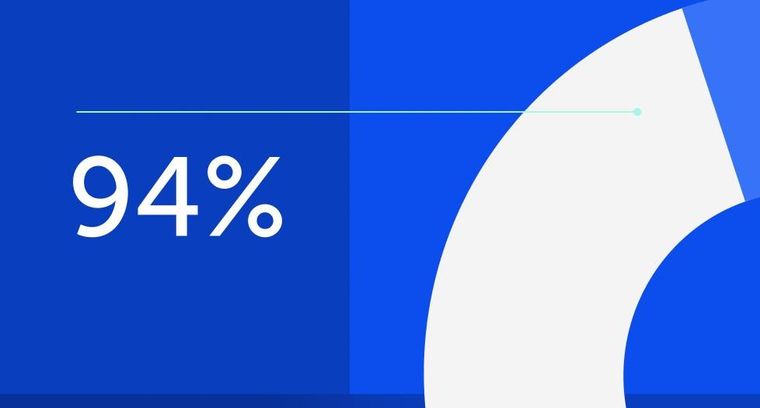
94% of researchers rate our articles as excellent or good
Learn more about the work of our research integrity team to safeguard the quality of each article we publish.
Find out more
REVIEW article
Front. Immunol., 12 May 2020
Sec. Multiple Sclerosis and Neuroimmunology
Volume 11 - 2020 | https://doi.org/10.3389/fimmu.2020.00689
This article is part of the Research TopicImmune Response to Cerebral Ischemia: Exploring Mechanisms and Potential Treatment TargetsView all 20 articles
Stroke is a disease that occurs due to a sudden interruption of the blood supply to the brain. It is a leading cause of death and disability worldwide. It is well-known that the immune system drives brain injury following an episode of ischemic stroke. The innate system and the adaptive system play distinct but synergistic roles following ischemia. The innate system can be activated by damage-associated molecular patterns (DAMPs), which are released from cells in the ischemic region. Damaged cells also release various other mediators that serve to increase inflammation and compromise the integrity of the blood–brain barrier (BBB). Within 24 h of an ischemic insult, the adaptive immune system is activated. This involves T cell and B cell-mediated inflammatory and humoral effects. These cells also stimulate the release of various interleukins and cytokines, which can modulate the inflammatory response. The adaptive immune system has been shown to contribute to a state of immunodepression following an ischemic episode, and this can increase the risk of infections. However, this phenomenon is equally important in preventing autoimmunity of the body to brain antigens that are released into the peripheral system as a result of BBB compromise. In this review, we highlight the key components of the adaptive immune system that are activated following cerebral ischemia.
Stroke occurs due to a compromise of the blood supply to a particular region of the brain leading to permanent neurological deficits (1) such as weakness, sensory deficits, visual field defects, and aphasia (2). Approximately 85% of all cases of stroke are of the ischemic type, and it is the third leading cause of death in the United States (3).
The ischemic cascade is initiated following the onset of stroke. It is characterized by a loss of adenosine triphosphate (ATP) leading to ion pump failure, accumulation of intracellular calcium, and glutamate-induced excitotoxicity of cells (4). In addition to the central neuronal response, there is activation of immune responses in the injured tissue. The cells most sensitive to ischemic damage in the brain are neurons (5). Dying neuronal and non-neuronal cells release damage associated molecular patterns (DAMPs) such as high-mobility group box 1 (HMGB1) and heat shock proteins (HSPs) (Figure 1). These activate resident cells such as microglia (6), which alongside other pro-inflammatory mediators contribute to disruption of the blood–brain barrier (BBB). Once the BBB has been compromised, there is a prolonged flux of systemic inflammatory cells such as monocytes, neutrophils, and T cells into the injured area, which further exacerbates the injury (Figure 2).
Figure 1. Timeline of immune response in ischemic stroke. Ischemic stroke begins with a cascade of events as a result of arterial occlusion leading to hypoxia accompanied by ROS production within minutes and glucose deprivation. The integrity of the BBB is compromised leading to increased permeability within 1–2 h, allowing infiltration of circulatory cells which contribute to the inflammatory process and exacerbate neuronal death. BBB, blood–brain barrier; ROS, reactive oxygen species; MMPs, matrix metalloproteinases; DAMPs, damage-associated molecular patterns; ECM, extracellular matrix.
Figure 2. Innate immunity in ischemic stroke. Immediately following an episode of stroke, the innate immune system is activated. It is characterized by the presence of various mediators such as DAMPs that have been released from the injured neurons and which induce the secondary activation of microglia via TLRs signaling and NLRP3 activity. TLR, Toll-like receptors; NLRP3, nod-like receptor pyrin domain-containing 3; APC, antigen-presenting cell; DAMPs, damage-associated molecular patterns.
Activation of T and B cells is a key component of the adaptive immune system and occurs within 24 h following injury (7). T cell activation occurs following recognition of T cells by the T cell receptor and engagement of costimulatory molecules such as cluster of differentiation (CD) 28 with B7 or those from the tumor necrosis factor (TNF) receptor family such as CD137 (TNFRSF9, 4-1BB) (8). The adaptive system appears to play a beneficial role following cerebral ischemia. However, there is also evidence that activation of the adaptive system may exacerbate the ischemic injury and contribute to systemic immune suppression, leading to increased susceptibility to infections (9). A recent study showed that CD137 costimulation is associated with increased systemic inflammation following cerebral ischemia and may exert deleterious effects (10).
Although advances in the field have been made and are continuing to evolve, our understanding of the process is by far from complete. In this review, we highlight the key components of the adaptive immune system in cerebral ischemia and discuss potential therapeutic targets.
The key cells involved in the adaptive immune system are T cells and B cells. T cells are divided into CD8+ cytotoxic T cells and CD4+ T helper (Th) cells. Most T cells are of the alpha beta (αβ) type, and the remaining are of the gamma delta (γδ) type. The presence of these cells in the healthy brain is limited and regulated by the intact BBB (11); however, following an episode of ischemia, they rapidly infiltrate the diseased brain (Figure 3) (12).
Figure 3. Adaptive immunity in ischemic stroke. Within 1 day of the ischemic event, there is infiltration of system cells such as CD4+ T and CD8+ T cells. CD4 cell cells differentiate into Th1, Th2, Th17, or Tregs to produce pro-inflammatory or anti-inflammatory effects. CD8+ T cells lead to neuronal death by release of perforin and granzyme. B cells produce anti-inflammatory effects via the release of interleukins such as IL-10. TLR, Toll-like receptors; NLRP3, nod-like receptor pyrin domain-containing 3; APC, antigen-presenting cell; CD4+, cluster of differentiation 4+; Th, T helper; Treg, regulatory T cell.
The first cells of the adaptive immune system to migrate to the ischemic region are the CD8+ cytotoxic cells, which can be seen as early as a few hours following stroke (12) and are usually abundant between 1 and 7 days post injury (13).
Between 1 and 2 days post ischemia, T cells have been observed in the subpial region, and by day 7, they can be seen in the edges of the ischemic region (14). T cells reduce in number by day 14 (14) but have been observed in the peri-infarct area up to 1 month following injury (15, 16). T cell activation markers CD44 and CD25 and pro-inflammatory cytokines are also present in the ischemic region (15). Infiltration of CD4+ cells has been observed within 24 h after the onset of ischemia (17). Regulatory T cells (Tregs) have been observed a few days after the onset of ischemia and can persist for more than 30 days (18, 19). Interestingly, the time-dependent increase of T cells can differ by sex, with higher levels of CD8+ T cells and Tregs observed at day 15 in aged male mice compared to females despite a similar pattern of ischemic brain injury (20).
CD8+ lymphocytes cells are widely found in the ischemic penumbra (15). CD8+ T cells may play deleterious roles in the ischemic infarct, with evidence of reduced infarct size and improved neurological outcome following induced cerebral ischemia in CD8+ knockout models (21) and antibody depletion models (22). CD8+ T cell damage may lead to direct neuronal cytotoxicity via a number of pathways such as the Fas ligand (FasL) pathway, with evidence of reduced neuronal cell death following use of FasL and 3-phosphoinositide-dependent protein kinase 1 (PDPK) inhibitors (23). CD8+ T cell-induced neuronal damage can also occur via humoral pathways by releasing inflammatory mediators such as interferon gamma (IFN-γ) (24). It is unclear whether CD8+ T cells are a major source of IFN-γ, and there is conflicting evidence of whether IFN-γ plays a pivotal (21) or a minimal role in the evolution of the infarct under normal conditions (25). CD8+ cells have been shown to release various other cytokines such as interleukin 16 (IL-16), which have been found in the ischemic penumbra. IL-16 serves to recruit and activate immune cells and can lead to blood vessel and BBB damage. They have been found to accumulate in the necrotic lesion and can reach maximum levels 3–4 days after ischemia (26). In addition to releasing pro-inflammatory cytokines, cytotoxic CD8+ T cells can also mediate their actions via direct cytolytic pathways by releasing cytotoxic proteins such as granzymes and perforin (22). Cerebral ischemia is associated with white matter injury.
A major pathobiological change seen following white matter brain injury is demyelination. Interestingly, demyelination is closely related to T cell-mediated changes. It has recently been shown that cytotoxic CD8+ T cells may worsen white matter injury and demyelination in cerebral ischemia. Given that CD8+ differentiation can be affected by IL-2 homeostasis; one group showed that IL-2 monoclonal antibody can preserve white matter integrity following experimental ischemia and therefore may be a useful therapeutic target (27).
Gamma delta (γδ) T cells represent a small subset of T cells. The majority of these cells are activated in a major histocompatibility complex (MHC)-independent manner, in contrast to the classic MHC-restricted αβ T cells. γδ T cells play a role in both the innate immune system and the adaptive immune system following an episode of stroke. They have been detected in infarcts at 6 h post ischemia. They can secrete IL-17 and mediate the infiltration of pro-inflammatory cytokines (28, 29). IL-17 works synergistically with TNF-α to induce neutrophil infiltration via chemokines such as chemokine (C-X-C motif) ligand 1 (CXCL-1). Blocking IL-17 or CXCL-1 has been shown to reduce infarct size (30). However, IL-17-mediated effects are short lived and therefore may not be responsible for the prolonged inflammation seen after ischemia.
Studies investigating the role of T cells in ischemic stroke have predominantly focused on infiltrating CD3+CD8+ and CD3+CD4+ T cells (31). However, the role of double-negative T cells (DNTs), which account for 1–5% of the total T cell population, has seldom been investigated (32). This is perhaps because the function of DNTS in the normal brain is not fully known. All T cells begin as a double-negative cell and subsequently rearrange into the common CD4+/CD8+ forms. In a mouse model of cerebral ischemia, it was demonstrated that DNTs infiltrate the brain 1–3 days after ischemia and are located near activated microglia. DNT-derived TNF-α was shown to contribute to activation of microglia via the FasL pathway, and TNF-α expression in DNTs was regulated by T cell protein tyrosine phosphatase (PTPN2). Targeting the FasL/PTPN2/TNF-α signaling pathway may therefore be an attractive option in treating ischemic stroke (33). However, the role of DNTs in the ischemic brain is not fully understood, and certainly their role in both the innate and the adaptive systems including their interactions with other cells and cytokine release profile need to be further explored.
CD4+ T cells are divided into conventional Th cells and Tregs. Th cells play a crucial role in the adaptive immune system. There are two effector CD4+ Th cell responses that can be induced, and these are designated Th1 and Th2. The Th1 response is characterized by the production of IFN-γ, which has been shown to create a delayed immune response to stroke. Blocking the IFN-γ signaling pathway reduces inflammatory chemokine IFN-inducible protein 10 (IP-10) expression and decreases neurodegeneration (34) following middle cerebral artery occlusion (MCAO). Levels of IFN-γ and IP-10 are also reduced following CD4 T cell depletion 3 days after stroke onset, and this is associated with improved behavioral outcomes (35). However, other studies have shown that mice deficient in IFN-γ developed infarcts similar to control (25).
The presence of Th2 cells can lead to a humoral immune response characterized by the release of various ILs such as IL-10. IL-10 has been shown to play a central immunomodulatory role and can reduce infarct volume. In the MCAO model, IL-10−/− C57BL/6 mice were shown to have 30% larger infarct volumes compared with wild-type mice 24 h following a stroke (36). IL-10 mediates its anti-inflammatory effect by downregulating various pathways such as the nuclear factor kappa-light-chain enhancer of activated B cells (NF-κB). One study demonstrated improved BBB integrity in the cerebral ischemia–reperfusion model using hydrogen sulfide donors, and this was accompanied by enhanced IL-10 expression and reduced NF-κB nuclear translocation (37). The extent of the impact of Th2 cytokines ischemic sequelae in mice subjected to MCAO is however unclear (38).
Various pro-inflammatory mediators are elevated in CD4+ T cells following ischemia. A recent study showed increased expression of soluble CD137 on CD4+ T cells in peripheral blood of patients following an episode of stroke compared to controls (39) and may therefore be a potential therapeutic target. Various novel immune modulatory targets of cerebral ischemia have been recently investigated. One study investigated the role of acetyl coenzyme A carboxylase 1 (ACC1), an enzyme that mediates fatty acid synthesis and CD4+ T cell-associated inflammation. Following transient MCAO, there was evidence of increased fatty acid synthesis and expression of ACC1 in CD4+ T cells. Inhibition of ACC1 depletion was associated with reduced neuro-inflammation, preservation of peripheral Tregs/Th17 cells and improved neurological outcome following ischemia (40).
Regulatory T cells (Tregs) are a type of CD4+ cells that can suppress the proliferation of CD4+ and CD8+ T cells and maintain self-tolerance (41). The most well-understood of the naturally occurring Tregs are those that express CD4, CD25, and forkhead/winged-helix transcription factor box P3 (FOXP3). However, these markers are not specific for Tregs and are in fact T-cell activation markers (41). Tregs have been found in both normal healthy brains and also following an ischemic episode up to 30 days post injury (18).
Whether Tregs are a core component of the adaptive immune system or the innate immune system is the subject of much controversy, Liesz et al. showed that endogenous Tregs are protective in later stages of stroke (42) and that their beneficial functions depend on IL-10 (28). However, a recent study suggests that Tregs have an early detrimental role by inducing dysfunction of the cerebral microcirculation (37) and are therefore more important for the innate system. Tregs can induce microvascular dysfunction via the lymphocyte function-associated antigen 1/intercellular adhesion molecule (LFA-1/ICAM-1) pathway, and ablation of Tregs can improve cerebral reperfusion in stroke (43).
Many studies however show that adoptive transfer of Tregs can improve outcome following stroke (18), with worse neurological outcomes and larger infarcts seen in ischemic mice with Treg depletion (42). A number of different mechanisms of Treg-mediated neuroprotection exist. These include Treg-induced increase in IL-16 (44) and reduction in inflammatory mediators such as NF-κB (45). Tregs can also suppress matrix metalloproteinase 9 (MMP9) production in neutrophils and thereby prevent breakdown of the BBB (46, 47). Despite Tregs being an attractive target in ischemic stroke, their low frequency limits their clinical use. Effective methods to expand Tregs in vivo are therefore desirable.
The IL-2/IL-2 antibody complex (IL-2/IL-2Ab) has been shown to increase the number of Tregs and promote the expression of CD39 and CD73 in expanded Tregs in experimental ischemia models. This is associated with a reduced infarct size, improved neurological outcome. Tregs can be expanded using IL-33 (48), which can improve outcomes following ischemia by reducing IFN-γ+ T cells and increasing Foxp3+ T cells in the spleen (49). However, the beneficial effects of IL-33 may be mitigated by an increased rate of respiratory infections (50). Brain Tregs express neural cell-specific genes such as the serotonin receptor (Htr7) and can be modulated with serotonin or selective serotonin reuptake inhibitors (SSRIs) to reduce neurological symptoms (51). Another method of Treg expansion is the use of poly (ADP-ribose) polymerase-1 (PARP-1) inhibitors, which has been shown to increase the proportion of Tregs in peripheral blood mononuclear cells (PBMCs), reduce pro-inflammatory cytokines (IFN-γ, TNF-α, and IL-17), and increase inflammatory cytokines [IL-4, IL-10, and transforming growth factor β1 (TGF-β1)] (52). The signaling protein sirtuin is known to reduce T cell activation and is a negative regulator of Treg function (53). Cerebral ischemia is associated with increased expression of sirtuin in infiltrating Tregs, and this is mediated by the transcription factor, hypoxia-inducible factor 1-alpha (HIF-1α) (54), which can be modulated to expand Treg numbers.
There are various Th cells which are closely related to Tregs and are involved in cerebral ischemia. These include Th17 cells, which produce IL-17. Th17 cells mediate the recruitment of neutrophils and macrophages to damaged tissues. These cells are thought to be the preferential producers of iIL-17A, IL-17F, IL-21, and IL-22. IL-17 can also be produced by other cells such as neuroglial cells (11) and γδ T lymphocytes (55).
In a study of patients with ischemic stroke, IL-17A was found to be significantly expressed at day 7 following a stroke. It continues to be present up to day 28, although significantly less than day 7. It must be noted however that patients were taking various anti-inflammatory medications from day 7 onward, which may have affected the results. Levels of Tregs followed an opposite pattern and were markedly reduced in patients at day 7 but increased after day 28. The interaction between IL-17A and Tregs and its significance are not fully understood (56).
The mechanism of IL-17-induced damage following ischemia is not fully understood; however, one study demonstrated evidence of increased activation of the calpain-transient receptor potential canonical (subtype) 6 (TRPC6) signaling pathway, which is known to be involved in cerebral ischemia (47). TRPC6 is a cation channel that protects neurons from excitotoxicity and ischemic damage. Cerebral ischemia is associated with intracellular calcium overload leading to activation of calpain, which hydrolyzes TRPC6. Suppression of TRPC6 degradation may therefore be useful in cerebral ischemia to preserve neurons. However, there are many other mechanisms of calpain-mediated downregulation of TRPC6 contributing to ischemic brain injury and therefore without accounting for other factors, it is difficult to measure the significance of IL-17-mediated calpain damage (56).
B Cells are the key players in humoral immunity. Upon activation, B cells produce antibodies, which recognizes specific antigens. The role of B cells in cerebral ischemia is inconclusive. Some found a beneficial role of B cells (57, 58). Whereas, others found no impact on infarct volume and functional outcome (21, 31, 59).
The beneficial effects of B cells include reduced infarct volume compared to the control group (60). B cells can also limit the production of cytokines such as IFN-γ and TNF-α and infiltration of inflammatory T cells (57). Cerebral ischemia is associated with splenic atrophy, which is associated with a reduction of inflammatory cytokines, T cells and B cells. The profound loss of B cell limits the ability of the humoral immune system to provide protection, and this contributes to systemic immunosuppression (61). Splenectomy, on the other hand, can reduce the pool of immune cells and rats splenectomized 2 weeks before experimental ischemia show reduced infarction volume in the brain (62).
A number of studies have shown that B cells may not influence infarct size and outcome in ischemic models. Mice homozygous for the Rag1−/− mutation which produce no mature T cells or B cells did not have reduced infarct size following ischemia compared to wild type. Interestingly, Rag1−/− mice supplemented with T cells developed significant ischemic brain damage (63).
Natural killer (NK) cells are key players of the innate immune system; however, they also have attributes of the adaptive system. Following an ischemic episode, there is an increased amount of NK cells found in brain tissues (64) within 3 h, with peak levels between days 3 and 5 (64). NK cells modulate adaptive immunity via cytokine production such as IFN-γ, which can induce neuronal necrosis in cerebral ischemia. NK cells can also modulate the adaptive immune response via the perforin-granzyme-mediated cytolytic pathway. Granzymes and perforin are stored in the cytoplasmic granules of NK cells and when released can augment local inflammation (64). Additional evidence of NK cells being a part of the adaptive immune system in cerebral ischemia are from an observed increase of circulating NK cells in patients with post-stroke infections suggesting that they have a role in post-stroke immunodepression (64). NK cells are therefore an attractive target in cerebral ischemia. However, it is not clear how modulating NK cells would affect infarct evolution or immunodepression. Furthermore, NK cells are well-known players of the innate immune system, and therefore the timing of NK cell modulation may play a crucial role in its effect on the ischemic infarct.
Following an episode of stroke, there is a breakdown of the BBB, which allows antigens from the CNS to migrate into the systemic circulation to be recognized by the peripheral immune system and modulate an inflammatory response. Many researchers have exploited this mechanism to induce immunological tolerance to a specific antigen such as MBP. MBP is a protein which increases in the early period after acute ischemic stroke (65). Using an induced oral tolerance to antigen model, it was shown that cell-mediated Th1 immune responses are involved following an episode of stroke. In an experimental MCAO model, rats were fed MBP 3 h before the procedure. This is a common model to induce immunological tolerance to a specific antigen by feeding of that antigen, which can lead to clonal depletion of antigen-reactive T cells and cause active tolerance. Upon re-stimulation with the antigen, the T cells in the animal which has been tolerized can secrete cytokines such as TGF-β1, and this can then suppress Th1 immune response and lead to deviation toward a humoral Th2 response. This leads to bystander immunosuppression, where the activation of T cells led to non-specific effects despite being activated in an antigen-specific manner (66, 67). Immunological tolerance to antigens such as MBP could therefore decrease cerebral infarction via the bystander suppression phenomena. Indeed, in the MCAO model, rats that were fed MBP had reduced infarct size up to 4 days post procedure compared to control. Immunohistochemistry revealed increased levels of TGF-β1 production by T cells in the brain, suggesting that modulation of the antigen-specific modulation can decrease infarct size (68). MCAO however is also associated with an autoimmune response to the brain MBP. One month after MCAO, lymphocytes from spleens of MBP tolerized animals show a regulatory response (Treg) toward MBP compared to control. Rats which demonstrated an inflammatory response (Th1) had worse neurological outcome (69). However, the benefit of this form of therapy does not extend beyond 3 months after MCAO, which could be due to induction of mucosal tolerance to MBP only serving to delay recovery. Mucosal administration of antigen may also lead to detrimental autoimmunity (70).
E-selectin (CD62E) is a cell adhesion molecule, which is activated following an inflammatory insult that can guide tolerized Tregs to blood vessels where they can release cytokines to inhibit the development of ischemic stroke (71). E-selectin is distributed in the vascular endothelium and can serve as an antigenic target for Treg guidance to vessel segments. E-selectin has been shown to reduce infarct size in the MCAO stroke model. Rats tolerized to nasally administered E-selectin had a reduction in brain infarct volume compared with controls. A similar effect was seen following adoptive transfer of splenocytes from E-selectin-tolerized donors (72). Th1 cells are generally regarded as mediators of delayed-type hypersensitivity (DTH) reactions. E-selectin-specific DTH responses were significantly suppressed in E-selectin-tolerized animals. Suppression of the Th1-mediated DTH reaction to E-selectin provides evidence for the generation of antigen-specific Tregs in rats tolerized to E-selectin. These findings demonstrate cell-mediated immunomodulation as the basis for the observed cytoprotection in this MCAO stroke model (72).
A recent study demonstrates that cerebral ischemia induces varied neuroantigen-mediated T cell responses, which can exacerbate the injury. Brain ischemia induces diversified neuroantigen-specific T cell responses that exacerbate brain injury. This was confirmed using adoptive transfer of neuroantigen-experienced MOG35−55 (myelin oligodendrocyte glycoprotein) epitope (2D2) which worsened brain injury by inducing Th1/Th17 responses (73).
The adaptive immune system plays a significant role in post-stroke immunodepression and susceptibility to infection. During this period, there is evidence of a shift from cell-mediated inflammatory Th1 type response to a humoral-mediated anti-inflammatory Th2 type response. This occurs to protect the brain from further damage. However, this is in detriment to the body's systemic immune system, which is suppressed and therefore there is increased risk of developing systemic infections (74). There is increased Th2 cytokine production and reduction of T, B, and NK lymphocytes, TNF-α, and IFN-γ. Administration of IFN-γ at day 1 after stroke however can reduce the development of infection (75).
Three days following induced cerebral ischemia in mice, there is evidence of increased incidence of infections such as pneumonia, a major cause of mortality. Stroke-induced immunosuppression directly affects pulmonary immunity and leads to an increased risk of bacterial infection. Following an episode of stroke, there is increased percentage of alveolar macrophages and neutrophils and reduced amount of CD4+ T cells, CD8+ T cells, B cells, NK cells, and eosinophils in the lungs (76). CD147 or extracellular matrix metalloproteinase inducer (EMMPRIN) is a transmembrane glycoprotein and a member of the immunoglobulin superfamily, which is upregulated in the lungs following stroke. Inhibition of CD147 leads to reduced pulmonary edema, and this is associated with IL-17A expression in lung γδ T cells. It may therefore be a promising therapeutic adjunct in stroke-associated pneumonia (77).
Bacterial infections are also thought to be increased in response to activation of the sympathetic nervous system (SNS) and the hypothalamic–pituitary–adrenal (HPA) axis (Figure 4). Noradrenaline (NA), the main neurotransmitter of the SNS, can regulate immune cell development (78). Plasma levels of NA are elevated following ischemia, and this is associated with a higher incidence of infections (79). Studies have shown that reduced IFN-γ response and associated infections following ischemia can be prevented by targeted inhibition of SNS signaling (75, 80, 81). It is not possible to inhibit the entire SNS or the HPA axis in patients, and therefore the extent to which these pathways are clinically relevant needs to be further elucidated in animal models.
Figure 4. Immunodepression after ischemic stroke. Stroke-induced immunodepression leads to an increased incidence of infections. HPA, hypothalamic–pituitary–adrenal axis; SNS, sympathetic nervous system; PNS, parasympathetic nervous system; Treg, regulatory T cell.
Despite significant advances in the field, the role of adaptive immunity in cerebral ischemia is far from being well-understood. The greatest concern is the observed opposing effects of cells and mediators seen in different experimental models, limiting their applicability to humans. Although many groups use the MCAO method to induce ischemia, the wide variations in inflammatory responses between rodents and humans remain an issue. Furthermore, the presence of other comorbidities which may have an effect on the inflammatory system cannot be replicated in rodents, and this itself raises questions regarding the generalization of the data. Another key factor is the vast interactions between immune cells which cannot be replicated in a single study. This has implications for application to patients, as there needs to be a fine balance between inducing the beneficial aspects of the immune system while preventing deleterious effects. The greatest challenge therefore is identifying and modulating the most significant immune target in cerebral ischemia.
The brain is an immune-privileged organ due to the presence of the BBB. However, during an episode of ischemia, the integrity of this BBB is compromised. Cells of the adaptive immune system play varied roles within an ischemic episode. There is clear evidence of CD8+ cells migrating into the infarcted region following ischemia; this serves to increase inflammation with release of numerous cytokines and ILs. However, CD4+ cells and Tregs are also increased in the infarcted region and can play a protective role. Ischemic stroke is associated with post-stroke immunodepression, and this is known to be associated with increased incidence of infection. However, it is also required to prevent stroke-related autoimmunity. Following ischemia, CNS antigens enter the peripheral circulation and leukocytes to enter the brain. This can increase the likelihood of developing an autoimmune response to the brain after a stroke. Post-stroke immunodepression is therefore an adaptive response to acute cerebral ischemia and is required to reduce the likelihood of developing autoimmunity to CNS antigens.
XQ, RL, and SW designed the paper and recommended a structure for the review. XQ and FA wrote the initial draft and revised the manuscript. Figures and submission were prepared by LQ, JC, and MG. SY, ZJ, and SW helped to revise the manuscript.
This work was supported by the Science Foundation for Youth Scholars of Wuhan University (2042019kf0095) and China Scholarship Council.
The authors declare that the research was conducted in the absence of any commercial or financial relationships that could be construed as a potential conflict of interest.
1. Hossmann K-A. Pathophysiology and therapy of experimental stroke. Cell Mol Neurobiol. (2006) 26:1057–83. doi: 10.1007/s10571-006-9008-1
2. Musuka TD, Wilton SB, Traboulsi M, Hill MD. Diagnosis and management of acute ischemic stroke: speed is critical. CMAJ. (2015) 187:887–93. doi: 10.1503/cmaj.140355
3. Beal C. Gender and Stroke Symptoms: a review of the current literature. J Neurosci Nurs. (2010) 42:80–7. doi: 10.1097/JNN.0b013e3181ce5c70
4. Taxin ZH, Neymotin SA, Mohan A, Lipton P, Lytton WW. Modeling molecular pathways of neuronal ischemia. Prog Mol Biol Transl Sci. (2014) 123:249–75. doi: 10.1016/B978-0-12-397897-4.00014-0
5. Taoufik E, Probert L. Ischemic neuronal damage. Curr Pharmac Design. (2008) 14:F3565–73. doi: 10.2174/138161208786848748
6. Gülke E, Gelderblom M, Magnus T. Danger signals in stroke and their role on microglia activation after ischemia. Ther Adv Neurol Disord. (2018) 11:1756286418774254. doi: 10.1177/1756286418774254
7. Brait VH, Arumugam TV, Drummond GR, Sobey CG. Importance of T lymphocytes in brain injury, immunodeficiency, and recovery after Cerebral Ischemia. J Cereb Blood Flow Metab. (2012) 32:598–611. doi: 10.1038/jcbfm.2012.6
8. Esensten JH, Helou YA, Chopra G, Weiss A, Bluestone JA. CD28 costimulation: from mechanism to therapy. Immunity. (2016) 44:973–88. doi: 10.1016/j.immuni.2016.04.020
9. Van de Beek D, Wijdicks EFM, Vermeij FH, de Haan RJ, Prins JM, Spanjaard L, et al. Preventive antibiotics for infections in acute stroke: a systematic review and meta-analysis. Arch Neurol. (2009) 66:1076–81. doi: 10.1001/archneurol.2009.176
10. Li XQ, Wang YY, Yang TT, Qian YN, Yin H, Zhong SS, et al. Increased Peripheral CD137 expression in a mouse model of permanent focal cerebral ischemia. Cell Mol Neurobiol. (2019) 39:451–60. doi: 10.1007/s10571-019-00661-z
11. Engelhardt B, Sorokin L. The blood-brain and the blood-cerebrospinal fluid barriers: function and dysfunction. Semin Immunopathol. (2009) 31:497–511. doi: 10.1007/s00281-009-0177-0
12. Liesz A, Zhou W, Mracskó É, Karcher S, Bauer H, Schwarting S, et al. Inhibition of lymphocyte trafficking shields the brain against deleterious neuroinflammation after stroke. Brain. (2011) 134:704–20. doi: 10.1093/brain/awr008
13. Li G-Z, Zhong D, Yang L-M, Sun B, Zhong Z-H, Yin Y-H, et al. Expression of interleukin-17 in ischemic brain tissue. Scand J Immunol. (2005) 62:481–6. doi: 10.1111/j.1365-3083.2005.01683.x
14. Ortolano F, Maffia P, Dever G, Rodolico G, Millington OR, De Simoni MG, et al. Advances in imaging of new targets for pharmacological intervention in stroke: real-time tracking of T-cells in the ischaemic brain. Br J Pharmacol. (2010) 159:808–11. doi: 10.1111/j.1476-5381.2009.00527.x
15. Schroeter M, Jander S, Witte OW, Stoll G. Local immune responses in the rat cerebral cortex after middle cerebral artery occlusion. J Neuroimmunol. (1994) 55:195–203. doi: 10.1016/0165-5728(94)90010-8
16. Xie L, Li W, Hersh J, Liu R, Yang S-H. Experimental ischemic stroke induces long-term T cell activation in the brain. J Cereb Blood Flow Metab. (2019) 39:2268–76. doi: 10.1177/0271678X18792372
17. Chu HX, Kim HA, Lee S, Moore JP, Chan CT, Vinh A, et al. Immune cell infiltration in malignant middle cerebral artery infarction: comparison with transient cerebral ischemia. J Cereb Blood Flow Metab. (2014) 34:450–9. doi: 10.1038/jcbfm.2013.217
18. Stubbe T, Ebner F, Richter D, Engel O, Randolf Engel O, Klehmet J, et al. Regulatory T cells accumulate and proliferate in the ischemic hemisphere for up to 30 days after MCAO. J Cereb Blood Flow Metab. (2013) 33:37–47. doi: 10.1038/jcbfm.2012.128
19. Doyle KP, Quach LN, Solé M, Axtell RC, Nguyen T-VV, Soler-Llavina GJ, et al. B-lymphocyte-mediated delayed cognitive impairment following stroke. J Neurosci. (2015) 35:2133–45. doi: 10.1523/JNEUROSCI.4098-14.2015
20. Ahnstedt H, Patrizz A, Chauhan A, Roy-O'Reilly M, Furr JW, Spychala MS, et al. Sex differences in T cell immune responses, gut permeability and outcome after ischemic stroke in aged mice. Brain Behav Immun. (2020) S0889-1591:31459-X. doi: 10.1016/j.bbi.2020.02.001
21. Yilmaz G, Arumugam TV, Stokes KY, Granger DN. Role of T lymphocytes and interferon-gamma in ischemic stroke. Circulation. (2006) 113:2105–12. doi: 10.1161/CIRCULATIONAHA.105.593046
22. Mracsko E, Liesz A, Stojanovic A, Lou WP-K, Osswald M, Zhou W, et al. Antigen dependently activated cluster of differentiation 8-positive T cells cause perforin-mediated neurotoxicity in experimental stroke. J Neurosci. (2014) 34:16784–95. doi: 10.1523/JNEUROSCI.1867-14.2014
23. Fan L, Zhang CJ, Zhu L, Chen J, Zhang Z, Liu P, et al. FasL-PDPK1 pathway promotes the cytotoxicity of CD8+ T cells during ischemic stroke. Transl Stroke Res. (2020) doi: 10.1007/s12975-019-00749-0
24. Harty JT, Tvinnereim AR, White DW. CD8+ T cell effector mechanisms in resistance to infection. Annu Rev Immunol. (2000) 18:275–308. doi: 10.1146/annurev.immunol.18.1.275
25. Lambertsen KL, Gregersen R, Meldgaard M, Clausen BH, Heibøl EK, Ladeby R, et al. A role for interferon-gamma in focal cerebral ischemia in mice. J Neuropathol Exp Neurol. (2004) 63:942–55. doi: 10.1093/jnen/63.9.942
26. Schwab JM, Nguyen TD, Meyermann R, Schluesener HJ. Human focal cerebral infarctions induce differential lesional interleukin-16 (IL-16) expression confined to infiltrating granulocytes, CD8+ T-lymphocytes and activated microglia/macrophages. J Neuroimmunol. (2001) 114:232–41. doi: 10.1016/s0165-5728(00)00433-1
27. Zhou YX, Wang X, Tang D, Li Y, Jiao YF, Gan Y, et al. IL-2mAb reduces demyelination after focal cerebral ischemia by suppressing CD8+ T cells. CNS Neurosci Ther. (2019) 25:532–43. doi: 10.1111/cns.13084
28. Born WK, Jin N, Aydintug MK, Wands JM, French JD, Roark CL, et al. gammadelta T lymphocytes-selectable cells within the innate system? J Clin Immunol. (2007) 27:133–44. doi: 10.1007/s10875-007-9077-z
29. Gelderblom M, Weymar A, Bernreuther C, Velden J, Arunachalam P, Steinbach K, et al. Neutralization of the IL-17 axis diminishes neutrophil invasion and protects from ischemic stroke. Blood. (2012) 120:3793–802. doi: 10.1182/blood-2012-02-412726
30. Gelderblom M, Arunachalam P, Magnus T. γδ T cells as early sensors of tissue damage and mediators of secondary neurodegeneration. Front Cell Neurosci. (2014) 8:368. doi: 10.3389/fncel.2014.00368
31. Kleinschnitz C, Schwab N, Kraft P, Hagedorn I, Dreykluft A, Schwarz T, et al. Early detrimental T-cell effects in experimental cerebral ischemia are neither related to adaptive immunity nor thrombus formation. Blood. (2010) 115:3835–42. doi: 10.1182/blood-2009-10-249078
32. Ford MS, Chen W, Wong S, Li C, Vanama R, Elford AR, et al. Peptide-activated double-negative T cells can prevent autoimmune type-1 diabetes development. Eur J Immunol. (2007) 37:2234–41. doi: 10.1002/eji.200636991
33. Meng H, Zhao H, Cao X, Hao J, Zhang H, Liu Y, et al. Double-negative T cells remarkably promote neuroinflammation after ischemic stroke. Proc Natl Acad Sci USA. (2019) 116:5558–63. doi: 10.1073/pnas.1814394116
34. Seifert HA, Collier LA, Chapman CB, Benkovic SA, Willing AE, Pennypacker KR. Pro-inflammatory interferon gamma signaling is directly associated with stroke induced neurodegeneration. J Neuroimmune Pharmacol. (2014) 9:679–89. doi: 10.1007/s11481-014-9560-2
35. Harris NM, Roy-O'Reilly M, Ritzel RM, Holmes A, Sansing LH, O'Keefe LM, et al. Depletion of CD4 T cells provides therapeutic benefits in aged mice after ischemic stroke. Exp Neurol. (2020) 326:113202. doi: 10.1016/j.expneurol.2020.113202
36. Grilli M, Barbieri I, Basudev H, Brusa R, Casati C, Lozza G, et al. Interleukin-10 modulates neuronal threshold of vulnerability to ischaemic damage. Eur J Neurosci. (2000) 12:2265–2272. doi: 10.1046/j.1460-9568.2000.00090.x
37. Wang Y, Jia J, Ao G, Hu L, Liu H, Xiao Y, et al. Hydrogen sulfide protects blood-brain barrier integrity following cerebral ischemia. J Neurochem. (2014) 129:827–38. doi: 10.1111/jnc.12695
38. Perego C, Fumagalli S, Miteva K, Kallikourdis M, De Simoni MG. Combined Genetic Deletion of IL (Interleukin)-4, IL-5, IL-9, and IL-13 Does Not Affect Ischemic Brain Injury in Mice. Stroke. (2019) 50:2207–15. doi: 10.1161/STROKEAHA.119.025196
39. He Y, Ao DH, Li XQ, Zhong SS, Wang YY, Xiang YJ, et al. Increased Soluble CD137 levels and CD4+ T-cell-associated expression of CD137 in acute atherothrombotic stroke. Clin Transl Sci. (2018) 11:428–34. doi: 10.1111/cts.12553
40. Wang X, Zhou Y, Tang D, Zhu Z, Li Y, Huang T, et al. ACC1 (Acetyl Coenzyme A Carboxylase 1) Is a Potential Immune Modulatory Target of Cerebral Ischemic Stroke. Stroke. (2019) 50:1869–78. doi: 10.1161/STROKEAHA.119.024564
41. Bodhankar S, Chen Y, Lapato A, Vandenbark AA, Murphy SJ, Saugstad JA, et al. Regulatory CD8(+)CD122 (+) T-cells predominate in CNS after treatment of experimental stroke in male mice with IL-10-secreting B-cells. Metab Brain Dis. (2015) 30:911–24. doi: 10.1007/s11011-014-9639-8
42. Liesz A, Suri-Payer E, Veltkamp C, Doerr H, Sommer C, Rivest S, et al. Regulatory T cells are key cerebroprotective immunomodulators in acute experimental stroke. Nat Med. (2009) 15:192–9. doi: 10.1038/nm.1927
43. Kleinschnitz C, Kraft P, Dreykluft A, Hagedorn I, Göbel K, Schuhmann MK, et al. Regulatory T cells are strong promoters of acute ischemic stroke in mice by inducing dysfunction of the cerebral microvasculature. Blood. (2013) 121:679–91. doi: 10.1182/blood-2012-04-426734
44. Neal EG, Acosta SA, Kaneko Y, Ji X, Borlongan CV. Regulatory T-cells within bone marrow-derived stem cells actively confer immunomodulatory neuroprotective effects against stroke. J. Cereb. Blood Flow Metab. (2019) 39:1750–8. doi: 10.1177/0271678X18766172
45. Zhao Y, Zhu T, Li H, Zhao J, Li X. Transplantation of lymphocytes co-cultured with human cord blood-derived multipotent stem cells attenuates inflammasome activity in ischemic stroke. Clin Interv Aging. (2019) 14:2261–71. doi: 10.2147/CIA.S223595
46. Li P, Gan Y, Sun B-L, Zhang F, Lu B, Gao Y, et al. Adoptive regulatory T-cell therapy protects against cerebral ischemia. Ann Neurol. (2013) 74:458–71. doi: 10.1002/ana.23815
47. Zhang J, Mao X, Zhou T, Cheng X, Lin Y. IL-17A contributes to brain ischemia reperfusion injury through calpain-TRPC6 pathway in mice. Neuroscience. (2014) 274:419–28. doi: 10.1016/j.neuroscience.2014.06.001
48. Guo S, Luo Y. Brain Foxp3+ regulatory T cells can be expanded by Interleukin-33 in mouse ischemic stroke. Int Immunopharmacol. (2019) 81:106027. doi: 10.1016/j.intimp.2019.106027
49. Xiao W, Guo S, Chen L, Luo Y. The role of Interleukin-33 in the modulation of splenic T-cell immune responses after experimental ischemic stroke. J Neuroimmunol. (2019) 333:576970. doi: 10.1016/j.jneuroim.2019.576970
50. Zhang Y, Wei L, Du Y, Xie Y, Wu W, Yuan Y. Association between programed cell death-1 and CD4+ T cell alterations in different phases of ischemic stroke patients. Front Cell Neurosci. (2018) 12:170. doi: 10.3389/fncel.2018.00170
51. Ito M, Komai K, Nakamura T, Srirat T, Yoshimura A. Tissue regulatory T cells and neural repair. Int Immunol. (2019) 31:361–9. doi: 10.1093/intimm/dxz031
52. Noh MY, Lee WM, Lee SJ, Kim HY, Kim SH, Kim YS. Regulatory T cells increase after treatment with poly (ADP-ribose) polymerase-1 inhibitor in ischemic stroke patients. Int Immunopharmacol. (2018) 60:104–10. doi: 10.1016/j.intimp.2018.04.043
53. Zou T, Yang Y, Xia F, Huang A, Gao X, Fang D, et al. Resveratrol inhibits CD4+ T cell activation by enhancing the expression and activity of Sirt1. PLoS ONE. (2013) 8:e75139. doi: 10.1371/journal.pone.0075139
54. Shu L, Xu CQ, Yan ZY, Yan Y, Jiang SZ, Wang YR. Post-stroke microglia induce Sirtuin2 expression to suppress the anti-inflammatory function of infiltrating regulatory T cells. Inflammation. (2019) 42:1968–79. doi: 10.1007/s10753-019-01057-3
55. Shichita T, Sugiyama Y, Ooboshi H, Sugimori H, Nakagawa R, Takada I, et al. Pivotal role of cerebral interleukin-17-producing gammadeltaT cells in the delayed phase of ischemic brain injury. Nat Med. (2009) 15:946–50. doi: 10.1038/nm.1999
56. Hu Y, Zheng Y, Wu Y, Ni B, Shi S. Imbalance between IL-17A-producing cells and regulatory T cells during ischemic stroke. Mediat Inflamm. (2014) 2014:813045. doi: 10.1155/2014/813045
57. Du W, Huang J, Yao H, Zhou K, Duan B, Wang Y. Inhibition of TRPC6 degradation suppresses ischemic brain damage in rats. J Clin Invest. (2010) 120:3480–92. doi: 10.1172/JCI43165
58. Ren X, Akiyoshi K, Dziennis S, Vandenbark AA, Herson PS, Hurn PD, et al. Regulatory B cells limit CNS inflammation and neurologic deficits in murine experimental stroke. J Neurosci. (2011) 31:8556–63. doi: 10.1523/JNEUROSCI.1623-11.2011
59. Offner H, Hurn PD. A novel hypothesis: regulatory B Lymphocytes shape outcome from experimental stroke. Transl Stroke Res. (2012) 3:324–30. doi: 10.1007/s12975-012-0187-4
60. Schuhmann MK, Langhauser F, Kraft P, Kleinschnitz C. B cells do not have a major pathophysiologic role in acute ischemic stroke in mice. J Neuroinflammation. (2017) 14:112. doi: 10.1186/s12974-017-0890-x
61. Bodhankar S, Chen Y, Vandenbark AA, Murphy SJ, Offner H. Treatment of experimental stroke with IL-10-producing B-cells reduces infarct size and peripheral and CNS inflammation in wild-type B-cell-sufficient mice. Metab Brain Dis. (2014) 29:59–73. doi: 10.1007/s11011-013-9474-3
62. Offner H, Subramanian S, Parker SM, Wang C, Afentoulis ME, Lewis A, et al. Splenic atrophy in experimental stroke is accompanied by increased regulatory T cells and circulating macrophages. J Immunol. (2006) 176:6523–31. doi: 10.4049/jimmunol.176.11.6523
63. Ajmo CT, Vernon DOL, Collier L, Hall AA, Garbuzova-Davis S, Willing A, et al. The spleen contributes to stroke-induced neurodegeneration. J Neurosci Res. (2008) 86:2227–34. doi: 10.1002/jnr.21661
64. Gan Y, Liu Q, Wu W, Yin J-X, Bai X-F, Shen R, et al. Ischemic neurons recruit natural killer cells that accelerate brain infarction. Proc Natl Acad Sci USA. (2014) 111:2704–9. doi: 10.1073/pnas.1315943111
65. Chen C, Ai Q-D, Chu S-F, Zhang Z, Chen N-H. NK cells in cerebral ischemia. Biomed Pharmacother. (2019) 109:547–54. doi: 10.1016/j.biopha.2018.10.103
66. Lee GA, Lin T-N, Chen C-Y, Mau S-Y, Huang W-Z, Kao Y-C, et al. Interleukin 15 blockade protects the brain from cerebral ischemia-reperfusion injury. Brain Behav Immun. (2018) 73:562–70. doi: 10.1016/j.bbi.2018.06.021
67. Lamers KJB, Vos P, Verbeek MM, Rosmalen F, van Geel WJA, van Engelen BGM. Protein S-100B, neuron-specific enolase (NSE), myelin basic protein (MBP) and glial fibrillary acidic protein (GFAP) in cerebrospinal fluid (CSF) and blood of neurological patients. Brain Res Bull. (2003) 61:261–4. doi: 10.1016/s0361-9230(03)00089-3
68. Miller A, Lider O, Weiner HL. Antigen-driven bystander suppression after oral administration of antigens. J Exp Med. (1991) 174:791–8. doi: 10.1084/jem.174.4.791
69. Becker KJ, McCarron RM, Ruetzler C, Laban O, Sternberg E, Flanders KC, et al. Immunologic tolerance to myelin basic protein decreases stroke size after transient focal cerebral ischemia. Proc Natl Acad Sci USA. (1997) 94:10873–8. doi: 10.1073/pnas.94.20.10873
70. Gee JM, Kalil A, Thullbery M, Becker KJ. Induction of immunologic tolerance to myelin basic protein prevents central nervous system autoimmunity and improves outcome after stroke. Stroke. (2008) 39:1575–82. doi: 10.1161/STROKEAHA.107.501486
71. Gee JM, Zierath D, Hadwin J, Savos A, Kalil A, Thullbery M, et al. Long term immunologic consequences of experimental stroke and mucosal tolerance. Exp Transl Stroke Med. (2009) 1:3. doi: 10.1186/2040-7378-1-3
72. Takeda H, Spatz M, Ruetzler C, McCarron R, Becker K, Hallenbeck J. Induction of mucosal tolerance to E-selectin prevents ischemic and hemorrhagic stroke in spontaneously hypertensive genetically stroke-prone rats. Stroke. (2002) 33:2156–63. doi: 10.1161/01.str.0000029821.82531.8b
73. Chen Y, Ruetzler C, Pandipati S, Spatz M, McCarron RM, Becker K, et al. Mucosal tolerance to E-selectin provides cell-mediated protection against ischemic brain injury. Proc Natl Acad Sci USA. (2003) 100:15107–12. doi: 10.1073/pnas.2436538100
74. Jin WN, Gonzales R, Feng Y, et al. Brain ischemia induces diversified neuroantigen-specific T-cell responses that exacerbate brain injury. Stroke. (2018) 49:1471–8. doi: 10.1161/STROKEAHA.118.020203
75. Dirnagl U, Klehmet J, Braun JS, Harms H, Meisel C, Ziemssen T, et al. Stroke-induced immunodepression: experimental evidence and clinical relevance. Stroke. (2007) 38:770–3. doi: 10.1161/01.STR.0000251441.89665.bc
76. Prass K, Meisel C, Höflich C, Braun J, Halle E, Wolf T, et al. Stroke-induced immunodeficiency promotes spontaneous bacterial infections and is mediated by sympathetic activation reversal by poststroke T helper cell type 1-like immunostimulation. J Exp Med. (2003) 198:725–36. doi: 10.1084/jem.20021098
77. Farris BY, Monaghan KL, Zheng W, Amend CD, Hu H, Ammer AG, et al. Ischemic stroke alters immune cell niche and chemokine profile in mice independent of spontaneous bacterial infection. Immun Inflamm Dis. (2019) 7:326–41. doi: 10.1002/iid3.277
78. Jin R, Liu S, Wang M, Zhong W, Li G. Inhibition of CD147 attenuates stroke-associated pneumonia through modulating lung immune response in mice. Front Neurol. (2019) 10:853. doi: 10.3389/fneur.2019.00853
79. Ordovas-Montanes J, Rakoff-Nahoum S, Huang S, Riol-Blanco L, Barreiro O, von Andrian UH. The regulation of immunological processes by peripheral neurons in homeostasis and disease. Trends Immunol. (2015) 36:578–604. doi: 10.1016/j.it.2015.08.007
80. Walter U, Kolbaske S, Patejdl R, Steinhagen V, Abu-Mugheisib M, Grossmann A, et al. Insular stroke is associated with acute sympathetic hyperactivation and immunodepression. Eur J Neurol. (2013) 20:153–9. doi: 10.1111/j.1468-1331.2012.03818.x
Keywords: cerebral ischemia, stroke, immune response, innate immunity, adaptive immunity
Citation: Qin X, Akter F, Qin L, Cheng J, Guo M, Yao S, Jian Z, Liu R and Wu S (2020) Adaptive Immunity Regulation and Cerebral Ischemia. Front. Immunol. 11:689. doi: 10.3389/fimmu.2020.00689
Received: 05 October 2019; Accepted: 26 March 2020;
Published: 12 May 2020.
Edited by:
Creed Stary, Stanford University, United StatesReviewed by:
Jorge Tolivia, University of Oviedo, SpainCopyright © 2020 Qin, Akter, Qin, Cheng, Guo, Yao, Jian, Liu and Wu. This is an open-access article distributed under the terms of the Creative Commons Attribution License (CC BY). The use, distribution or reproduction in other forums is permitted, provided the original author(s) and the copyright owner(s) are credited and that the original publication in this journal is cited, in accordance with accepted academic practice. No use, distribution or reproduction is permitted which does not comply with these terms.
*Correspondence: Xingping Qin, cXhwNzE4QHdodS5lZHUuY24=; Renzhong Liu, bGl1cmVuemhvbmdAd2h1LmVkdS5jbg==; Songlin Wu, d3Vzb25nbGluQHdodS5lZHUuY24=
†These authors have contributed equally to this work
Disclaimer: All claims expressed in this article are solely those of the authors and do not necessarily represent those of their affiliated organizations, or those of the publisher, the editors and the reviewers. Any product that may be evaluated in this article or claim that may be made by its manufacturer is not guaranteed or endorsed by the publisher.
Research integrity at Frontiers
Learn more about the work of our research integrity team to safeguard the quality of each article we publish.