani CP, Moutsopoulos HM. Sjogren syndrome. CMAJ. (2014) 186:E579–86. doi: 10.1503/cmaj.122037
116. Forsblad-d'Elia H, Carlsten H, Labrie F, Konttinen YT, Ohlsson C. Low serum levels of sex steroids are associated with disease characteristics in primary Sjogren's syndrome; supplementation with dehydroepiandrosterone restores the concentrations. J Clin Endocrinol Metab. (2009) 94:2044–51. doi: 10.1210/jc.2009-0106
117. Vendramini AC, Soo C, Sullivan DA. Testosterone-induced suppression of autoimmune disease in lacrimal tissue of a mouse model (NZB/NZW F1) of Sjogren's syndrome. Invest Ophthalmol Vis Sci. (1991) 32:3002–6.
118. Morthen MK, Tellefsen S, Richards SM, Lieberman SM, Rahimi Darabad R, Kam WR, et al. Testosterone influence on gene expression in lacrimal glands of mouse models of sjogren syndrome. Invest Ophthalmol Vis Sci. (2019) 60:2181–97. doi: 10.1167/iovs.19-26815
119. Abdulkarim M, Zenouzi R, Sebode M, Schulz L, Quaas A, Lohse AW, et al. Sex differences in clinical presentation and prognosis in patients with primary biliary cholangitis. Scand J Gastroenterol. (2019) 54:1391–6. doi: 10.1080/00365521.2019.1683226
120. Carbone M, Mells GF, Pells G, Dawwas MF, Newton JL, Heneghan MA, et al. Sex and age are determinants of the clinical phenotype of primary biliary cirrhosis and response to ursodeoxycholic acid. Gastroenterology. (2013) 144:560–9 e7. quiz e13–4. doi: 10.1053/j.gastro.2012.12.005
121. Lleo A, Jepsen P, Morenghi E, Carbone M, Moroni L, Battezzati PM, et al. Evolving trends in female to male incidence and male mortality of primary biliary cholangitis. Sci Rep. (2016) 6:25906. doi: 10.1038/srep25906
122. Lleo A, Marzorati S, Anaya JM, Gershwin ME. Primary biliary cholangitis: a comprehensive overview. Hepatol Int. (2017) 11:485–99. doi: 10.1007/s12072-017-9830-1
123. Rubel LR, Rabin L, Seeff LB, Licht H, Cuccherini BA. Does primary biliary cirrhosis in men differ from primary biliary cirrhosis in women? Hepatology. (1984) 4:671–7. doi: 10.1002/hep.1840040418
124. van Gerven NM, de Boer YS, Zwiers A, Verwer BJ, Drenth JP, van Hoek B, et al. HLA-DRB1*03:01 and HLA-DRB1*04:01 modify the presentation and outcome in autoimmune hepatitis type-1. Genes Immun. (2015) 16:247–52. doi: 10.1038/gene.2014.82
125. Heneghan MA, Yeoman AD, Verma S, Smith AD, Longhi MS. Autoimmune hepatitis. Lancet. (2013) 382:1433–44. doi: 10.1016/S0140-6736(12)62163-1
126. Bianchi I, Lleo A, Gershwin ME, Invernizzi P. The X chromosome and immune associated genes. J Autoimmun. (2012) 38:J187–92. doi: 10.1016/j.jaut.2011.11.012
127. Tukiainen T, Villani AC, Yen A, Rivas MA, Marshall JL, Satija R, et al. Landscape of X chromosome inactivation across human tissues. Nature. (2017) 550:244–8. doi: 10.1038/nature24265
128. Moulton VR. Sex hormones in acquired immunity and autoimmune disease. Front Immunol. (2018) 9:2279. doi: 10.3389/fimmu.2018.02279
129. Smith-Bouvier DL, Divekar AA, Sasidhar M, Du S, Tiwari-Woodruff SK, King JK, et al. A role for sex chromosome complement in the female bias in autoimmune disease. J Exp Med. (2008) 205:1099–108. doi: 10.1084/jem.20070850
130. Souyris M, Cenac C, Azar P, Daviaud D, Canivet A, Grunenwald S, et al. TLR7 escapes X chromosome inactivation in immune cells. Sci Immunol. (2018) 3:aap8855. doi: 10.1126/sciimmunol.aap8855
131. Invernizzi P, Miozzo M, Battezzati PM, Bianchi I, Grati FR, Simoni G, et al. Frequency of monosomy X in women with primary biliary cirrhosis. Lancet. (2004) 363:533–5. doi: 10.1016/S0140-6736(04)15541-4
132. Miozzo M, Selmi C, Gentilin B, Grati FR, Sirchia S, Oertelt S, et al. Preferential X chromosome loss but random inactivation characterize primary biliary cirrhosis. Hepatology. (2007) 46:456–62. doi: 10.1002/hep.21696
133. Invernizzi P, Miozzo M, Selmi C, Persani L, Battezzati PM, Zuin M, et al. X chromosome monosomy: a common mechanism for autoimmune diseases. J Immunol. (2005) 175:575–8. doi: 10.4049/jimmunol.175.1.575
134. Trivedi PJ, Kumagi T, Al-Harthy N, Coltescu C, Ward S, Cheung A, et al. Good maternal and fetal outcomes for pregnant women with primary biliary cirrhosis. Clin Gastroenterol Hepatol. (2014) 12:1179–85.e1. doi: 10.1016/j.cgh.2013.11.030
135. Schramm C, Herkel J, Beuers U, Kanzler S, Galle PR, Lohse AW. Pregnancy in autoimmune hepatitis: outcome and risk factors. Am J Gastroenterol. (2006) 101:556–60. doi: 10.1111/j.1572-0241.2006.00479.x
136. Westbrook RH, Yeoman AD, Kriese S, Heneghan MA. Outcomes of pregnancy in women with autoimmune hepatitis. J Autoimmun. (2012) 38:J239–44. doi: 10.1016/j.jaut.2011.12.002
137. Bremer L, Schramm C, Tiegs G. Immunology of hepatic diseases during pregnancy. Semin Immunopathol. (2016) 38:669–85. doi: 10.1007/s00281-016-0573-1
138. Bae HR, Leung PS, Tsuneyama K, Valencia JC, Hodge DL, Kim S, et al. Chronic expression of interferon-gamma leads to murine autoimmune cholangitis with a female predominance. Hepatology. (2016) 64:1189–201. doi: 10.1002/hep.28641
139. Bae HR, Hodge DL, Yang GX, Leung PSC, Chodisetti SB, Valencia JC, et al. The interplay of type I and type II interferons in murine autoimmune cholangitis as a basis for sex-biased autoimmunity. Hepatology. (2018) 67:1408–19. doi: 10.1002/hep.29524
140. van Nieuwerk CM, Groen AK, Ottenhoff R, van Wijland M, van den Bergh Weerman MA, Tytgat GN, et al. The role of bile salt composition in liver pathology of mdr2 (-/-) mice: differences between males and females. J Hepatol. (1997) 26:138–45. doi: 10.1016/S0168-8278(97)80020-7
141. Roubinian JR, Papoian R, Talal N. Androgenic hormones modulate autoantibody responses and improve survival in murine lupus. J Clin Invest. (1977) 59:1066–70. doi: 10.1172/JCI108729
142. Roubinian JR, Talal N, Greenspan JS, Goodman JR, Siiteri PK. Effect of castration and sex hormone treatment on survival, anti-nucleic acid antibodies, and glomerulonephritis in NZB/NZW F1 mice. J Exp Med. (1978) 147:1568–83. doi: 10.1084/jem.147.6.1568
143. Roubinian J, Talal N, Siiteri PK, Sadakian JA. Sex hormone modulation of autoimmunity in NZB/NZW mice. Arthritis Rheum. (1979) 22:1162–9. doi: 10.1002/art.1780221102
144. Michalski JP, McCombs CC, Roubinian JR, Talal N. Effect of androgen therapy on survival and suppressor cell activity in aged NZB/NZW F1 hybrid mice. Clin Exp Immunol. (1983) 52:229–33.
145. Walker SE, Keisler LW, Caldwell CW, Kier AB, vom Saal FS. Effects of altered prenatal hormonal environment on expression of autoimmune disease in NZB/NZW mice. Environ Health Perspect. (1996) 104(Suppl. 4):815–21. doi: 10.1289/ehp.96104s4815
146. Matejuk A, Hopke C, Vandenbark AA, Hurn PD, Offner H. Middle-age male mice have increased severity of experimental autoimmune encephalomyelitis and are unresponsive to testosterone therapy. J Immunol. (2005) 174:2387–95. doi: 10.4049/jimmunol.174.4.2387
147. Bao M, Yang Y, Jun HS, Yoon JW. Molecular mechanisms for gender differences in susceptibility to T cell-mediated autoimmune diabetes in nonobese diabetic mice. J Immunol. (2002) 168:5369–75. doi: 10.4049/jimmunol.168.10.5369
148. Lapierre P, Djilali-Saiah I, Vitozzi S, Alvarez F. A murine model of type 2 autoimmune hepatitis: xenoimmunization with human antigens. Hepatology. (2004) 39:1066–74. doi: 10.1002/hep.20109
149. Rojas M, Restrepo-Jimenez P, Monsalve DM, Pacheco Y, Acosta-Ampudia Y, Ramirez-Santana C, et al. Molecular mimicry and autoimmunity. J Autoimmun. (2018) 95:100–23. doi: 10.1016/j.jaut.2018.10.012
150. Lapierre P, Beland K, Yang R, Alvarez F. Adoptive transfer of ex vivo expanded regulatory T cells in an autoimmune hepatitis murine model restores peripheral tolerance. Hepatology. (2013) 57:217–27. doi: 10.1002/hep.26023
151. Landi A, Weismuller TJ, Lankisch TO, Santer DM, Tyrrell DL, Manns MP, et al. Differential serum levels of eosinophilic eotaxins in primary sclerosing cholangitis, primary biliary cirrhosis, and autoimmune hepatitis. J Interferon Cytokine Res. (2014) 34:204–14. doi: 10.1089/jir.2013.0075
152. Lleo A, Zhang W, Zhao M, Tan Y, Bernuzzi F, Zhu B, et al. DNA methylation profiling of the X chromosome reveals an aberrant demethylation on CXCR3 promoter in primary biliary cirrhosis. Clin Epigenetics. (2015) 7:61. doi: 10.1186/s13148-015-0098-9
153. Tomassini V, Onesti E, Mainero C, Giugni E, Paolillo A, Salvetti M, et al. Sex hormones modulate brain damage in multiple sclerosis: MRI evidence. J Neurol Neurosurg Psychiatry. (2005) 76:272–5. doi: 10.1136/jnnp.2003.033324
154. Kurth F, Luders E, Sicotte NL, Gaser C, Giesser BS, Swerdloff RS, et al. Neuroprotective effects of testosterone treatment in men with multiple sclerosis. Neuroimage Clin. (2014) 4:454–60. doi: 10.1016/j.nicl.2014.03.001
155. Ramirez Sepulveda JI, Bolin K, Mofors J, Leonard D, Svenungsson E, Jonsen A, et al. Sex differences in clinical presentation of systemic lupus erythematosus. Biol Sex Differ. (2019) 10:60. doi: 10.1186/s13293-019-0274-2
156. Webb K, Peckham H, Radziszewska A, Menon M, Oliveri P, Simpson F, et al. Sex and pubertal differences in the Type 1 interferon pathway associate with both X chromosome number and serum sex hormone concentration. Front Immunol. (2018) 9:3167. doi: 10.3389/fimmu.2018.03167
157. Markle JG, Frank DN, Mortin-Toth S, Robertson CE, Feazel LM, Rolle-Kampczyk U, et al. Sex differences in the gut microbiome drive hormone-dependent regulation of autoimmunity. Science. (2013) 339:1084–8. doi: 10.1126/science.1233521
158. Chen W, Wei Y, Xiong A, Li Y, Guan H, Wang Q, et al. Comprehensive analysis of serum and fecal bile acid profiles and interaction with gut microbiota in primary biliary cholangitis. Clin Rev Allergy Immunol. (2020) 58:25–38. doi: 10.1007/s12016-019-08731-2
Keywords: testosterone, androgen, immunity, autoimmunity, androgen receptor, sex-bias, T cell, sex hormones
Citation: Henze L, Schwinge D and Schramm C (2020) The Effects of Androgens on T Cells: Clues to Female Predominance in Autoimmune Liver Diseases? Front. Immunol. 11:1567. doi: 10.3389/fimmu.2020.01567
Received: 13 March 2020; Accepted: 15 June 2020;
Published: 29 July 2020.
Edited by:
Susan Kovats, Oklahoma Medical Research Foundation, United StatesReviewed by:
Erin E. McClelland, Independent Researcher, Murfreesboro, United StatesSally A. Huber, University of Vermont, United States
Monika Fijak, Justus-Liebig-University Giessen, Germany
Copyright © 2020 Henze, Schwinge and Schramm. This is an open-access article distributed under the terms of the Creative Commons Attribution License (CC BY). The use, distribution or reproduction in other forums is permitted, provided the original author(s) and the copyright owner(s) are credited and that the original publication in this journal is cited, in accordance with accepted academic practice. No use, distribution or reproduction is permitted which does not comply with these terms.
*Correspondence: Dorothee Schwinge, ZC5zY2h3aW5nZUB1a2UuZGU=; Christoph Schramm, Y3NjaHJhbW1AdWtlLmRl