- Divisions of Neonatology and Developmental Biology, David Geffen School of Medicine at the University of California Los Angeles, Los Angeles, CA, United States
Acute chorioamnionitis is characterized by neutrophilic infiltration and inflammation at the maternal fetal interface. It is a relatively common complication of pregnancy and can have devastating consequences including preterm labor, maternal infections, fetal infection/inflammation, fetal lung, brain, and gastrointestinal tract injury. In this review, we will discuss current understanding of the pathogenesis, immunobiology, and mechanisms of this condition. Most commonly, acute chorioamnionitis is a result of ascending infection with relatively low-virulence organisms such as the Ureaplasma species. Furthermore, recent vaginal microbiome studies suggest that there is a link between vaginal dysbiosis, vaginal inflammation, and ascending infection. Although less common, microorganisms invading the maternal-fetal interface via hematogenous route (e.g., Zika virus, Cytomegalovirus, and Listeria) can cause placental villitis and severe fetal inflammation and injury. We will provide an overview of the knowledge gleaned from different animal models of acute chorioamnionitis and the role of different immune cells in different maternal-fetal compartments. Lastly, we will discuss how infectious agents can break the maternal tolerance of fetal allograft during pregnancy and highlight the novel future therapeutic approaches.
Introduction
Intrauterine infection or inflammation (IUI), also known as chorioamnionitis, is responsible for ~40% of preterm labor cases (1). Prematurity, which affects nearly 10% of pregnancies world-wide, is the most significant cause of perinatal mortality or morbidity (2).
In this paper, we explore the current knowledge of the mechanisms of IUI. In particular, we review how inflammation is propagated in different tissue compartments at the maternal-fetal interface, the role of resident cells interacting with immune cells at the interface, the role of inflammatory mediators, and how host-microbe interactions affect pathology. Although sterile inflammation (3, 4), environmental pollutants (5–7), cigarette smoke (8, 9), and other toxicants play an important role in the pathogenesis of IUI, these considerations are beyond the scope of this review.
Intraamniotic infection is an infection with resultant inflammation of any combination of the amniotic fluid (AF), placenta, fetus, fetal membranes, or decidua. The amniotic sac is composed of maternal (decidua) and fetal components (chorion and amniotic membranes) which surround the fetus and represent one site of maternal/fetal immune interaction. The amnion is a fetal tissue comprising a layer of epithelial cells and underlying mesenchymal cells, and an extracellular matrix and collagen that has a high tensile strength. The chorion is composed of a reticular layer, basement membrane, and trophoblasts. The decidua, the transformed maternal endometrium of pregnancy, is in direct proximity with the chorion and consists of maternal immune cells, decidual stromal cells, and extravillous fetal trophoblasts (10). Placental villous and intervillous space have a specialized architecture adapted for nutrient exchange and have distinct immune cells. The cellular interactions within these layers and in the placenta are important in coordinating the immune response for maintaining a semi-allogeneic fetus (11, 12).
Intraamniotic infection and chorioamnionitis are commonly used interchangeably; however, these two conditions are not the same, as we will discuss below. Furthermore, chorioamnionitis can be induced by sterile damage-associated molecules (13, 14).
Clinical Definitions
Most often, the diagnosis of chorioamnionitis is made clinically based on the presence of fever, uterine tenderness, maternal leukocytosis, purulent cervical drainage, or fetal tachycardia (15). However, due to the vague nature of the definition and heterogeneity of clinical manifestations, an NIH expert panel proposed to replace the term “chorioamnionitis,” with a more general, descriptive term, “Intrauterine Inflammation and/or Infection,” abbreviated as Triple I (16). In this scheme, fever alone during labor is classified separately, while “suspected Triple I” is classified as fever with one or more of the following symptoms: leukocytosis, fetal tachycardia, or purulent cervical discharge. In order to be confirmed, “suspected Triple I" should be accompanied by AF infection (e.g., positive gram stain for bacteria, low AF glucose, high WBC count in the absence of a bloody tap, and/or positive AF culture results) or histopathological evidence of infection/inflammation in the placenta, fetal membranes or the umbilical cord vessels (funisitis).
Anatomy of Fetal Membranes
There are four fetal membranes early in fetal life: the chorion, amnion, yolk sac, and allantois. The chorion and amnion are derived from trophoblastic ectoderm and extraembryonic somatic mesoderm. The yolk sac and allantois are derived from endoderm and extraembryonic splanchnic mesoderm. In humans, the yolk sac degenerates with fetal growth while the allantois is vestigial and may regress, but the blood vessels persist as umbilical arteries connect the embryo with the placenta (17). The reproductive tissues of mammals have many features in common but there are unique species-associated characteristics. For example, the development of fetal membranes in rodents is unique to those species and there are significant architectural differences between rodent and human placenta, although both have hemochorial placentation (18). Specifically, rodents have an inverted yolk sac placenta, where the fetal endoderm lies between the maternal tissue and the mesoderm, while in other species the fetal mesoderm lies between the ectoderm and endoderm (17, 19).
Histopathological Definitions
Acute inflammation characterized by the infiltration of neutrophils in the chorion and/or amnion is termed acute chorioamnionitis (1), and can involve the placental and/or extraplacental fetal membranes. “Maternal inflammation” refers to the infiltration of largely maternal neutrophils and macrophages in the fetal tissues of the chorion and amnion (Figure 1). Inflammatory processes involving the umbilical cord (umbilical vein, umbilical artery, and the Wharton's jelly) are referred to as acute funisitis, and constitutes fetal inflammation or fetal inflammatory response syndrome (FIRS). Placental inflammation affecting the villous tree is called acute villitis. A widely used classification by Redline (20) further divided the maternal and fetal inflammatory response into stages and grades. The term “stage” refers to the progression of the inflammatory process based on the anatomical regions infiltrated by neutrophils; the term “grade” refers to the intensity of the acute inflammatory process at a particular site. Interestingly, the characteristic location of initial neutrophil infiltration is the choriodecidual junction, with invasion into the amnion denoting higher stages of inflammation. The incidence of histologic chorioamnionitis is inversely related to the gestational age at preterm delivery (defined as delivery <37 weeks' gestation) (21). Interestingly, histologic chorioamnionitis is diagnosed in >70% of preterm births occurring at <28 weeks' gestation (22, 23) (Figure 2). The precise reasons for different rates of chorioamnionitis at different gestational ages are not clear. One possibility is gestational dependence of immune function (24). Studies have shown that the expression of innate immune receptors [e.g., Toll-like receptors (TLRs)] in the placenta (24, 25) and fetal membranes are increased after 20 weeks of pregnancy (26). The vast majority of preterm deliveries occur in the late third trimester with medically indicated preterm deliveries contributing to ~30% of cases (22). This may also decrease the proportion of prematurity attributable to infection/inflammation during the late third trimester.
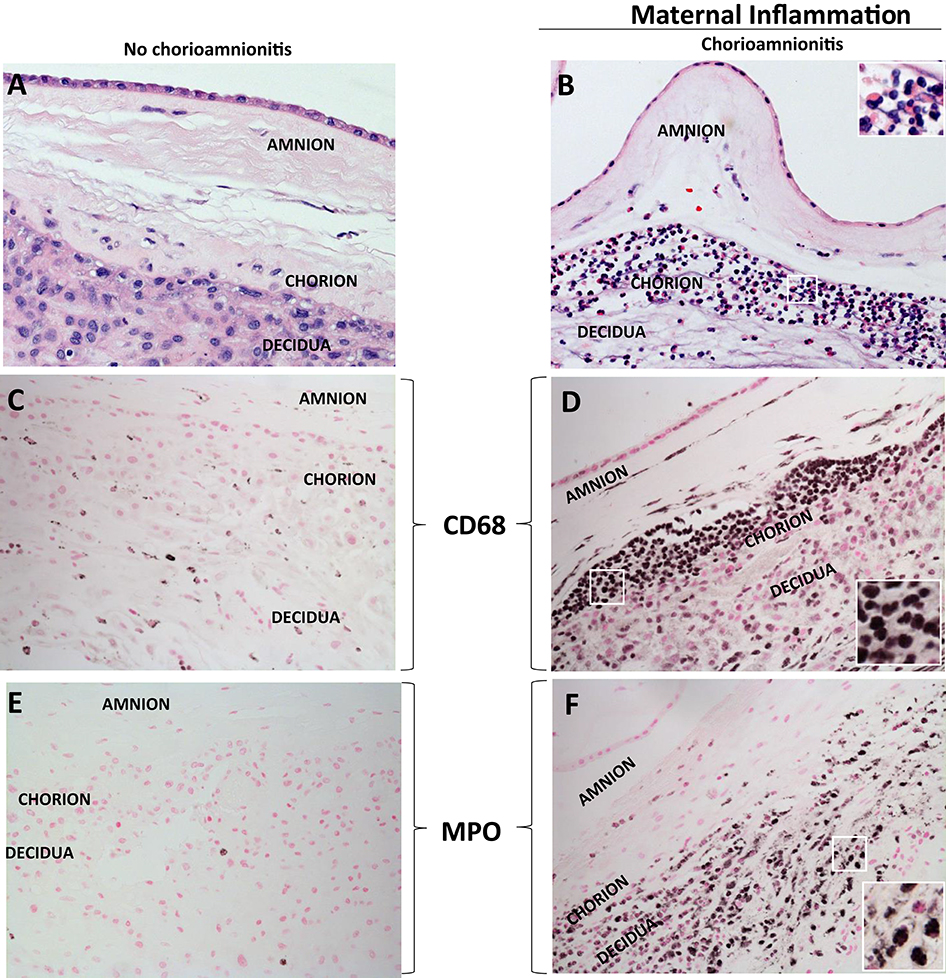
Figure 1. H&E histology of intrauterine inflammations. (A,B) Cross sections of human fetal membranes H&E histology showing neutrophil infiltration. Chorioamnionitis is characterized by infiltration of (D) CD68+ macrophages and (F) neutrophils expressing Myeloperoxidase+ (MPO) predominantly located at the choriodecidua junction. Note relatively much fewer CD68 or MPO expressing cells in the no chorioamnionitis group (C,E). Insets in (B,D,F) show higher power magnification of demarcated area in white and demonstrate inflammatory cells including neutrophils and macrophages.
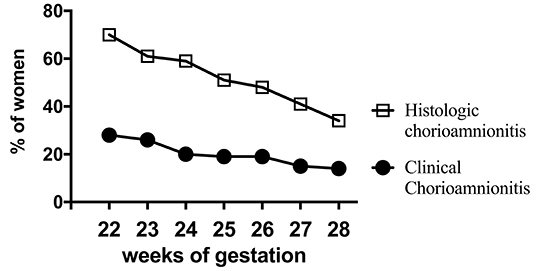
Figure 2. Chorioamnionitis during second trimester. Higher documentation of histologic vs. clinically diagnosed chorioamnionitis in the same mothers whose Infants were born at 22–28 weeks Gestational Age (GA) in the NICHD funded Neonatal Network database (2003-2007). Also note that chorioamnionitis is more frequently diagnosed at earlier gestations (inverse correlation of gestational age with incidence of chorioamnionitis). Adapted from Stoll et al. (23).
Microbiology
Ascending Infections
The most common route for microbes to cause intrauterine infection is by ascending from the lower genital tract (1). The most frequent microorganisms found in the amniotic cavity are genital mycoplasmas, in particular the Ureaplasma species (27). Of note, Group B Streptococcus (GBS) colonizes the genitourinary tract in 20–30% of pregnant women (28) and is associated with chorioamnionitis, preterm premature rupture of amniotic membrane (PPROM) and preterm birth (29–31). Other common organisms include Gardenella vaginalis, Fusobacteria species (32–35), and E. coli (36). Although not reported frequently, fungi can also cause chorioamnionitis. Among the fungi, Candida species, particularly C. albicans (37, 38) and less commonly C. glabrata (39, 40) have been reported. Unlike invasive infections in other parts of the body, polymicrobial invasion of the amniotic cavity is present in ~30% of cases (41).
Hematogenous Infections
In a minority of cases, microorganisms can also invade the placenta by the hematogenous route, and the profile of organisms is different compared to the ascending route. Microorganisms that invade the placenta by the hematogenous route include Listeria monocytogenes (42), Zika virus (43, 44), Treponema pallidum (45), Cytomegalovirus (46), Plasmodium species (47), and microorganisms causing toxoplasmosis, syphilis, varicella-zoster, parvovirus B19, Rubella, and Herpes infections (TORCH) (48). These organisms gain access through the maternal circulation to the intervillous space, from where they invade the villi and fetal circulation. The oral pathogen Fusobacter nucleatum can also cause IUI through the hematogenous route (49). Indeed, bad oral hygiene and multiple different oral pathogens have been implicated in IUI and preterm births, although a randomized controlled trial of treatment of periodontal disease did not reduce adverse pregnancy outcomes (50). In contrast to the ascending infections causing inflammation primarily in the choriodecidua and amnion, organisms invading through the hematogenous route cause inflammation primarily in the placental villi and intervillous space.
Influenza Infection
Pregnant women are at increased risk of developing severe disease with seasonal influenza, being hospitalized at a rate of 1–2/1,000, a risk that is 18-fold greater than that for healthy non-pregnant women (51–55). Severe maternal infections such as influenza—particularly pandemic infections (e.g., 1918 and 2009 pandemics and 2005 avian influenza), can lead to still birth and preterm delivery, although the precise mechanisms of the disease are not well-understood (56). Influenza vaccines are one of the most effective interventions. An epidemiological study suggests that vaccination against influenza virus protects from preterm birth (57, 58). Generally, viruses may impact maternal and fetal health by infecting gestational tissues and modulating intrauterine immune responses (59, 60). However, whether influenza virus infection can cause chorioamnionitis is not known.
Priming Infections
Viral infections, such as influenza virus, can prime or accentuate bacterial infection-mediated preterm labor and the intensity of inflammatory response at the maternal-fetal interface (61–66). Specifically, pathogen/pathogen-associated molecular pattern-driven activation of type I Interferon (IFN)/IFN receptor (IFNAR) was sufficient to prime for systemic and uterine proinflammatory chemokine and cytokine production and induction of preterm birth in mice (67). The synergy during combined stimulation of different Toll-like receptors (TLRs) is considered to be a “two-hit hypothesis” (68). These findings might explain how subclinical type I IFN-producing systemic infections (either virus or bacteria) act as initial inflammatory triggers and increase susceptibility for secondary inflammatory challenge-driven adverse pregnancy outcomes (67).
Vaginal Microbiome
Bacterial vaginosis (BV), a dysbiotic state of the vagina, is known to be a risk factor for prematurity (69). Recent metagenomic studies have shed light on normal composition of vaginal microorganisms during the non-pregnant state and during pregnancy in women of different racial groups. Compared to the gastrointestinal (GI) microbiome, the vaginal microbiota has much less alpha-diversity (different microbial species within the vaginal ecosystem) (70). Notably, the vaginal microbiota has a Lactobacillus predominance during pregnancy, different from the GI microbiota, but the vaginal microbiota becomes more similar to the GI microbiota during the postpartum period with less Lactobacillus predominance (70). Five different profiles of vaginal community states in non-pregnant women of reproductive age were described (71). Type I profile is dominated by L. crispatus, type II is dominated by L. gasseri, type III is dominated by L. iners, type IV-A or IV-B are characterized by high relative abundance of species of genus Atopobium, Prevotella, Sneathia, Gardnerella, Ruminococcaceae, Parvimonas, Mobiluncus, and other taxa previously shown to be associated with bacterial vaginosis. Type V is dominated by L. jensenii (71). Compared to vaginal microbiota of non-pregnant women, the vaginal microbiota during pregnancy tends to be relatively stable with a dominance of Lactobacilli species (72). Recent microbiota studies have clarified that vaginal Lactobacillus deficiency, particularly L. crispatum deficiency and dominance of community state type IV accompanied by elevated Gardnerella or Ureaplasma abundances (vaginal dysbiosis) predisposes to prematurity (70, 73). Furthermore, dysbiotic organisms have higher transcriptomic activity in women delivering preterm compared to those delivering at term (74). Lactobacillus predominance is important because it is protective for increased vaginal microbiological diversity, and growth of type IV organisms. Prematurity was associated with increased abundance of pro-inflammatory cytokines in vaginal wash (74), and dysbiotic vaginal flora was associated with preterm premature rupture of membranes (PPROM), chorioamnionitis, funisitis, and early onset neonatal sepsis (73). Thus, vaginal dysbiosis appears to cause local vaginal inflammation and increase the likelihood of an ascending infection and chorioamnionitis, leading to increased risk for prematurity. Studies in different racial groups have shown that there are significant racial variations in the vaginal microbiota. The association between the lower frequency of Lactobacillus and higher Gardnerella with increased risk for PTB was demonstrated in Caucasian but not African American women. While a lower abundance of Lactobacillus crispatus was associated with low risk of preterm birth in both cohorts, the presence of Lactobacillus iners was not associated with the risk for PTB (75). Thus, more work is needed to understand how vaginal flora may play a role in ascending infection and prematurity in different racial groups.
Compartments Within the Intrauterine Space and Pathways of Microbial Invasion
The intrauterine space has several sub-compartments that although contiguous have distinct immune and functional roles. The sub-compartments include the amniotic fluid, fetus, choriodecidua, amnion, villous placenta, and the uterus. One particular cytokine, IL-6, was identified as a critical marker of intrauterine inflammation (76) and a predictor of preterm birth (77). Experimental evidence supports the crucial role of IL-6 during parturition. In mice, IL-6 deficiency delayed normal parturition (78), and protected from inflammation-driven preterm birth (67). Normally, intrauterine infection is mainly a localized inflammatory process. In humans, IL-6 levels are >100-fold higher in the amniotic fluid compared to the maternal or fetal blood during IUI (79, 80). This information is important in interpreting the relevance of animal models of intrauterine infection induced by intraperitoneal LPS in mice, which causes systemic inflammation rather than amniotic fluid inflammation. Data in the non-human primate demonstrate that infectious agents confined to the choriodecidua induce preterm labor at a much lower frequency compared to infection in the amniotic fluid (81–83). Our data demonstrate that macromolecules such as cytokines (IL1ra) do not efficiently cross the amniotic epithelial barrier but readily cross the placenta from the mother to the fetus (84). Thus, the evaluation of compartment-specific mechanisms of inflammation is important. Indeed, differentially expressed genes in spontaneous preterm birth compared to gestational controls had different transcriptomic profiles in different tissues (85). These results are consistent with different tissue-specific roles in the intrauterine space. In a prospective study with detailed phenotyping of preterm and term labor, the largest difference in gene expression groups occurred at the maternal fetal-interface in the decidua, chorion, and amnion (86).
During intrauterine infections, ascending organisms are thought to spread diffusely through the choriodecidual or the chorioamnion plane and then invade the amniotic cavity. However, a study using molecular microbiologic techniques in human placentae demonstrated that the initial event is a localized choriodecidual infection, which then invades the amniotic cavity, infecting the amniotic fluid and fetus prior to causing diffuse choriodecidual inflammation (87).
Animal Models
Several animal models have been reported for IUI (see Table 1 with representative references for each model). Each model has some advantages but also important limitations. Mice are commonly used in reproductive research, have the advantage of being genetically modifiable to enable mechanistic studies, and are also relatively inexpensive. Other species include rats, rabbits, guinea pigs, sheep, non-human primates and others. A major advantage of non-human primates, mice, rats, and guinea pigs is that all of them have hemochorial placenta, while sheep have epithelio-chorial placenta. In all of these animal models, IUI can be induced by injecting different pathogen-associated molecular pattern molecules (PAMPs), damage-associated molecular patterns (DAMPs), or live microorganisms (107). The characteristic features of acute chorioamnionitis are diffuse infiltration of neutrophils into the chorion and amnion membranes, and increased inflammatory cytokines and chemokines (108).
The route of injection is important since TLR activation in different compartments elicits different responses. Systemic (intravenous, intraperitoneal, or subcutaneous) injection of agents will cause a systemic inflammatory response. In mice, systemic inflammation can cause progesterone withdrawal due to the regression of the corpus luteum, triggering preterm birth (109). Localized intrauterine inflammation can be induced in mice by injecting agents into the uterine horn between the gestational sacs (88). Most commonly, this has been done by mini-laparotomy to expose the uterine horns (96); however, such procedure could induce adverse pregnancy outcomes due to a rapid localized inflammatory response that surrounds the incision area. In the last few years, new less-invasive techniques have been developed, including ultrasound-guided intraamniotic and intrauterine injection of TLR, thus minimizing the effects of surgery (88). Similarly, ultrasound-guided intraamniotic injection has been used in a variety of species. Other non-invasive methods including intravaginal injection/inoculation have also been used (88, 98).
In non-human primates, both intraamniotic or choriodecidual injection/infusion of agents has been used. In some studies, the animals are placed in a nylon jacket and tether with the catheters/electrodes tracked through the tether system (with 360° mobility), requiring prior adaption of the animal and considerable animal handling skills (31). Although sheep have been traditionally used for study of fetal physiology and fetal inflammation, they are not suited for preterm labor studies since they lack the decidua and the placental architecture is considerably different from humans (110). Thus, while interpreting and comparing studies, the species used, route of injection, dosage of agents, and other experimental details need to be carefully considered to correctly interpret experimental data.
Immune and Non-Immune Cells at the Feto-maternal Interface
Several distinct immune cells populate the normal maternal-fetal interface with distinct homeostatic roles during normal pregnancy (111). Immune cells in the choriodecidua have distinct immunological profiles and functions compared to their counterparts in blood (112). However, the repertoire of immune cells changes during IUI and parturition (113) and will be reviewed below (Table 2).
Neutrophils
Neutrophilic infiltration of choriodecidua and amnion is a characteristic feature during IUI and forms the basis for the pathological definition of chorioamnionitis (146). Neutrophils infiltrating the choriodecidua are largely of maternal origin (147, 148), while neutrophils in the amniotic fluid are largely of fetal origin (149), although a recent study reported mixed fetal/maternal origin of neutrophils in the amniotic fluid (150). During normal pregnancy, there are small numbers of neutrophils, but the numbers increase dramatically during IUI presumably due to secretion of neutrophilic chemotactic factors such as IL-8 by the amnion (84, 108). CD15+ neutrophils represent the most abundant leukocyte population in AF from women with intraamniotic infection/inflammation (151). During IUI, the choriodecidual neutrophils predominantly produce TNFα, IL-8, and MIP-1β/CCL4 (114, 116). Although blood neutrophils have a short life-span, neutrophils in the decidua have greatly increased survival, mediated in part by up-regulating anti-apoptotic mediators including Bcl-2 family members (e.g., Bcl2A1, called Bfl-1 in humans) (84, 152). Neutrophils can amplify the inflammatory response and neutralize microorganisms by releasing neutrophil extracellular traps (NETs), producing antimicrobial enzymes such as defensins, neutrophil elastase, and myeloperoxidase (MPO), and releasing reactive oxygen species (ROS) (153–155). Neutrophil-derived pro-inflammatory mediators can trigger preterm labor and matrix metalloproteinases (MMPs) can weaken the collagen scaffolding, resulting in preterm rupture of membranes (156–158). However, studies in mice with systemic neutrophil depletion did not protect against inflammation-induced preterm birth (159, 160). Although the reasons for the apparent contradiction are not clear, neutrophil depletion in mice causes a systemic up-regulation of G-CSF, IL-17, and IL-23, leading to hyper-cellular bone marrow as a homeostatic “neutrostat” mechanism to restore the blood neutrophil pool (161, 162). Thus, strategies other than neutrophil depletion are needed to understand the role of neutrophils in triggering preterm labor.
Although neutrophils are classically considered to be pro-inflammatory, regulatory neutrophils have been increasingly recognized in different tissues (163). In the decidua, neutrophils expressing anti-inflammatory indoleamine 2,3-dioxigenase 1 (IDO1) (116), and pro-angiogenic vascular endothelial growth factor (VEGF) are previously postulated to play a role in cyclic vascular proliferation in the endometrium (164, 165), and in 1st and 2nd trimester human decidua basalis (166, 167). Based on peak neutrophil influx after the onset of normal labor, post-partum uterus/decidua matrix remodeling and wound healing function has been attributed to decidual neutrophils (113, 168–170). Thus, there appears to be neutrophil heterogeneity and a role for neutrophils in tissue homeostasis at the maternal-fetal interface similar to that for other tissues (163, 171).
Monocytes/Macrophages
Monocytes/macrophages represent 20–30% of the leukocyte population at the maternal-fetal interface. These include macrophages of maternal origin in the decidua, and the Hofbauer cells of fetal origin in the placental villi (172, 173). Monocytes/macrophages in the amniotic fluid, especially in cases of IUI, can be of mixed maternal/fetal origin (174). Placental macrophages are essential for normal pregnancy as genetic depletion of CD11b+ macrophages prevents embryo implantation via regulation of corpus luteum development in mice, and the csf1-deficient osteopetrosis mice have severe infertility (175). Decidual macrophages play a key role in angiogenesis, tissue remodeling, immune surveillance, host-defense, antigen-presentation, and immune tolerance. For example, decidual macrophages inhibit NK cell-mediated lysis of human cytotrophoblasts by TGF-β1 secretion (176), and by phagocytosis of apoptotic cells (177). Macrophages also play a key role in decidualization, angiogenesis, uterine contraction, and tissue remodeling (168, 173, 178).
Since decidual macrophages perform diverse functions, the polarization varies from being largely pro-inflammatory (M1-like) during the peri-implantation period, anti-inflammatory (M2-like phenotype) during mid to late pregnancy, and pro-inflammatory (M1-like phenotype) during parturition (179). However, decidual macrophages cannot be strictly phenotyped as either M1 or M2. Rather, Houser et al. described two unique decidual macrophage populations based on their CD11c expression (180). Recently, these observations were further clarified with the description of three decidual macrophage subsets, CCR2−CD11clow in the decidua parietalis, and CCR2−CD11chi and CCR2+CD11chi in proximity of extra villous trophoblasts during the first trimester of human pregnancy. Although the three different subsets exhibit phagocytic capacity, CCR2−CD11clow macrophages showed an M2-like anti-inflammatory phenotype while CCR2+CD11chi an M1-like pro-inflammatory phenotype, suggesting that those different macrophage subsets contribute to maintaining an inflammatory balance at the maternal-fetal interface (181).
During IUI, decidual macrophage numbers increase (84), with a predominance of M2-like phenotype in humans (120). Similar to neutrophil NETs, macrophage extracellular traps were recently reported in the decidua in response to group B Streptococcus infection (121). These traps containing MMPs effectively kill invading microorganisms, thereby protecting the host. Although not a topic of this review, disruption of appropriate macrophage polarization is also associated with other abnormal pregnancies, including spontaneous abortions and preeclampsia (179).
T Cells
T cells play an important role in the setting of IUI and are involved in the mechanisms responsible of induction of labor (182–184). In vivo T cell activation by intraperitoneal injection of αCD3ε antibody in late gestation causes pathological inflammation and initiates innate and adaptive immune responses which, in turn, lead to preterm labor and birth (185). Our group recently reported an increase of CD3+ T cells in uterine tissues after intraamniotic Ureaplasma exposure in Rhesus macaques (99). T cells represent ~20–30% of CD45+ cells at the maternal-fetal interface in a Rhesus model of LPS-induced IUI as well as in human preterm pregnancy (84). In humans, more CD45RO memory T cells accumulated in the choriodecidua at term (regardless of the presence of labor) than in preterm pregnancy without labor in a CXCL10/CCL5-dependent manner (184, 186). These cells express high levels of MMP-9, IL-1β, and TNFα (184), cytokines involved in the onset of labor as well as mechanisms of membrane rupture (187).
During IUI, maternal CD8 T cells are increased in both the placenta and peripheral circulation and they express CCR3, perforin, and granzyme B (188–190). CD8+ T cells have the ability to kill fetal cells and therefore should be excluded from the maternal-fetal interface. Indeed, Nancy et al. showed that impaired effector T cell accumulation in the decidua is partly mediated by epigenetic silencing of key T cell-attracting inflammatory chemokines (11) during normal pregnancy. However, after L. monocytogenes prenatal infection, maternal CD8+ T cells with fetal specificity selectively upregulate the expression of CXCR3, CXCL9 receptor, and are recruited to the decidua by CXCL9-secreting neutrophils (93). CXCR3 blockade before or shortly after L. monocytogenes infection correlated with fewer CD8+ T cells in the decidua and no fetal death (93).
Maternal-Fetal Tolerance and Tregs
A number of protective mechanisms have been identified to explain the immunologic paradox of maternal tolerance of the semi-allogenic fetus (191). These include: (1) fetal cells in contact with maternal cells expressing non-classical MHC antigens; (2) maternal NK cells being less cytotoxic and recognizing the fetal trophoblasts with non-classical MHC (192–194); (3) the role of decidua stromal in local immune modulation and maintenance of immune tolerance (11, 195); (4) a skew toward anti-inflammatory macrophage phenotype; (5) disappearance of lymphatics from the endometrium upon decidualization (196); and (6) chemokine silencing to decrease trafficking of cytotoxic T-cells in the decidua (11).
Regulatory T-cells (Tregs) play a pivotal role in maternal-fetal tolerance (197). Immature dendritic cells induce Treg formation by secreting IDO and TGFβ (198, 199). Decidual NK cells can also facilitate the generation of Tregs (199, 200). Regulatory B cells (Bregs) are the B cells producing IL-10 under the influence of gonadotropins. Bregs are increased in early human pregnancy and suppress TNFα production by T cells (201, 202). Maternal Tregs, stimulated by chorionic gonadotrophins, accumulate at the maternal-fetal interface during early gestation (203, 204), peak in the second trimester (205), and mediate tolerance to paternal antigens, facilitating embryo implantation and preventing embryo resorption (206–209).
Immune tolerance of the fetus during pregnancy places the mother at a higher risk for impaired host defense. Indeed, Treg expansion in mouse models increased susceptibility to Listeria and Salmonella infections during pregnancy (210). Apart from fetal immune tolerance, Tregs also suppress activity of other decidual immune cells. Indeed, Treg secretion of TGFβ and IL10 downregulate decidual NK cell activation, and this suppression is protective against pregnancy complications such as pre-eclampsia and recurrent abortions (211, 212). However, the numbers of CD3+CD8−FOXP3+ Tregs at the maternal-fetal interface did not change upon intraamniotic LPS exposure in Rhesus macaques (84). Treg phenotype in the fetus during chorioamnionitis is discussed elsewhere in this journal.
NK Cells
In the first trimester, decidual natural killer cells (dNK) cells comprise ~70% of immune cells at the choriodecidua, representing the largest population of leukocytes at the maternal-fetal interface. Both immunological phenotypes and function of decidua NK cells differ from their blood counterparts. Compared with circulating CD56brightCD16+ NK cells, CD56brightCD16−dNK cells present a lower cytotoxicity and higher levels of killer immunoglobulin-like receptors (KIRs) and natural killer group 2 (NKG2) receptors (192, 213). dNK cells express exclusively CD151, CD9, and tetrasparan-5 compared to peripheral NK cells in the first trimester of gestation (214). After implantation, CXCR4+ NK progenitor cells migrate into the uterus via a CCL3/CXCL12 gradient (215), and proliferate in response to IL-15 (216). The number of the uterine NK cells decreases significantly at gd 6 in mice (217), peaks at gd10 (218), and decreases dramatically at d12, when the majority of NK cells have become senescent (219). Uterine NK cell-derived IL-8, CXCL10, IFNγ, and vascular growth factors are critically needed for decidual spiral artery remodeling and successful pregnancy outcomes 149, (220–222). Decidual NK cells play an important role in recognizing paternal MHC on trophoblast cells via killer-cell immunoglobin-like receptor interactions, mediating tolerance (192–194). Furthermore, a cross-talk between dNK and dCD14+IDO+ cells could promote the generation of Tregs, thereby facilitating fetal immune tolerance (199, 200).
Despite important roles performed by NK cells during normal pregnancy, the role of these cells is not clear in the pathogenesis of chorioamnionitis. In mice, intraperitoneal LPS injection increased the frequency of activated NK cells CD69+CD49b+ at the maternal-fetal interface (135), while we did not demonstrate changes in NK cell frequency in the choriodecidua of Rhesus macaques given intraamniotic LPS (84). In humans, both decidua basalis and parietalis showed an increased frequency of CD56+NK cells in women that underwent pre-term labor with acute chorioamnionitis compared to those without chorioamnionitis (134). Although NK cells perform homeostatic roles, under pathological conditions such as fetal alloimmune thrombocytopenia, dNK cells can become activated with prolonged survival, elevated NKp46 and CD107 expression, and perforin release, and can induce trophoblast apoptosis and placental pathology, leading to miscarriage (223).
Dendritic Cells (DCs)
DCs are instrumental for decidualization and angiogenesis in mice because their depletion prevents blastocyst implantation and formation of the decidua (224, 225). Large amounts of DEC-205+ DCs accumulated in both the decidua basalis and parietalis obtained from women who underwent preterm birth regardless of the presence of acute chorioamnionitis (134). Peripheral blood myeloid and plasmacytoid DCs numbers fall in the second trimester but subsequently increase in the third trimester (226, 227) and become more activated (228, 229). These DC populations show lower levels of costimulatory molecules CD40, CD80, and CD86 during pregnancy complications compared to healthy pregnancy (230).
Little is known about the role of DCs during chorioamnionitis. A number of studies in experimental models and humans demonstrate minimal changes in circulating DCs during chorioamnionitis (99, 136). Interestingly, DEC-205+ DCs obtained from women who underwent preterm birth without acute chorioamnionitis had a higher expression of the costimulatory molecule CD86 and produced more IL-12. These DCs preferentially enhanced the proliferation of iNKT cells in vitro (134). On the contrary, DEC-205+ DCs from women who underwent preterm birth with acute chorioamnionitis induced proliferation of NK cells (134).
B Cells
Although B-cell deficient mice have normal pregnancies, IL10 producing B cells protected fetuses from intrauterine demise when exposed to LPS (140). In human term and preterm pregnancy, total CD19+ B cells represent a small fraction (<5%) of decidual leukocytes in both the decidua parietalis and basalis (138, 231). Furthermore, B cell frequency did not significantly change during the course of pregnancy in decidua basalis even during systemic inflammation (231). However, the frequency of CD19+ B cells were higher in the decidua parietalis of women who underwent preterm birth regardless of labor (138, 139). These results are consistent with our results of a ~1.5-fold increase in CD19+CD20+ B cells numbers in the decidua parietalis in Rhesus macaque exposed to intraamniotic LPS compared to IA saline controls (84). Functionally, B cells were reported to be protective for preterm birth in mice via IL-33 and progesterone-induced blocking factor, decreasing LPS induced neutrophil recruitment (139), although this protective action has been questioned in a recent study (138).
Decidual B cells can secrete both pro-inflammatory IL-12 and IL-6, as well as immunosuppressive IL-35 (138) suggesting multiple context-dependent roles for B cells during intrauterine inflammation. Furthermore, CD19+CD24hiCD27+ B cells can produce IL-10 under the influence of gonadotropins, and these regulatory B cells (Bregs) are increased in early human pregnancy and suppress TNFα production by T cells (201, 202). Additionally, adoptive transfer of IL-10 producing B cells increased the number of uterine Tregs and protected against LPS-induced adverse pregnancy effects by decreasing the production of IL-17A and IL-6 by naïve CD4+CD25− T cells (140).
iNKT Cells
Invariant natural killer T cells (iNKT) specifically recognize glycolipid antigens presented by MHC class I-related protein CD1d and produce large amounts of both Th1 and Th2 cytokines upon stimulation (232). iNKT cells are present in both human and mouse decidua (233–236), and their specific ligand CD1d is expressed in human villous and invasive extra villous trophoblast (EVT) (235). Indeed, injection of the CD1d ligand α-galactosylceramide (αGalCer) resulted in iNKT cell-mediated pregnancy loss that was dependent on gestational age, strain, and route of administration (141, 233, 235). Furthermore, placenta from women with non-infectious preterm delivery demonstrated an increase in activated iNKT cells (134, 141, 237). Although the mechanism is not clear, one possibility is that iNKT mediates the activation of decidual DC, and increases the expression of co-stimulatory molecules CD40, CD80, and CD86 (142). Moreover, Jα18−/− mice lack iNKT cells and are resistant to LPS-induced preterm birth. Further support of the role of iNKT in inflammation-mediated preterm delivery in mice is that adoptive transfer of decidual iNKT cells into LPS-stimulated Jα18−/− mice significantly induced preterm birth by recruiting adopted iNKT cells into the decidua (143). Overall, the results suggest that activation of iNKT cells in the decidua may play a role in triggering preterm birth of non-infectious etiology.
ILCs
Innate lymphoid cells (ILCs) are a group of immune cells belonging to the lymphoid lineage, although they do not express antigen-specific receptors. These cells are found at the mucosal surface and are extremely important in innate immune responses to infectious microorganisms and in the regulation of homeostasis and inflammation (238). To date, three distinct subsets of ILCs have been identified based on their different phenotype and functions: ILC1s (dependent on the expression of T-bet); ILC2s (dependent on the expression of GATA3 and RORα); and ILC3s (dependent on the expression of RORγ) (239). ILC2 cells are the most common ILCs expressed in preterm and term decidua (124). However, their role in the context of acute chorioamnionitis is not well-defined.
In preterm birth patients, an increase in both ILC2s and ILC3s were observed in the decidua basalis and decidua parietalis, respectively (124). Interestingly, ILC3s in the decidua from women with spontaneous preterm labor were activated since they expressed higher levels of IL-13 and IFNγ, cytokines normally produced by ILC2s and ILC1s, respectively (124, 240). Further studies are needed to elucidate the biology ILCs during normal and complicated pregnancies.
Trophoblast Cells
The trophoblast cells in villous placenta include the cytotrophoblast and syncytiotrophoblast. In extraplacental fetal membranes, the trophoblast cells are called EVTs. Fetal trophoblasts are a component of innate immune system and play an important role in orchestrating the maternal innate immune response to infection at the maternal-fetal interface (241, 242).
Fetal trophoblasts employ several mechanisms to suppress maternal immune cells: (1) HLA-G protects EVTs from NK-cell mediated cytotoxicity (243); (2) HLA-G+ EVTs express anti-inflammatory mediators including IDO (244), programmed death ligand PDL-1 (245); IL-10 and TGFβ (246, 247); (3) EVTs enhance the expansion of maternal Tim3+PD-1+CD8+ T cells in the decidua which recognize PDL-1+ EVTs and downregulate cytotoxicity (248); (4) HLA-G+ EVTs induces tolerogenic DCs by disruption of the MHCII presentation pathway, inducing differentiation of anergic and immunosuppressive CD4+ and CD8+ effector T cells (249); (5) Cocultures of HLA-G+ EVTs with sample matched decidual CD4+ T cells results in increased number of Tregs (250). How these mechanisms are altered in the context of IUI is not known.
Studies in experimental models clearly demonstrate that the trophoblasts are capable of response to an inflammatory challenge and modulate the immune response. In mice, intraperitoneal poly[I:C] injection induces stimulation of TLR3, and activates trophoblast cells through the production of KC, GM-CSF, IL-12p40, MIP-1α, and MIP-1β (145). In vitro, first trimester trophoblast cells can promote monocyte migration through the production of GRO-α, MCP-1/CCL2, and CXCL8/IL-8 in response to LPS (251, 252). Interestingly, the trophoblast-activation response to LPS was dose dependent. Compared to high doses of LPS, low doses of LPS result in the lesser production of chemokines and cytokines, and therefore less trophoblast activation (251, 252). Trophoblast cells can also release high amounts of IL-8 that triggers NET formation in preeclampsia (253). On the other hand, trophoblast cells can inhibit neutrophil activation, survival, NET formation, and ROS synthesis via vasointestinal peptide and other glucose metabolism pathways (254, 255).
Amnion
As the amnion is in contact with AF, it is strategically located to transduce inflammatory signals in the AF to mount the immune response (84). In a Rhesus macaque model of IUI, the amnion upregulated phospho-IRAK1-expressed neutrophil chemoattractants CXCL8 and CSF3 in an IL-1-dependent manner (84). Moreover, amniotic cells express a set of TLRs suggesting that the amnion plays an important role as sentinel cells that recognize a wide variety of pathogen derived molecular patterns (26, 256). When stimulated or exposed to inflammatory signals, the amnion can also secrete pro-inflammatory mediators including prostaglandins and cytokines/chemokines (256, 257). Thus, it appears that the amnion may be a sensor and regulator of the inflammatory response to infectious/inflammatory stimuli and play a role in triggering preterm labor.
Decidua Stroma Cells
The decidual stromal cells express immune receptors that respond to ascending infections during pregnancy (258). Stromal cells are in contact with multiple different immune cells and modify the decidual immune response. Their role in during IUI needs to be better defined. In in vitro studies, decidual stromal cells exerted a powerful inhibitory effect on NK cell proliferation and DC differentiation (259). Decidual stromal cells can also interact with macrophages in regulating the immune response against pathogens through the release of PGE2 and IL-8 (260, 261). Moreover, decidual stromal cells also regulate the interplay between pro-inflammatory cytokines and the reproductive endocrine system that may modulate inflammation-mediated preterm birth (262). Thus, decidual mesenchymal cells, like mesenchymal cells in other niches, can both downregulate or upregulate the activity of decidual immune cells in a context-dependent manner.
Pathogenesis of Chorioamnionits and Potential Therapeutic Strategies
As mentioned above, the pathological hallmark of chorioamnionitis is neutrophil infiltration in the fetal membranes, and is often associated with neutrophils in the amniotic fluid. Although the mechanisms of neutrophil recruitment at the maternal-fetal interface is not entirely clear, the amnion may play an important role by secreting chemoattractants (84). Neutrophils that accumulate at the maternal-fetal interface are activated with increased survival mediated by the anti-apoptotis factors belonging to the Bcl family (84). The interplay between neutrophils, macrophages, Tregs, CD8 T cells, and the decidual stromal cells regulate the intensity of inflammation, and secretion of cytokines, chemokines, and prostaglandins (Figure 3). The net result is a feed-forward loop of inflammation that can result in preterm labor and birth. Viral infections or activation of the type-I interferon signaling can further potentiate inflammation (61, 65, 67, 145). If the inflammatory stimulus subsides, resolution of the inflammatory process can also occur.
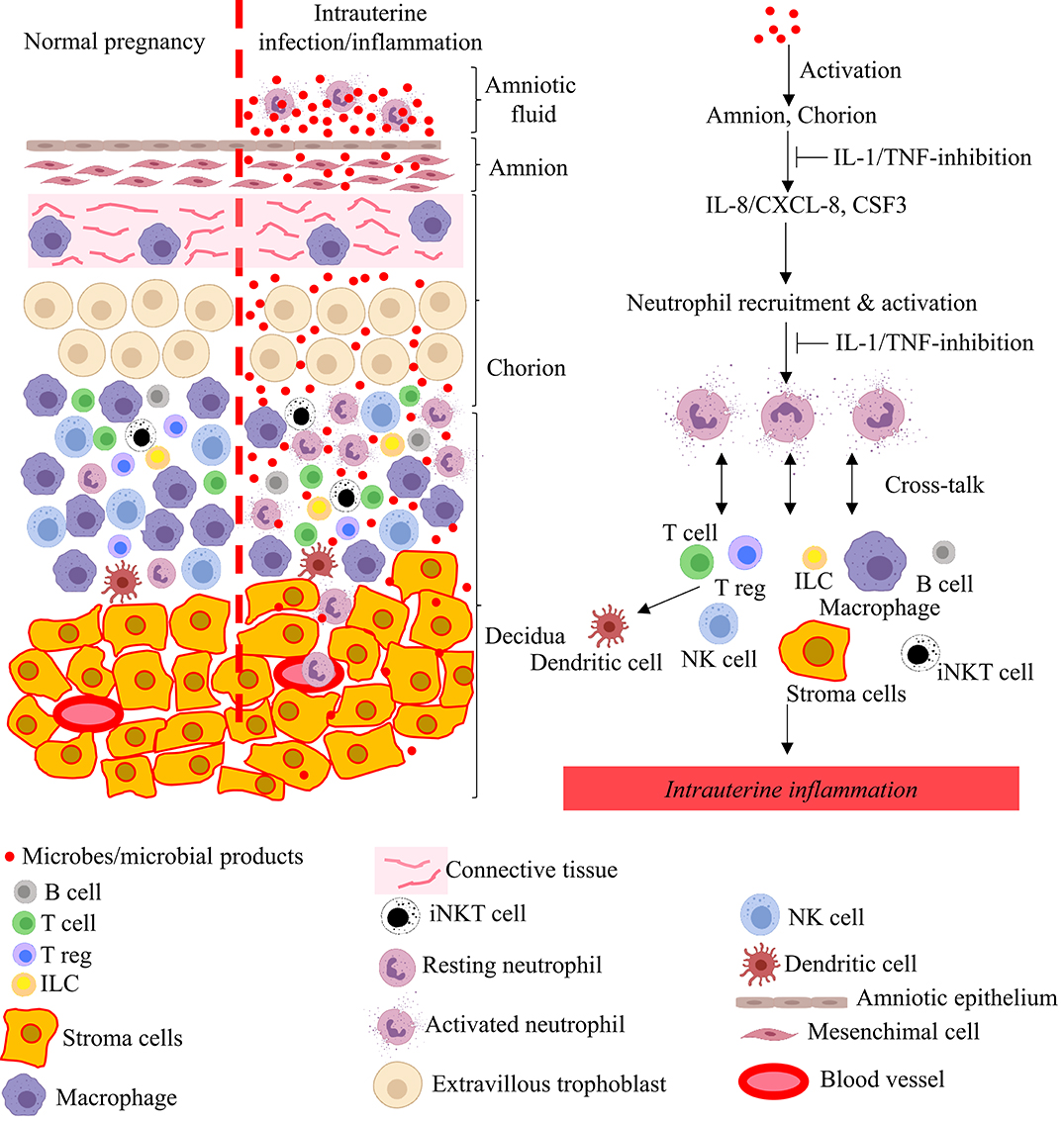
Figure 3. Model for pathogenesis of intrauterine infection/inflammation. Representative cells in the different tissue layers of fetal membrane are shown. The left panel in figure depicts normal pregnancy and the right panel shows changes during IUI. Inflammatory products and microbial products (red dots) in the amniotic fluid and choriodecidua activate the amnion and chorion, resulting in the release of neutrophil chemoattractant (CXCL-8/IL-8 and CSF3) in a IL-1 and TNF-dependent manner. Neutrophils accumulate at choriodecidua junction, get activated, and greatly amplify the inflammation at the maternal-fetal interface with cross-talk with other immune and resident cells.
Antibiotics, the mainstay of the current treatment, are largely ineffective in preventing IUI-associated morbidities (263, 264), partly because residual inflammation from the infection can cause fetal and maternal injury (100, 128, 265, 266). Therefore, the development of alternative therapeutic approaches is essential (267).
The nuclear factor-κB (NF-κB) proteins are prototypic molecules involved in inflammation and immune signaling. Upon activation by a variety of stimuli including LPS, the normally inactive NF-κB proteins retained in the cytoplasm by IκB, are activated and translocate to the nucleus, where they increase the transcription of target genes (268). Since NF-kB plays a pivotal role in cellular inflammatory response, several NF-kB inhibitors have been tested to block IUI. N-acetyl-cysteine (NAC) inhibits inflammation in human fetal membranes in vitro (269) and in vivo (270–272). In a clinical trial, NAC administered to women between 16 and 18 weeks' gestation with previous preterm labor and bacterial vaginosis reduced the recurrence of preterm birth (273). High concentrations of sulfasalazine, another suppressor of NF-kB activity, reduced inflammation but also induced apoptosis in the chorion in an ex-vivo model of fetal membrane inflammation (274). Cytokine-suppressive anti-inflammatory drugs (CSAIDs) specifically target inflammatory signaling pathway such as NF-kB, and are therefore candidates for the treatment of IUI. TPCA-1 and parthenolide are selective inhibitors of the kinase complex that regulates the NF-κB cascade, such as IKKβ. Both TPCA-1 and parthenolide inhibited human choriodecidual IL-6 and TNFα production and inflammatory gene expression in vitro (275). Similarly, in an ovine model of IA LPS-induced chorioamnionitis, TPCA-1 and 5z-7-oxozeaenol abrogated the stimulatory effects of LPS on prostaglandin production in the AF. However, fetal lung inflammation was not affected by the treatment of those two compounds, suggesting that the beneficial effects on the fetus were minimal (276). Non-steroidal anti-inflammatory drugs (NSAIDS) are another class of compounds used in the treatment of inflammation with some success in Rhesus macaque models (277, 278), but they are not without risks for the fetus (279).
TNFα is a major pro-inflammatory cytokine whose levels are increased during IUI. TNFα blockade decreased adverse pregnancy outcomes in rodents (280, 281). A small human study demonstrated that TNFα-blockade can improve outcomes in women with recurrent spontaneous abortions (282). However, a concern with using the clinically approved anti-TNFα antibodies is that the drug freely crosses placenta and is detectable in the infant after birth since the half-life is several weeks, and prolonged inhibition of TNFα can result in immune suppression. Another important cytokine implicated in IUI is IL-1β (277, 283–285). In a variety of animal models, inhibitors of IL1 signaling such as Anakinra (recombinant IL-1 receptor antagonist) and peptide inhibitors substantially reduced intrauterine neutrophil infiltration and inflammation (84) and fetal inflammation (3, 128, 286–290). However, the efficacy of IL-1 inhibitors in preventing preterm birth has been questioned (284, 291). An attractive feature of the widely used clinical drug Anakinra (recombinant IL-1 receptor antagonist) is that it has a short half-life, thus decreasing the concern for prolonged immunosuppression of the fetus. Anakinra is widely used as an anti-inflammatory agent for Rheumatoid arthritis and other inflammatory diseases (292), and classified as a class B drug during pregnancy by the US FDA (No harm to the fetus in animal studies but lack of well-controlled studies in humans). Anecdotal use during pregnancy has been reported the drug to be safe but well-controlled trials are lacking (293).
Conclusions
Although the link between chorioamnionitis and the risk for maternal and fetal health has long been recognized, important questions remain about the immunobiology of IUI. Multiple lines of evidence from animal experiments and in humans have convincingly demonstrated that different microorganisms can cause IUI through various routes of invasion. Moreover, the emerging concept of priming viral infections and polymicrobial infection need to be further investigated.
Among different immune cells, neutrophils infiltrating the chorioamnion decidua tissue play a major role in the pathogenesis of IUI. The mechanisms regulating neutrophil recruitment to fetal membranes and their role in promotion of IUI currently represent an area of active investigation.
Antibiotic therapy for IUI has been disappointing so far, likely because of the residual intrauterine inflammation. There is a clear need to develop new intervention strategies aimed at reduction of the morbidity and mortality associated with IUI.
Lastly, more efforts are needed to build interdisciplinary teams spanning reproductive biology, infectious diseases, pharmacology and immunology, maternal and fetal health that would allow a broad approach in the understanding of the pathogenesis of chorioamnionitis and to develop new therapeutics to prevent/cure IUI.
Author Contributions
MC, PP, and SK contributed to the scope and setup of and wrote the review. All authors approved the final version.
Funding
This study was supported by R21HD90856 (SK) and R01HD98389 (SK).
Conflict of Interest
The authors declare that the research was conducted in the absence of any commercial or financial relationships that could be construed as a potential conflict of interest.
Acknowledgments
We also thanks Allison L. Fisher for the critical review the manuscript.
Abbreviations
IUI, Intrauterine Infection/Inflammation; TLR, Toll-like receptor; PAMP, Pathogen-associated molecular pattern; VEGF, Vascular endothelial growth factor; DAMPs, Damage-associated molecular patterns; MMPs, matrix metalloproteinases.
References
1. Kim CJ, Romero R, Chaemsaithong P, Chaiyasit N, Yoon BH, Kim YM. Acute chorioamnionitis and funisitis: definition, pathologic features, and clinical significance. Am J Obstet Gynecol. (2015) 213:S29–52. doi: 10.1016/j.ajog.2015.08.040
2. Iams JD, Romero R, Culhane JF, Goldenberg RL. Primary, secondary, and tertiary interventions to reduce the morbidity and mortality of preterm birth. Lancet. (2008) 371:164–75. doi: 10.1016/S0140-6736(08)60108-7
3. Nadeau-Vallee M, Obari D, Palacios J, Brien ME, Duval C, Chemtob S, et al. Sterile inflammation and pregnancy complications: a review. Reproduction. (2016) 152:R277–92. doi: 10.1530/REP-16-0453
4. Gomez-Lopez N, Romero R, Tarca AL, Miller D, Panaitescu B, Schwenkel G, et al. Gasdermin D: evidence of pyroptosis in spontaneous preterm labor with sterile intra-amniotic inflammation or intra-amniotic infection. Am J Reprod Immunol. (2019) 82:e13184. doi: 10.1111/aji.13184
5. Familari M, Naav A, Erlandsson L, De Iongh RU, Isaxon C, Strandberg B, et al. Exposure of trophoblast cells to fine particulate matter air pollution leads to growth inhibition, inflammation and ER stress. PLoS ONE. (2019) 14:e0218799. doi: 10.1371/journal.pone.0218799
6. Huynh M, Woodruff TJ, Parker JD, Schoendorf KC. Relationships between air pollution and preterm birth in California. Paediatr Perinat Epidemiol. (2006) 20:454–61. doi: 10.1111/j.1365-3016.2006.00759.x
7. Bove H, Bongaerts E, Slenders E, Bijnens EM, Saenen ND, Gyselaers W, et al. Ambient black carbon particles reach the fetal side of human placenta. Nat Commun. (2019) 10:3866. doi: 10.1038/s41467-019-11654-3
8. Menon R, Fortunato SJ, Yu J, Milne GL, Sanchez S, Drobek CO, et al. Cigarette smoke induces oxidative stress and apoptosis in normal term fetal membranes. Placenta. (2011) 32:317–22. doi: 10.1016/j.placenta.2011.01.015
9. Lannon SM, Vanderhoeven JP, Eschenbach DA, Gravett MG, Adams Waldorf KM. Synergy and interactions among biological pathways leading to preterm premature rupture of membranes. Reprod Sci. (2014) 21:1215–27. doi: 10.1177/1933719114534535
10. Menon R, Richardson LS, Lappas M. Fetal membrane architecture, aging and inflammation in pregnancy and parturition. Placenta. (2019) 79:40–5. doi: 10.1016/j.placenta.2018.11.003
11. Nancy P, Tagliani E, Tay CS, Asp P, Levy DE, Erlebacher A. Chemokine gene silencing in decidual stromal cells limits T cell access to the maternal-fetal interface. Science. (2012) 336:1317–21. doi: 10.1126/science.1220030
12. Prabhudas M, Bonney E, Caron K, Dey S, Erlebacher A, Fazleabas A, et al. Immune mechanisms at the maternal-fetal interface: perspectives and challenges. Nat Immunol. (2015) 16:328–34. doi: 10.1038/ni.3131
13. Chen GY, Nunez G. Sterile inflammation: sensing and reacting to damage. Nat Rev Immunol. (2010) 10:826–37. doi: 10.1038/nri2873
14. Romero R, Chaiworapongsa T, Alpay Savasan Z, Xu Y, Hussein Y, Dong Z, et al. Damage-associated molecular patterns (DAMPs) in preterm labor with intact membranes and preterm PROM: a study of the alarmin HMGB1. J Matern Fetal Neonatal Med. (2011) 24:1444–55. doi: 10.3109/14767058.2011.591460
15. Committee on Obstetric Practice. Committee Opinion No. 712: intrapartum management of intraamniotic infection. Obstet Gynecol. (2017) 130:e95–101. doi: 10.1097/AOG.0000000000002236
16. Higgins RD, Saade G, Polin RA, Grobman WA, Buhimschi IA, Watterberg K, et al. Evaluation and management of women and newborns with a maternal diagnosis of chorioamnionitis: summary of a workshop. Obstet Gynecol. (2016) 127:426–36. doi: 10.1097/AOG.0000000000001246
17. Hafez S. Comparative placental anatomy: divergent structures serving a common purpose. Prog Mol Biol Transl Sci. (2017) 145:1–28. doi: 10.1016/bs.pmbts.2016.12.001
18. Malassine A, Frendo JL, Evain-Brion D. A comparison of placental development and endocrine functions between the human and mouse model. Hum Reprod Update. (2003) 9:531–9. doi: 10.1093/humupd/dmg043
19. Carter AM. IFPA Senior award lecture: mammalian fetal membranes. Placenta. (2016) 48(Suppl. 1), S21–30. doi: 10.1016/j.placenta.2015.10.012
20. Redline RW, Faye-Petersen O, Heller D, Qureshi F, Savell V, Vogler C, et al. Amniotic infection syndrome: nosology and reproducibility of placental reaction patterns. Pediatr Dev Pathol. (2003) 6:435–48. doi: 10.1007/s10024-003-7070-y
21. Lahra MM, Beeby PJ, Jeffery HE. Intrauterine inflammation, neonatal sepsis, and chronic lung disease: a 13-year hospital cohort study. Pediatrics. (2009) 123:1314–9. doi: 10.1542/peds.2008-0656
22. Goldenberg RL, Culhane JF, Iams JD, Romero R. Epidemiology and causes of preterm birth. Lancet. (2008) 371:75–84. doi: 10.1016/S0140-6736(08)60074-4
23. Stoll BJ, Hansen NI, Bell EF, Shankaran S, Laptook AR, Walsh MC, et al. Neonatal outcomes of extremely preterm infants from the NICHD Neonatal Research Network. Pediatrics. (2010) 126:443–56. doi: 10.1542/peds.2009-2959
24. Koga K, Mor G. Toll-like receptors at the maternal-fetal interface in normal pregnancy and pregnancy disorders. Am J Reprod Immunol. (2010) 63:587–600. doi: 10.1111/j.1600-0897.2010.00848.x
25. Pudney J, He X, Masheeb Z, Kindelberger DW, Kuohung W, Ingalls RR. Differential expression of toll-like receptors in the human placenta across early gestation. Placenta. (2016) 46:1–10. doi: 10.1016/j.placenta.2016.07.005
26. Adams KM, Lucas J, Kapur RP, Stevens AM. LPS induces translocation of TLR4 in amniotic epithelium. Placenta. (2007) 28:477–81. doi: 10.1016/j.placenta.2006.08.004
27. Sweeney EL, Dando SJ, Kallapur SG, Knox CL. The human ureaplasma species as causative agents of chorioamnionitis. Clin Microbiol Rev. (2017) 30:349–79. doi: 10.1128/CMR.00091-16
28. Regan JA, Klebanoff MA, Nugent RP. The epidemiology of group B streptococcal colonization in pregnancy. Vaginal Infections and Prematurity Study Group. Obstet Gynecol. (1991) 77:604–10.
29. Newton ER, Clark M. Group B streptococcus and preterm rupture of membranes. Obstet Gynecol. (1988) 71:198–202.
30. Seyyed EZ, Toossi E, Jalalvand A, Sajadi M. Group B Streptococci investigation in pre-term labors. Med Arch. (2013) 67:124–5. doi: 10.5455/medarh.2013.67.124-125
31. Vanderhoeven JP, Bierle CJ, Kapur RP, Mcadams RM, Beyer RP, Bammler TK, et al. Group B streptococcal infection of the choriodecidua induces dysfunction of the cytokeratin network in amniotic epithelium: a pathway to membrane weakening. PLoS Pathog. (2014) 10:e1003920. doi: 10.1371/journal.ppat.1003920
32. Digiulio DB, Romero R, Kusanovic JP, Gomez R, Kim CJ, Seok KS, et al. Prevalence and diversity of microbes in the amniotic fluid, the fetal inflammatory response, and pregnancy outcome in women with preterm pre-labor rupture of membranes. Am J Reprod Immunol. (2010) 64:38–57. doi: 10.1111/j.1600-0897.2010.00830.x
33. Digiulio DB. Diversity of microbes in amniotic fluid. Semin Fetal Neonatal Med. (2012) 17:2–11. doi: 10.1016/j.siny.2011.10.001
34. Combs CA, Gravett M, Garite TJ, Hickok DE, Lapidus J, Porreco R, et al. Amniotic fluid infection, inflammation, and colonization in preterm labor with intact membranes. Am J Obstet Gynecol. (2014) 210:125e121–e115. doi: 10.1016/j.ajog.2013.11.032
35. Romero R, Miranda J, Chaiworapongsa T, Korzeniewski SJ, Chaemsaithong P, Gotsch F, et al. Prevalence and clinical significance of sterile intra-amniotic inflammation in patients with preterm labor and intact membranes. Am J Reprod Immunol. (2014) 72:458–74. doi: 10.1111/aji.12296
36. Sperling RS, Newton E, Gibbs RS. Intraamniotic infection in low-birth-weight infants. J Infect Dis. (1988) 157:113–7. doi: 10.1093/infdis/157.1.113
37. Nikiforou M, Jacobs EM, Kemp MW, Hornef MW, Payne MS, Saito M, et al. Intra-amniotic Candida albicans infection induces mucosal injury and inflammation in the ovine fetal intestine. Sci Rep. (2016) 6:29806. doi: 10.1038/srep29806
38. Maki Y, Fujisaki M, Sato Y, Sameshima H. Candida chorioamnionitis leads to preterm birth and adverse fetal-neonatal outcome. Infect Dis Obstet Gynecol. (2017) 2017:9060138. doi: 10.1155/2017/9060138
39. Jackel D, Lai K. Candida glabrata sepsis associated with chorioamnionitis in an in vitro fertilization pregnancy: case report and review. Clin Infect Dis. (2013) 56:555–8. doi: 10.1093/cid/cis936
40. Alfei A, Rizzo A, Cavanna C, Lallitto F, Spinillo A. Candida glabrata and pre-term premature rupture of membrane complicating in vitro pregnancy: case report and confirmation of mother to neonate transmission. Arch Gynecol Obstet. (2014) 290:211–4. doi: 10.1007/s00404-014-3222-8
41. Romero R, Schaudinn C, Kusanovic JP, Gorur A, Gotsch F, Webster P, et al. Detection of a microbial biofilm in intraamniotic infection. Am J Obstet Gynecol. (2008) 198:135 e131–135. doi: 10.1016/j.ajog.2007.11.026
42. Gellin BG, Broome CV, Bibb WF, Weaver RE, Gaventa S, Mascola L. The epidemiology of listeriosis in the United States−1986. Listeriosis Study Group. Am J Epidemiol. (1991) 133:392–401. doi: 10.1093/oxfordjournals.aje.a115893
43. Mysorekar IU, Diamond MS. Modeling zika virus infection in pregnancy. N Engl J Med. (2016) 375:481–4. doi: 10.1056/NEJMcibr1605445
44. Tabata T, Petitt M, Puerta-Guardo H, Michlmayr D, Wang C, Fang-Hoover J, et al. Zika virus targets different primary human placental cells, suggesting two routes for vertical transmission. Cell Host Microbe. (2016) 20:155–66. doi: 10.1016/j.chom.2016.07.002
45. Arora N, Sadovsky Y, Dermody TS, Coyne CB. Microbial vertical transmission during human pregnancy. Cell Host Microbe. (2017) 21:561–7. doi: 10.1016/j.chom.2017.04.007
46. Pereira L. Congenital viral infection: traversing the uterine-placental interface. Annu Rev Virol. (2018) 5:273–99. doi: 10.1146/annurev-virology-092917-043236
47. Sharma L, Shukla G. Placental malaria: a new insight into the pathophysiology. Front Med. (2017) 4:117. doi: 10.3389/fmed.2017.00117
48. Stegmann BJ, Carey JC. TORCH Infections. Toxoplasmosis, Other (syphilis, varicella-zoster, parvovirus B19), Rubella, Cytomegalovirus (CMV), and Herpes infections. Curr Womens Health Rep. (2002) 2:253–8.
49. Han YW, Redline RW, Li M, Yin L, Hill GB, Mccormick TS. Fusobacterium nucleatum induces premature and term stillbirths in pregnant mice: implication of oral bacteria in preterm birth. Infect Immun. (2004) 72:2272–9. doi: 10.1128/IAI.72.4.2272-2279.2004
50. Newnham JP, Newnham IA, Ball CM, Wright M, Pennell CE, Swain J, et al. Treatment of periodontal disease during pregnancy: a randomized controlled trial. Obstet Gynecol. (2009) 114:1239–48. doi: 10.1097/AOG.0b013e3181c15b40
51. Hartert TV, Neuzil KM, Shintani AK, Mitchel EFJr, Snowden MS, Wood LB, et al. Maternal morbidity and perinatal outcomes among pregnant women with respiratory hospitalizations during influenza season. Am J Obstet Gynecol. (2003) 189:1705–12. doi: 10.1016/S0002-9378(03)00857-3
52. Cox S, Posner SF, Mcpheeters M, Jamieson DJ, Kourtis AP, Meikle S. Hospitalizations with respiratory illness among pregnant women during influenza season. Obstet Gynecol. (2006) 107:1315–22. doi: 10.1097/01.AOG.0000218702.92005.bb
53. Dodds L, Mcneil SA, Fell DB, Allen VM, Coombs A, Scott J, et al. Impact of influenza exposure on rates of hospital admissions and physician visits because of respiratory illness among pregnant women. CMAJ. (2007) 176:463–8. doi: 10.1503/cmaj.061435
54. Schanzer DL, Langley JM, Tam TW. Influenza-attributed hospitalization rates among pregnant women in Canada 1994-2000. J Obstet Gynaecol Can. (2007) 29:622–9. doi: 10.1016/S1701-2163(16)32559-2
55. Mak TK, Mangtani P, Leese J, Watson JM, Pfeifer D. Influenza vaccination in pregnancy: current evidence and selected national policies. Lancet Infect Dis. (2008) 8:44–52. doi: 10.1016/S1473-3099(07)70311-0
56. Kourtis AP, Read JS, Jamieson DJ. Pregnancy and infection. N Engl J Med. (2014) 370:2211–8. doi: 10.1056/NEJMra1213566
57. Omer SB, Goodman D, Steinhoff MC, Rochat R, Klugman KP, Stoll BJ, et al. Maternal influenza immunization and reduced likelihood of prematurity and small for gestational age births: a retrospective cohort study. PLoS Med. (2011) 8:e1000441. doi: 10.1371/journal.pmed.1000441
58. Jamieson DJ, Kissin DM, Bridges CB, Rasmussen SA. Benefits of influenza vaccination during pregnancy for pregnant women. Am J Obstet Gynecol. (2012) 207:S17–20. doi: 10.1016/j.ajog.2012.06.070
59. Fatemi SH, Folsom TD, Rooney RJ, Mori S, Kornfield TE, Reutiman TJ, et al. The viral theory of schizophrenia revisited: abnormal placental gene expression and structural changes with lack of evidence for H1N1 viral presence in placentae of infected mice or brains of exposed offspring. Neuropharmacology. (2012) 62:1290–8. doi: 10.1016/j.neuropharm.2011.01.011
60. Wiley CA, Carter DM, Ross TM, Bissel SJ. Absence of fetal transmission of H1N1 despite severe maternal infection. Influenza Other Respir Viruses. (2012) 6:e1. doi: 10.1111/j.1750-2659.2011.00310.x
61. Cardenas I, Means RE, Aldo P, Koga K, Lang SM, Booth CJ, et al. Viral infection of the placenta leads to fetal inflammation and sensitization to bacterial products predisposing to preterm labor. J Immunol. (2010) 185:1248–57. doi: 10.4049/jimmunol.1000289
62. Cardenas I, Mor G, Aldo P, Lang SM, Stabach P, Sharp A, et al. Placental viral infection sensitizes to endotoxin-induced pre-term labor: a double hit hypothesis. Am J Reprod Immunol. (2011) 65:110–7. doi: 10.1111/j.1600-0897.2010.00908.x
63. Racicot K, Cardenas I, Wunsche V, Aldo P, Guller S, Means RE, et al. Viral infection of the pregnant cervix predisposes to ascending bacterial infection. J Immunol. (2013) 191:934–41. doi: 10.4049/jimmunol.1300661
64. Kwon JY, Romero R, Mor G. New insights into the relationship between viral infection and pregnancy complications. Am J Reprod Immunol. (2014) 71:387–90. doi: 10.1111/aji.12243
65. Racicot K, Kwon JY, Aldo P, Abrahams V, El-Guindy A, Romero R, et al. Type I interferon regulates the placental inflammatory response to bacteria and is targeted by virus: mechanism of polymicrobial infection-induced preterm birth. Am J Reprod Immunol. (2016) 75:451–60. doi: 10.1111/aji.12501
66. Racicot K, Aldo P, El-Guindy A, Kwon JY, Romero R, Mor G. Cutting edge: fetal/placental type I IFN Can affect maternal survival and fetal viral load during viral infection. J Immunol. (2017) 198:3029–32. doi: 10.4049/jimmunol.1601824
67. Cappelletti M, Presicce P, Lawson MJ, Chaturvedi V, Stankiewicz TE, Vanoni S, et al. Type I interferons regulate susceptibility to inflammation-induced preterm birth. JCI Insight. (2017) 2:e91288. doi: 10.1172/jci.insight.91288
68. Agrawal V, Hirsch E. Intrauterine infection and preterm labor. Semin Fetal Neonatal Med. (2012) 17:12–9. doi: 10.1016/j.siny.2011.09.001
69. Hillier SL, Nugent RP, Eschenbach DA, Krohn MA, Gibbs RS, Martin DH, et al. Association between bacterial vaginosis and preterm delivery of a low-birth-weight infant. The Vaginal Infections and Prematurity Study Group. N Engl J Med. (1995) 333:1737–42. doi: 10.1056/NEJM199512283332604
70. Digiulio DB, Callahan BJ, Mcmurdie PJ, Costello EK, Lyell DJ, Robaczewska A, et al. Temporal and spatial variation of the human microbiota during pregnancy. Proc Natl Acad Sci USA. (2015) 112:11060–5. doi: 10.1073/pnas.1502875112
71. Gajer P, Brotman RM, Bai G, Sakamoto J, Schutte UM, Zhong X, et al. Temporal dynamics of the human vaginal microbiota. Sci Transl Med. (2012) 4:132ra152. doi: 10.1126/scitranslmed.3003605
72. Romero R, Hassan SS, Gajer P, Tarca AL, Fadrosh DW, Nikita L, et al. The composition and stability of the vaginal microbiota of normal pregnant women is different from that of non-pregnant women. Microbiome. (2014) 2:4. doi: 10.1186/2049-2618-2-10
73. Brown RG, Marchesi JR, Lee YS, Smith A, Lehne B, Kindinger LM, et al. Vaginal dysbiosis increases risk of preterm fetal membrane rupture, neonatal sepsis and is exacerbated by erythromycin. BMC Med. (2018) 16:9. doi: 10.1186/s12916-017-0999-x
74. Fettweis JM, Serrano MG, Brooks JP, Edwards DJ, Girerd PH, Parikh HI, et al. The vaginal microbiome and preterm birth. Nat Med. (2019) 25:1012–21. doi: 10.1038/s41591-019-0450-2
75. Callahan BJ, Digiulio DB, Goltsman DSA, Sun CL, Costello EK, Jeganathan P, et al. Replication and refinement of a vaginal microbial signature of preterm birth in two racially distinct cohorts of US women. Proc Natl Acad Sci USA. (2017) 114:9966–71. doi: 10.1073/pnas.1705899114
76. Romero R, Espinoza J, Kusanovic JP, Gotsch F, Hassan S, Erez O, et al. The preterm parturition syndrome. BJOG. (2006) 113(Suppl. 3):17–42. doi: 10.1111/j.1471-0528.2006.01120.x
77. Goepfert AR, Goldenberg RL, Andrews WW, Hauth JC, Mercer B, Iams J, et al. The Preterm Prediction Study: association between cervical interleukin 6 concentration and spontaneous preterm birth. National Institute of Child Health and Human Development Maternal-Fetal Medicine Units Network. Am J Obstet Gynecol. (2001) 184:483–8. doi: 10.1067/mob.2001.109653
78. Robertson SA, Christiaens I, Dorian CL, Zaragoza DB, Care AS, Banks AM, et al. Interleukin-6 is an essential determinant of on-time parturition in the mouse. Endocrinology. (2010) 151:3996–4006. doi: 10.1210/en.2010-0063
79. Stallmach T, Hebisch G, Hi J-J., Orban P, Schwaller J, Engelmann M. Cytokine production and visualized effects in the feto-maternal unit. Quantitative and topographic data on cytokines during intrauterine disease. Lab. Invest. (1995) 73:384–92.
80. Dulay AT, Buhimschi IA, Zhao G, Bahtiyar MO, Thung SF, Cackovic M, et al. Compartmentalization of acute phase reactants Interleukin-6, C-Reactive Protein and Procalcitonin as biomarkers of intra-amniotic infection and chorioamnionitis. Cytokine. (2015) 76:236–43. doi: 10.1016/j.cyto.2015.04.014
81. Grigsby PL, Novy MJ, Adams Waldorf KM, Sadowsky DW, Gravett MG. Choriodecidual inflammation: a harbinger of the preterm labor syndrome. Reprod Sci. (2010) 17:85–94. doi: 10.1177/1933719109348025
82. Adams Waldorf KM, Gravett MG, Mcadams RM, Paolella LJ, Gough GM, Carl DJ, et al. Choriodecidual group B streptococcal inoculation induces fetal lung injury without intra-amniotic infection and preterm labor in Macaca nemestrina. PLoS ONE. (2011) 6:e28972. doi: 10.1371/journal.pone.0028972
83. Adams Waldorf KM, Stencel-Baerenwald JE, Kapur RP, Studholme C, Boldenow E, Vornhagen J, et al. Fetal brain lesions after subcutaneous inoculation of Zika virus in a pregnant nonhuman primate. Nat Med. (2016) 22:1256–9. doi: 10.1038/nm.4193
84. Presicce P, Park CW, Senthamaraikannan P, Bhattacharyya S, Jackson C, Kong F, et al. IL-1 signaling mediates intrauterine inflammation and chorio-decidua neutrophil recruitment and activation. JCI Insight. (2018) 3:e98306. doi: 10.1172/jci.insight.98306
85. Eidem HR, Ackerman WET, Mcgary KL, Abbot P, Rokas A. Gestational tissue transcriptomics in term and preterm human pregnancies: a systematic review and meta-analysis. BMC Med Genomics. (2015) 8:27. doi: 10.1186/s12920-015-0099-8
86. Bukowski R, Sadovsky Y, Goodarzi H, Zhang H, Biggio JR, Varner M, et al. Onset of human preterm and term birth is related to unique inflammatory transcriptome profiles at the maternal fetal interface. PeerJ. (2017) 5:e3685. doi: 10.7717/peerj.3685
87. Kim MJ, Romero R, Gervasi MT, Kim JS, Yoo W, Lee DC, et al. Widespread microbial invasion of the chorioamniotic membranes is a consequence and not a cause of intra-amniotic infection. Lab Invest. (2009) 89:924–36. doi: 10.1038/labinvest.2009.49
88. Rinaldi SF, Makieva S, Frew L, Wade J, Thomson AJ, Moran CM, et al. Ultrasound-guided intrauterine injection of lipopolysaccharide as a novel model of preterm birth in the mouse. Am J Pathol. (2015) 185:1201–6. doi: 10.1016/j.ajpath.2015.01.009
89. Filipovich Y, Lu SJ, Akira S, Hirsch E. The adaptor protein MyD88 is essential for E coli-induced preterm delivery in mice. Am J Obstet Gynecol. (2009) 200:93 e91–98. doi: 10.1016/j.ajog.2008.08.038
90. Randis TM, Gelber SE, Hooven TA, Abellar RG, Akabas LH, Lewis EL, et al. Group B Streptococcus beta-hemolysin/cytolysin breaches maternal-fetal barriers to cause preterm birth and intrauterine fetal demise in vivo. J Infect Dis. (2014) 210:265–73. doi: 10.1093/infdis/jiu067
91. Chin PY, Dorian CL, Hutchinson MR, Olson DM, Rice KC, Moldenhauer LM, et al. Novel Toll-like receptor-4 antagonist (+)-naloxone protects mice from inflammation-induced preterm birth. Sci Rep. (2016) 6:36112. doi: 10.1038/srep36112
92. Cappelletti M, Lawson MJ, Chan CC, Wilburn AN, Divanovic S. Differential outcomes of TLR2 engagement in inflammation-induced preterm birth. J Leukoc Biol. (2018) 103:535–43. doi: 10.1002/JLB.3MA0717-274RR
93. Chaturvedi V, Ertelt JM, Jiang TT, Kinder JM, Xin L, Owens KJ, et al. CXCR3 blockade protects against Listeria monocytogenes infection-induced fetal wastage. J Clin Invest. (2015) 125:1713–25. doi: 10.1172/JCI78578
94. Caro MR, Buendia AJ, Del Rio L, Ortega N, Gallego MC, Cuello F, et al. Chlamydophila abortus infection in the mouse: a useful model of the ovine disease. Vet Microbiol. (2009) 135:103–11. doi: 10.1016/j.vetmic.2008.09.029
95. Chattopadhyay A, Robinson N, Sandhu JK, Finlay BB, Sad S, Krishnan L. Salmonella enterica serovar Typhimurium-induced placental inflammation and not bacterial burden correlates with pathology and fatal maternal disease. Infect Immun. (2010) 78:2292–301. doi: 10.1128/IAI.01186-09
96. Dada T, Rosenzweig JM, Al Shammary M, Firdaus W, Al Rebh S, Borbiev T, et al. Mouse model of intrauterine inflammation: sex-specific differences in long-term neurologic and immune sequelae. Brain Behav Immun. (2014) 38:142–50. doi: 10.1016/j.bbi.2014.01.014
97. Gomez-Lopez N, Romero R, Arenas-Hernandez M, Panaitescu B, Garcia-Flores V, Mial TN, et al. Intra-amniotic administration of lipopolysaccharide induces spontaneous preterm labor and birth in the absence of a body temperature change. J Matern Fetal Neonatal Med. (2018) 31:439–46. doi: 10.1080/14767058.2017.1287894
98. Akgul Y, Word RA, Ensign LM, Yamaguchi Y, Lydon J, Hanes J, et al. Hyaluronan in cervical epithelia protects against infection-mediated preterm birth. J Clin Invest. (2014) 124:5481–9. doi: 10.1172/JCI78765
99. Senthamaraikannan P, Presicce P, Rueda CM, Maneenil G, Schmidt AF, Miller LA, et al. Intra-amniotic Ureaplasma parvum-induced maternal and fetal inflammation and immune responses in rhesus macaques. J Infect Dis. (2016) 214:1597–604. doi: 10.1093/infdis/jiw408
100. Coffey LL, Keesler RI, Pesavento PA, Woolard K, Singapuri A, Watanabe J, et al. Intraamniotic Zika virus inoculation of pregnant rhesus macaques produces fetal neurologic disease. Nat Commun. (2018) 9:2414. doi: 10.1038/s41467-018-04777-6
101. Dudley DM, Aliota MT, Mohr EL, Weiler AM, Lehrer-Brey G, Weisgrau KL, et al. A rhesus macaque model of Asian-lineage Zika virus infection. Nat Commun. (2016) 7:12204. doi: 10.1038/ncomms12204
102. Nguyen SM, Antony KM, Dudley DM, Kohn S, Simmons HA, Wolfe B, et al. Highly efficient maternal-fetal Zika virus transmission in pregnant rhesus macaques. PLoS Pathog. (2017) 13:e1006378. doi: 10.1371/journal.ppat.1006378
103. Kallapur SG, Bachurski CJ, Le Cras TD, Joshi SN, Ikegami M, Jobe AH. Vascular changes after intra-amniotic endotoxin in preterm lamb lungs. Am J Physiol Lung Cell Mol Physiol. (2004) 287:L1178–1185. doi: 10.1152/ajplung.00049.2004
104. Gisslen T, Hillman NH, Musk GC, Kemp MW, Kramer BW, Senthamaraikannan P, et al. Repeated exposure to intra-amniotic LPS partially protects against adverse effects of intravenous LPS in preterm lambs. Innate Immun. (2014) 20:214–24. doi: 10.1177/1753425913488430
105. Kallapur SG, Kramer BW, Knox CL, Berry CA, Collins JJ, Kemp MW, et al. Chronic fetal exposure to Ureaplasma parvum suppresses innate immune responses in sheep. J Immunol. (2011) 187:2688–95. doi: 10.4049/jimmunol.1100779
106. Moss TJ, Nitsos I, Newnham JP, Ikegami M, Jobe AH. Chorioamnionitis induced by subchorionic endotoxin infusion in sheep. Am J Obstet Gynecol. (2003) 189:1771–6. doi: 10.1016/S0002-9378(03)00810-X
107. Cappelletti M, Della Bella S, Ferrazzi E, Mavilio D, Divanovic S. Inflammation and preterm birth. J Leukoc Biol. (2016) 99:67–78. doi: 10.1189/jlb.3MR0615-272RR
108. Kallapur SG, Presicce P, Rueda CM, Jobe AH, Chougnet CA. Fetal immune response to chorioamnionitis. Semin Reprod Med. (2014) 32:56–67. doi: 10.1055/s-0033-1361823
109. Keelan JA. Intrauterine inflammatory activation, functional progesterone withdrawal, and the timing of term and preterm birth. J Reprod Immunol. (2018) 125:89–99. doi: 10.1016/j.jri.2017.12.004
110. Elovitz MA, Mrinalini C. Animal models of preterm birth. Trends Endocrinol Metab. (2004) 15:479–87. doi: 10.1016/j.tem.2004.10.009
111. Erlebacher A. Immunology of the maternal-fetal interface. Annu Rev Immunol. (2013) 31:387–411. doi: 10.1146/annurev-immunol-032712-100003
112. Arenas-Hernandez M, Gomez-Lopez N, Garcia-Flores V, Rangel-Escareno C, Alvarez-Salas LM, Martinez-Acuna N, et al. Choriodecidual leukocytes display a unique gene expression signature in spontaneous labor at term. Genes Immun. (2019) 20:56–68. doi: 10.1038/s41435-017-0010-z
113. Gomez-Lopez N, Stlouis D, Lehr MA, Sanchez-Rodriguez EN, Arenas-Hernandez M. Immune cells in term and preterm labor. Cell Mol Immunol. (2014) 11:571–81. doi: 10.1038/cmi.2014.46
114. Martinez-Varea A, Romero R, Xu Y, Miller D, Ahmed AI, Chaemsaithong P, et al. Clinical chorioamnionitis at term VII: the amniotic fluid cellular immune response. J Perinat Med. (2017) 45:523–38. doi: 10.1515/jpm-2016-0225
115. Salafia CM, Weigl C, Silberman L. The prevalence and distribution of acute placental inflammation in uncomplicated term pregnancies. Obstet Gynecol. (1989) 73:383–9.
116. Presicce P, Senthamaraikannan P, Alvarez M, Rueda CM, Cappelletti M, Miller LA, et al. Neutrophil recruitment and activation in decidua with intra-amniotic IL-1beta in the preterm rhesus macaque. Biol Reprod. (2015) 92:56. doi: 10.1095/biolreprod.114.124420
117. Kallapur SG, Jobe AH, Ball MK, Nitsos I, Moss TJ, Hillman NH, et al. Pulmonary and systemic endotoxin tolerance in preterm fetal sheep exposed to chorioamnionitis. J Immunol. (2007) 179:8491–9. doi: 10.4049/jimmunol.179.12.8491
118. Von Chamier M, Allam A, Brown MB, Reinhard MK, Reyes L. Host genetic background impacts disease outcome during intrauterine infection with Ureaplasma parvum. PLoS ONE. (2012) 7:e44047. doi: 10.1371/journal.pone.0044047
119. Bae GE, Hong JS, Kim JS, Park HY, Jang JY, Kim YS, et al. Differential immunophenotype of macrophages in acute and chronic chorioamnionitis. J Perinat Med. (2017) 45:483–91. doi: 10.1515/jpm-2015-0353
120. Jasic M, Stifter S, Sindicic Dessardo N, Rukavina KM, Mustac E, Belci D. The relationship between histologic chorioamnionitis and decidual macrophage polarization and their influence on outcomes of neonates born before the 32nd gestational week. J Matern Fetal Neonatal Med. (2019) 1–10. doi: 10.1080/14767058.2019.1638906
121. Doster RS, Sutton JA, Rogers LM, Aronoff DM, Gaddy JA. Streptococcus agalactiae induces placental macrophages to release extracellular traps loaded with tissue remodeling enzymes via an oxidative burst-dependent mechanism. MBio. (2018) 9. doi: 10.1128/mBio.02084-18
122. Jaiswal MK, Agrawal V, Mallers T, Gilman-Sachs A, Hirsch E, Beaman KD. Regulation of apoptosis and innate immune stimuli in inflammation-induced preterm labor. J Immunol. (2013) 191:5702–13. doi: 10.4049/jimmunol.1301604
123. Agrawal V, Jaiswal MK, Pamarthy S, Katara GK, Kulshrestha A, Gilman-Sachs A, et al. Role of Notch signaling during lipopolysaccharide-induced preterm labor. J Leukoc Biol. (2016) 100:261–74. doi: 10.1189/jlb.3HI0515-200RR
124. Xu Y, Romero R, Miller D, Silva P, Panaitescu B, Theis KR, et al. Innate lymphoid cells at the human maternal-fetal interface in spontaneous preterm labor. Am J Reprod Immunol. (2018) 79:e12820. doi: 10.1111/aji.12820
125. Rito DC, Viehl LT, Buchanan PM, Haridas S, Koenig JM. Augmented Th17-type immune responses in preterm neonates exposed to histologic chorioamnionitis. Pediatr Res. (2017) 81:639–45. doi: 10.1038/pr.2016.254
126. Singh AM, Sherenian MG, Kim KY, Erickson KA, Yang A, Mestan K, et al. Fetal cord blood and tissue immune responses to chronic placental inflammation and chorioamnionitis. Allergy Asthma Clin Immunol. (2018) 14:66. doi: 10.1186/s13223-018-0297-y
127. Rueda CM, Wells CB, Gisslen T, Jobe AH, Kallapur SG, Chougnet CA. Effect of chorioamnionitis on regulatory T cells in moderate/late preterm neonates. Hum Immunol. (2015) 76:65–73. doi: 10.1016/j.humimm.2014.10.016
128. Rueda CM, Presicce P, Jackson CM, Miller LA, Kallapur SG, Jobe AH, et al. Lipopolysaccharide-induced chorioamnionitis promotes IL-1-dependent inflammatory FOXP3+ CD4+ T cells in the fetal rhesus macaque. J Immunol. (2016) 196:3706–15. doi: 10.4049/jimmunol.1502613
129. Kallapur SG, Kramer BW, Nitsos I, Pillow JJ, Collins JJ, Polglase GR, et al. Pulmonary and systemic inflammatory responses to intra-amniotic IL-1alpha in fetal sheep. Am J Physiol Lung Cell Mol Physiol. (2011) 301:L285–95. doi: 10.1152/ajplung.00446.2010
130. Wolfs TG, Kallapur SG, Polglase GR, Pillow JJ, Nitsos I, Newnham JP, et al. IL-1alpha mediated chorioamnionitis induces depletion of FoxP3+ cells and ileal inflammation in the ovine fetal gut. PLoS ONE. (2011) 6:e18355. doi: 10.1371/journal.pone.0018355
131. Kuypers E, Wolfs TG, Collins JJ, Jellema RK, Newnham JP, Kemp MW, et al. Intraamniotic lipopolysaccharide exposure changes cell populations and structure of the ovine fetal thymus. Reprod Sci. (2013) 20:946–56. doi: 10.1177/1933719112472742
132. Wolfs TG, Kallapur SG, Knox CL, Thuijls G, Nitsos I, Polglase GR, et al. Antenatal ureaplasma infection impairs development of the fetal ovine gut in an IL-1-dependent manner. Mucosal Immunol. (2013) 6:547–56. doi: 10.1038/mi.2012.97
133. Wang F, Xiao M, Chen RJ, Lin XJ, Siddiq M, Liu L. Adoptive transfer of T regulatory cells inhibits lipopolysaccharide-induced inflammation in fetal brain tissue in a late-pregnancy preterm birth mouse model. Cell Biol Int. (2017) 41:155–62. doi: 10.1002/cbin.10710
134. Negishi Y, Shima Y, Takeshita T, Takahashi H. Distribution of invariant natural killer T cells and dendritic cells in late pre-term birth without acute chorioamnionitis. Am J Reprod Immunol. (2017) 77. doi: 10.1111/aji.12658
135. Li L, Kang J, Lei W. Role of Toll-like receptor 4 in inflammation-induced preterm delivery. Mol Hum Reprod. (2010) 16:267–72. doi: 10.1093/molehr/gap106
136. Marchant EA, Kan B, Sharma AA, Van Zanten A, Kollmann TR, Brant R, et al. Attenuated innate immune defenses in very premature neonates during the neonatal period. Pediatr Res. (2015) 78:492–7. doi: 10.1038/pr.2015.132
137. Gleditsch DD, Shornick LP, Van Steenwinckel J, Gressens P, Weisert RP, Koenig JM. Maternal inflammation modulates infant immune response patterns to viral lung challenge in a murine model. Pediatr Res. (2014) 76:33–40. doi: 10.1038/pr.2014.57
138. Leng Y, Romero R, Xu Y, Galaz J, Slutsky R, Arenas-Hernandez M, et al. Are B cells altered in the decidua of women with preterm or term labor? Am J Reprod Immunol. (2019) 81:e13102. doi: 10.1111/aji.13102
139. Huang B, Faucette AN, Pawlitz MD, Pei B, Goyert JW, Zhou JZ, et al. Interleukin-33-induced expression of PIBF1 by decidual B cells protects against preterm labor. Nat Med. (2017) 23:128–35. doi: 10.1038/nm.4244
140. Busse M, Campe KJ, Nowak D, Schumacher A, Plenagl S, Langwisch S, et al. IL-10 producing B cells rescue mouse fetuses from inflammation-driven fetal death and are able to modulate T cell immune responses. Sci Rep. (2019) 9:9335. doi: 10.1038/s41598-019-45860-2
141. St Louis D, Romero R, Plazyo O, Arenas-Hernandez M, Panaitescu B, Xu Y, et al. Invariant NKT cell activation induces late preterm birth that is attenuated by rosiglitazone. J Immunol. (2016) 196:1044–59. doi: 10.4049/jimmunol.1501962
142. Li LP, Fang YC, Dong GF, Lin Y, Saito S. Depletion of invariant NKT cells reduces inflammation-induced preterm delivery in mice. J Immunol. (2012) 188:4681–9. doi: 10.4049/jimmunol.1102628
143. Li L, Yang J, Jiang Y, Tu J, Schust DJ. Activation of decidual invariant natural killer T cells promotes lipopolysaccharide-induced preterm birth. Mol Hum Reprod. (2015) 21:369–81. doi: 10.1093/molehr/gav001
144. Balkundi DR, Ziegler JA, Watchko JF, Craven C, Trucco M. Regulation of FasL/Fas in human trophoblasts: possible implications for chorioamnionitis. Biol Reprod. (2003) 69:718–24. doi: 10.1095/biolreprod.102.013102
145. Koga K, Cardenas I, Aldo P, Abrahams VM, Peng B, Fill S, et al. Activation of TLR3 in the trophoblast is associated with preterm delivery. Am J Reprod Immunol. (2009) 61:196–212. doi: 10.1111/j.1600-0897.2008.00682.x
146. Redline RW. Inflammatory response in acute chorioamnionitis. Semin Fetal Neonatal Med. (2012) 17:20–5. doi: 10.1016/j.siny.2011.08.003
147. Mcnamara MF, Wallis T, Qureshi F, Jacques SM, Gonik B. Determining the maternal and fetal cellular immunologic contributions in preterm deliveries with clinical or subclinical chorioamnionitis. Infect Dis Obstet Gynecol. (1997) 5:273–9. doi: 10.1002/(SICI)1098-0997(1997)5:4<273::AID-IDOG3>3.0.CO;2-Y
148. Steel JH, O'donoghue K, Kennea NL, Sullivan MH, Edwards AD. Maternal origin of inflammatory leukocytes in preterm fetal membranes, shown by fluorescence in situ hybridisation. Placenta. (2005) 26:672–7. doi: 10.1016/j.placenta.2004.10.003
149. Sampson JE, Theve RP, Blatman RN, Shipp TD, Bianchi DW, Ward BE, et al. Fetal origin of amniotic fluid polymorphonuclear leukocytes. Am J Obstet Gynecol. (1997) 176:77–81. doi: 10.1016/S0002-9378(97)80015-4
150. Gomez-Lopez N, Romero R, Xu Y, Leng Y, Garcia-Flores V, Miller D, et al. Are amniotic fluid neutrophils in women with intraamniotic infection and/or inflammation of fetal or maternal origin? Am J Obstet Gynecol. (2017) 217:693 e691–e616. doi: 10.1016/j.ajog.2017.09.013
151. Romero R, Quintero R, Nores J, Avila C, Mazor M, Hanaoka S, et al. Amniotic fluid white blood cell count: a rapid and simple test to diagnose microbial invasion of the amniotic cavity and predict preterm delivery. Am J Obstet Gynecol. (1991) 165:821–30. doi: 10.1016/0002-9378(91)90423-O
152. Berberich I, Hildeman DA. The Bcl2a1 gene cluster finally knocked out: first clues to understanding the enigmatic role of the Bcl-2 protein A1. Cell Death Differ. (2017) 24:572–4. doi: 10.1038/cdd.2017.25
153. Boldenow E, Gendrin C, Ngo L, Bierle C, Vornhagen J, Coleman M, et al. Group B streptococcus circumvents neutrophils and neutrophil extracellular traps during amniotic cavity invasion and preterm labor. Sci Immunol. (2016) 1. doi: 10.1126/sciimmunol.aah4576
154. Gomez-Lopez N, Romero R, Leng Y, Garcia-Flores V, Xu Y, Miller D, et al. Neutrophil extracellular traps in acute chorioamnionitis: a mechanism of host defense. Am J Reprod Immunol. (2017) 77:e12617. doi: 10.1111/aji.12617
155. Boeckel SRV, Hrabalkova L, Baker TL, Macpherson H, Frew L, Boyle AK, et al. Cathelicidins and the onset of labour. Sci Rep. (2019) 9:7356. doi: 10.1038/s41598-019-43766-7
156. Oh KJ, Park KH, Kim SN, Jeong EH, Lee SY, Yoon HY. Predictive value of intra-amniotic and serum markers for inflammatory lesions of preterm placenta. Placenta. (2011) 32:732–6. doi: 10.1016/j.placenta.2011.07.080
157. Regan JK, Kannan PS, Kemp MW, Kramer BW, Newnham JP, Jobe AH, et al. Damage-associated molecular pattern and fetal membrane vascular injury and collagen disorganization in lipopolysaccharide-induced intra-amniotic inflammation in fetal sheep. Reprod Sci. (2016) 23:69–80. doi: 10.1177/1933719115594014
158. Kumar D, Moore RM, Sharma A, Mercer BM, Mansour JM, Moore JJ. In an in-vitro model using human fetal membranes, alpha-lipoic acid inhibits inflammation induced fetal membrane weakening. Placenta. (2018) 68:9–14. doi: 10.1016/j.placenta.2018.06.305
159. Rinaldi SF, Catalano RD, Wade J, Rossi AG, Norman JE. Decidual neutrophil infiltration is not required for preterm birth in a mouse model of infection-induced preterm labor. J Immunol. (2014) 192:2315–25. doi: 10.4049/jimmunol.1302891
160. Filipovich Y, Agrawal V, Crawford SE, Fitchev P, Qu X, Klein J, et al. Depletion of polymorphonuclear leukocytes has no effect on preterm delivery in a mouse model of Escherichia coli-induced labor. Am J Obstet Gynecol. (2015) 213:697 e691–e610. doi: 10.1016/j.ajog.2015.07.025
161. Bugl S, Wirths S, Radsak MP, Schild H, Stein P, Andre MC, et al. Steady-state neutrophil homeostasis is dependent on TLR4/TRIF signaling. Blood. (2013) 121:723–33. doi: 10.1182/blood-2012-05-429589
162. Zhang D, Chen G, Manwani D, Mortha A, Xu C, Faith JJ, et al. Neutrophil ageing is regulated by the microbiome. Nature. (2015) 525:528–32. doi: 10.1038/nature15367
163. Mantovani A, Cassatella MA, Costantini C, Jaillon S. Neutrophils in the activation and regulation of innate and adaptive immunity. Nat Rev Immunol. (2011) 11:519–31. doi: 10.1038/nri3024
164. Mueller MD, Lebovic DI, Garrett E, Taylor RN. Neutrophils infiltrating the endometrium express vascular endothelial growth factor: potential role in endometrial angiogenesis. Fertil Steril. (2000) 74:107–12. doi: 10.1016/S0015-0282(00)00555-0
165. Gargett CE, Lederman F, Heryanto B, Gambino LS, Rogers PA. Focal vascular endothelial growth factor correlates with angiogenesis in human endometrium. Role of intravascular neutrophils. Hum Reprod. (2001) 16:1065–75. doi: 10.1093/humrep/16.6.1065
166. Amsalem H, Kwan M, Hazan A, Zhang J, Jones RL, Whittle W, et al. Identification of a novel neutrophil population: proangiogenic granulocytes in second-trimester human decidua. J Immunol. (2014) 193:3070–9. doi: 10.4049/jimmunol.1303117
167. Croxatto D, Micheletti A, Montaldo E, Orecchia P, Loiacono F, Canegallo F, et al. Group 3 innate lymphoid cells regulate neutrophil migration and function in human decidua. Mucosal Immunol. (2016) 9:1372–83. doi: 10.1038/mi.2016.10
168. Shynlova O, Nedd-Roderique T, Li Y, Dorogin A, Nguyen T, Lye SJ. Infiltration of myeloid cells into decidua is a critical early event in the labour cascade and post-partum uterine remodelling. J Cell Mol Med. (2013) 17:311–24. doi: 10.1111/jcmm.12012
169. Witko-Sarsat V, Pederzoli-Ribeil M, Hirsch E, Sozzani S, Cassatella MA. Regulating neutrophil apoptosis: new players enter the game. Trends Immunol. (2011) 32:117–24. doi: 10.1016/j.it.2011.01.001
170. Tecchio C, Micheletti A, Cassatella MA. Neutrophil-derived cytokines: facts beyond expression. Front Immunol. (2014) 5:508. doi: 10.3389/fimmu.2014.00508
171. Silvestre-Roig C, Hidalgo A, Soehnlein O. Neutrophil heterogeneity: implications for homeostasis and pathogenesis. Blood. (2016) 127:2173–81. doi: 10.1182/blood-2016-01-688887
172. Reyes L, Wolfe B, Golos T. Hofbauer cells: placental macrophages of fetal origin. Results Probl Cell Differ. (2017) 62:45–60. doi: 10.1007/978-3-319-54090-0_3
173. Yao Y, Xu XH, Jin L. Macrophage polarization in physiological and pathological pregnancy. Front Immunol. (2019) 10:792. doi: 10.3389/fimmu.2019.00792
174. Gomez-Lopez N, Romero R, Leng Y, Xu Y, Slutsky R, Levenson D, et al. The origin of amniotic fluid monocytes/macrophages in women with intra-amniotic inflammation or infection. J Perinat Med. (2019) 47:822–40. doi: 10.1515/jpm-2019-0262
175. Pollard JW, Hunt JS, Wiktor-Jedrzejczak W, Stanley ER. A pregnancy defect in the osteopetrotic (op/op) mouse demonstrates the requirement for CSF-1 in female fertility. Dev Biol. (1991) 148:273–83. doi: 10.1016/0012-1606(91)90336-2
176. Co EC, Gormley M, Kapidzic M, Rosen DB, Scott MA, Stolp HA, et al. Maternal decidual macrophages inhibit NK cell killing of invasive cytotrophoblasts during human pregnancy. Biol Reprod. (2013) 88:155. doi: 10.1095/biolreprod.112.099465
177. Straszewski-Chavez SL, Abrahams VM, Mor G. The role of apoptosis in the regulation of trophoblast survival and differentiation during pregnancy. Endocr Rev. (2005) 26:877–97. doi: 10.1210/er.2005-0003
178. Brown MB, Von Chamier M, Allam AB, Reyes L. M1/M2 macrophage polarity in normal and complicated pregnancy. Front Immunol. (2014) 5:606. doi: 10.3389/fimmu.2014.00606
179. Zhang YH, He M, Wang Y, Liao AH. Modulators of the balance between M1 and M2 macrophages during pregnancy. Front Immunol. (2017) 8:120. doi: 10.3389/fimmu.2017.00120
180. Houser BL, Tilburgs T, Hill J, Nicotra ML, Strominger JL. Two unique human decidual macrophage populations. J Immunol. (2011) 186:2633–42. doi: 10.4049/jimmunol.1003153
181. Jiang X, Du MR, Li M, Wang H. Three macrophage subsets are identified in the uterus during early human pregnancy. Cell Mol Immunol. (2018) 15:1027–37. doi: 10.1038/s41423-018-0008-0
182. Gomez-Lopez N, Estrada-Gutierrez G, Jimenez-Zamudio L, Vega-Sanchez R, Vadillo-Ortega F. Fetal membranes exhibit selective leukocyte chemotaxic activity during human labor. J Reprod Immunol. (2009) 80:122–31. doi: 10.1016/j.jri.2009.01.002
183. Xu Y, Tarquini F, Romero R, Kim CJ, Tarca AL, Bhatti G, et al. Peripheral CD300a+CD8+ T lymphocytes with a distinct cytotoxic molecular signature increase in pregnant women with chronic chorioamnionitis. Am J Reprod Immunol. (2012) 67:184–97. doi: 10.1111/j.1600-0897.2011.01088.x
184. Gomez-Lopez N, Vega-Sanchez R, Castillo-Castrejon M, Romero R, Cubeiro-Arreola K, Vadillo-Ortega F. Evidence for a role for the adaptive immune response in human term parturition. Am J Reprod Immunol. (2013) 69:212–30. doi: 10.1111/aji.12074
185. Gomez-Lopez N, Romero R, Arenas-Hernandez M, Ahn H, Panaitescu B, Vadillo-Ortega F, et al. In vivo T-cell activation by a monoclonal alphaCD3epsilon antibody induces preterm labor and birth. Am J Reprod Immunol. (2016) 76:386–90. doi: 10.1111/aji.12562
186. Gomez-Lopez N, Hernandez-Santiago S, Lobb AP, Olson DM, Vadillo-Ortega F. Normal and premature rupture of fetal membranes at term delivery differ in regional chemotactic activity and related chemokine/cytokine production. Reprod Sci. (2013) 20:276–84. doi: 10.1177/1933719112452473
187. Romero R, Mazor M, Brandt F, Sepulveda W, Avila C, Cotton DB, et al. Interleukin-1 alpha and interleukin-1 beta in preterm and term human parturition. Am J Reprod Immunol. (1992) 27:117–23. doi: 10.1111/j.1600-0897.1992.tb00737.x
188. Tilburgs T, Roelen DL, Van Der Mast BJ, Van Schip JJ, Kleijburg C, De Groot-Swings GM, et al. (2006). Differential distribution of CD4(+)CD25(bright) and CD8(+)CD28(-) T-cells in decidua and maternal blood during human pregnancy. Placenta. 27(Suppl. A):S47–53. doi: 10.1016/j.placenta.2005.11.008
189. Kim MJ, Romero R, Kim CJ, Tarca AL, Chhauy S, Lajeunesse C, et al. Villitis of unknown etiology is associated with a distinct pattern of chemokine up-regulation in the feto-maternal and placental compartments: implications for conjoint maternal allograft rejection and maternal anti-fetal graft-versus-host disease. J Immunol. (2009) 182:3919–27. doi: 10.4049/jimmunol.0803834
190. Tilburgs T, Schonkeren D, Eikmans M, Nagtzaam NM, Datema G, Swings GM, et al. Human decidual tissue contains differentiated CD8+ effector-memory T cells with unique properties. J Immunol. (2010) 185:4470–7. doi: 10.4049/jimmunol.0903597
191. Trowsdale J, Betz AG. Mother's little helpers: mechanisms of maternal-fetal tolerance. Nat Immunol. (2006) 7:241–6. doi: 10.1038/ni1317
192. Moffett-King A. Natural killer cells and pregnancy. Nat Rev Immunol. (2002) 2:656–63. doi: 10.1038/nri886
193. Veenstra Van Nieuwenhoven AL, Heineman MJ, Faas MM. The immunology of successful pregnancy. Hum Reprod Update. (2003) 9:347–57. doi: 10.1093/humupd/dmg026
194. Moffett A, Colucci F. Uterine NK cells: active regulators at the maternal-fetal interface. J Clin Invest. (2014) 124:1872–9. doi: 10.1172/JCI68107
195. Vinketova K, Mourdjeva M, Oreshkova T. Human decidual stromal cells as a component of the implantation niche and a modulator of maternal immunity. J Pregnancy. (2016) 2016:8689436. doi: 10.1155/2016/8689436
196. Volchek M, Girling JE, Lash GE, Cann L, Kumar B, Robson SC, et al. Lymphatics in the human endometrium disappear during decidualization. Hum Reprod. (2010) 25:2455–64. doi: 10.1093/humrep/deq224
197. Deshmukh H, Way SS. Immunological basis for recurrent fetal loss and pregnancy complications. Annu Rev Pathol. (2019) 14:185–210. doi: 10.1146/annurev-pathmechdis-012418-012743
198. Mellor AL, Sivakumar J, Chandler P, Smith K, Molina H, Mao D, et al. Prevention of T cell-driven complement activation and inflammation by tryptophan catabolism during pregnancy. Nat Immunol. (2001) 2:64–8. doi: 10.1038/83183
199. Vacca P, Cantoni C, Vitale M, Prato C, Canegallo F, Fenoglio D, et al. Crosstalk between decidual NK and CD14+ myelomonocytic cells results in induction of Tregs and immunosuppression. Proc Natl Acad Sci USA. (2010) 107:11918–23. doi: 10.1073/pnas.1001749107
200. Sasaki Y, Sakai M, Miyazaki S, Higuma S, Shiozaki A, Saito S. Decidual and peripheral blood CD4+CD25+ regulatory T cells in early pregnancy subjects and spontaneous abortion cases. Mol Hum Reprod. (2004) 10:347–53. doi: 10.1093/molehr/gah044
201. Rolle L, Memarzadeh Tehran M, Morell-Garcia A, Raeva Y, Schumacher A, Hartig R, et al. Cutting edge: IL-10-producing regulatory B cells in early human pregnancy. Am J Reprod Immunol. (2013) 70:448–53. doi: 10.1111/aji.12157
202. Fettke F, Schumacher A, Canellada A, Toledo N, Bekeredjian-Ding I, Bondt A, et al. Maternal and fetal mechanisms of B cell regulation during pregnancy: human chorionic gonadotropin stimulates B cells to produce IL-10 while alpha-fetoprotein drives them into apoptosis. Front Immunol. (2016) 7:495. doi: 10.3389/fimmu.2016.00495
203. Schumacher A, Brachwitz N, Sohr S, Engeland K, Langwisch S, Dolaptchieva M, et al. Human chorionic gonadotropin attracts regulatory T cells into the fetal-maternal interface during early human pregnancy. J Immunol. (2009) 182:5488–97. doi: 10.4049/jimmunol.0803177
204. Schumacher A, Heinze K, Witte J, Poloski E, Linzke N, Woidacki K, et al. Human chorionic gonadotropin as a central regulator of pregnancy immune tolerance. J Immunol. (2013) 190:2650–8. doi: 10.4049/jimmunol.1202698
205. Somerset DA, Zheng Y, Kilby MD, Sansom DM, Drayson MT. Normal human pregnancy is associated with an elevation in the immune suppressive CD25+ CD4+ regulatory T-cell subset. Immunology. (2004) 112:38–43. doi: 10.1111/j.1365-2567.2004.01869.x
206. Kahn DA, Baltimore D. Pregnancy induces a fetal antigen-specific maternal T regulatory cell response that contributes to tolerance. Proc Natl Acad Sci USA. (2010) 107:9299–304. doi: 10.1073/pnas.1003909107
207. Samstein RM, Josefowicz SZ, Arvey A, Treuting PM, Rudensky AY. Extrathymic generation of regulatory T cells in placental mammals mitigates maternal-fetal conflict. Cell. (2012) 150:29–38. doi: 10.1016/j.cell.2012.05.031
208. Shima T, Inada K, Nakashima A, Ushijima A, Ito M, Yoshino O, et al. Paternal antigen-specific proliferating regulatory T cells are increased in uterine-draining lymph nodes just before implantation and in pregnant uterus just after implantation by seminal plasma-priming in allogeneic mouse pregnancy. J Reprod Immunol. (2015) 108:72–82. doi: 10.1016/j.jri.2015.02.005
209. Heitmann RJ, Weitzel RP, Feng Y, Segars JH, Tisdale JF, Wolff EF. Maternal T regulatory cell depletion impairs embryo implantation which can be corrected with adoptive T regulatory cell transfer. Reprod Sci. (2017) 24:1014–24. doi: 10.1177/1933719116675054
210. Rowe JH, Ertelt JM, Aguilera MN, Farrar MA, Way SS. Foxp3(+) regulatory T cell expansion required for sustaining pregnancy compromises host defense against prenatal bacterial pathogens. Cell Host Microbe. (2011) 10:54–64. doi: 10.1016/j.chom.2011.06.005
211. Bao SH, Wang XP, De Lin Q, Wang WJ, Yin GJ, Qiu LH. Decidual CD4+CD25+CD127dim/- regulatory T cells in patients with unexplained recurrent spontaneous miscarriage. Eur J Obstet Gynecol Reprod Biol. (2011) 155:94–8. doi: 10.1016/j.ejogrb.2010.11.007
212. Zhang J, Dunk CE, Shynlova O, Caniggia I, Lye SJ. TGFb1 suppresses the activation of distinct dNK subpopulations in preeclampsia. EBiomedicine. (2019) 39:531–9. doi: 10.1016/j.ebiom.2018.12.015
213. Cerdeira AS, Rajakumar A, Royle CM, Lo A, Husain Z, Thadhani RI, et al. Conversion of peripheral blood NK cells to a decidual NK-like phenotype by a cocktail of defined factors. J Immunol. (2013) 190:3939–48. doi: 10.4049/jimmunol.1202582
214. Koopman LA, Kopcow HD, Rybalov B, Boyson JE, Orange JS, Schatz F, et al. Human decidual natural killer cells are a unique NK cell subset with immunomodulatory potential. J Exp Med. (2003) 198:1201–12. doi: 10.1084/jem.20030305
215. Bernardini G, Sciume G, Bosisio D, Morrone S, Sozzani S, Santoni A. CCL3 and CXCL12 regulate trafficking of mouse bone marrow NK cell subsets. Blood. (2008) 111:3626–34. doi: 10.1182/blood-2007-08-106203
216. Boyman O, Krieg C, Homann D, Sprent J. Homeostatic maintenance of T cells and natural killer cells. Cell Mol Life Sci. (2012) 69:1597–608. doi: 10.1007/s00018-012-0968-7
217. Takashima A, Ishikawa F, Kuwabara T, Tanaka Y, Kinoshita T, Ito M, et al. Uterine natural killer cells severely decrease in number at gestation day 6 in mice. Biol Reprod. (2013) 89:101. doi: 10.1095/biolreprod.113.109009
218. Kalkunte S, Chichester CO, Gotsch F, Sentman CL, Romero R, Sharma S. Evolution of non-cytotoxic uterine natural killer cells. Am J Reprod Immunol. (2008) 59:425–32. doi: 10.1111/j.1600-0897.2008.00595.x
219. Rajagopalan S. HLA-G-mediated NK cell senescence promotes vascular remodeling: implications for reproduction. Cell Mol Immunol. (2014) 11:460–6. doi: 10.1038/cmi.2014.53
220. Ashkar AA, Di Santo JP, Croy BA. Interferon gamma contributes to initiation of uterine vascular modification, decidual integrity, and uterine natural killer cell maturation during normal murine pregnancy. J Exp Med. (2000) 192:259–270. doi: 10.1084/jem.192.2.259
221. Hanna J, Goldman-Wohl D, Hamani Y, Avraham I, Greenfield C, Natanson-Yaron S, et al. Decidual NK cells regulate key developmental processes at the human fetal-maternal interface. Nat Med. (2006) 12:1065–74. doi: 10.1038/nm1452
222. Lash GE, Schiessl B, Kirkley M, Innes BA, Cooper A, Searle RF, et al. Expression of angiogenic growth factors by uterine natural killer cells during early pregnancy. J Leukoc Biol. (2006) 80:572–80. doi: 10.1189/jlb.0406250
223. Yougbare I, Tai WS, Zdravic D, Oswald BE, Lang S, Zhu G, et al. Activated NK cells cause placental dysfunction and miscarriages in fetal alloimmune thrombocytopenia. Nat Commun. (2017) 8:224. doi: 10.1038/s41467-017-00269-1
224. Plaks V, Birnberg T, Berkutzki T, Sela S, Benyashar A, Kalchenko V, et al. Uterine DCs are crucial for decidua formation during embryo implantation in mice. J Clin Invest. (2008) 118:3954–65. doi: 10.1172/JCI36682
225. Blois SM, Klapp BF, Barrientos G. Decidualization and angiogenesis in early pregnancy: unravelling the functions of DC and NK cells. J Reprod Immunol. (2011) 88:86–92. doi: 10.1016/j.jri.2010.11.002
226. Ueda Y, Hagihara M, Okamoto A, Higuchi A, Tanabe A, Hirabayashi K, et al. Frequencies of dendritic cells (myeloid DC and plasmacytoid DC) and their ratio reduced in pregnant women: comparison with umbilical cord blood and normal healthy adults. Hum Immunol. (2003) 64:1144–51. doi: 10.1016/j.humimm.2003.08.342
227. Della Bella S, Giannelli S, Cozzi V, Signorelli V, Cappelletti M, Cetin I, et al. Incomplete activation of peripheral blood dendritic cells during healthy human pregnancy. Clin Exp Immunol. (2011) 164:180–92. doi: 10.1111/j.1365-2249.2011.04330.x
228. Bachy V, Williams DJ, Ibrahim MA. Altered dendritic cell function in normal pregnancy. J Reprod Immunol. (2008) 78:11–21. doi: 10.1016/j.jri.2007.09.004
229. Shah NM, Herasimtschuk AA, Boasso A, Benlahrech A, Fuchs D, Imami N, et al. Changes in T cell and dendritic cell phenotype from mid to late pregnancy are indicative of a shift from immune tolerance to immune activation. Front Immunol. (2017) 8:1138. doi: 10.3389/fimmu.2017.01138
230. Cappelletti M, Giannelli S, Martinelli A, Cetin I, Colombo E, Calcaterra F, et al. Lack of activation of peripheral blood dendritic cells in human pregnancies complicated by intrauterine growth restriction. Placenta. (2013) 34:35–41. doi: 10.1016/j.placenta.2012.10.016
231. Bartmann C, Segerer SE, Rieger L, Kapp M, Sutterlin M, Kammerer U. Quantification of the predominant immune cell populations in decidua throughout human pregnancy. Am J Reprod Immunol. (2014) 71:109–19. doi: 10.1111/aji.12185
232. Brennan PJ, Brigl M, Brenner MB. Invariant natural killer T cells: an innate activation scheme linked to diverse effector functions. Nat Rev Immunol. (2013) 13:101–17. doi: 10.1038/nri3369
233. Ito K, Karasawa M, Kawano T, Akasaka T, Koseki H, Akutsu Y, et al. Involvement of decidual Valpha14 NKT cells in abortion. Proc Natl Acad Sci USA. (2000) 97:740–4. doi: 10.1073/pnas.97.2.740
234. Tsuda H, Sakai M, Michimata T, Tanebe K, Hayakawa S, Saito S. Characterization of NKT cells in human peripheral blood and decidual lymphocytes. Am J Reprod Immunol. (2001) 45:295–302. doi: 10.1111/j.8755-8920.2001.450505.x
235. Boyson JE, Rybalov B, Koopman LA, Exley M, Balk SP, Racke FK, et al. CD1d and invariant NKT cells at the human maternal-fetal interface. Proc Natl Acad Sci USA. (2002) 99:13741–6. doi: 10.1073/pnas.162491699
236. Wang S, Li C, Kawamura H, Watanabe H, Abo T. Unique sensitivity to alpha-galactosylceramide of NKT cells in the uterus. Cell Immunol. (2002) 215:98–105. doi: 10.1016/S0008-8749(02)00009-6
237. Rinaldi SF, Rossi AG, Saunders PT, Norman JE. Immune cells and preterm labour: do invariant NKT cells hold the key? Mol Hum Reprod. (2015) 21:309–12. doi: 10.1093/molehr/gav002
238. Eberl G, Colonna M, Di Santo JP, Mckenzie AN. Innate lymphoid cells. Innate lymphoid cells: a new paradigm in immunology. Science. (2015) 348:aaa6566. doi: 10.1126/science.aaa6566
239. Fang D, Zhu J. Dynamic balance between master transcription factors determines the fates and functions of CD4 T cell and innate lymphoid cell subsets. J Exp Med. (2017) 214:1861–76. doi: 10.1084/jem.20170494
240. Filipovic I, Chiossone L, Vacca P, Hamilton RS, Ingegnere T, Doisne JM, et al. Molecular definition of group 1 innate lymphoid cells in the mouse uterus. Nat Commun. (2018) 9:4492. doi: 10.1038/s41467-018-06918-3
241. Guleria I, Pollard JW. The trophoblast is a component of the innate immune system during pregnancy. Nat Med. (2000) 6:589–93. doi: 10.1038/75074
242. Mor G, Romero R, Aldo PB, Abrahams VM. Is the trophoblast an immune regulator? The role of Toll-like receptors during pregnancy. Crit Rev Immunol. (2005) 25:375–88. doi: 10.1615/CritRevImmunol.v25.i5.30
243. Li C, Houser BL, Nicotra ML, Strominger JL. HLA-G homodimer-induced cytokine secretion through HLA-G receptors on human decidual macrophages and natural killer cells. Proc Natl Acad Sci USA. (2009) 106:5767–72. doi: 10.1073/pnas.0901173106
244. Honig A, Rieger L, Kapp M, Sutterlin M, Dietl J, Kammerer U. Indoleamine 2,3-dioxygenase (IDO) expression in invasive extravillous trophoblast supports role of the enzyme for materno-fetal tolerance. J Reprod Immunol. (2004) 61:79–86. doi: 10.1016/j.jri.2003.11.002
245. Liang SC, Latchman YE, Buhlmann JE, Tomczak MF, Horwitz BH, Freeman GJ, et al. Regulation of PD-1, PD-L1, and PD-L2 expression during normal and autoimmune responses. Eur J Immunol. (2003) 33:2706–16. doi: 10.1002/eji.200324228
246. Roth I, Corry DB, Locksley RM, Abrams JS, Litton MJ, Fisher SJ. Human placental cytotrophoblasts produce the immunosuppressive cytokine interleukin 10. J Exp Med. (1996) 184:539–48. doi: 10.1084/jem.184.2.539
247. Ramhorst R, Fraccaroli L, Aldo P, Alvero AB, Cardenas I, Leiros CP, et al. Modulation and recruitment of inducible regulatory T cells by first trimester trophoblast cells. Am J Reprod Immunol. (2012) 67:17–27. doi: 10.1111/j.1600-0897.2011.01056.x
248. Wang SC, Li YH, Piao HL, Hong XW, Zhang D, Xu YY, et al. PD-1 and Tim-3 pathways are associated with regulatory CD8+ T-cell function in decidua and maintenance of normal pregnancy. Cell Death Dis. (2015) 6:e1738. doi: 10.1038/cddis.2015.112
249. Ristich V, Liang S, Zhang W, Wu J, Horuzsko A. Tolerization of dendritic cells by HLA-G. Eur J Immunol. (2005) 35:1133–42. doi: 10.1002/eji.200425741
250. Tilburgs T, Crespo AC, Van Der Zwan A, Rybalov B, Raj T, Stranger B, et al. Human HLA-G+ extravillous trophoblasts: immune-activating cells that interact with decidual leukocytes. Proc Natl Acad Sci USA. (2015) 112:7219–24. doi: 10.1073/pnas.1507977112
251. Abrahams VM, Visintin I, Aldo PB, Guller S, Romero R, Mor G. A role for TLRs in the regulation of immune cell migration by first trimester trophoblast cells. J Immunol. (2005) 175:8096–104. doi: 10.4049/jimmunol.175.12.8096
252. Fest S, Aldo PB, Abrahams VM, Visintin I, Alvero A, Chen R, et al. Trophoblast-macrophage interactions: a regulatory network for the protection of pregnancy. Am J Reprod Immunol. (2007) 57:55–66. doi: 10.1111/j.1600-0897.2006.00446.x
253. Gupta AK, Hasler P, Holzgreve W, Gebhardt S, Hahn S. Induction of neutrophil extracellular DNA lattices by placental microparticles and IL-8 and their presence in preeclampsia. Hum Immunol. (2005) 66:1146–54. doi: 10.1016/j.humimm.2005.11.003
254. Petty HR, Kindzelskii AL, Espinoza J, Romero R. Trophoblast contact deactivates human neutrophils. J Immunol. (2006) 176:3205–14. doi: 10.4049/jimmunol.176.5.3205
255. Calo G, Sabbione F, Vota D, Paparini D, Ramhorst R, Trevani A, et al. Trophoblast cells inhibit neutrophil extracellular trap formation and enhance apoptosis through vasoactive intestinal peptide-mediated pathways. Hum Reprod. (2017) 32:55–64. doi: 10.1093/humrep/dew292
256. Gillaux C, Mehats C, Vaiman D, Cabrol D, Breuiller-Fouche M. Functional screening of TLRs in human amniotic epithelial cells. J Immunol. (2011) 187:2766–74. doi: 10.4049/jimmunol.1100217
257. Mitchell CM, Hirst JJ, Mitchell MD, Murray HG, Zakar T. Genes upregulated in the amnion at labour are bivalently marked by activating and repressive histone modifications. Mol Hum Reprod. (2019) 25:228–40. doi: 10.1093/molehr/gaz007
258. Anders AP, Gaddy JA, Doster RS, Aronoff DM. Current concepts in maternal-fetal immunology: Recognition and response to microbial pathogens by decidual stromal cells. Am J Reprod Immunol. (2017) 77. doi: 10.1111/aji.12623
259. Croxatto D, Vacca P, Canegallo F, Conte R, Venturini PL, Moretta L, et al. Stromal cells from human decidua exert a strong inhibitory effect on NK cell function and dendritic cell differentiation. PLoS ONE. (2014) 9:e89006. doi: 10.1371/journal.pone.0089006
260. Rogers LM, Anders AP, Doster RS, Gill EA, Gnecco JS, Holley JM, et al. Decidual stromal cell-derived PGE2 regulates macrophage responses to microbial threat. Am J Reprod Immunol. (2018) 80:e13032. doi: 10.1111/aji.13032
261. Lockwood CJ, Murk WK, Kayisli UA, Buchwalder LF, Huang SJ, Arcuri F, et al. Regulation of interleukin-6 expression in human decidual cells and its potential role in chorioamnionitis. Am J Pathol. (2010) 177:1755–64. doi: 10.2353/ajpath.2010.090781
262. Guzeloglu-Kayisli O, Kayisli UA, Semerci N, Basar M, Buchwalder LF, Buhimschi CS, et al. Mechanisms of chorioamnionitis-associated preterm birth: interleukin-1beta inhibits progesterone receptor expression in decidual cells. J Pathol. (2015) 237:423–34. doi: 10.1002/path.4589
263. Van Den Broek NR, White SA, Goodall M, Ntonya C, Kayira E, Kafulafula G, et al. The APPLe study: a randomized, community-based, placebo-controlled trial of azithromycin for the prevention of preterm birth, with meta-analysis. PLoS Med. (2009) 6:e1000191. doi: 10.1371/journal.pmed.1000191
264. Subramaniam A, Abramovici A, Andrews WW, Tita AT. Antimicrobials for preterm birth prevention: an overview. Infect Dis Obstet Gynecol. (2012) 2012:157159. doi: 10.1155/2012/157159
265. Dasari S, Pereira L, Reddy AP, Michaels JE, Lu X, Jacob T, et al. Comprehensive proteomic analysis of human cervical-vaginal fluid. J Proteome Res. (2007) 6:1258–68. doi: 10.1021/pr0605419
266. Kenyon S, Pike K, Jones DR, Brocklehurst P, Marlow N, Salt A, et al. Childhood outcomes after prescription of antibiotics to pregnant women with spontaneous preterm labour: 7-year follow-up of the ORACLE II trial. Lancet. (2008) 372:1319–27. doi: 10.1016/S0140-6736(08)61203-9
267. Ireland DJ, Nathan EA, Li S, Charles AK, Stinson LF, Kemp MW, et al. Preclinical evaluation of drugs to block inflammation-driven preterm birth. Innate Immun. (2017) 23:20–33. doi: 10.1177/1753425916672313
268. Dorrington MG, Fraser IDC. NF-kappaB signaling in macrophages: dynamics, crosstalk, and signal integration. Front Immunol. (2019) 10:705. doi: 10.3389/fimmu.2019.00705
269. Lappas M, Permezel M, Rice GE. N-Acetyl-cysteine inhibits phospholipid metabolism, proinflammatory cytokine release, protease activity, and nuclear factor-kappaB deoxyribonucleic acid-binding activity in human fetal membranes in vitro. J Clin Endocrinol Metab. (2003) 88:1723–9. doi: 10.1210/jc.2002-021677
270. Beloosesky R, Gayle DA, Ross MG. Maternal N-acetylcysteine suppresses fetal inflammatory cytokine responses to maternal lipopolysaccharide. Am J Obstet Gynecol. (2006) 195:1053–7. doi: 10.1016/j.ajog.2006.06.081
271. Paintlia MK, Paintlia AS, Singh AK, Singh I. Attenuation of lipopolysaccharide-induced inflammatory response and phospholipids metabolism at the feto-maternal interface by N-acetyl cysteine. Pediatr Res. (2008) 64:334–9. doi: 10.1203/PDR.0b013e318181e07c
272. Chang EY, Zhang J, Sullivan S, Newman R, Singh I. N-acetylcysteine attenuates the maternal and fetal proinflammatory response to intrauterine LPS injection in an animal model for preterm birth and brain injury. J Matern Fetal Neonatal Med. (2011) 24:732–40. doi: 10.3109/14767058.2010.528089
273. Shahin AY, Hassanin IM, Ismail AM, Kruessel JS, Hirchenhain J. Effect of oral N-acetyl cysteine on recurrent preterm labor following treatment for bacterial vaginosis. Int J Gynaecol Obstet. (2009) 104:44–8. doi: 10.1016/j.ijgo.2008.08.026
274. Keelan JA, Khan S, Yosaatmadja F, Mitchell MD. Prevention of inflammatory activation of human gestational membranes in an ex vivo model using a pharmacological NF-kappaB inhibitor. J Immunol. (2009) 183:5270–8. doi: 10.4049/jimmunol.0802660
275. De Silva D, Mitchell MD, Keelan JA. Inhibition of choriodecidual cytokine production and inflammatory gene expression by selective I-kappaB kinase (IKK) inhibitors. Br J Pharmacol. (2010) 160:1808–22. doi: 10.1111/j.1476-5381.2010.00839.x
276. Ireland DJ, Kemp MW, Miura Y, Saito M, Newnham JP, Keelan JA. Intra-amniotic pharmacological blockade of inflammatory signalling pathways in an ovine chorioamnionitis model. Mol Hum Reprod. (2015) 21:479–89. doi: 10.1093/molehr/gav005
277. Sadowsky DW, Haluska GJ, Gravett MG, Witkin SS, Novy MJ. Indomethacin blocks interleukin 1beta-induced myometrial contractions in pregnant rhesus monkeys. Am J Obstet Gynecol. (2000) 183:173–80. doi: 10.1067/mob.2000.105968
278. Gravett MG, Adams KM, Sadowsky DW, Grosvenor AR, Witkin SS, Axthelm MK, et al. Immunomodulators plus antibiotics delay preterm delivery after experimental intraamniotic infection in a nonhuman primate model. Am J Obstet Gynecol. (2007) 197:518 e511–518. doi: 10.1016/j.ajog.2007.03.064
279. Kaplan BS, Restaino I, Raval DS, Gottlieb RP, Bernstein J. Renal failure in the neonate associated with in utero exposure to non-steroidal anti-inflammatory agents. Pediatr Nephrol. (1994) 8:700–4. doi: 10.1007/BF00869093
280. Renaud SJ, Cotechini T, Quirt JS, Macdonald-Goodfellow SK, Othman M, Graham CH. Spontaneous pregnancy loss mediated by abnormal maternal inflammation in rats is linked to deficient uteroplacental perfusion. J Immunol. (2011) 186:1799–808. doi: 10.4049/jimmunol.1002679
281. Gelber SE, Brent E, Redecha P, Perino G, Tomlinson S, Davisson RL, et al. Prevention of defective placentation and pregnancy loss by blocking innate immune pathways in a syngeneic model of placental insufficiency. J Immunol. (2015) 195:1129–38. doi: 10.4049/jimmunol.1402220
282. Winger EE, Reed JL. Treatment with tumor necrosis factor inhibitors and intravenous immunoglobulin improves live birth rates in women with recurrent spontaneous abortion. Am J Reprod Immunol. (2008) 60:8–16. doi: 10.1111/j.1600-0897.2008.00585.x
283. Romero R, Mazor M, Tartakovsky B. Systemic administration of interleukin-1 induces preterm parturition in mice. Am J Obstet Gynecol. (1991) 165:969–71. doi: 10.1016/0002-9378(91)90450-6
284. Hirsch E, Filipovich Y, Mahendroo M. Signaling via the type I IL-1 and TNF receptors is necessary for bacterially induced preterm labor in a murine model. Am J Obstet Gynecol. (2006) 194:1334–40. doi: 10.1016/j.ajog.2005.11.004
285. Sadowsky DW, Adams KM, Gravett MG, Witkin SS, Novy MJ. Preterm labor is induced by intraamniotic infusions of interleukin-1beta and tumor necrosis factor-alpha but not by interleukin-6 or interleukin-8 in a nonhuman primate model. Am J Obstet Gynecol. (2006) 195:1578–89. doi: 10.1016/j.ajog.2006.06.072
286. Kallapur SG, Nitsos I, Moss TJ, Polglase GR, Pillow JJ, Cheah FC, et al. IL-1 mediates pulmonary and systemic inflammatory responses to chorioamnionitis induced by lipopolysaccharide. Am J Respir Crit Care Med. (2009) 179:955–61. doi: 10.1164/rccm.200811-1728OC
287. Girard S, Tremblay L, Lepage M, Sebire G. IL-1 receptor antagonist protects against placental and neurodevelopmental defects induced by maternal inflammation. J Immunol. (2010) 184:3997–4005. doi: 10.4049/jimmunol.0903349
288. Nadeau-Vallee M, Quiniou C, Palacios J, Hou X, Erfani A, Madaan A, et al. Novel noncompetitive IL-1 receptor-biased ligand prevents infection- and inflammation-induced preterm birth. J Immunol. (2015) 195:3402–15. doi: 10.4049/jimmunol.1500758
289. Nadeau-Vallee M, Chin PY, Belarbi L, Brien ME, Pundir S, Berryer MH, et al. Antenatal suppression of IL-1 protects against inflammation-induced fetal injury and improves neonatal and developmental outcomes in mice. J Immunol. (2017) 198:2047–62. doi: 10.4049/jimmunol.1601600
290. Lei J, Vermillion MS, Jia B, Xie H, Xie L, Mclane MW, et al. IL-1 receptor antagonist therapy mitigates placental dysfunction and perinatal injury following Zika virus infection. JCI Insight. (2019) 4. doi: 10.1172/jci.insight.122678
291. Leitner K, Al Shammary M, Mclane M, Johnston MV, Elovitz MA, Burd I. IL-1 receptor blockade prevents fetal cortical brain injury but not preterm birth in a mouse model of inflammation-induced preterm birth and perinatal brain injury. Am J Reprod Immunol. (2014) 71:418–26. doi: 10.1111/aji.12216
292. Dinarello CA, Simon A, Van Der Meer JW. Treating inflammation by blocking interleukin-1 in a broad spectrum of diseases. Nat Rev Drug Discov. (2012) 11:633–52. doi: 10.1038/nrd3800
Keywords: inflammation, infection, immune cells, animal model, fetal membrane
Citation: Cappelletti M, Presicce P and Kallapur SG (2020) Immunobiology of Acute Chorioamnionitis. Front. Immunol. 11:649. doi: 10.3389/fimmu.2020.00649
Received: 07 December 2019; Accepted: 23 March 2020;
Published: 16 April 2020.
Edited by:
Nardhy Gomez-Lopez, Wayne State University, United StatesReviewed by:
Indira Mysorekar, Washington University School of Medicine in St. Louis, United StatesKristina M. Adams Waldorf, University of Washington, United States
Copyright © 2020 Cappelletti, Presicce and Kallapur. This is an open-access article distributed under the terms of the Creative Commons Attribution License (CC BY). The use, distribution or reproduction in other forums is permitted, provided the original author(s) and the copyright owner(s) are credited and that the original publication in this journal is cited, in accordance with accepted academic practice. No use, distribution or reproduction is permitted which does not comply with these terms.
*Correspondence: Suhas G. Kallapur, c2thbGxhcHVyQG1lZG5ldC51Y2xhLmVkdQ==
†These authors have contributed equally to this work