- 1Food Safety and Enteric Pathogens Research Unit, National Animal Disease Center, Agricultural Research Services, USDA, Ames, IA, United States
- 2Department of Veterinary Microbiology and Preventive Medicine, Iowa State University, Ames, IA, United States
Antimicrobial resistance (AMR) is a significant problem in health care, animal health, and food safety. To limit AMR, there is a need for alternatives to antibiotics to enhance disease resistance and support judicious antibiotic usage in animals and humans. Immunomodulation is a promising strategy to enhance disease resistance without antibiotics in food animals. One rapidly evolving field of immunomodulation is innate memory in which innate immune cells undergo epigenetic changes of chromatin remodeling and metabolic reprogramming upon a priming event that results in either enhanced or suppressed responsiveness to secondary stimuli (training or tolerance, respectively). Exposure to live agents such as bacille Calmette-Guerin (BCG) or microbe-derived products such as LPS or yeast cell wall ß-glucans can reprogram or “train” the innate immune system. Over the last decade, significant advancements increased our understanding of innate training in humans and rodent models, and strategies are being developed to specifically target or regulate innate memory. In veterinary species, the concept of enhancing the innate immune system is not new; however, there are few available studies which have purposefully investigated innate training as it has been defined in human literature. The development of targeted approaches to engage innate training in food animals, with the practical goal of enhancing the capacity to limit disease without the use of antibiotics, is an area which deserves attention. In this review, we provide an overview of innate immunomodulation and memory, and the mechanisms which regulate this long-term functional reprogramming in other animals (e.g., humans, rodents). We focus on studies describing innate training, or similar phenomenon (often referred to as heterologous or non-specific protection), in cattle, sheep, goats, swine, poultry, and fish species; and discuss the potential benefits and shortcomings of engaging innate training for enhancing disease resistance.
Innate Modulation
While various approaches are used to limit disease and antibiotic usage in agricultural animals, efficacious intervention strategies remain unavailable for many diseases. Immunomodulation is one approach to engage or prime (1) the host's own immune system to defend against infectious disease. Vaccines are effective immunomodulators, priming the adaptive immune system, and well-understood by infectious disease experts. However, less common or at least less frequently discussed is immunomodulation of the innate arm of the immune system for enhanced disease protection. A related, rapidly evolving field of immunomodulation is innate training, which relies on memory of the innate immune system (see Text Box).
Box 1. Defining immunomodulation.
Immunomodulation
Changes to the immune system after exposure to a substance or compound (i.e., agonist) that stimulates or suppresses the immune response. This review is focused on immunomodulation that alters the immune response to subsequent exposure with non-related (heterologous) immune agonist, not the priming agonist.
Immunomodulation of adaptive immunity: altered vaccination or natural exposure of an animal to pathogens or other foreign agents induces the generation of effector and memory T- and B-cells to provide long-term (multi-year to lifetime) protection against the foreign agent.
Exposure of an animal to a priming substance or compound, often a protein or protein-polysaccharide, such that subsequent exposure to similar compound results in cross-reactive response. dependent on B- and T-cells. anergy and tolerance are functions of non-repsonsiveness or suppressive responses by B- or T-cells
Immunomodulation of innate immunity (innate memory): exposure of an animal or cells to a priming substance or compound (i.e, agonist), often a microbial-associated molecular pattern (MAMP), such that exposure to non-related immune agonist results in heightened (trained) or suppressed (tolerant) response. Observed in non-T, non-B cells, and primarily in myeloid lineage and NK cells. Mechanism includes epigenetic and metabolic reprograming of innate immune cells and progenitor cells. Duration of effect is not yet determined, but there is evidence for months to a few years.
Training: enhanced response to heterologous agonists
Tolerance: decreased response to heterologous agonists
While adaptive immune memory is well-understood at the cellular and molecular level, the innate system was not known to have memory, and concordantly disease prevention strategies primarily targeted the adaptive immune system (e.g., vaccination). However, the paradigm on innate memory has recently shifted, with substantial evidence indicating that innate immune cells functionally adapt after stimulation or microbial exposure. More specifically, circulating monocytes, monocyte-derived macrophages, and NK cells have altered secondary responses to various pathogens or microbe-associated molecular patterns (MAMPs) after an initial priming event with the same or different MAMP (1–4). In vitro, purified monocytes stimulated with specific innate agonists, such as β-glucan or live-attenuated tuberculosis vaccine [Mycobacterium bovis bacillus Calmette-Guerin (BCG)], and restimulated days later with a heterologous MAMP, had heightened responses compared to cells that were not primed with β-glucan or BCG [reviewed in (3)]. Thus, the adaptive immune system may not be the only consideration for development of disease intervention strategies for enhancing food animal health.
The sustained effect of trained innate immunity is dependent on epigenetic changes, chromatin remodeling, and basal metabolic shifts that occur in the cell after primary stimulation, with effects that can be long-lasting. Primary MAMP (5, 6) exposure leaves the cell in a “poised” state, or a state in which the cell is ready to respond to secondary insult or exposure. In a trained response, cells respond with increased production of effector molecules, including proinflammatory cytokines, upon secondary stimulation or exposure (Figure 1). The trained response differs from innate tolerance, in which poised cells respond with reduced production of effector molecules (Figure 1). While circulating immune cells such as monocytes and NK cells may exhibit a trained response, the lifespan of circulating cells is relatively short-lived (7) and the length of time cells exhibit a trained phenotype may concordantly be short-lived. However, epigenetic modification of bone marrow progenitor cells that become circulating effector cells (including monocytes and NK cells), underlies innate training in vivo and contributes to the longevity of innate training (8, 9). Experimental vaccination of humans with BCG enhances in vitro PBMC pro-inflammatory cytokine production upon stimulation with the MAMP lipopolysaccharide (LPS), even 12 months after BCG administration (1). Furthermore, epidemiological studies with human infants found that BCG vaccination is associated with non-specific (i.e., not related to BCG) protection. In other words, BCG vaccinated children had enhanced resistance to other diseases (5, 6), leading to increased overall survival and decreased incidences of morbidity. Importantly, the protective benefits were noted months to years after vaccination (10). Food animals are relatively short lived, and changes to innate immunity in early life may afford a protective effect against disease for the animals' entire lifespan.
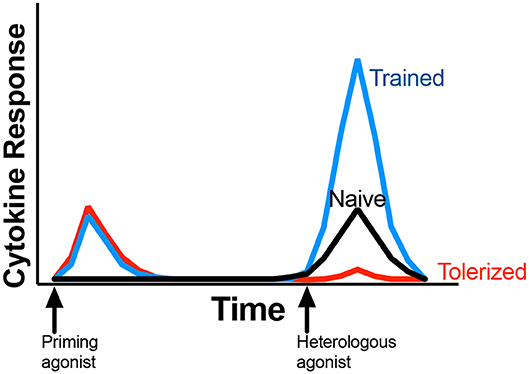
Figure 1. General outline of immune response associated with innate memory. Initial exposure to a priming agonist (red and blue lines) induces an innate response, typically measured as induction of proinflammatory cytokine production. After a rest period, subsequent stimulation of the same cells or animal with a heterologous agonist (commonly LPS or Pam3CSK4) results in tolerance or trained response, marked by reduced (red line) or enhanced (blue line) cytokine production when compared to naïve response (black line). Proinflammatory cytokines, including IL-1β, TNF-α, or IL-6 are the most common cytokines measured for innate memory. Priming and heterologous stimulation may all occur in vitro with primary cells; in vivo; or a combinantion of in vivo with ex vivo cell stimulation.
In this paper, we briefly review important concepts from mouse, rabbit, and human literature, including mechanisms of innate memory, training, and tolerizing agents, and evidence for innate training in various cells types. However, the primary focus of this review is to summarize the available evidence for innate immunomodulation and memory in agricultural species including cattle, pigs, poultry, fish, and small ruminants.
Review of Human and Animal Model Literature
Immunomodulator Molecules
Bacterial endotoxin, or LPS, is the earliest described and best-known agonists capable of modulating the innate immune system (11). Priming of innate cells, most notably monocytes, with low doses of LPS, followed by homologous LPS restimulation resulted in a depressed inflammatory immune response (i.e., tolerance) (12, 13). However, Gregory Shwartzman described a phenomenon whereby rabbits intradermally injected with super low doses of gram-negative sterile culture filtrate had dermal necrosis at the site of injection following intravenous rechallenge with the same filtrate (14), suggesting the cells primed at the initial injection site responded to the secondary stimulation with a heightened response. The discovery of the “Shwartzman phenomenon” was followed by multiple studies highlighting the importance of dose, as low doses of LPS induced tolerance while super low doses resulted in heightened immune responses (15–18). Compounds besides LPS can induce a tolerant state in myeloid cells when restimulated with the same molecule (homologous tolerance). Additionally, priming with one agonist could induce tolerance in response to restimulation with a heterologous agonist, a process termed cross-tolerance (13, 19, 20). Zymosan, a particulate preparation of β-glucans, mannans, and proteins from Saccharomyces cerevisiae was one of the earliest known inducers of cross-tolerance (21, 22). Collectively, various molecules have been implicated in tolerance, and the dose upon primary exposure impacts the induction of tolerance.
As LPS is known as a classic tolerizing agent, the BCG vaccine is a highly described inducer of innate training. There are numerous reports on the non-specific effects of BCG vaccination of infants in countries that actively administer neonatal BCG, with significant reductions in non-tuberculosis diseases (5, 6, 23). In a set of particularly compelling studies, BCG vaccination of low-birth weight infants in Guinea-Bissau was associated with a nearly 50% reduction in mortality rates, primarily due to reductions in sepsis and respiratory infections (5, 6). Monocytes and NK cells from BCG-vaccinated adults and infants, compared to non-vaccinated cohorts, display increased expression of toll-like receptors and increased cytokine production in response to various pathogens and their products (e.g., M. tuberculosis, Candida albicans, Staphylcoccus aureus, LPS, and Pam3CSK4) (17–19). Mice vaccinated with BCG are protected from lethal C. albicans challenge via a mechanism that requires macrophages (24) and humans vaccinated with BCG show reduced viral titer when experimentally infected with the attenuated yellow fever virus vaccine strain (25). However, BCG-induced trained immunity did not protect mice against experimental influenza A infection (26). Early studies with Mycobacterium tuberculosis or BCG indicate enhanced resistance against subsequent disease may not be a universal phenomomen. While protection in mice against Bacillus anthracis, Brucella suis, Staphylococcus aureus, Pasteurella pestis, Listeria monocytogenes, and Klebsiella pneumonia has been noted (27–30), BCG treated mice are more sensitive to endotoxin and had similar mortality to untreated mice when challenged intravenously with a low dose of Salmonella enteritis (1 × 104 CFU) (31). While not all encompassing, numerous reports indicate BCG administration in humans or rodent animals models enhances resistance to subsequent disease with increased immune responses to the secondary agent, a hallmark of innate training.
β-glucans, which activate innate cells via the Dectin-1 receptor or CR3, can also induce innate training. However, the source and type of β-glucan impacts the primary immune response and subsequent induction of innate memory. Human myeloid-lineage cells require cross-linkage of the Dectin-1 receptor and formation of a phagocytic synapse for downstream signaling (32). Soluble β-glucans, such as laminarin with (1–3)(1–6) linkages, can bind to the Dectin-1 receptor but are incapable of cross-linking multiple receptors and thereby fail to initiate an immune response (32, 33). As discussed above, zymosan induces tolerance in monocytes. It is a complex compound of β-glucan with highly branched (1–3)(1–6) linkages that, along with mannan and proteins, forms the cell wall. However, human and mouse monocytes primed with β-glucan from C. albicans exhibited a trained phenotype upon heterologous restimulation (17, 34–36). In fact, heat-inactivated C. albicans alone is sufficient to induce a trained state in human monocytes (34). β-glucans primarily induce innate training, but there are instances in which tolerance is induced, though the reason may not be related to dose, but receptor binding or signaling by additional cell wall components contained within the product.
There are many documented and perceived benefits associated with innate memory, but some potential drawbacks. While β-glucans and BCG are the most studied innate priming agonists other MAMP molecules, such as flagellin, muramyl dipeptide (MDP), polyinosinic-polycytidylic [Poly(I:C)] can induce innate training (1, 17). Innate training is the proposed mechanism for the non-specific benefits observed with certain vaccines, including the yellow fever vaccine, measles vaccine, vaccinia, and the influenza vaccine (37, 38). However, a heightened immune response to subsequent infections may result in enhanced pathology, an undesired effect. Indeed, trained immunity is hypothesized to contribute to autoimmune diseases (39, 40) and in a controlled experiment, patients vaccinated with BCG and later infected with malaria experienced earlier and more clinically severe symptoms than those not vaccinated with BCG (41). Thus, a deeper understanding of the implications associated with harnessing innate memory are warranted.
Mechanisms and Cells in Trained Immunity
Trained immunity is based on epigenetic reprogramming in innate immune cells, which has been documented primarily in monocytes (35, 42). The epigenetic modifications lead to changes in gene expression and consequent protein production upon secondary stimulation. Chromatin modifications and changes in DNA accessibility are the central processes of epigenetic reprogramming associated with trained immunity. Histone modifications, such as increased methylation of the latent enhancer histone H3 at K4 (H3K4me1), reduced methylation of the repressor histone marks such as histone H3 at K9 (H3K9me3) and the most informative histone marker, increased acetylation at the poised/active enhancer mark (H3K27ac), are associated with a trained phenotype (1, 2, 35, 36). Other posttranscriptional regulatory mechanisms, such as microRNA (miRNA) modulation of mRNA levels, are involved in the regulation of the immune response (43). MicroRNA genes, as well as protein coding genes, can be regulated by histone modifications, and at the same time, miRNAs can directly and indirectly target effectors of the epigenetic machinery (44) and immune system mRNAs.
Changes to metabolic state are also noted in trained cells, and likely the result of epigenetic reprogramming whereby cells are primed to respond to secondary stimulation. Metabolic state is important for rapid release of intermediate substrates, such as nucleic and amino acids, necessary for the production of effector molecules (45). Innate training by C. albicans β-glucan is evidenced by an increase in basal glycolysis and a decrease in basal mitochondrial respiration, a measure of oxidative phosphorylation (Warburg effect) (46). The importance of glycolysis in β-glucan mediated innate training was noted in a clinical trial wherein β-glucan injection was administered to human volunteers, with a cohort also receiving metformin (a drug that prevents gluconeogenesis). Individuals on metformin did not exhibit enhanced ex vivo cytokine production following heterologous restimulation, which was in contrast to volunteers who received the β-glucan injection without prior metformin treatment (47). Thus, the availability and capacity to utilize glucose is critical in trained innate cells.
In addition to evidence of metabolic state impacting β-glucan induced training, BCG impacts cellular metabolism. BCG-treated cells have an increase in basal glycolysis as well as oxidative phosphorylation (48). Activation of the metabolic Akt/mTOR/HIF1 pathway is a critical feature of BCG-mediated trained immunity. Inhibition of glutamine or mTOR/glycolysis metabolism during in vitro training with BCG inhibited mRNA expression, and also prevented the epigenetic changes (H3K4me3 and H3K9me3) normally associated with BCG trained immunity (48). Collectively, the epigenetic and metabolic changes associated with BCG-induced training, or lack thereof, are linked. Understanding the mechanisms associated with training can provide a more targeted approach to modulate immune status.
Innate training and tolerance are well-described in monocytes and monocyte-derived macrophages, and to a lesser extent in NK cells (19, 34, 46, 49, 50). In vitro studies with purified monocytes and in vivo studies with severe combined immunodeficient (SCID) mice indicate T and B cells are not required for development of trained immunity (1). Epigenetic reprograming of myeloid progenitor cells leads to long lasting changes to emigrating monocytes, contributing to the longevity of innate training (8, 9). Tissue resident macrophages (e.g., Kupffer cells in the liver) are terminally-differentiated and do not rely on circulating monocytes for regeneration (51). It is unclear if tissue-resident macrophages can be trained, or if presence of trained myeloid lineage cells in tissue is the result of circulating monocytes migrating into a tissue. A study identifying alveolar macrophages with a trained phenotype showed CD8 T cells were required for induction of a trained state following a viral infection (52). In another study, reprogramming of tissue-resident macrophages occurred upon placement in new microenvironments, and cells may be driven into a trained or tolerant phenotype (53). Innate training is noted in other cell types, including dendritic cells (54, 55), non-immune cells such as mesenchymal and epithelial stem cells, and intestinal stromal cells (56). Additional research will be required to understand how alterations in the innate responsiveness of various cell types may contribute to disease resistance at the level of the organism.
Innate Memory in Food Animals
Innate memory, defined as both training and tolerance, is well-described in human and rodent literature, and a mechanistic model of innate training as described above is beginning to be defined. However, there exists a paucity of information regarding innate memory in agriculture animals. Although broadly similar to human and rodent immune systems, there are important species-specific differences in the innate immune systems of individual food animals which can significantly impact the development of innate memory. For example, LPS dose plays a critical role in the induction of tolerance or training. However, it is difficult to draw parallels across species because of differences in LPS sensitivities. A very low dose of LPS can induce cellular and physiological changes in sheep, while a much higher dose is needed for a similar effect in chickens (57, 58). Thus, in the future, it will be critically important to assess the induction and effects of innate training in each individual species, ensuring that species-specific differences in innate immune function are fully acknowledged. As an impetus to encourage futher research, this review is focused on evidence for innate training in individual commercially important agricultural species and the potentiall benefits and limitations of innate training to enhance disease resistance.
Cattle
To date, there are only a handful of reports detailing innate training as described by Netea et al. (3) in cattle. In one report, vaccination of 3- to 6- months old beef calves with heat-killed M. bovis resulted in an enhanced capacity for monocyte-derived macrophages from these animals to phagocytose and kill M. bovis in vitro. This effect was independent of cellular or humoral adaptive immune responses, and lasted up to 6 months after vaccination (59). Recent data from our group has shown that aerosol BCG vaccination induces a trained phenotype in circulating bovine monocytes. Specifically, monocytes isolated from BCG-vaccinated calves produce more proinflammatory cytokines in response to stimulation with LPS or Pam3CSK4 compared to cells from non-vaccinated, control calves (60). Thus, it is clear that the bovine innate immune system can be trained in a similar fashion as that of humans and rodents. Further analysis of the literature suggests other instances in which innate training may occur in cattle, although without experiments designed to specifically address the duration or mechanisms of innate memory, one must infer based upon the nature of the stimuli or resulting phenotype. In one report, immunization of cattle with an ultrasonicated lysate of Corynebacterium cutis had positive effects on morbidity and mortality in three different age groups of animals (61). Ten days old calves receiving the C. cutis lysate demonstrated a nearly 50% reduction in morbidity compared to controls due to enteric and respiratory diseases in the first 6 months of life. When pregnant cows were given C. cutis lysate in the final month of pregnancy, the resultant calves had a higher birth weight and greater weight gain in the first 3 months of life. Of the 23 control calves that were born, only 15 calves survived to 3 months of age, while 25 of 25 calves from the C. cutis immunized dams survived to the study endpoint (61). In a number of early studies, oral vaccination of calves using attenuated, live auxotrophic mutants of Salmonella enteritidis serovar Typhimirium (S. Typhimurium) resulted in homologous and heterologous protection against S. Typhimurium and S. Dublin (62–64). Protection was non-specific and T cell-independent, and endured for about 1 month after vaccination. Similar results were subsequently recapitulated in mouse models (65); and in fact, more recent results have shown that oral vaccination with live, attenuated S. Typhimurium induces sufficient non-specific protection to prevent lethal influenza virus infection in a mouse model (66). Thus, while the authors did not investigate the mechanisms of non-specific resistance in the calves, we speculate that the live Salmonella vaccine may have induced some form of innate memory.
Several recent commercial therapies have emerged with potential to enhance the bovine innate immune response during times of stress. One such DNA-based immunostimulant, marketed as the commercial product Zelnate™, can reduce lung-pathology scores in cattle experimentally challenged with M. haemolytica (67), and significantly reduce mortality in high-risk cattle after feedlot placement (68, 69). While the product's exact mechanism(s) of action is not well-defined, it is likely stimulating the immune system through pattern-recognition receptors such as TLR9 or the innate cytosolic DNA sensing c-GAS-STING pathway (70). It is unclear, however, if Zelnate™'s mechanism of action can be classified as innate memory. Product literature encourages the use of Zelnate immediately prior or within 24 h of a perceived stressful event. Given that a critical aspect of innate training is the duration of the effect, this form of immunomodulation may not fit the definition. Another immunomodulatory product, marketed as Amplimune™, is a mycobacterial cell wall fraction derived from the non-pathogenic Mycobacterium phlei. Amplimune™ non-specifically activates the innate immune system and can significantly reduce the incidence and severity of K99 Escherichia coli infection in newborn calves (71). It is currently marketed in the United States and Canada for this use. A recent study revealed that Amplimune™ also had significant beneficial effects in reducing the incidence and mortality associated with bovine respiratory disease in newly received, light-weight beef calves (72), suggesting it may have broader applications for ruminant health. Another commercial immunomodulator, Baypamun™, an inactivated preparation of Orf virus (Parapoxvirus ovis), was sold in Europe for several years for use in food animals and horses. Treatment with Baypamun™ immediately prior to, or in the early stages of infectious bovine rhinotracheitis infection was shown to significantly reduce clinical disease and virus shedding (73–76). Again, while it is evident that these commercial products have enhancing effects on the innate immune system, it is unclear if the immune system remains in a poised state for prolonged periods following treatment. More research will be required to determine if these products have the capacity to induce the long-term effects of innate memory, or simply a transient increase in innate activation.
The use of immunomodulatory feed compounds has grown with the increasing interest in alternatives to antibiotics. Many of these compounds are comprised of a mixture of whole yeast or yeast cell wall components. Several can promote innate immune functions, such as increasing phagocytic activity, increasing the generation of reactive oxygen species or restoring proinflammatory cytokine secretion to leukocytes from transition cows (77–82). The use of particular immunomodulatory feed additives can increase disease resistance in bovine. For example, supplementing with a S. cerevisiae fermentation product improves outcome of experimental Salmonella or Cryptosporidium challenges in preweaned calves (83–85); and reduces the size and number of liver abscesses in finishing beef steers, with efficacy comparable to standard in-feed antibiotic regimens (86). Yeast-supplemented cattle have reduced incidences of bovine respiratory disease during the receiving period (87, 88); while preweaned dairy calves receiving a yeast-based supplement have improved fecal scores and overall reductions in morbidity and mortality during the first 70 days of life. Given the capacity of the yeast cell wall component, β-glucan, to train the innate immune system in rodents and humans (34, 35, 46), it seems likely that at least some of the positive effects of such yeast-based additives on bovine health may be attributed to the induction of innate memory. More in-depth analyses of innate cell function and the specific epigenetic and metabolic alterations accompanying these changes will be required to determine if innate memory is a mechanism contributing to enhanced disease resistance.
Sheep and Goats
Similar to the other species in this review, β-glucans are the most common immunomodulatory compounds investigated in sheep. Oral supplementation of β-(1-3)(1-6)-glucans to ewes has positive effects on reproductive performance, and on growth rate and body composition of the resultant lambs (89), potentially due to the positive effects of β-glucan supplementation on milk yield and milk composition in lactating ewes (89, 90). Monocytes and neutrophils isolated from lambs fed β-glucans have increased phagocytic and respiratory burst activities, and increased lysozyme activity (90, 91). Lactating ewes fed β-glucans have reduced somatic cell counts in milk (89), while an intramammary infusion of β-glucans resulted in selective recruitment of CD14+ monocytes/macrophages to the udder (92), potentially priming the animal to be more resistant to mastitis.
A recent series of studies has shown that the marine yeast, Debaryomyces hansenii and its cell wall, has the potential to train the innate immune system in newborn goats (93–96). In vivo supplementation of newborn goats with live D. hansenii induced upregulation of the genes encoding for TLR2, 4, and 6, IL-1β and TNF-α in circulating leukocytes, and resulted in increased respiratory burst, catalase and superoxide dismutase activity (94–96). The cell wall of D. hansenii is comprised primarily of (1-6)-branched (1-3)-β-D-glucan (96). In vitro training of goat monocytes with purified D. hansenii β-glucans results in increased expression of CD11b and the macrophage-associated gene F4/80, increased viability upon LPS challenge and increased phagocytic activity (93). In vivo, newborn kid goats supplemented with purified β-glucans from D. hansenii and subsequently challenged with LPS demonstrate increased plasma concentrations of IL-6, IL-1β, and TNF-α, and isolated leukocytes show increased respiratory burst activity and nitric oxide production (93).
BCG has not been widely studied in sheep. However, a few early studies showed that vaccination with BCG affords some resistance to infection with rift valley fever virus (97) and resistance to caseous lymphadenitis caused by Corynebacterium pseudotuberculosis (98). In the former study, a fraction of the BCG immunized sheep developed short fevers and viremia for only 24–48 h, while control sheep were viremic and febrile for up to 8 days after challenge. Sheep receiving two doses of BCG were completely protected from liver involvement due to rift valley fever infection (97). The latter study followed more than 500 head of sheep and used a model of natural C. pseudotuberculosis infection by seeding the herd with clinically infected animals. Over a period of 4 years, 99% of the lambs vaccinated with BCG were protected from development of caseious lymphadenitis (98). Thus, it appears that the innate immune systems of sheep and goats have the capacity to be trained, and the strategy holds significant potential for promoting disease resistance in small ruminants.
Swine
β-glucans are readily used in pig production systems across the world with noted health benefits [reviewed in (99)]. Commerical in-feed products may be formulated with purified β-glucan from various sources (yeast, algae, fungi), or contain live yeast, yeast with fermentation products, or a semi-purified mix of cell-wall polysaccharides (mannans). Each product includes some amount of β-glucan, either purified or in the cell wall, which is presumably the ingredient responsible for noted changes in health status. Oral supplementation with β-glucans can improve weight gain, though not every trial indicates improved performance (100–103). Oral β-glucan can improve performance when low levels of aflatoxin are also present (104). Post-weaning diarrhea in pigs is caused by enterotoxigenic E. coli (ETEC), and antibiotics are commonly administered to limit ETEC. Oral β-glucan supplementation for the 2 weeks post-weaning decreased susceptibility to ETEC (105). Addition of yeast fermentation product to the diet decreases ETEC attachment to mucosa (106). However, frequency of diarrhea after ETEC challenge increases with a yeast-whole cell supplemented diet, although E. coli levels in feces do not increase (107). Interestingly, dietary β-glucan improves piglet health after rotavirus infection (108). However, inclusion of β-glucan in the diet increases susceptibility to intravenous Streptococcus suis challenge, even with increased performance measures (100). The authors hypothesize dietary β-glucan increases expression of IL-1R antagonist, which may enhance feed uptake by blocking IL-1R signaling, but enhances susceptibility to disease due to lack of necessary IL-1R signaling. Following LPS injection, pigs on a β-glucan diet have less TNF-α and IL-6 in the plasma (103), suggesting reduced responsiveness upon secondary stimulation. While β-glucan and related products are used in pig production systems, the mechanisms of improved health are not completely understood and various factors, including microbiota, age, and pathogen insult may impact outcomes.
Though β-glucan is most commonly administered by the oral route, changes in peripheral, as opposed to intestinal, immune status is often assessed. Multiple cell types have receptors for β-glucans in pigs, including myeloid progenitor cells in the bone marrow (22, 109, 110); and in mice, intravenous injected fluorescent labeled β-glucan is located in bone marrow macrophages and neutrophils (111). Thus, β-glucans may translocate from the intestine into the periphery to modulate progenitor cells, with peripheral impact. As noted above, levels of proflammatory cytokines in the sera are lower in response to LPS injection when pigs are fed a β-glucan supplemented diet (102, 103). Inclusion of whole yeast cells in the diet leads to shifts in circulating leukocyte populations (107, 112), though the impact of changes on disease resistance are unclear. Peripheral blood mononuclear cells produce less proinflammatory cytokine following LPS stimulation if pig diet is supplemented with β-glucan (103). While Dectin-1 and CR3 are expressed by different pig leukocytes (109), and intestinal dendritic cells may be the first to encounter dietary β-glucan (113), it's unclear how dietary supplementation alters responsiveness of peripheral immune cells to heterologous stimulation. Overall, dietary products containining yeast and/or β-glucan can modulate peripheral immune responses, but the longevity of the shifts and translation to innate memory warrant further investigation.
While BCG is a classically defined training agonist across multiple species, there are few reports on the impact of BCG administration on pig innate immunity. Coe et al. report BCG administration in pigs did not alter neutrophil function (114). In a recent study, pigs administered inactivated Mycobacterium paratuberculosis vaccine had enhanced pathology and inflammatory responses following Actinobacillus pleuropneumoniae challenge (115), suggesting a heightened secondary response indicative of innate training. BCG readily interacts with cells of the innate immune system; however, in pigs it's unclear if changes result in protection against heterologous infections. Pigs are a proposed model for tuberculosis research (116) and BCG vaccination in wild boar to limit M. bovis infection is proposed (117, 118). Given the conserved aspects of innate immunity across species, BCG is anticipated to induce innate memory in pigs, but has yet to be adequately demonstrated.
Poultry
Of the food animals, commercial meat poultry (including chickens, turkeys, ducks, and quail) have some of the shortest times to market, with broiler chickens reaching market weights at an average of 47 days post hatch (119). While poultry breeds raised for egg production are longer-lived (1–2 years) (120), they have early life disease challenges and adaptive immune system limitations similar to meat poultry. Disease prevention via the adaptive immune system is controlled through vaccination against specific organisms and reaches full potential at around 3 weeks after vaccination (almost half a commercial broilers life span) (119, 121). Durning that 3 weeks span, maternal antibodies provide additional protection to the chick, but are dependent on multiple factors such as individual antibody titer levels and time post vaccination (122). Vaccination is relatively expensive with each vaccine providing protection against few pathogens. Contrasted to the adaptive immune response, the fast induction and breadth of innate memory presents a prime mechanism to reduce disease and foodborne organisms in poultry.
β-glucans are the most well-studied of the known innate memory immunostimulants in poultry (123, 124). However, due to the practical limitation of inoculating thousands of birds with β-glucans, stimulation with β-glucan occurs almost exclusively through the oral route via feed supplementation (125–129). Yeast are the most common form of dietary β-glucan as cereal β-glucans with (1–4)(1–6) linkages (i.e., barley and oat) are detrimental to poultry production due to reduced nutrient digestion and adsorption (130). In chicks, dietary supplementation with yeast β-glucans reduces Salmonella colonization of the cecum (131) and visceral organs (131, 132). Intermittent feeding of a β-glucan containing yeast product decreased the effects of transportation stress in turkey poults and tended to decrease colonization of the ceca with the foodborne pathogens, Salmonella and Campylobacter (129, 133). Interestingly, the same positive effect was not observed with continuous feeding of the yeast β-glucan product (129). No benefit of a β-glucan diet was observed when broiler chicks were challenged with Eimeria oocysts (128). While no studies in poultry have directly addressed the ability of β-glucans or other immunostimulants to induce trained immunity, β-glucan can alter the chicken immune system both in vitro and in vivo. Nitric oxide and IL-1, but not IL-6, production was increased in a chicken macrophage cell line following β-(1-3)(1-6)-glucan stimulation (134). The same study also detected increases in ex vivo macrophage phagocytic activity. Heterophil leukocyte function (phagocytosis, bactericidal killing, oxidative burst) is altered in yeast and β-glucan fed broiler chicks and turkey poults (125, 132, 133).
The production benefits (body weight, feed:gain ratios, feed consumption) of dietary β-glucans in poultry are less clear, as β-glucans and yeast products can enhance, reduce, or not change production parameters in chickens, turkey, or ducks (125, 127, 135). It is unclear if the conflicting results are due to different products (whole yeast, mannan oligosaccharides, purified β-glucan, etc.), source of yeast product (Saccharomyces cerevisiae, Aureobasidium pullulan, or other), relative dose of β-glucan, age of the animals, or some other factor. Huff et al. (135) suggest a potential mechanism for differences in production parameters, as they found in absence of E. coli challenge, chicks on control diets had higher body weights and feed:gain ratios than β-glucan fed chicks, but with challenge, the β-glucan chicks had higher production parameters. Dietary β-glucan is also associated with enhanced intestinal barrier functions (increased villus height/crypt depth ratio, number of goblet cells, and secretary IgA levels), but the authors did not determine if the effect was due to direct β-glucan stimulation of host immune cells or alterations in the gut microbial populations (131, 136).
In ovo injection of vaccines or immunostimulants represents an interesting way to alter the immune system of poultry before hatch and environmental exposure to pathogens. For the past 25 years, poultry producers have utilized in ovo technologies to safety and effectively vaccinate chicken, turkey, and quail embryos for common poultry diseases such as Marek's disease, infectious bursal disease (IBD), and coccidiosis (137). Recently, immunostimulants have come to the forefront of in ovo applications as a mechanism to non-specifically enhance the immune system of poultry before hatch. In ovo injection of resiquimod, a TLR7/8 agonist, at embryo day 18 increased MCR1L-B positive macrophages in the trachea, lungs, duodenum, and large intestine of chicks at hatch (138). Furthermore, the authors show that following infection with infectious laryngotracheitis virus (ILTV) 1 day post hatch, cloacal shedding of ILTV at 7 d post infection was significantly reduced in resiquimod injected embryos and that resiquimod treatment induced type 1 IFN activity in macrophages. Of the in ovo immunostimulants, CpG DNA is perhaps the most well-studied (139–141). Abdul-Cader et al. (140) show that CpG DNA delivered in ovo upregulates IL-1β expression and macrophage proportions in the lungs and these changes are associated with reduced ILTV induced mortality and weight loss in chicks. A 2018 study (139) with in ovo administration of CpG DNA reported reduced mortality and clinical scores from experimental E. coli infection of yolk sacs in day old chicks. Indeed, a commercial product Victrio®, is an in ovo DNA immunostimulant marketed to reduce mortality in embryonated eggs and chicks from E. coli and is shown to activate TLR21 on chicken macrophages and increase nitric oxide production (70). Overall, in ovo exposure to innate agonists altered immune responses to disease; however, it is unclear if the impact is the result of ongoing immune activation, or was the result of innate memory. The length of time from agonist exposure to challenge testing suggests innate memory may be at play, but targeted studies are warranted to clearly define the mechanism of protection.
Fish
Unlike most other food animal species, review articles have been published summarizing the evidence for trained immunity in fish (142, 143) and we would direct readers to those sources for an in-depth review of innate training in various fish species. Since the 1990's, β-glucan from a variety of sources was studied or fed in commercial fisheries for its growth promoting and immunomodulating effects (144, 145). However, detailed analysis suggests the observed benefits are dependent on β-glucan source and dose, fish species, and age (145). Heterologous protection against bacterial challenge occurs after intraperitoneal or oral administration of β-glucans in multiple fish species ranging from Zebrafish (Danio rerio) (146) to Yellowtail (Seriola quinqueradiata) (147) to Orange spotted grouper (Epinephelus coioides) (148). Researchers observed lower mortality; increased oxidative burst, cytokine production, and lysozyme activity (143, 146, 147). The decreased mortality was observed up to 30 days after β-glucan feeding ceased (148). The length of effect after withdrawl suggests, similar to mammalian studies (34, 36, 123), dietary β-glucan treatment in fish induces epigenetic changes at the progenitor level allowing for sustained changes to innate immune cells. Fish express a higher diversity and variation of innate receptors that are both similar to and distinct from mammalian receptors (149). Dectin-1, a C-type lectin, is the primary myeloid receptor for β-glucans in mammals (32, 150–152); however, no corresponding β-glucan receptor has been identified in fish. Recently, Petit et al. (153) identified several potential candidate receptors for β-glucan in European common carp (Cyprinus carpio carpio). They also showed that, as with mammals, the C-type lectin pathway is involved in detection and signaling in response to β-glucan (153). This and other studies lay a foundation for mechanistic work in fish to determine the direct and long-lasting effect of β-glucan on the fish immune system.
Of the molecules known to stimulate innate memory (1, 123, 154), β-glucans are those most commonly used in aquaculture, but others, including mycobacteria, have been studied. Reviewed in Petit and Wiegertjes (142), studies show intraperitoneal injection with Mycobacterium butyricum enhances bactericidal activity up to 33 d post injection (155). A series of studies by Kato et al. (156–158) indicate injection with BCG enhances innate immune responses in multiple fish species and induces protection against challenge with Nocardia seriolae in Japanese flounder. Yellowtail (Seriola quinqueradiata) first exposed to one of several immunostimulants were protected against Pasteurella piscicada disease. Specifically, pre-exposure to Freund's complete adjuvant (CFA), which contains inactivated M. bovis, was found to be the most protective (147). Indeed, while enhanced survival and immune markers were observed with glucan pretreatment, the effect was markedly heightened with CFA treatment. The observed cross protection observed with these last studies are hallmarks of innate training and is strong evidence for innate memory in fish.
When reviewing literature published before innate memory was well-described, and in the absence of studies specificly designed to investigate the induction of heterologous protection independent of adaptive immune system, it can be difficult to assign innate memory as the mechanism for enhanced disease resistance. For example, Lorenzen et al. (159) observed cross-protection against viral hemorrhagic septicemia virus (VHSV) in rainbow trout inoculated with a plasmid DNA encoding the viral glycoprotein from an unrelated virus. Mortality was decreased in plasmid inoculated fish when challenged with virus either 4 and 7 days after plasmid inoculation, but not at 60 or 84 days post inoculation. The limited window of protection suggests a mechanism independent of adaptive immunity. A later study of juvinal turbot (Scophthalmus maximus) inoculated with DNA plasmid encoding the VHSV envelope glycoprotein and challenged with unrelated virus also observed reduced mortailities in the plasmid inoculated fish (160). It's unclear if the protection was due to innate training, or just non-specific protection due to the primary reponse to plasmid DNA. Regarless, some immunomodulation occurred to enhance disease resistance. Mechanistic evidence of contemporary trained immunity (as described by human and rodent literature) remains to be described. As demand for fish increases, methods to enhance disease resistance in farmed fish without high cost and antibiotics is desired.
Implementing Innate Modulation in Food Animal Agriculture
The primary objective of innate immunomodulation in food animals is to enhance the immune status of the animal, thus resisting disease to enhance animal welfare and production efficiency. Enhancing the animal's ability to resist disease could reduce the need for antibiotics and amount of feed required to get an animal to market weight. In most production systems there are clearly defined periods in which animals are known to be at high risk for infection. Universal to all production systems is the susceptibility of the neonatal or very young animal to disease (161). As maternal immunity wanes, and an infant's own adaptive immune system is inexperienced, there is a window of heightened vulnerability to disease. The adaptive immune system may not be fully matured in neonates, but the innate immune system is active and provides a key role in immune responses at this age [reviewed in (162)] making it a good target for enhanced protection. In humans, innate training mediated by BCG is effective for more than a year (124). While longevity of protection by innate training is important, it may also be important to initiate protection early in life. The time from birth to market for a particular species can range from weeks to years, for example broilers go to market at around 6 weeks of age, pigs at 6 months, and beef cattle at 2 years. Thus, an important consideration for harnessing innate training is length of protection but also how quickly innate training protection is evident in the animal. One recent review of antibiotic usage by pig producers in Belgium reported that more than 80% of all antibiotics are administered to piglets <10 weeks of age (163); and similar results were reported for North America and several other countries in Europe (164). An important consideration for harnessing innate training is length of protection but also how quickly innate training protection is induced to minimize disease during a high-risk period.
Calves are most susceptible to diarrheal diseases in the first 4 weeks of life, and then the risk for respiratory diseases increases as they mature to 2–6 months of age. Induction of innate training in the neonatal period would be expected to promote improved disease resistance through at least 6 months of age, when the “window of susceptibility” to infection is the greatest (165). Subsequent high-risk periods for beef cattle include the shipping and receiving period (first 50 days on feed), when cattle are weaned, trucked, co-mingled, and placed in the feedlot (166). Administration of BCG, β-glucan, or other known immunomodulator in the period prior to weaning and shipping could protect the animal through the receiving period. In support of this supposition, the commercial immunomodulators Zelnate™ and Amplimune™ have both shown some benefit when administered to calves immediately prior to or following placement in the feedlot (67–69, 72). Dairy cattle are known to go through a period of immunosuppression during the transition period (the 3 weeks prior to calving, through the 3 weeks after calving) leading to a sharp increase in the prevalence of infectious and metabolic diseases (167, 168). Exploiting the effects of innate training or tolerance during the periparturient period could have beneficial effects on cow health and performance.
As with pigs and cattle, fish and poultry are most susceptible to disease while they are very young. In fish, immunostimulants have been administered to newly hatched larvae directly via feed pellets, or indirectly via bath treatment (143, 144, 169–171). In poultry, as described in detail above, in ovo vaccination is industry standard and there exists both experimental and commercial (Victrio®) evidence of protection from disease with non-specific immunostimulants (70, 139, 140). As egg laying animals, immunomodulation can occur before hatch and before the fry or chick is exposed to a broader range of pathogens. Additionally, the passive transfer of antibodies from the mother to the progeny via the yolk in egg-laying species is well-described, but in fish, innate immune components have been shown to transfer from the dams to the oocytes and direct passage of immunostimulants from mother to young have also been described (172–174). One last benefit of innate modulation in both poultry and fish is the evidence of passage of transgenerational epigenetic changes to innate immune phenotypes and genes for both broiler chickens (175) and fish (176, 177). Combined with a dam's ability to produce large numbers of eggs, changes in epigenetic phenotypes (the hallmark of innate memory) could be inherited by the embroyos allowing for animals to hatch in a primed state to face bacterial or viral challenge. In some ways fish and poultry producers have an advantage beyond that of mammalian species to harness innate memory to prevent disease, and both industries have made strides to study and utilize these evolutionary advantages.
Potential Pitfalls of Innate Training
Although harnessing innate training for enhancing disease resistance is appealing, particularly in the context of the known periods of susceptibility or immunosuppression described above, there are still potential pitfalls that warrant consideration. In humans and rodents, innate training can have deleterious effects in the context of chronic inflammatory conditions such as autoimmunity, atherosclerosis, and diabetes (3). In food producing animals, while heightened immune responses may be beneficial for pathogen clearance, an increased inflammatory response may lead to tissue damage. In the case of respiratory diseases, for example, dysregulated inflammatory responses are often implicated as causing more damage to the host than the pathogen itself (178–181). Further enhancing this innate inflammatory response may not be ideal. However, if innate training has the capacity to reduce shedding of the organism, its use may still provide significant benefit to the health of the herd by reducing the risk of disease transmission. The impact of innate training on tissue pathology, pathogen burden and overall outcome of disease will need to be carefully evaluated in the context of particular disease settings in order to determine the risk vs. reward of engaging innate memory.
In addition to the potential of enhancing pathology of a disease, harnessing the immune system for disease resistance may negatively impact production parameters. Activation of the immune system comes at a considerable metabolic cost to an organism (182–185) and becomes energy not spent on production of muscle or milk. Activation of cells for the synthesis and secretion of cytokines and acute phase proteins, and cellular proliferation all require glucose, amino acids, and energy. To ensure survival of the host, the integrity of the immune system is maintained above nearly all other biological functions, partitioning nutrients away from growth, reproduction, and lactation (182). Although there is currently little supporting evidence available, it is almost certain that induction and maintenance of the innate immune cells in a trained state comes at some catabolic cost to the animal. Glycolysis, the metabolic pathway favored by trained monocytes and macrophages (46, 47) is less efficient than oxidative phosphorylation; thus increasing the cost of per-cell energy use. In addition, the initial activation of the immune system to induce a trained state utilizes metabolic resources. Although there is significant benefit to the animal in limiting disease upon pathogen exposure, it is currently unknown if the energy costs of a trained immune system will negatively affect performance, or if this possible performance loss will outweigh the benefit of increased disease resistance. Additional studies focused on the efficacy of innate training for preventing disease in food animals, as well as the impacts of training on animal performance and growth, will be required to unravel these possibilities. Regardless, strategies that enhance disease resistance without antibiotics warrant consideration to limit the impacts of antimicrobial resistance.
Summary and Conclusions
Few studies have directly examined trained immunity in food animal species. However, as discussed here, a plethora of evidence exists for a variety of immunostimulants to enhance non-specific, heterologous protection against bacterial and viral disease in cattle, swine, poultry, fish, and small ruminants. Innate memory presents an exciting opportunity to prevent or limit disease as well as reduce antibiotic use and AMR in agricultural animals. A number of opportunities exist for mechanistic studies to elucidate the cell types, pathways, and molecules involved in innate memory in food animals. Until innate training and tolerance are better understood, caution is warranted to determine the immunological and metabolic costs and efficacy of protection to specific diseases. Within innate memory is the potential to reduce disease burden and antibiotic use in animal agriculture, and we feel this area of investigation represents one of the most exciting fields of study for a new generation of scientists.
Author Contributions
KB, CL, and JM wrote and reviewed the manuscript.
Funding
All opinions expressed in this paper are the authors' and do not necessarily reflect the policies and views of USDA or ISU.
Conflict of Interest
CL and JM have received research funding from different companies to perform studies on in-feed β-glucan or yeast product immunomodulation in swine or cattle. Results from those studies are not provided within this review.
The remaining author declares that the research was conducted in the absence of any commercial or financial relationships that could be construed as a potential conflict of interest.
Acknowledgments
We thank Christopher Tuggle, Haibo Liu, and Juber Herrera-Uribe at Iowa State University for discussions of innate training in cattle and swine, especially molecular assays to assess epigenetic changes.
References
1. Kleinnijenhuis J, Quintin J, Preijers F, Joosten LA, Ifrim DC, Saeed S, et al. Bacille Calmette-Guerin induces NOD2-dependent nonspecific protection from reinfection via epigenetic reprogramming of monocytes. Proc Natl Acad Sci USA. (2012) 109:17537–42. doi: 10.1073/pnas.1202870109
2. Kleinnijenhuis J, Quintin J, Preijers F, Joosten LA, Jacobs C, Xavier RJ, et al. BCG-induced trained immunity in NK cells: Role for non-specific protection to infection. Clin Immunol. (2014) 155:213–9. doi: 10.1016/j.clim.2014.10.005
3. Netea MG, Joosten LAB, Latz E, Mills KHG, Natoli G, Stunnenberg HG, et al. Trained immunity: a program of innate immune memory in health and disease. Science. (2016) 352:aaf1098. doi: 10.1126/science.aaf1098
4. Jensen KJ, Larsen N, Biering-Sorensen S, Andersen A, Eriksen HB, Monteiro I, et al. Heterologous immunological effects of early BCG vaccination in low-birth-weight infants in guinea-bissau: a randomized-controlled trial. J Infect Dis. (2015) 211:956–67. doi: 10.1093/infdis/jiu508
5. Biering-Sorensen S, Aaby P, Napirna BM, Roth A, Ravn H, Rodrigues A, et al. Small randomized trial among low-birth-weight children receiving bacillus calmette-guerin vaccination at first health center contact. Pediatr Infect Dis J. (2012) 31:306–8. doi: 10.1097/INF.0b013e3182458289
6. Aaby P, Roth A, Ravn H, Napirna BM, Rodrigues A, Lisse IM, et al. Randomized trial of BCG vaccination at birth to low-birth-weight children: beneficial nonspecific effects in the neonatal period? J Infect Dis. (2011) 204:245–52. doi: 10.1093/infdis/jir240
7. Patel AA, Zhang Y, Fullerton JN, Boelen L, Rongvaux A, Maini AA, et al. The fate and lifespan of human monocyte subsets in steady state and systemic inflammation. J Exp Med. (2017) 214:1913–23. doi: 10.1084/jem.20170355
8. Mitroulis I, Ruppova K, Wang B, Chen LS, Grzybek M, Grinenko T, et al. Modulation of myelopoiesis progenitors is an integral component of trained immunity. Cell. (2018) 172:147–61.e12. doi: 10.1016/j.cell.2017.11.034
9. Kaufmann E, Sanz J, Dunn JL, Khan N, Mendonca LE, Pacis A, et al. BCG educates hematopoietic stem cells to generate protective innate immunity against tuberculosis. Cell. (2018) 172:176–90.e19. doi: 10.1016/j.cell.2017.12.031
10. Benn CS, Netea MG, Selin LK, Aaby P. A small jab - a big effect: nonspecific immunomodulation by vaccines. Trends Immunol. (2013) 34:431–9. doi: 10.1016/j.it.2013.04.004
11. Beeson PB. Development of tolerance to typhoid bacterial pyrogen and its abolition by reteculo-endothelial blockade. Proc Soc Exp Biol Med. (1946) 61:248–50. doi: 10.3181/00379727-61-15291P
12. Morris M, Li L. Molecular mechanisms and pathological consequences of endotoxin tolerance and priming. Arch Immunol Ther Exp (Warsz). (2012) 60:13–8. doi: 10.1007/s00005-011-0155-9
13. Biswas SK, Lopez-Collazo E. Endotoxin tolerance: new mechanisms, molecules and clinical significance. Trends in Immunology. (2009) 30:475–87. doi: 10.1016/j.it.2009.07.009
14. Shwartzman G. Studies on bacillus typhosus toxic substances I. phenomenon of local skin reactivity to B. Typhosus culture filtrate. J Exp Med. (1928) 48:247–68. doi: 10.1084/jem.48.2.247
15. Heremans H, van Damme J, Dillen C, Dijkmans R, Billiau A. Interferon gamma, a mediator of lethal lipopolysaccharide-induced Shwartzman-like shock reactions in mice. J Exp Med. (1990) 171:1853–69. doi: 10.1084/jem.171.6.1853
16. Chahin AB, Opal JM, Opal SM. Whatever happened to the Shwartzman phenomenon? Innate Immun. (2018) 24:466–79. doi: 10.1177/1753425918808008
17. Ifrim DC, Quintin J, Joosten LA, Jacobs C, Jansen T, Jacobs L, et al. Trained immunity or tolerance: opposing functional programs induced in human monocytes after engagement of various pattern recognition receptors. Clin Vaccine Immunol. (2014) 21:534–45. doi: 10.1128/CVI.00688-13
18. van Meirt AS, Frens J. The reaction of different animal species to bacterial pyrogens. Transbound Emerg Dis. (1968) 15:532–43. doi: 10.1111/j.1439-0442.1968.tb00456.x
19. Cavaillon JM, Pitton C, Fitting C. Endotoxin tolerance is not a LPS-specific phenomenon: partial mimicry with IL-1, IL-10 and TGFβ. J Endotoxin Res. (2016) 1:21–9. doi: 10.1177/096805199400100105
20. Cavaillon JM. The nonspecific nature of endotoxin tolerance. Trends Microbiol. (1995) 3:320–4. doi: 10.1016/S0966-842X(00)88963-5
21. Underhill DM. Macrophage recognition of zymosan particles. J Endotoxin Res. (2003) 9:176–80. doi: 10.1179/096805103125001586
22. Sonck E, Stuyven E, Goddeeris B, Cox E. The effect of beta-glucans on porcine leukocytes. Vet Immunol Immunopathol. (2010) 135:199–207. doi: 10.1016/j.vetimm.2009.11.014
23. Levy O, Levy O. Ready to benefit from training: heterologous effects of early life immunization. Trans R Soc Trop Med Hyg. (2015) 109:3–4. doi: 10.1093/trstmh/tru185
24. van't Wout JW, Poell R, van Furth R. The role of BCG/PPD-activated macrophages in resistance against systemic candidiasis in mice. Scand J Immunol. (1992) 36:713–20. doi: 10.1111/j.1365-3083.1992.tb03132.x
25. Arts RJW, Moorlag SJCFM, Novakovic B, Li Y, Wang SY, Oosting M, et al. BCG Vaccination protects against experimental viral infection in humans through the induction of cytokines associated with trained immunity. Cell Host Microbe. (2018) 23:89–100.e5. doi: 10.1016/j.chom.2017.12.010
26. de Bree CLCJ, Marijnissen RJ, Kel JM, Huber SKR, Aaby P, Benn CS. Bacillus calmette-guérin-induced trained immunity is not protective for experimental influenza A/Anhui/1/2013 (H7N9) infection in mice. Front Immunol. (2018) 9:869. doi: 10.3389/fimmu.2018.00869
27. Henderson DW, Lancaster MC, Packman L, Peacock S. The influence of a pre-existing respiratory infection on the course of another superimposed by the respiratory route. Br J Exp Pathol. (1956) 37:597–611.
28. Dubos RJ, Schaedler RW. Effects of cellular constituents of mycobacteria on the resistance of mice to heterologous infections. J Exp Med. (1957) 106:703–17. doi: 10.1084/jem.106.5.703
29. Weiss D. Enhanced resistance of mice to infection with pasteurella pestis following vaccination with fractions of phenolkilled tubercle bacilli. Nature. (1960) 186:1060–1. doi: 10.1038/1861060a0
30. Coppel S, Youmans GP. Specificity of acquired resistance produced by immunization with mycobacterial cells and mycobacterial fractions. J Bacteriol. (1968) 97:114–20. doi: 10.1128/JB.97.1.114-120.1969
31. Howard JG, Biozzi G, Halpern BN, Stiffel C, Mouton D. The effect of mycobacterium tuberculosis (BCG) infection on the resistance of mice to bacterial endotoxin and salmonella enteritidis infection. Br J Exp Pathol. (1959) 40:281–90.
32. Goodridge HS, Reyes CN, Becker CA, Katsumoto TR, Ma J, Wolf AJ, et al. Activation of the innate immune receptor Dectin-1 upon formation of a 'phagocytic synapse'. Nature. (2011) 472:471–5. doi: 10.1038/nature10071
33. Goodridge HS, Wolf AJ, Underhill DM. B-glucan recognition by the innate immune system. Immunol Rev. (2009) 2:38–50. doi: 10.1111/j.1600-065X.2009.00793.x
34. Quintin J, Saeed S, Martens JHA, Giamarellos-Bourboulis EJ, Ifrim DC, Logie C, et al. Candida albicans infection affords protection against reinfection via functional reprogramming of monocytes. Cell Host Microbe. (2012) 12:223–32. doi: 10.1016/j.chom.2012.06.006
35. Saeed S, Quintin J, Kerstens HHD, Nagesha AR, Aghajanirefah A, Matarese F, et al. Epigenetic programming of monocyte-to-macrophage differentiation and trained innate immunity. Science. (2014) 345:1251086. doi: 10.1126/science.1251086
36. Novakovic B, Habibi E, Wang SY, Arts RJW, Davar R, Megchelenbrink W, et al. β-Glucan reverses the epigenetic state of LPS-induced immunological tolerance. Cell. (2016) 167:1354–68.e14. doi: 10.1016/j.cell.2016.09.034
37. Blok BA, Arts RJW, van Crevel R, Benn CS, Netea MG. Trained innate immunity as underlying mechanism for the long-term, nonspecific effects of vaccines. J Leukocyte Biol. (2015) 98:347–56. doi: 10.1189/jlb.5RI0315-096R
38. Jensen KJ, Benn CS, van Crevel R. Unravelling the nature of non-specific effects of vaccines-A challenge for innate immunologists. Semin Immunol. (2016) 28:377–83. doi: 10.1016/j.smim.2016.05.005
39. Christ A, Bekkering S, Latz E, Riksen NP. Long-term activation of the innate immune system in atherosclerosis. Semin Immunol. (2016) 28:384–93. doi: 10.1016/j.smim.2016.04.004
40. Arts RJW, Joosten LAB, Netea MG. The potential role of trained immunity in autoimmune and autoinflammatory disorders. Front Immunol. (2018) 9:298. doi: 10.3389/fimmu.2018.00298
41. Walk J, de Bree LCJ, Graumans W, Stoter R, van Gemert GJ, van de Vegte-Bolmer M, et al. Outcomes of controlled human malaria infection after BCG vaccination. Nat Commun. (2019) 10:874. doi: 10.1038/s41467-019-08659-3
42. van der Heijden C, Noz MP, Joosten LAB, Netea MG, Riksen NP, Keating ST. Epigenetics and trained immunity. Antioxid Redox Signal. (2018) 29:1023–40. doi: 10.1089/ars.2017.7310
43. Mehta A, Baltimore D. MicroRNAs as regulatory elements in immune system logic. Nat Rev Immunol. (2016) 16:279–94. doi: 10.1038/nri.2016.40
44. Moutinho C, Esteller M. MicroRNAs and epigenetics. Adv Cancer Res. (2017) 135:189–220. doi: 10.1016/bs.acr.2017.06.003
45. O'Neill LA, Kishton RJ, Rathmell J. A guide to immunometabolism for immunologists. Nat Rev Immunol. (2016) 16:553–65. doi: 10.1038/nri.2016.70
46. Cheng SC, Quintin J, Cramer RA, Shepardson KM, Saeed S, Kumar V, et al. mTOR- and HIF-1alpha-mediated aerobic glycolysis as metabolic basis for trained immunity. Science. (2014) 345:1250684. doi: 10.1126/science.1250684
47. Arts RJ, Novakovic B, Ter Horst R, Carvalho A, Bekkering S, Lachmandas E, et al. Glutaminolysis and fumarate accumulation integrate immunometabolic and epigenetic programs in trained immunity. Cell Metab. (2016) 24:807–19. doi: 10.1016/j.cmet.2016.10.008
48. Arts RJW, Carvalho A, La Rocca C, Palma C, Rodrigues F, Silvestre R, et al. Immunometabolic pathways in BCG-induced trained immunity. Cell Rep. (2016) 17:2562–71. doi: 10.1016/j.celrep.2016.11.011
49. Foster SL, Hargreaves DC, Medzhitov R. Gene-specific control of inflammation by TLR-induced chromatin modifications. Nature. (2007) 447:972–8. doi: 10.1038/nature05836
50. Schlums H, Cichocki F, Tesi B, Theorell J, Beziat V, Holmes TD, et al. Cytomegalovirus infection drives adaptive epigenetic diversification of NK cells with altered signaling and effector function. Immunity. (2015) 42:443–56. doi: 10.1016/j.immuni.2015.02.008
51. Hashimoto D, Chow A, Noizat C, Teo P, Mary Beasley B, Leboeuf M, et al. Tissue-resident macrophages self-maintain locally throughout adult life with minimal contribution from circulating monocytes. Immunity. (2013) 38:792–804. doi: 10.1016/j.immuni.2013.04.004
52. Yao Y, Jeyanathan M, Haddadi S, Barra NG, Vaseghi-Shanjani M, Damjanovic D, et al. Induction of autonomous memory alveolar macrophages requires T cell help and is critical to trained immunity. Cell. (2018) 175:1634–50.e17. doi: 10.1016/j.cell.2018.09.042
53. Lavin Y, Winter D, Blecher-Gonen R, David E, Keren-Shaul H, Merad M, et al. Tissue-resident macrophage enhancer landscapes are shaped by the local microenvironment. Cell. (2014) 159:1312–26. doi: 10.1016/j.cell.2014.11.018
54. Hole CR, Wager CML, Castro-Lopez N, Campuzano A, Cai H, Wozniak KL, et al. Induction of memory-like dendritic cell responses in vivo. Nat Commun. (2019) 10:2955. doi: 10.1038/s41467-019-10486-5
55. Pacis A, Tailleux L, Morin AM, Lambourne J, MacIsaac JL, Yotova V, et al. Bacterial infection remodels the DNA methylation landscape of human dendritic cells. Genome Res. (2015) 25:1801–11. doi: 10.1101/gr.192005.115
56. Hamada A, Torre C, Drancourt M, Ghigo E. Trained immunity carried by non-immune cells. Front Microbiol. (2018) 9:3225. doi: 10.3389/fmicb.2018.03225
57. De Boever S, Beyaert R, Vandemaele F, Baert K, Duchateau L, Goddeeris B, et al. The influence of age and repeated lipopolysaccharide administration on body temperature and the concentration of interleukin-6 and IgM antibodies against lipopolysaccharide in broiler chickens. Avian Pathol. (2008) 37:39–44. doi: 10.1080/03079450701784875
58. Whyte RI, Warren HS, Greene E, Glennon ML, Robinson DR, Zapol WM. Tolerance to low-dose endotoxin in awake sheep. J Appl Physiol. (1989) 66:2546–52. doi: 10.1152/jappl.1989.66.6.2546
59. Juste RA, Alonso-Hearn M, Garrido JM, Abendaño N, Sevilla IA, Gortazar C, et al. Increased lytic efficiency of bovine macrophages trained with killed mycobacteria. PLoS ONE. (2016) 11:e0165607. doi: 10.1371/journal.pone.0165607
60. Guerra-Maupome M, Vang DX, McGill JL. Aerosol vaccination with bacille calmette-guerin induces a trained innate immune phenotype in calves. PLoS ONE. (2019) 14:e0212751. doi: 10.1371/journal.pone.0212751
61. Shalaby MA, Saleh SM, el-Atrash S, Sami AM, el-Sanousi AA, Saber MA, et al. Application of Corynebacterium cutis lysate as an immune stimulant in cattle. Mol Biother. (1992) 4:147–50.
62. Rankin JD, Taylor RJ, Newman G. The protection of calves against infection with Salmonella typhimurium by means of a vaccine prepared from salmonella dublin (strain 51). Vet Rec. (1967) 80:720–6. doi: 10.1136/vr.80.25.720
63. Smith BP, Reina-Guerra M, Stocker BA, Hoiseth SK, Johnson E. Aromatic-dependent salmonella dublin as a parenteral modified live vaccine for calves. Am J Vet Res. (1984) 45:2231–5.
64. Wray C, Sojka WJ, Morris JA, Brinley Morgan WJ. The immunization of mice and calves with gal E mutants of Salmonella typhimurium. J Hyg (Lond). (1977) 79:17–24. doi: 10.1017/S0022172400052803
65. Hormaeche CE, Joysey HS, Desilva L, Izhar M, Stocker BA. Immunity conferred by aro- salmonella live vaccines. Microb Pathog. (1991) 10:149–58. doi: 10.1016/0882-4010(91)90075-L
66. Kamble NM, Hajam IA, Lee JH. Orally administered live attenuated Salmonella typhimurium protects mice against lethal infection with H1N1 influenza virus. Vet Microbiol. (2017) 201:1–6. doi: 10.1016/j.vetmic.2017.01.006
67. Nickell JS, Keil DJ, Settje TL, Lechtenberg KF, Singu V, Woolums AR. Efficacy and safety of a novel DNA immunostimulant in cattle. Bov Pract. (2016) 50:9–20. doi: 10.21423/bovine-vol50no1p9-20
68. Rogers KC, Miles DG, Renter DG, Sears JE, Woodruff JL. Effects of delayed respiratory viral vaccine and/or inclusion of an immunostimulant on feedlot health, performance, and carcass merits of auction-market derived feeder heifers. Bov Pract. (2016) 50:154–162. doi: 10.21423/bovine-vol50no2p154-164
69. Woolums AR, Karisch BB, Parish JA, Park J, Seo KS, Badial P, et al. Effect of a DNA-based immunostimulant on growth, performance, and expression of inflammatory and immune mediators in beef calves abruptly weaned and introduced to a complete ration. J Anim Sci. (2019) 97:111–121. doi: 10.1093/jas/sky392
70. Ilg T. Investigations on the molecular mode of action of the novel immunostimulator ZelNate: activation of the cGAS-STING pathway in mammalian cells. Mol Immunol. (2017) 90:182–9. doi: 10.1016/j.molimm.2017.07.013
71. Radoslaw R, Rick C, Stan A, Maira JMP, Dejan B, Aleksandar M, et al. Mycobacterium cell wall fraction immunostimulant (AMPLIMUNE™) efficacy in the reduction of the severity of ETEC induced diarrhea in neonatal calves. Acta Veterinaria. (2017) 67:222–37. doi: 10.1515/acve-2017-0019
72. Nosky B, Biwer J, Alkemade S, Prunic B, Milovanovic A, Maletic M, et al. Effect of a non-specific immune stimulant (Amplimune™) on the health and production of light feedlot calves. J Dairy Vet Animal Res. (2017) 6:00179. doi: 10.15406/jdvar.2017.06.00179
73. Castrucci G, Osburn BI, Frigeri F, Ferrari M, Salvatori D, Lo Dico M, et al. The use of immunomodulators in the control of infectious bovine rhinotracheitis. Comp Immunol Microbiol Infect Dis. (2000) 23:163–73. doi: 10.1016/S0147-9571(99)00069-7
74. Castrucci G, Frigeri F, Osburn BI, Ferrari M, Barreca F, Salvatori D. Further investigations on the efficacy of a non-specific defence inducer evaluated in calves exposed to infectious bovine rhinotracheitis virus. Comp Immunol Microbiol Infect Dis. (1998) 21:155–63. doi: 10.1016/S0147-9571(97)00014-3
75. Castrucci G, Ferrari M, Osburn BI, Frigeri F, Barreca F, Tagliati S, et al. A non-specific defence inducer in preventing clinical signs of infectious bovine rhinotracheitis in calves. Comp Immunol Microbiol Infect Dis. (1996) 19:163–9. doi: 10.1016/0147-9571(96)00001-X
76. Castrucci G, Ferrari M, Osburn BI, Frigeri F, Barreca F, Tagliati S, et al. The use of a non-specific defence mechanism inducer in calves exposed to bovine herpesvirus-1 infection: preliminary trials. Comp Immunol Microbiol Infect Dis. (1995) 18:85–91. doi: 10.1016/0147-9571(95)98849-D
77. Wu ZH, Yu Y, Alugongo GM, Xiao JX, Li JH, Li YX, et al. Short communication: effects of an immunomodulatory feed additive on phagocytic capacity of neutrophils and relative gene expression in circulating white blood cells of transition Holstein cows. J Dairy Sci. (2017) 100:7549–55. doi: 10.3168/jds.2016-12528
78. Ryman VE, Nickerson SC, Kautz FM, Hurley DJ, Ely LO, Wang YQ, et al. Effect of dietary supplementation on the antimicrobial activity of blood leukocytes isolated from Holstein heifers. Res Vet Sci. (2013) 95:969–74. doi: 10.1016/j.rvsc.2013.09.009
79. Wang YQ, Puntenney SB, Burton JL, Forsberg NE. Use of gene profiling to evaluate the effects of a feed additive on immune function in periparturient dairy cattle. J Anim Physiol Anim Nutr (Berl). (2009) 93:66–75. doi: 10.1111/j.1439-0396.2007.00780.x
80. Nace EL, Nickerson SC, Kautz FM, Breidling S, Wochele D, Ely LO, et al. Modulation of innate immune function and phenotype in bred dairy heifers during the periparturient period induced by feeding an immunostimulant for 60 days prior to delivery. Vet Immunol Immunopathol. (2014) 161:240–50. doi: 10.1016/j.vetimm.2014.08.013
81. Knoblock CE, Shi W, Yoon I, Oba M. Effects of supplementing a Saccharomyces cerevisiae fermentation product during the periparturient period on the immune response of dairy cows fed fresh diets differing in starch content. J Dairy Sci. (2019) 102:6199–209. doi: 10.3168/jds.2018-16224
82. Alugongo GM, Xiao JX, Chung YH, Dong SZ, Li SL, Yoon I, et al. Effects of saccharomyces cerevisiae fermentation products on dairy calves: performance and health. J Dairy Sci. (2017) 100:1189–99. doi: 10.3168/jds.2016-11399
83. Brewer MT, Anderson KL, Yoon I, Scott MF, Carlson SA. Amelioration of salmonellosis in pre-weaned dairy calves fed Saccharomyces cerevisiae fermentation products in feed and milk replacer. Vet Microbiol. (2014) 172:248–55. doi: 10.1016/j.vetmic.2014.05.026
84. Vélez J, Lange MK, Zieger P, Yoon I, Failing K, Bauer C. Long-term use of yeast fermentation products in comparison to halofuginone for the control of cryptosporidiosis in neonatal calves. Vet Parasitol. (2019) 269:57–64. doi: 10.1016/j.vetpar.2019.04.008
85. Vázquez Flores S, de Jesús Guerrero Carrillo M, Scott MF, Hamann J, Almanza SB, Guizar Bravo C, et al. 1472 Effects of Saccharomyces cerevisiae fermentation products on intestinal villi integrity in neonatal calves naturally infected with cryptosporidium spp. J Anim Sci. (2016) 94:714–15. doi: 10.2527/jam2016-1472
86. Shen Y, Davedow T, Ran T, Saleem AM, Yoon I, Narvaez C, et al. Ruminally protected and unprotected Saccharomyces cerevisiae fermentation products as alternatives to antibiotics in finishing beef steers1. J Anim Sci. (2019) 97:4323–33. doi: 10.1093/jas/skz270
87. Duff GC, Galyean ML. Board-invited review: recent advances in management of highly stressed, newly received feedlot cattle. J Anim Sci. (2007) 85:823–40. doi: 10.2527/jas.2006-501
88. Finck DN, Ribeiro FRB, Burdick NC, Parr SL, Carroll JA, Young TR, et al. Yeast supplementation alters the performance and health status of receiving cattle. Prof Anim Sci. (2014) 30:333–41. doi: 10.15232/S1080-7446(15)30125-X
89. Zaleska B, Milewski S, Zabek K. Impact of Saccharomyces cerevisiae supplementation on reproductive performance, milk yield in ewes and offspring growth. Arch. Anim. Breed. (2015) 58:79–83. doi: 10.5194/aab-58-79-2015
90. Zabek K, Milewski S, Wojcik R, Siwicki AK. Effect of β-1,3/1,6-D-glucan in diet on productivity and humoral and cellular defense mechanisms in sheep. Acta Veterinaria Brno. (2013) 82:141–6. doi: 10.2754/avb201382020141
91. Khalkhane AS, Abbasi K, Zadeh FS, Arian AH. Effect of dietary beta-glucan supplementation on humoral and cellular immunologic factors in lambs. Glob Vet. (2013) 11:38–43. doi: 10.5829/idosi.gv.2013.11.1.73188
92. Waller KP, Colditz IG. Effect of intramammary infusion of beta-1,3-glucan or interleukin-2 on leukocyte subpopulations in mammary glands of sheep. Am J Vet Res. (1999) 60:703–7.
93. Angulo M, Reyes-Becerril M, Cepeda-Palacios R, Angulo C. Oral administration of debaryomyces hansenii CBS8339-β-glucan induces trained immunity in newborn goats. Dev Comp Immunol. (2020) 105:103597. doi: 10.1016/j.dci.2019.103597
94. Angulo M, Reyes-Becerril M, Cepeda-Palacios R, Tovar-Ramírez D, Esteban M, Angulo C. Probiotic effects of marine debaryomyces hansenii CBS 8339 on innate immune and antioxidant parameters in newborn goats. Appl Microbiol Biotechnol. (2019) 103:2339–52. doi: 10.1007/s00253-019-09621-5
95. Angulo M, Reyes-Becerril M, Tovar-Ramírez D, Ascencio F, Angulo C. Debaryomyces hansenii CBS 8339 β-glucan enhances immune responses and down-stream gene signaling pathways in goat peripheral blood leukocytes. Dev Comp Immunol. (2018) 88:173–82. doi: 10.1016/j.dci.2018.07.017
96. Medina-Córdova N, Reyes-Becerril M, Ascencio F, Castellanos T, Campa-Córdova AI, Angulo C. Immunostimulant effects and potential application of β-glucans derived from marine yeast debaryomyces hansenii in goat peripheral blood leucocytes. Int J Biol Macromol. (2018) 116:599–606. doi: 10.1016/j.ijbiomac.2018.05.061
97. Barakat A. Preliminary studies on the use of BCG as an immunopotentiating agent against rift valley fever among sheep in Egypt. Bull Off Int Epiz. (1981) 93:1387–93.
98. Barakat A. Immunization against caseous lymphadenitis of sheep using attenuated bovine tubercle bacillus of calmette and Guerin (BCG). Bull Off Int Epiz. (1979) 91:671–92.
99. Vetvicka V, Vannucci L, Sima P. The effects of β-glucan on pig growth and immunity. Open Biochem J. (2014) 8:89–93. doi: 10.2174/1874091X01408010089
100. Dritz SS, Shi J, Kielian TL, Goodband RD, Nelssen JL, Tokach MD, et al. Influence of dietary beta-glucan on growth performance, nonspecific immunity, and resistance to Streptococcus suis infection in weanling pigs. J Anim Sci. (1995) 73:3341–50.
101. Hiss S, Sauerwein H. Influence of dietary ss-glucan on growth performance, lymphocyte proliferation, specific immune response and haptoglobin plasma concentrations in pigs. J Anim Physiol Anim Nutr (Berl). (2003). 87:2–11. doi: 10.1046/j.1439-0396.2003.00376.x
102. Vetvicka V, Oliveira C. β(1-3)(1-6)-D-glucans modulate immune status in pigs: potential importance for efficiency of commercial farming. Ann Transl Med. (2014) 2:16. doi: 10.3978/j.issn.2305-5839.2014.01.04
103. Li J, Li DF, Xing JJ, Cheng ZB, Lai CH. Effects of beta-glucan extracted from Saccharomyces cerevisiae on growth performance, and immunological and somatotropic responses of pigs challenged with Escherichia coli lipopolysaccharide. J Anim Sci. (2006) 84:2374–81. doi: 10.2527/jas.2004-541
104. Sun Y, Park I, Guo J, Weaver AC, Kim SW. Impacts of low level aflatoxin in feed and the use of modified yeast cell wall extract on growth and health of nursery pigs. Anim Nutr. (2015) 1:177–83. doi: 10.1016/j.aninu.2015.08.012
105. Stuyven E, Cox E, Vancaeneghem S, Arnouts S, Deprez P, Goddeeris BM. Effect of beta-glucans on an ETEC infection in piglets. Vet Immunol Immunopathol. (2009) 128:60–6. doi: 10.1016/j.vetimm.2008.10.311
106. Kiarie E, Bhandari S, Scott M, Krause DO, Nyachoti CM. Growth performance and gastrointestinal microbial ecology responses of piglets receiving saccharomyces cerevisiae fermentation products after an oral challenge with Escherichia coli (K88). J Anim Sci. (2011) 89:1062–78. doi: 10.2527/jas.2010-3424
107. Wojnicki SJ, Morris A, Smith BN, Maddox CW, Dilger RN. Immunomodulatory effects of whole yeast cells and capsicum in weanling pigs challenged with pathogenic Escherichia coli1. J Anim Sci. (2019) 97:1784–95. doi: 10.1093/jas/skz063
108. Chethan GE, Garkhal J, Sircar S Y., Malik PS, Mukherjee R, Sahoo NR, et al. Immunomodulatory potential of beta-glucan as supportive treatment in porcine rotavirus enteritis. Vet Immunol Immunopathol. (2017) 191:36–43. doi: 10.1016/j.vetimm.2017.07.012
109. Baert K, Sonck E, Goddeeris BM, Devriendt B, Cox E. Cell type-specific differences in beta-glucan recognition and signalling in porcine innate immune cells. Dev Comp Immunol. (2015) 48:192–203. doi: 10.1016/j.dci.2014.10.005
110. Chaung HC, Huang TC, Yu JH, Wu ML, Chung WB. Immunomodulatory effects of beta-glucans on porcine alveolar macrophages and bone marrow haematopoietic cell-derived dendritic cells. Vet Immunol Immunopathol. (2009) 131:147–57. doi: 10.1016/j.vetimm.2009.04.004
111. Li B, Allendorf DJ, Hansen R, Marroquin J, Ding C, Cramer DE, et al. Yeast beta-glucan amplifies phagocyte killing of iC3b-opsonized tumor cells via complement receptor 3-Syk-phosphatidylinositol 3-kinase pathway. J Immunol. (2006) 177:1661–9. doi: 10.4049/jimmunol.177.3.1661
112. Li J, Xing J, Li D, Wang X, Zhao L, Lv S, et al. Effects of beta-glucan extracted from saccharomyces cerevisiae on humoral and cellular immunity in weaned piglets. Arch Anim Nutr. (2005) 59:303–12. doi: 10.1080/17450390500247832
113. Sonck E, Devriendt B, Goddeeris B, Cox E. Varying effects of different beta-glucans on the maturation of porcine monocyte-derived dendritic cells. Clin Vaccine Immunol. (2011) 18:1441–6. doi: 10.1128/CVI.00080-11
114. Coe NE, Frank DE, Wood RL, Roth JA. Alteration of neutrophil function in BCG-treated and non-treated swine after exposure to Salmonella typhimurium. Vet Immunol Immunopathol. (1992) 33:37–50. doi: 10.1016/0165-2427(92)90033-M
115. Jensen KJ, Hansen MS, Heegaard PMH, Benn CS, Jungersen G. The effect of inactivated mycobacterium paratuberculosis vaccine on the response to a heterologous bacterial challenge in pigs. Front Immunol. (2019) 10:1557. doi: 10.3389/fimmu.2019.01557
116. Ramos L, Obregon-Henao A, Henao-Tamayo M, Bowen R, Lunney JK, Gonzalez-Juarrero M. The minipig as an animal model to study Mycobacterium tuberculosis infection and natural transmission. Tuberculosis (Edinb). (2017) 106:91–8. doi: 10.1016/j.tube.2017.07.003
117. Diez-Delgado I, Sevilla IA, Romero B, Tanner E, Barasona JA, White AR, et al. Impact of piglet oral vaccination against tuberculosis in endemic free-ranging wild boar populations. Prev Vet Med. (2018) 155:11–20. doi: 10.1016/j.prevetmed.2018.04.002
118. Gortazar C, Beltran-Beck B, Garrido JM, Aranaz A, Sevilla IA, Boadella M, et al. de la fuente, oral re-vaccination of eurasian wild boar with Mycobacterium bovis BCG yields a strong protective response against challenge with a field strain. BMC Vet Res. (2014) 10:96. doi: 10.1186/1746-6148-10-96
122. Gadde U, Kim WH, Oh ST, Lillehoj HS. Alternatives to antibiotics for maximizing growth performance and feed efficiency in poultry: a review. Anim Health Res Rev. (2017) 18:26–45. doi: 10.1017/S1466252316000207
123. van der Meer JW, Joosten LA, Riksen N, Netea MG. Trained immunity: a smart way to enhance innate immune defence. Mol Immunol. (2015) 68:40–4. doi: 10.1016/j.molimm.2015.06.019
124. Netea MG, van Crevel R. BCG-induced protection: effects on innate immune memory. Semin Immunol. (2014) 26:512–7. doi: 10.1016/j.smim.2014.09.006
125. Cox CM, Stuard LH, Kim S, McElroy AP, Bedford MR, Dalloul RA. Performance and immune responses to dietary beta-glucan in broiler chicks. Poult Sci. (2010) 89:1924–33. doi: 10.3382/ps.2010-00865
126. Zhou TX, Jung JH, Zhang ZF, Kim IH. Effect of dietary β-glucan on growth performance, fecal microbial shedding and immunological responses after lipopolysaccharide challenge in weaned pigs. Anim Feed Sci Technol. (2013) 179:85–92. doi: 10.1016/j.anifeedsci.2012.10.008
127. Tang XY, Gao JS, Yuan F, Zhang WX, Shao YJ, Sakurai F, et al. Effects of sophy beta-glucan on growth performance, carcass traits, meat composition, and immunological responses of peking ducks. Poult Sci. (2011) 90:737–45. doi: 10.3382/ps.2010-01008
128. Ott CP, Omara II, Persia ME, Dalloul RA. The impact of beta-glucans on performance and response of broiler chickens during a coccidiosis challenge. Poult Sci. (2018) 97:2713–21. doi: 10.3382/ps/pey148
129. Huff GR, Huff WE, Jalukar S, Oppy J, Rath NC, Packialakshmi B. The effects of yeast feed supplementation on turkey performance and pathogen colonization in a transport stress/Escherichia coli challenge. Poult Sci. (2013) 92:655–62. doi: 10.3382/ps.2012-02787
130. Jacob JP, Pescatore AJ. Barley beta-glucan in poultry diets. Ann Transl Med. (2014) 2:20. doi: 10.3978/j.issn.2305-5839.2014.01.02
131. Shao Y, Guo Y, Wang Z. 1,3/1,6-Glucan alleviated intestinal mucosal barrier impairment of broiler chickens challenged with Salmonella enterica serovar typhimurium. Poul Sci. (2013) 92:1764–73. doi: 10.3382/ps.2013-03029
132. Lowry VK, Farnell MB, Ferro PJ, Swaggerty CL, Bahl A, Kogut MH. Purified beta-glucan as an abiotic feed additive up-regulates the innate immune response in immature chickens against salmonella enterica serovar Enteritidis. Int J Food Microbiol. (2005) 98:309–18. doi: 10.1016/j.ijfoodmicro.2004.06.008
133. Huff GR, Huff WE, Farnell MB, Rath NC, Solis de Los Santos F, Donoghue AM. Bacterial clearance, heterophil function, and hematological parameters of transport-stressed turkey poults supplemented with dietary yeast extract. Poult Sci. (2010) 89:447–56. doi: 10.3382/ps.2009-00328
134. Guo Y, Ali RA, Qureshi MA. The influence of beta-glucan on immune responses in broiler chicks. Immunopharmacol Immunotoxicol. (2003) 23:461–72. doi: 10.1081/IPH-120024513
135. Huff GR, Huff WE, Rath NC, Tellez G. Limited treatment with beta-1,3/1,6-glucan improves production values of broiler chickens challenged with Escherichia coli. Poult Sci. (2006) 85:613–8. doi: 10.1093/ps/85.4.613
136. Teng PY, Kim WK. Review: roles of prebiotics in intestinal ecosystem of broilers. Front Vet Sci. (2018) 5:245. doi: 10.3389/fvets.2018.00245
137. Peebles ED. In ovo applications in poultry: a review. Poult Sci. (2018) 97:2322–38. doi: 10.3382/ps/pey081
138. Abdul-Cader MS, De Silva Senapathi U, Ahmed-Hassan H, Sharif S, Abdul-Careem MF. Single stranded (ss)RNA-mediated antiviral response against infectious laryngotracheitis virus infection. BMC Microbiol. (2019) 19:34. doi: 10.1186/s12866-019-1398-6
139. Allan B, Wheler C, Koster W, Sarfraz M, Potter A, Gerdts V, et al. In ovo administration of innate immune stimulants and protection from early chick mortalities due to yolk sac infection. Avian Dis. (2018) 62:316–21. doi: 10.1637/11840-041218-Reg.1
140. Thapa S, Cader MS, Murugananthan K, Nagy E, Sharif S, Czub M, et al. In ovo delivery of CpG DNA reduces avian infectious laryngotracheitis virus induced mortality and morbidity. Viruses. (2015) 7:1832–52. doi: 10.3390/v7041832
141. Abdul-Cader MS, Amarasinghe A, Palomino-Tapia V, Ahmed-Hassan H, Bakhtawar K, Nagy E, et al. In ovo CpG DNA delivery increases innate and adaptive immune cells in respiratory, gastrointestinal and immune systems post-hatch correlating with lower infectious laryngotracheitis virus infection. PLoS ONE. (2018) 13:e0193964. doi: 10.1371/journal.pone.0193964
142. Petit J, Wiegertjes GF. Long-lived effects of administering beta-glucans: indications for trained immunity in fish. Dev Comp Immunol. (2016) 64:93–102. doi: 10.1016/j.dci.2016.03.003
143. Zhang Z, Chi H, Dalmo RA. Trained innate immunity of fish is a viable approach in larval aquaculture. Front Immunol. (2019) 10:42. doi: 10.3389/fimmu.2019.00042
144. Magnadottir B, Gudmundsdottir BK, Lange S, Steinarsson A, Oddgeirsson M, Bowden T, et al. Immunostimulation of larvae and juveniles of cod, Gadus morhua L. J Fish Dis. (2006) 29:147–55. doi: 10.1111/j.1365-2761.2006.00701.x
145. Dalmo RA, Bogwald J. Beta-glucans as conductors of immune symphonies. Fish Shellfish Immunol. (2008) 25:384–96. doi: 10.1016/j.fsi.2008.04.008
146. Rodriguez I, Chamorro R, Novoa B, Figueras A. beta-Glucan administration enhances disease resistance and some innate immune responses in zebrafish (Danio rerio). Fish Shellfish Immunol. (2009) 27:369–73. doi: 10.1016/j.fsi.2009.02.007
147. Kawakami H, Shinohara N, Sakai M. The non-specific immunostimulation and adjuvant effect of vibrio anguillarum bacterin, M-glucan, chitin, freund's complete adjuvant against Pasteurella piscicida n in yellowtail. Fish Pathol. (1998) 33:267–92. doi: 10.3147/jsfp.33.287
148. Chang CS, Huang SL, Chen S, Chen SN. Innate immune responses and efficacy of using mushroom beta-glucan mixture (MBG) on orange-spotted grouper, epinephelus coioides, aquaculture. Fish Shellfish Immunol. (2013) 35:115–25. doi: 10.1016/j.fsi.2013.04.004
149. Buchmann K. Evolution of innate immunity: clues from invertebrates via fish to mammals. Front Immunol. (2014) 5:459. doi: 10.3389/fimmu.2014.00459
150. Sonck E, Stuyven E, Goddeeris B, Cox E. Identification of the porcine C-type lectin dectin-1. Vet Immunol Immunopathol. (2009) 130:131–4. doi: 10.1016/j.vetimm.2009.01.010
151. Gow NA, Netea MG, Munro CA, Ferwerda G, Bates S, Mora-Montes HM, et al. Immune recognition of candida albicans beta-glucan by dectin-1. J Infect Dis. (2007) 196:1565–71. doi: 10.1086/523110
152. Gantner BN, Simmons RM, Canavera SJ, Akira S, Underhill DM. Collaborative induction of inflammatory responses by dectin-1 and Toll-like receptor 2. J Exp Med. (2003) 197:1107–17. doi: 10.1084/jem.20021787
153. Petit J, Bailey EC, Wheeler RT, de Oliveira CAF, Forlenza M, Wiegertjes GF. Studies into beta-glucan recognition in fish suggests a key role for the C-type lectin pathway. Front Immunol. (2019) 10:280. doi: 10.3389/fimmu.2019.00280
154. WHO. Observed Rate of Vaccine Reactions Bacille Calmette-Guerin (BCG) Vaccine. in: I. Global Vaccine Safety, Vaccines, and Biologicals, (Ed.), Geneva: World Health Organization (2012). Available online at: https://www.who.int/vaccine_safety/initiative/tools/BCG_Vaccine_rates_information_sheet.pdf
155. Oliver G, Eaton CA, Campbell N. Interactions between aeromonas salmonicida and peritoneal macrophages of brook trout (Salvelinus fontinalis). Vet Immunol Immunopathol. (1986) 12:223–34. doi: 10.1016/0165-2427(86)90126-1
156. Kato G, Kondo H, Aoki T, Hirono I. BCG vaccine confers adaptive immunity against Mycobacterium sp. infection in fish. Dev Comp Immunol. (2010) 34:133–40. doi: 10.1016/j.dci.2009.08.013
157. Kato G, Kondo H, Aoki T, Hirono I. Mycobacterium bovis BCG vaccine induces non-specific immune responses in Japanese flounder against nocardia seriolae. Fish Shellfish Immunol. (2012) 33:243–50. doi: 10.1016/j.fsi.2012.05.002
158. Kato G, Kato K, Saito K, Pe Y, Kondo H, Aoki T, et al. Vaccine efficacy of Mycobacterium bovis BCG against Mycobacterium sp. infection in amberjack seriola dumerili. Fish Shellfish Immunol. (2011) 30:467–72. doi: 10.1016/j.fsi.2010.11.002
159. Lorenzen N, Lorenzen E, Einer-Jensen K, LaPatra SE. Immunity induced shortly after DNA vaccination of rainbow trout against rhabdoviruses protects against heterologous virus but not against bacterial pathogens. Dev Comp Immunol. (2002) 26:173–9. doi: 10.1016/S0145-305X(01)00059-3
160. Sommerset I, Lorenzen E, Lorenzen N, Bleie H, Nerland AH. A DNA vaccine directed against a rainbow trout rhabdovirus induces early protection against a nodavirus challenge in turbot. Vaccine. (2003) 21:4661–7. doi: 10.1016/S0264-410X(03)00526-7
161. Kollmann TR, Kampmann B, Mazmanian SK, Marchant A, Levy O. Protecting the newborn and young infant from infectious diseases: lessons from immune ontogeny. Immunity. (2017) 46:350–63. doi: 10.1016/j.immuni.2017.03.009
162. Tsafaras GP, Ntontsi P, Xanthou G. Advantages and limitations of the neonatal immune system. Front Pediatr. (2020) 8:5. doi: 10.3389/fped.2020.00005
163. Callens B, Persoons D, Maes D, Laanen M, Postma M, Boyen F, et al. Prophylactic and metaphylactic antimicrobial use in belgian fattening pig herds. Prev Vet Med. (2012) 106:53–62. doi: 10.1016/j.prevetmed.2012.03.001
164. Lekagul A, Tangcharoensathien V, Yeung S. Patterns of antibiotic use in global pig production: a systematic review. Vet Anim Sci. (2019) 7:100058. doi: 10.1016/j.vas.2019.100058
165. Chase CC, Hurley DJ, Reber AJ. Neonatal immune development in the calf and its impact on vaccine response. Vet Clin North Am Food Anim Pract. (2008) 24:87–104. doi: 10.1016/j.cvfa.2007.11.001
166. Miles DG. Overview of the North American beef cattle industry and the incidence of bovine respiratory disease (BRD). Anim Health Res Rev. (2009) 10:101–3. doi: 10.1017/S1466252309990090
167. Drackley JK. Biology of dairy cows during the transition period: the final frontier? J Dairy Sci. (1999) 82:2259–73. doi: 10.3168/jds.S0022-0302(99)75474-3
168. Sordillo LM, Contreras GA, Aitken SL. Metabolic factors affecting the inflammatory response of periparturient dairy cows. Anim Health Res Rev. (2009) 10:53–63. doi: 10.1017/S1466252309990016
169. Zhang Z, Swain T, Bøgwald J, Dalmo RA, Kumari J. Bath immunostimulation of rainbow trout (Oncorhynchus mykiss) fry induces enhancement of inflammatory cytokine transcripts, while repeated bath induce no changes. Fish Shellfish Immunol. (2009) 26:677–84. doi: 10.1016/j.fsi.2009.02.014
170. Dalmo RA, Kjerstad AA, Arnesen SM, Tobias PS, Bogwald J. Bath exposure of atlantic halibut (Hippoglossus hippoglossus L.) yolk sac larvae to bacterial lipopolysaccharide (LPS): absorption and distribution of the LPS and effect on fish survival. Fish Shellfish Immunol. (2000) 10:107–28. doi: 10.1006/fsim.1999.0231
171. Bricknell I, Dalmo R. The use of immunostimulants in fish larval aquaculture. Fish Shellfish Immunol. (2005) 19:457–72. doi: 10.1016/j.fsi.2005.03.008
172. Wang S, Wang Y, Ma J, Ding Y, Zhang S. Phosvitin plays a critical role in the immunity of zebrafish embryos via acting as a pattern recognition receptor and an antimicrobial effector. J Biol Chem. (2011) 286:22653–64. doi: 10.1074/jbc.M111.247635
173. Hara A, Hiramatsu N, Fujita T. Vitellogenesis and choriogenesis in fishes. Fish Sci. (2016) 82:187–202. doi: 10.1007/s12562-015-0957-5
174. Mulero I, García-Ayala A, Meseguer J, Mulero V. Maternal transfer of immunity and ontogeny of autologous immunocompetence of fish: a minireview. Aquaculture. (2007) 268:244–50. doi: 10.1016/j.aquaculture.2007.04.046
175. Berghof TV, Parmentier HK, Lammers A. Transgenerational epigenetic effects on innate immunity in broilers: an underestimated field to be explored? Poult Sci. (2013) 92:2904–13. doi: 10.3382/ps.2013-03177
176. Beemelmanns A, Roth O. Grandparental immune priming in the pipefish syngnathus typhle. BMC Evol Biol. (2017) 17:44. doi: 10.1186/s12862-017-0885-3
177. Beemelmanns A, Roth O. Biparental immune priming in the pipefish syngnathus typhle. Zoology. (2016) 119:262–72. doi: 10.1016/j.zool.2016.06.002
178. Slocombe RF, Malark J, Ingersoll R, Derksen FJ, Robinson NE. Importance of neutrophils in the pathogenesis of acute pneumonic pasteurellosis in calves. Am J Vet Res. (1985) 46:2253–8.
179. Caverly JM, Radi ZA, Andreasen CB, Dixon RA, Brogden KA, Ackermann MR. Comparison of bronchoalveolar lavage fluid obtained from mannheimia haemolytica-inoculated calves with and without prior treatment with the selectin inhibitor TBC1269. Am J Vet Res. (2001) 62:665–72. doi: 10.2460/ajvr.2001.62.665
180. Radi ZA, Caverly JM, Dixon RA, Brogden KA, Ackermann MR. Effects of the synthetic selectin inhibitor TBC1269 on tissue damage during acute mannheimia haemolytica-induced pneumonia in neonatal calves. Am J Vet Res. (2001) 62:17–22. doi: 10.2460/ajvr.2001.62.17
181. Hagglund S, Blodorn K, Naslund K, Vargmar K, Lind SB, Mi J, et al. Proteome analysis of bronchoalveolar lavage from calves infected with bovine respiratory syncytial virus-Insights in pathogenesis and perspectives for new treatments. PLoS ONE. (2017) 12:e0186594. doi: 10.1371/journal.pone.0186594
182. Rauw W. Immune response from a resource allocation perspective. Front Genet. (2012) 3:267. doi: 10.3389/fgene.2012.00267
183. Iseri VJ, Klasing KC. Changes in the amount of lysine in protective proteins and immune cells after a systemic response to dead escherichia coli: implications for the nutritional costs of immunity. Integr Comp Biol. (2014) 54:922–30. doi: 10.1093/icb/icu111
184. Sordillo LM. Nutritional strategies to optimize dairy cattle immunity1. J Dairy Sci. (2016) 99:4967–82. doi: 10.3168/jds.2015-10354
Keywords: trained innate immunity, veterinary species, disease resistance, beta-glucans, innate memory
Citation: Byrne KA, Loving CL and McGill JL (2020) Innate Immunomodulation in Food Animals: Evidence for Trained Immunity? Front. Immunol. 11:1099. doi: 10.3389/fimmu.2020.01099
Received: 06 March 2020; Accepted: 06 May 2020;
Published: 05 June 2020.
Edited by:
Jayne Hope, University of Edinburgh, United KingdomReviewed by:
Gregers Jungersen, Statens Serum Institute, DenmarkLonneke Vervelde, University of Edinburgh, United Kingdom
Copyright © 2020 Byrne, Loving and McGill. This is an open-access article distributed under the terms of the Creative Commons Attribution License (CC BY). The use, distribution or reproduction in other forums is permitted, provided the original author(s) and the copyright owner(s) are credited and that the original publication in this journal is cited, in accordance with accepted academic practice. No use, distribution or reproduction is permitted which does not comply with these terms.
*Correspondence: Jodi L. McGill, amxtY2dpbGxAaWFzdGF0ZS5lZHU=