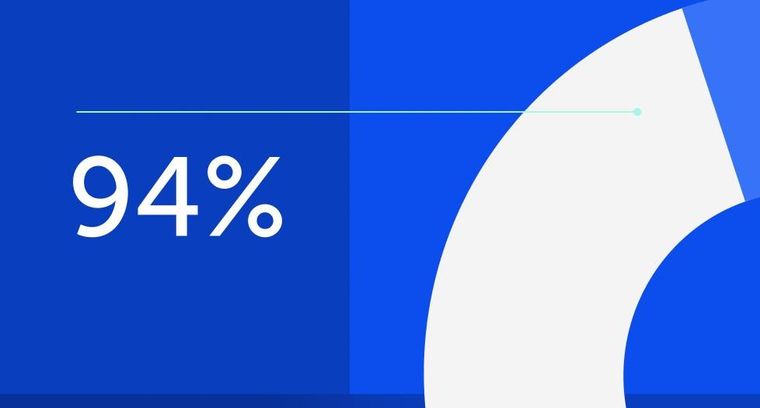
94% of researchers rate our articles as excellent or good
Learn more about the work of our research integrity team to safeguard the quality of each article we publish.
Find out more
MINI REVIEW article
Front. Immunol., 31 March 2020
Sec. Molecular Innate Immunity
Volume 11 - 2020 | https://doi.org/10.3389/fimmu.2020.00553
This article is part of the Research TopicPhagocytosis: Molecular Mechanisms and Physiological ImplicationsView all 19 articles
Efficient inflammation resolution is important not only for the termination of the inflammatory response but also for the restoration of tissue integrity. An integral process to resolution of inflammation is the phagocytosis of dying cells by macrophages, known as efferocytosis. This function is mediated by a complex and well-orchestrated network of interactions amongst specialized phagocytic receptors, bridging molecules, as well as “find-me” and “eat-me” signals. Efferocytosis serves not only as a waste disposal mechanism (clearance of the apoptotic cells) but also promotes a pro-resolving phenotype in efferocytic macrophages and thereby termination of inflammation. Alterations in cellular metabolism are critical for shaping the phenotype and function of efferocytic macrophages, thus, representing an important determinant of macrophage plasticity. Impaired efferocytosis can result in inflammation-associated pathologies or autoimmunity. The present mini review summarizes current knowledge regarding the mechanisms regulating macrophage efferocytosis during clearance of inflammation.
Specific recognition and engulfment of “foreign” material or pathogens by host cells, designated as phagocytosis, is an essential process modulating the immune response and tissue homeostasis (1, 2). Besides phagocytosis of opsonized pathogens by phagocytes during an infection, host cells undergoing apoptosis are also cleared by macrophages; the specific phagocytosis of dying cells by macrophages is designated efferocytosis (3). The specific pathways for phagocytosis of different types of cargo point to the versatility of the phagocytic machinery (1, 2).
A central player in sterile inflammation or inflammation associated with infection are neutrophils recruited to the inflamed site (4, 5). Recruited neutrophils phagocytose and kill pathogens, produce reactive oxygen species (ROS) and pro-inflammatory factors, such as cytokines, and can either release neutrophil extracellular traps (NETs) or undergo apoptosis (4–6). Efferocytosis is therefore of great importance in the regulation of neutrophilic inflammation (3, 7–10). Effective removal of dying neutrophils promotes not only inflammation resolution but also contributes to restoration of tissue and organ homeostasis (3). A complex network of interactions between receptors mediating phagocytosis, bridging molecules, “find-me” and “eat-me” signals, such as phosphatidylserine (PS), which is presented on the outer part of the membrane of cells undergoing apoptosis, contributes to the formation of phagocytic synapse (Figure 1) and the operation of the efferocytic machinery (7, 8, 11). As resolution of inflammation occurs, efferocytic macrophages acquire a resolving phenotype producing factors that dampen inflammation and promote restoration of tissue integrity, such as IL-10 or transforming growth factor β (TGFβ) (3), as as well as specialized pro-resolving lipid mediators (SPM), such as resolvins, lipoxins, and maresins (9, 12). SPM synthesis can further promote efferocytosis, thereby further potentiating inflammation resolution (9, 12). Defective removal of apoptotic cells resulting from impaired efferocytosis can lead to chronicity of inflammation and development of inflammatory disorders, such as atherosclerosis and autoimmune diseases (9, 13–15).
Figure 1. The structure of engulfment synapse. During efferocytosis, the clearance of apoptotic cells by macrophages is orchestrated by the recognition of the major “eat-me” signal PS, either directly by PS receptors or via bridging molecules that mediate binding of PS to phagocytic receptors. Depicted are a few examples of phagocytic receptors and bridging molecules that are described in the text (see text for primary references).
Emerging evidence suggests that macrophage function is regulated by alterations in their cellular metabolism in response to environmental cues within the inflammatory milieu (16). For instance, specific metabolic components may promote or suppress inflammatory responses in macrophages (16). Importantly, efferocytosis also promotes immunometabolic reprograming in macrophages (17) (Figure 2). For example, digestion of the engulfed apoptotic cargo through phagolysosomal activity results in a load of lipid components derived from the apoptotic cell membranes that is linked to enhanced fatty acid oxidation and regulates macrophage function (17–19). The mechanisms underlying apoptotic cell removal by macrophages as well as the immunometabolic alterations in efferocytic macrophages during inflammation resolution is the focus of the present review.
Figure 2. Metabolic cues implicated in macrophage efferocytosis. Upon efferocytosis, increased glucose uptake via upregulated SLC2A1 and enhanced glycolysis are linked with enhanced lactate release via SLC16A (102). The metabolism of arginine and ornithine to putrescine is involved in promoting continual efferocytosis (105). In addition, enhanced lipid metabolism upon efferocytosis is associated with fatty acid oxidation and Sirtuin 1 (Sirt1)-dependent upregulation of IL-10 (18). Moreover, activation of lipid transcription factors (e.g., the LXR/RXR or PPAR/RXR heterodimers) promotes the upregulation of bridging molecules and phagocytic receptors and the resolving macrophage phenotype (107).
The engulfment of dying cells by efferocytic macrophages requires the recognition of the former by the latter and the formation of the engulfment synapse, which is regulated by a network of “find-me,” “eat-me” and bridging molecules, “don’t eat-me” signals and specialized phagocytic receptors (11) (Figure 1). Neutrophils undergoing apoptosis during an inflammatory response, secrete molecules serving as “find-me” signals that can attract phagocytes to eliminate apoptotic cell corpses (20, 21). These include the nucleotides adenosine triphosphate (ATP) and uridine triphosphate (UTP), which are recognized by the macrophage purinergic receptor P2Y2 (22), or the lipids lysophosphatidylcholine (LPC) (23) and sphingosine-1-phosphate (24), which bind to macrophage G-protein-coupled receptors G2A and S1P1-5, respectively. Furthermore, recognition of dying cells by macrophages may be facilitated by the interaction of intercellular adhesion molecule 3 (ICAM3 or CD50) on the former with CD14 on macrophages (25) as well as by the thrombospondin (TSP1)–CD36 interaction (26). Moreover, the specific recognition of apoptotic cells is ensured by the presence of “eat-me” signals. PS is the most well characterized “eat-me” signal (Figure 1). During apoptosis, this phospholipid is found on the outer part of the membrane and binds directly or indirectly, via bridging molecules (opsonins), to phagocytic receptors (27, 28). Calreticulin (Crt) is a membrane-associated protein that functions as an “eat-me” signal’ on the surface of dying cells and is recognized by the LDL-receptor-related protein 1 (LRP1 or CD91) on phagocytes (29). The long pentraxin PTX3 may also act as an “eat-me” signal to facilitate the capture of dying neutrophils by macrophages (30).
As alluded to above, bridging molecules are often key to efficient interactions between apoptotic neutrophils and macrophages (11). Milk fat globule-EGF factor 8 protein (MFG-E8 or lactadherin) promotes efferocytosis by binding to PS on apoptotic cells and to macrophage phagocytic integrin receptors αvβ3 and αvβ5 (31, 32). MFG-E8 shares homology with developmental endothelial locus-1 (DEL-1). Besides the established anti-inflammatory role of DEL-1 as inhibitor of β2 integrin-dependent leukocyte recruitment and IL-17-mediated inflammation (8, 33–38) and its role as a regulator of bone marrow myelopoiesis (39, 40), secreted DEL-1 promotes engulfment of apoptotic cells and inflammation resolution (41). In particular, DEL-1 functions as a molecular bridge that binds concomitantly to PS on the apoptotic neutrophil surface via its C-terminal discoidin I-like domains and to αvβ3 integrin [also known as vitronectin receptor (42)] on macrophages via its N-terminal RGD motif within the second EGF-like domain (41, 43). Importantly, the compartmentalized localization of DEL-1 differentially regulates the inflammatory response. Specifically, the endothelial cell-derived molecule promotes anti-inflammatory activity by blocking β2 integrin-dependent leukocyte recruitment, whereas DEL-1 derived from macrophages promotes efferocytosis-dependent resolution of inflammation (33, 41) (Figure 1). Other molecules that act as a molecular bridge to facilitate interactions between apoptotic cargo and phagocytes include annexin A1 or lipocortin-1 (44), β2-glycoprotein-I (β2-GPI) (45), and galectin-3 (Gal-3) (46). Moreover, the bridging molecules Growth arrest-specific factor 6 (Gas6) and protein S have been implicated in PS-mediated apoptotic cell clearance (47, 48) via interacting with the receptor tyrosine kinases Tyro-3, Axl and Mer (TAM) (49–52) (Figure 1).
Phagocytic receptors on macrophages involved in the regulation of efferocytosis include also the PS receptors of the T-cell membrane protein (Tim) family, such as TIM-1 and TIM-4 (53, 54). The brain angiogenesis inhibitor 1 (BAI1) (55) and stabilin-2 (56) also serve as PS receptors (Figure 1). Additionally, CD14, the scavenger receptor CD36, and the integrin CD11b/CD18 (αMβ2) (besides the integrins αvβ5 and αvβ3 that were mentioned above) are implicated as efferocytosis receptors (8, 11, 26, 42, 57, 58).
The presence of “don’t eat-me” signals further adds to the complexity of the regulation of apoptotic cell clearance. Specifically, surface expression of CD47 (also named integrin-associated protein) prevents phagocytosis by macrophages (59, 60). Binding of CD47 to the macrophage signal regulatory protein alpha (SIRPα) modulates rearrangement of actin cytoskeleton, thereby downregulating phagocytosis (59, 60). However, apoptotic cells have decreased levels of CD47 that allows their clearance by macrophages (59–61). Platelet and endothelial cell adhesion molecule 1 (PECAM-1, CD31) also exerts a “don’t eat-me” function. In this regard, homotypic CD31 interaction between non-apoptotic neutrophils and macrophages may prevent phagocytic clearance (62). Furthermore, CD24 (63) and the complement receptor CD46 (64) have been described as repulsive signals that interfere with efferocytosis. Decreased presence or alterations in the distribution of “don’t eat-me” signals have been associated with enhanced efferocytic activity (65, 66).
Efficient efferocytosis is critical for shaping the pro-resolving phenotype in macrophages that includes production of immunomodulators, which in turn further enhance resolution of inflammation (67–71). For instance, production of TGFβ by efferocytic macrophages is a major orchestrator of inflammation resolution. Indeed, upregulation of TGFβ owing to efferocytosis promotes downregulation of the pro-inflammatory mediators TNF, IL-1β and IL-8. Consistently, antibody-mediated inhibition of TGFβ restored expression of inflammatory mediators. Along the same line, administration of apoptotic cells in vivo models of inflammation triggers resolution of inflammation in a manner dependent on TGFβ upregulation (72, 73). Additionally, interleukin 13 derived from regulatory T cells acts on macrophages and promotes production of IL-10, which in turn enhances efferocytosis via activation of Rac1 GTPase and thereby inflammation resolution in atherosclerosis (74). Besides the upregulation of immune-modulating factors, such as TGFβ or IL-10, the direct inhibition of pro-inflammatory cytokines further contributes to inflammation resolution. As an exemplar, formation of NETs that aggregate at the inflamed site leads to protease–dependent degradation of inflammatory cytokines and chemokines, thereby promoting resolution of acute neutrophilic inflammation (75).
The phenotype of efferocytic macrophages is additionally controlled by the enzyme 12/15 lipoxygenase (12/15-LO) that oxidizes polyunsaturated fatty acids and generates bioactive lipid metabolites leading to the biosynthesis of pro-resolving lipid mediators (9, 76). Specifically, apoptotic cell engulfment can be performed by resident or monocyte-derived resolution phase macrophages expressing 12/15-LO (77–80). Apoptotic cell engulfment further promotes the expression of this enzyme (81). Moreover, 12/15-LO has been implicated to function in preventing induction of autoimmunity (77).
Plasminogen and its cleavage product plasmin not only regulate the initiation but also the resolution phase of inflammation. Treatment of mice with plasminogen/plasmin resulted in recruitment of pro-resolving macrophages and in upregulation of TGFβ. Administration of plasminogen/plasmin at the peak of inflammation was associated with increased neutrophil apoptosis and efferocytosis; the pro-resolving effect of plasminogen was mediated by annexin A1 (82). In accordance, impaired efferocytosis accompanied by decreased levels of annexin A1 was observed in mice deficient in plasminogen or its receptor (83). Besides being involved in pro-resolving actions of plasminogen, annexin A1 plays a broader role in inflammation resolution (84, 85). Annexin A1 levels are increased in the resolution phase of monosodium urate crystal–induced arthritis, a model of gout. Pharmacologic or genetic inactivation of annexin A1 resulted in insufficient resolution of gout-related inflammation in mice (86). In addition, treatment of mice with annexin A1 resulted in upregulation of IL-10 and downregulation of proinflammatory mediators, while, consistently, inhibition of annexin A1 abrogated inflammation resolution induced by glucocorticoids (87, 88).
Moreover, IFN-β from macrophages was recently identified as a factor promoting resolution of inflammation. IFN-β levels were higher in the resolution phase of pneumonia and peritoneal inflammation. Activation of IFN-β signaling via STAT3 enhances apoptosis of neutrophils and their subsequent efferocytic clearance, resulting in a pro-resolving reprograming of macrophages (79).
The impact of cellular metabolism on macrophage function and plasticity has gained much attention recently (16, 89–91). Metabolic pathways, such as glycolysis, tricarboxylic acid (TCA) cycle, pentose phosphate pathway and fatty acid oxidation, regulate macrophage phenotype in the context of inflammatory responses (91). For instance, increased glycolytic flux has been linked to pro-inflammatory M1-like activation of macrophages, whereas oxidative phosphorylation is associated with anti-inflammatory macrophage polarization (92).
Moreover, macrophage tissue specificity may be associated with differential metabolic activity. For example, resident peritoneal macrophage survival depends on the transcription factor GATA6 that is regulated by the vitamin A metabolite retinoic acid (93, 94), while the nuclear receptor liver × receptor (LXR) alpha that is activated by lipids regulates differentiation of marginal zone splenic macrophages (95). It is now established that tissue-specific resident macrophages have distinct transcriptomic profiles and phenotypes depending on the particular microenvironment (96, 97). Importantly, in this regard, the manner by which efferocytosis is regulated in resident macrophages may be dictated by the tissue microenvironment. Indeed, parabiosis-based experiments have revealed substantial heterogeneity in the utilization of bridging molecules, efferocytic receptors and transcription factors by macrophages from different tissues. For instance, the mannose receptor CD206 is upregulated in phagocytic macrophages in bone marrow and intestine but not in the spleen (98). Moreover, the profile of upregulated anti-inflammatory mediators by efferocytic macrophages is tissue-specific (98).
Regulation of macrophage metabolic activity in the context of efferocytosis (Figure 2) is of major importance for the outcome of inflammation resolution and tissue repair (99). Following engulfment, macrophages degrade the apoptotic material through phagolysosomal activity (100, 101), resulting in substantial metabolic load and influence on macrophage metabolism (17).
Aerobic glycolysis was recently implicated in regulation of efferocytosis and shaping an anti-inflammatory environment by efferocytic macrophages (102). Specifically, transcriptomic analysis of macrophages engaging in active phagocytosis of apoptotic cells revealed an upregulation of several members of the solute carrier (SLC) membrane transport protein family, including the glucose transporter protein type 1 (GLUT1; encoded by the gene Slc2a1) and monocarboxylate transporter 1 (encoded by Slc16a1) promoting lactate release. Enhanced glycolysis in efferocytic macrophages promoted actin polymerization and continued uptake of apoptotic cells. On the other hand, lactate from efferocytic macrophages contributed to establishment of an anti-inflammatory phenotype of the surrounding tissue (102) (Figure 2). Consistently, knockdown of Slc16a1 in the setting of efferocytosis resulted in reduced mRNA expression of factors linked to the resolving macrophage phenotype and resolution of inflammation, such as Tgfb1 and Il10 (102). Furthermore, specific deletion of GLUT1 in myeloid cells was associated with defective phagocytic ability of macrophages and with development of unstable lesions in a model of atherosclerosis (103). Moreover, deficiency of the glycolytic enzyme 6-phosphofructose-2-kinase and fructose-2,6-bisphosphatase (PFKFB3) in macrophages led to reduced efferocytosis capacity, thus further supporting a role for glycolysis in apoptotic cell clearance (104).
Metabolites derived from engulfed apoptotic cells serve to fine-tune the process of efferocytosis and resolution of inflammation. Apoptotic cells are a source of the amino acids arginine and ornithine that are metabolized in macrophages to putrescine. This metabolic process enables continual rounds of efferocytosis (Figure 2). Putrescine enhances, via the RNA-binding protein HuR, the mRNA stabilization of the GTP-exchange factor Dbl. Dbl in turn activates the GTPase Rac1, resulting in changes in the actin cytoskeleton that facilitate further apoptotic cell engulfment. Exogenous administration of putrescine increases inflammation resolution in the context of atherosclerosis. Consistently, deficiency in myeloid cells of either the enzyme arginase 1 (Arg1), which converts arginine to ornithine, or the enzyme ornithine decarboxylase (ODC), which mediates the decarboxylation of ornithine to putrescine (Figure 2), is associated with efferocytic dysfunction and defective atherosclerosis resolution (105).
Several lines of evidence support also an important role of mitochondrial metabolism on the modulation of efferocytosis and efferocytosis-dependent resolution of inflammation. Metabolomic analysis of macrophages that have engulfed apoptotic material revealed metabolic reprograming of efferocytic macrophages toward fatty acid oxidation. Specifically, upregulation of pro-resolving IL-10 in efferocytic macrophages was mediated by mitochondrial beta-oxidation and induction of sirtuin1 signaling (18) (Figure 2). The interplay between mitochondrial activity and effective efferocytosis was also illustrated by analyzing the function of mitochondrial uncoupling protein 2 (Ucp2). Besides its function in uncoupling oxidative phosphorylation from synthesis of ATP, Ucp2 can promote efferocytosis by reducing the mitochondrial membrane potential of macrophages. In the same vein, deficiency of Ucp2 resulted in defective apoptotic cell removal and was associated with development of atherosclerosis, whereas overexpression of Ucp2 enhanced efferocytosis (19). Additionally, dynamic alterations in mitochondrial physiology may dictate the outcome of efferocytosis. In particular, a major component of mitochondrial homeostasis, mitochondrial fission, mediated by the function of the GTPase dynamin-related protein 1 (Drp1), positively regulates continuous apoptotic cell removal by macrophages. Accordingly, the absence of Drp1 was associated with higher atherosclerosis development in low-density lipoprotein receptor 1 (Ldlr1) deficient mice (106).
Lipids deriving from the apoptotic cargo are abundant post-engulfment digestion products (17); lipid metabolism induces activation of the nuclear receptors peroxisome proliferator-activated receptor (PPAR) gamma and delta, LXR alpha and beta and retinoid × receptor (RXR) alpha and beta (107) in macrophages (Figure 2). Activation of these transcription factors promotes upregulation of phagocytic receptors and bridging molecules and the resolving macrophage phenotype. For instance, TGFβ and IL-10, which both promote inflammation resolution, are upregulated in efferocytic macrophages in an LXR- and PPAR delta–dependent manner (108, 109). Importantly, deficiency of these nuclear receptors has been linked to defective efferocytosis and development of chronic inflammation or autoimmune manifestations (108, 109). Moreover, LXR activation is involved in DEL-1–dependent efferocytosis and macrophage reprograming to a proresolving phenotype (41). LXR signaling also regulates expression of transglutaminase 2 (Tgm2) (110), which acts as a co-receptor to αvβ3-integrin and promotes formation of engulfing portals (111).
Cholesterol metabolism has been also implicated in the modulation of efferocytosis. Treatment of macrophages with the cholesterol-lowering drug lovastatin, which inhibits the rate-limiting enzyme of cholesterol synthesis 3-hydroxyl-3-methylglutaryl coenzyme A (HMG-CoA) reductase, leads to increased apoptotic cell clearance (112). Administration of another HMG-CoA reductase inhibitor, simvastatin, enhances the efferocytosis-dependent amelioration of inflammation in the context of lung fibrosis (113). Along the same line, the ATP-binding cassette transporter (ABCA1), a protein that modulates cholesterol efflux, is upregulated during efferocytosis in a manner dependent on LXR signaling (114). Furthermore, decreased hydrolysis of cholesterol esters and impaired oxysterol production, due to blockade of the enzyme lysosomal acid lipase, negatively affect LXR pathway activation and apoptotic cell removal, resulting in chronic inflammation (115). These studies point to the intimate crosstalk between cholesterol metabolism and nuclear receptor signaling involved in efferocytosis.
Macrophage efferocytosis is a major player in resolution of inflammation. Efferocytosis not only paves the way toward the timely termination of the inflammatory response, but also promotes restoration of tissue homeostasis. In this context, alterations in macrophage cellular metabolism are important regulators of efferocytosis. At the same time, metabolic reprograming in efferocytic macrophages induced by digested apoptotic material substantially regulates the function and plasticity of efferocytic macrophages. Given the relevance of efferocytosis as a mechanism against chronic inflammatory disease, a better mechanistic understanding of the pathways that orchestrate the mutual interaction between clearance of dying cells and metabolic alterations in macrophages is required. This knowledge will provide a scaffold for designing therapeutic approaches to improve macrophage function in inflammation resolution and harness macrophage efferocytosis for the treatment of pathologies associated with chronic inflammation or autoimmunity.
IK, GH, and TC wrote the manuscript.
The authors were supported by grants from the National Institutes of Health (DE024716 to GH, DE028561 and DE026152 to GH and TC), the ERC (DEMETINL to TC), and the Deutsche Forschungsgemeinschaft (SFB1181 to TC).
The authors declare that the research was conducted in the absence of any commercial or financial relationships that could be construed as a potential conflict of interest.
1. Rosales, C, and Uribe-Querol, E. Phagocytosis: a fundamental process in immunity. Biomed Res Int. (2017) 2017:9042851. doi: 10.1155/2017/9042851
2. Stuart, LM, and Ezekowitz, RA. Phagocytosis: elegant complexity. Immunity. (2005) 22:539–50. doi: 10.1016/j.immuni.2005.05.002
3. Ortega-Gomez, A, Perretti, M, and Soehnlein, O. Resolution of inflammation: an integrated view. EMBO Mol Med. (2013) 5:661–74. doi: 10.1002/emmm.201202382
4. Hajishengallis, G, Chavakis, T, Hajishengallis, E, and Lambris, JD. Neutrophil homeostasis and inflammation: novel paradigms from studying periodontitis. J Leukoc Biol. (2015) 98:539–48. doi: 10.1189/jlb.3VMR1014-468R
5. Soehnlein, O, Steffens, S, Hidalgo, A, and Weber, C. Neutrophils as protagonists and targets in chronic inflammation. Nat Rev Immunol. (2017) 17:248–61. doi: 10.1038/nri.2017.10
6. Ley, K, Hoffman, HM, Kubes, P, Cassatella, MA, Zychlinsky, A, Hedrick, CC, et al. Neutrophils: new insights and open questions. Sci Immunol. (2018) 3:eaat4579. doi: 10.1126/sciimmunol.aat4579
7. Doran, AC, Yurdagul, A Jr., and Tabas, I. Efferocytosis in health and disease. Nat Rev Immunol. (2019). doi: 10.1038/s41577-019-0240-6 [Epub ahead of print].
8. Kourtzelis, I, Mitroulis, I, von Renesse, J, Hajishengallis, G, and Chavakis, T. From leukocyte recruitment to resolution of inflammation: the cardinal role of integrins. J Leukoc Biol. (2017) 102:677–83. doi: 10.1189/jlb.3MR0117-024R
9. Serhan, CN, and Levy, BD. Resolvins in inflammation: emergence of the pro-resolving superfamily of mediators. J Clin Invest. (2018) 128:2657–69. doi: 10.1172/JCI97943
10. Headland, SE, and Norling, LV. The resolution of inflammation: principles and challenges. Semin Immunol. (2015) 27:149–60. doi: 10.1016/j.smim.2015.03.014
11. Ravichandran, KS. Find-me and eat-me signals in apoptotic cell clearance: progress and conundrums. J Exp Med. (2010) 207:1807–17. doi: 10.1084/jem.20101157
12. Serhan, CN, Chiang, N, Dalli, J, and Levy, BD. Lipid mediators in the resolution of inflammation. Cold Spring Harb Perspect Biol. (2014) 7:a016311. doi: 10.1101/cshperspect.a016311
13. Fond, AM, and Ravichandran, KS. Clearance of dying cells by phagocytes: mechanisms and implications for disease pathogenesis. Adv Exp Med Biol. (2016) 930:25–49. doi: 10.1007/978-3-319-39406-0_2
14. Thorp, E, and Tabas, I. Mechanisms and consequences of efferocytosis in advanced atherosclerosis. J Leukoc Biol. (2009) 86:1089–95. doi: 10.1189/jlb.0209115
15. Kawano, M, and Nagata, S. Efferocytosis and autoimmune disease. Int Immunol. (2018) 30:551–8. doi: 10.1093/intimm/dxy055
16. O’Neill, LA, and Pearce, EJ. Immunometabolism governs dendritic cell and macrophage function. J Exp Med. (2016) 213:15–23. doi: 10.1084/jem.20151570
17. Stunault, MI, Bories, G, Guinamard, RR, and Ivanov, S. Metabolism plays a key role during macrophage activation. Mediators Inflamm. (2018) 2018:2426138. doi: 10.1155/2018/2426138
18. Zhang, S, Weinberg, S, DeBerge, M, Gainullina, A, Schipma, M, Kinchen, JM, et al. Efferocytosis fuels requirements of fatty acid oxidation and the electron transport chain to polarize macrophages for tissue repair. Cell Metab. (2019) 29:443–56.e5. doi: 10.1016/j.cmet.2018.12.004
19. Park, D, Han, CZ, Elliott, MR, Kinchen, JM, Trampont, PC, Das, S, et al. Continued clearance of apoptotic cells critically depends on the phagocyte Ucp2 protein. Nature. (2011) 477:220–4. doi: 10.1038/nature10340
20. Park, SY, and Kim, IS. Engulfment signals and the phagocytic machinery for apoptotic cell clearance. Exp Mol Med. (2017) 49:e331. doi: 10.1038/emm.2017.52
21. Medina, CB, and Ravichandran, KS. Do not let death do us part: ‘find-me’ signals in communication between dying cells and the phagocytes. Cell Death Differ. (2016) 23:979–89. doi: 10.1038/cdd.2016.13
22. Elliott, MR, Chekeni, FB, Trampont, PC, Lazarowski, ER, Kadl, A, Walk, SF, et al. Nucleotides released by apoptotic cells act as a find-me signal to promote phagocytic clearance. Nature. (2009) 461:282–6. doi: 10.1038/nature08296
23. Lauber, K, Bohn, E, Krober, SM, Xiao, YJ, Blumenthal, SG, Lindemann, RK, et al. Apoptotic cells induce migration of phagocytes via caspase-3-mediated release of a lipid attraction signal. Cell. (2003) 113:717–30. doi: 10.1016/s0092-8674(03)00422-7
24. Gude, DR, Alvarez, SE, Paugh, SW, Mitra, P, Yu, J, Griffiths, R, et al. Apoptosis induces expression of sphingosine kinase 1 to release sphingosine-1-phosphate as a “come-and-get-me” signal. FASEB J. (2008) 22:2629–38. doi: 10.1096/fj.08-107169
25. Moffatt, OD, Devitt, A, Bell, ED, Simmons, DL, and Gregory, CD. Macrophage recognition of ICAM-3 on apoptotic leukocytes. J Immunol. (1999) 162:6800–10.
26. Savill, J, Hogg, N, Ren, Y, and Haslett, C. Thrombospondin cooperates with CD36 and the vitronectin receptor in macrophage recognition of neutrophils undergoing apoptosis. J Clin Invest. (1992) 90:1513–22. doi: 10.1172/JCI116019
27. Elliott, MR, and Ravichandran, KS. The dynamics of apoptotic cell clearance. Dev Cell. (2016) 38:147–60. doi: 10.1016/j.devcel.2016.06.029
28. Poon, IK, Lucas, CD, Rossi, AG, and Ravichandran, KS. Apoptotic cell clearance: basic biology and therapeutic potential. Nat Rev Immunol. (2014) 14:166–80. doi: 10.1038/nri3607
29. Gardai, SJ, McPhillips, KA, Frasch, SC, Janssen, WJ, Starefeldt, A, Murphy-Ullrich, JE, et al. Cell-surface calreticulin initiates clearance of viable or apoptotic cells through trans-activation of LRP on the phagocyte. Cell. (2005) 123:321–34. doi: 10.1016/j.cell.2005.08.032
30. Jaillon, S, Jeannin, P, Hamon, Y, Fremaux, I, Doni, A, Bottazzi, B, et al. Endogenous PTX3 translocates at the membrane of late apoptotic human neutrophils and is involved in their engulfment by macrophages. Cell Death Differ. (2009) 16:465–74. doi: 10.1038/cdd.2008.173
31. Hanayama, R, Tanaka, M, Miyasaka, K, Aozasa, K, Koike, M, Uchiyama, Y, et al. Autoimmune disease and impaired uptake of apoptotic cells in MFG-E8-deficient mice. Science. (2004) 304:1147–50. doi: 10.1126/science.1094359
32. Hanayama, R, Tanaka, M, Miwa, K, Shinohara, A, Iwamatsu, A, and Nagata, S. Identification of a factor that links apoptotic cells to phagocytes. Nature. (2002) 417:182–7. doi: 10.1038/417182a
33. Hajishengallis, G, and Chavakis, T. DEL-1-regulated immune plasticity and inflammatory disorders. Trends Mol Med. (2019) 25:444–59. doi: 10.1016/j.molmed.2019.02.010
34. Choi, EY, Chavakis, E, Czabanka, MA, Langer, HF, Fraemohs, L, Economopoulou, M, et al. Del-1, an endogenous leukocyte-endothelial adhesion inhibitor, limits inflammatory cell recruitment. Science. (2008) 322:1101–4. doi: 10.1126/science.1165218
35. Kourtzelis, I, Kotlabova, K, Lim, JH, Mitroulis, I, Ferreira, A, Chen, LS, et al. Developmental endothelial locus-1 modulates platelet-monocyte interactions and instant blood-mediated inflammatory reaction in islet transplantation. Thromb Haemost. (2016) 115:781–8. doi: 10.1160/TH15-05-0429
36. Mitroulis, I, Alexaki, VI, Kourtzelis, I, Ziogas, A, Hajishengallis, G, and Chavakis, T. Leukocyte integrins: role in leukocyte recruitment and as therapeutic targets in inflammatory disease. Pharmacol Ther. (2015) 147:123–35. doi: 10.1016/j.pharmthera.2014.11.008
37. Hajishengallis, G, and Chavakis, T. Endogenous modulators of inflammatory cell recruitment. Trends Immunol. (2013) 34:1–6. doi: 10.1016/j.it.2012.08.003
38. Shin, J, Maekawa, T, Abe, T, Hajishengallis, E, Hosur, K, Pyaram, K, et al. DEL-1 restrains osteoclastogenesis and inhibits inflammatory bone loss in nonhuman primates. Sci Transl Med. (2015) 7:307ra155. doi: 10.1126/scitranslmed.aac5380
39. Chavakis, T, Mitroulis, I, and Hajishengallis, G. Hematopoietic progenitor cells as integrative hubs for adaptation to and fine-tuning of inflammation. Nat Immunol. (2019) 20:802–11. doi: 10.1038/s41590-019-0402-5
40. Mitroulis, I, Chen, LS, Singh, RP, Kourtzelis, I, Economopoulou, M, Kajikawa, T, et al. Secreted protein Del-1 regulates myelopoiesis in the hematopoietic stem cell niche. J Clin Invest. (2017) 127:3624–39. doi: 10.1172/JCI92571
41. Kourtzelis, I, Li, X, Mitroulis, I, Grosser, D, Kajikawa, T, Wang, B, et al. DEL-1 promotes macrophage efferocytosis and clearance of inflammation. Nat Immunol. (2019) 20:40–9. doi: 10.1038/s41590-018-0249-1
42. Savill, J, Dransfield, I, Hogg, N, and Haslett, C. Vitronectin receptor-mediated phagocytosis of cells undergoing apoptosis. Nature. (1990) 343:170–3. doi: 10.1038/343170a0
43. Hanayama, R, Tanaka, M, Miwa, K, and Nagata, S. Expression of developmental endothelial locus-1 in a subset of macrophages for engulfment of apoptotic cells. J Immunol. (2004) 172:3876–82. doi: 10.4049/jimmunol.172.6.3876
44. Arur, S, Uche, UE, Rezaul, K, Fong, M, Scranton, V, Cowan, AE, et al. Annexin I is an endogenous ligand that mediates apoptotic cell engulfment. Dev Cell. (2003) 4:587–98. doi: 10.1016/s1534-5807(03)00090-x
45. Balasubramanian, K, and Schroit, AJ. Characterization of phosphatidylserine-dependent beta2-glycoprotein I macrophage interactions. Implications for apoptotic cell clearance by phagocytes. J Biol Chem. (1998) 273:29272–7. doi: 10.1074/jbc.273.44.29272
46. Caberoy, NB, Alvarado, G, Bigcas, JL, and Li, W. Galectin-3 is a new MerTK-specific eat-me signal. J Cell Physiol. (2012) 227:401–7. doi: 10.1002/jcp.22955
47. Anderson, HA, Maylock, CA, Williams, JA, Paweletz, CP, Shu, H, and Shacter, E. Serum-derived protein S binds to phosphatidylserine and stimulates the phagocytosis of apoptotic cells. Nat Immunol. (2003) 4:87–91. doi: 10.1038/ni871
48. Lemke, G, and Burstyn-Cohen, T. TAM receptors and the clearance of apoptotic cells. Ann N Y Acad Sci. (2010) 1209:23–9. doi: 10.1111/j.1749-6632.2010.05744.x
49. Scott, RS, McMahon, EJ, Pop, SM, Reap, EA, Caricchio, R, Cohen, PL, et al. Phagocytosis and clearance of apoptotic cells is mediated by MER. Nature. (2001) 411:207–11. doi: 10.1038/35075603
50. Rothlin, CV, Ghosh, S, Zuniga, EI, Oldstone, MB, and Lemke, G. TAM receptors are pleiotropic inhibitors of the innate immune response. Cell. (2007) 131:1124–36. doi: 10.1016/j.cell.2007.10.034
51. Lemke, G, and Rothlin, CV. Immunobiology of the TAM receptors. Nat Rev Immunol. (2008) 8:327–36. doi: 10.1038/nri2303
52. Lumbroso, D, Soboh, S, Maimon, A, Schif-Zuck, S, Ariel, A, and Burstyn-Cohen, T. Macrophage-derived protein S facilitates apoptotic polymorphonuclear cell clearance by resolution phase macrophages and supports their reprogramming. Front Immunol. (2018) 9:358. doi: 10.3389/fimmu.2018.00358
53. Miyanishi, M, Tada, K, Koike, M, Uchiyama, Y, Kitamura, T, and Nagata, S. Identification of Tim4 as a phosphatidylserine receptor. Nature. (2007) 450:435–9. doi: 10.1038/nature06307
54. Kobayashi, N, Karisola, P, Pena-Cruz, V, Dorfman, DM, Jinushi, M, Umetsu, SE, et al. TIM-1 and TIM-4 glycoproteins bind phosphatidylserine and mediate uptake of apoptotic cells. Immunity. (2007) 27:927–40. doi: 10.1016/j.immuni.2007.11.011
55. Park, D, Tosello-Trampont, AC, Elliott, MR, Lu, M, Haney, LB, Ma, Z, et al. BAI1 is an engulfment receptor for apoptotic cells upstream of the ELMO/Dock180/Rac module. Nature. (2007) 450:430–4. doi: 10.1038/nature06329
56. Park, SY, Jung, MY, Kim, HJ, Lee, SJ, Kim, SY, Lee, BH, et al. Rapid cell corpse clearance by stabilin-2, a membrane phosphatidylserine receptor. Cell Death Differ. (2008) 15:192–201. doi: 10.1038/sj.cdd.4402242
57. Mevorach, D, Mascarenhas, JO, Gershov, D, and Elkon, KB. Complement-dependent clearance of apoptotic cells by human macrophages. J Exp Med. (1998) 188:2313–20. doi: 10.1084/jem.188.12.2313
58. Devitt, A, Moffatt, OD, Raykundalia, C, Capra, JD, Simmons, DL, and Gregory, CD. Human CD14 mediates recognition and phagocytosis of apoptotic cells. Nature. (1998) 392:505–19. doi: 10.1038/33169
59. Oldenborg, PA, Zheleznyak, A, Fang, YF, Lagenaur, CF, Gresham, HD, and Lindberg, FP. Role of CD47 as a marker of self on red blood cells. Science. (2000) 288:2051–4. doi: 10.1126/science.288.5473.2051
60. Oldenborg, PA, Gresham, HD, and Lindberg, FP. CD47-signal regulatory protein alpha (SIRPalpha) regulates Fcgamma and complement receptor-mediated phagocytosis. J Exp Med. (2001) 193:855–62. doi: 10.1084/jem.193.7.855
61. Kojima, Y, Volkmer, JP, McKenna, K, Civelek, M, Lusis, AJ, Miller, CL, et al. CD47-blocking antibodies restore phagocytosis and prevent atherosclerosis. Nature. (2016) 536:86–90. doi: 10.1038/nature18935
62. Brown, S, Heinisch, I, Ross, E, Shaw, K, Buckley, CD, and Savill, J. Apoptosis disables CD31-mediated cell detachment from phagocytes promoting binding and engulfment. Nature. (2002) 418:200–3. doi: 10.1038/nature00811
63. Barkal, AA, Brewer, RE, Markovic, M, Kowarsky, M, Barkal, SA, Zaro, BW, et al. CD24 signalling through macrophage Siglec-10 is a target for cancer immunotherapy. Nature. (2019) 572:392–6. doi: 10.1038/s41586-019-1456-0
64. Elward, K, Griffiths, M, Mizuno, M, Harris, CL, Neal, JW, Morgan, BP, et al. CD46 plays a key role in tailoring innate immune recognition of apoptotic and necrotic cells. J Biol Chem. (2005) 280:36342–54. doi: 10.1074/jbc.M506579200
65. Barth, ND, Marwick, JA, Vendrell, M, Rossi, AG, and Dransfield, I. The “Phagocytic Synapse” and clearance of apoptotic cells. Front Immunol. (2017) 8:1708. doi: 10.3389/fimmu.2017.01708
66. Kinchen, JM, and Ravichandran, KS. Phagocytic signaling: you can touch, but you can’t eat. Curr Biol. (2008) 18:R521–4. doi: 10.1016/j.cub.2008.04.058
67. Ariel, A, and Serhan, CN. New lives given by cell death: macrophage differentiation following their encounter with apoptotic leukocytes during the resolution of inflammation. Front Immunol. (2012) 3:4. doi: 10.3389/fimmu.2012.00004
68. Bratton, DL, and Henson, PM. Neutrophil clearance: when the party is over, clean-up begins. Trends Immunol. (2011) 32:350–7. doi: 10.1016/j.it.2011.04.009
69. Henson, PM. Cell removal: efferocytosis. Annu Rev Cell Dev Biol. (2017) 33:127–44. doi: 10.1146/annurev-cellbio-111315-125315
70. Henson, PM, and Bratton, DL. Antiinflammatory effects of apoptotic cells. J Clin Invest. (2013) 123:2773–4. doi: 10.1172/JCI69344
71. Sugimoto, MA, Vago, JP, Perretti, M, and Teixeira, MM. Mediators of the resolution of the inflammatory response. Trends Immunol. (2019) 40:212–27. doi: 10.1016/j.it.2019.01.007
72. Fadok, VA, Bratton, DL, Konowal, A, Freed, PW, Westcott, JY, and Henson, PM. Macrophages that have ingested apoptotic cells in vitro inhibit proinflammatory cytokine production through autocrine/paracrine mechanisms involving TGF-beta, PGE2, and PAF. J Clin Invest. (1998) 101:890–8. doi: 10.1172/JCI1112
73. Huynh, ML, Fadok, VA, and Henson, PM. Phosphatidylserine-dependent ingestion of apoptotic cells promotes TGF-beta1 secretion and the resolution of inflammation. J Clin Invest. (2002) 109:41–50. doi: 10.1172/JCI11638
74. Proto, JD, Doran, AC, Gusarova, G, Yurdagul, A Jr., Sozen, E, Subramanian, M, et al. Regulatory T cells promote macrophage efferocytosis during inflammation resolution. Immunity. (2018) 49:666–77.e6. doi: 10.1016/j.immuni.2018.07.015
75. Schauer, C, Janko, C, Munoz, LE, Zhao, Y, Kienhofer, D, Frey, B, et al. Aggregated neutrophil extracellular traps limit inflammation by degrading cytokines and chemokines. Nat Med. (2014) 20:511–7. doi: 10.1038/nm.3547
76. Levy, BD, Clish, CB, Schmidt, B, Gronert, K, and Serhan, CN. Lipid mediator class switching during acute inflammation: signals in resolution. Nat Immunol. (2001) 2:612–9. doi: 10.1038/89759
77. Uderhardt, S, Herrmann, M, Oskolkova, OV, Aschermann, S, Bicker, W, Ipseiz, N, et al. 12/15-lipoxygenase orchestrates the clearance of apoptotic cells and maintains immunologic tolerance. Immunity. (2012) 36:834–46. doi: 10.1016/j.immuni.2012.03.010
78. Stables, MJ, Shah, S, Camon, EB, Lovering, RC, Newson, J, Bystrom, J, et al. Transcriptomic analyses of murine resolution-phase macrophages. Blood. (2011) 118:e192–208. doi: 10.1182/blood-2011-04-345330
79. Kumaran Satyanarayanan, S, El Kebir, D, Soboh, S, Butenko, S, Sekheri, M, Saadi, J, et al. IFN-beta is a macrophage-derived effector cytokine facilitating the resolution of bacterial inflammation. Nat Commun. (2019) 10:3471. doi: 10.1038/s41467-019-10903-9
80. Schif-Zuck, S, Gross, N, Assi, S, Rostoker, R, Serhan, CN, and Ariel, A. Saturated-efferocytosis generates pro-resolving CD11b low macrophages: modulation by resolvins and glucocorticoids. Eur J Immunol. (2011) 41:366–79. doi: 10.1002/eji.201040801
81. Freire-de-Lima, CG, Xiao, YQ, Gardai, SJ, Bratton, DL, Schiemann, WP, and Henson, PM. Apoptotic cells, through transforming growth factor-beta, coordinately induce anti-inflammatory and suppress pro-inflammatory eicosanoid and NO synthesis in murine macrophages. J Biol Chem. (2006) 281:38376–84. doi: 10.1074/jbc.M605146200
82. Sugimoto, MA, Ribeiro, ALC, Costa, BRC, Vago, JP, Lima, KM, Carneiro, FS, et al. Plasmin and plasminogen induce macrophage reprogramming and regulate key steps of inflammation resolution via annexin A1. Blood. (2017) 129:2896–907. doi: 10.1182/blood-2016-09-742825
83. Vago, JP, Sugimoto, MA, Lima, KM, Negreiros-Lima, GL, Baik, N, Teixeira, MM, et al. Plasminogen and the plasminogen receptor, Plg-RKT, regulate macrophage phenotypic, and functional changes. Front Immunol. (2019) 10:1458. doi: 10.3389/fimmu.2019.01458
84. Sousa, LP, Alessandri, AL, Pinho, V, and Teixeira, MM. Pharmacological strategies to resolve acute inflammation. Curr Opin Pharmacol. (2013) 13:625–31. doi: 10.1016/j.coph.2013.03.007
85. Sugimoto, MA, Vago, JP, Teixeira, MM, and Sousa, LP. Annexin A1 and the resolution of inflammation: modulation of neutrophil recruitment, apoptosis, and clearance. J Immunol Res. (2016) 2016:8239258. doi: 10.1155/2016/8239258
86. Galvao, I, Vago, JP, Barroso, LC, Tavares, LP, Queiroz-Junior, CM, Costa, VV, et al. Annexin A1 promotes timely resolution of inflammation in murine gout. Eur J Immunol. (2017) 47:585–96. doi: 10.1002/eji.201646551
87. Souza, DG, Fagundes, CT, Amaral, FA, Cisalpino, D, Sousa, LP, Vieira, AT, et al. The required role of endogenously produced lipoxin A4 and annexin-1 for the production of IL-10 and inflammatory hyporesponsiveness in mice. J Immunol. (2007) 179:8533–43. doi: 10.4049/jimmunol.179.12.8533
88. Vago, JP, Nogueira, CR, Tavares, LP, Soriani, FM, Lopes, F, Russo, RC, et al. Annexin A1 modulates natural and glucocorticoid-induced resolution of inflammation by enhancing neutrophil apoptosis. J Leukoc Biol. (2012) 92:249–58. doi: 10.1189/jlb.0112008
89. O’Neill, LA, Kishton, RJ, and Rathmell, J. A guide to immunometabolism for immunologists. Nat Rev Immunol. (2016) 16:553–65. doi: 10.1038/nri.2016.70
90. Pearce, EL, and Pearce, EJ. Metabolic pathways in immune cell activation and quiescence. Immunity. (2013) 38:633–43. doi: 10.1016/j.immuni.2013.04.005
91. Van den Bossche, J, O’Neill, LA, and Menon, D. Macrophage immunometabolism: where are we (going)? Trends Immunol. (2017) 38:395–406. doi: 10.1016/j.it.2017.03.001
92. Diskin, C, and Palsson-McDermott, EM. Metabolic modulation in macrophage effector function. Front Immunol. (2018) 9:270. doi: 10.3389/fimmu.2018.00270
93. Rosas, M, Davies, LC, Giles, PJ, Liao, CT, Kharfan, B, Stone, TC, et al. The transcription factor Gata6 links tissue macrophage phenotype and proliferative renewal. Science. (2014) 344:645–8. doi: 10.1126/science.1251414
94. Okabe, Y, and Medzhitov, R. Tissue-specific signals control reversible program of localization and functional polarization of macrophages. Cell. (2014) 157:832–44. doi: 10.1016/j.cell.2014.04.016
95. A-Gonzalez, N, Guillen, JA, Gallardo, G, Diaz, M, de la Rosa, JV, Hernandez, IH, et al. The nuclear receptor LXRalpha controls the functional specialization of splenic macrophages. Nat Immunol. (2013) 14:831–9. doi: 10.1038/ni.2622
96. Davies, LC, and Taylor, PR. Tissue-resident macrophages: then and now. Immunology. (2015) 144:541–8. doi: 10.1111/imm.12451
97. Gautier, EL, Shay, T, Miller, J, Greter, M, Jakubzick, C, Ivanov, S, et al. Gene-expression profiles and transcriptional regulatory pathways that underlie the identity and diversity of mouse tissue macrophages. Nat Immunol. (2012) 13:1118–28. doi: 10.1038/ni.2419
98. A-Gonzalez, N, Quintana, JA, Garcia-Silva, S, Mazariegos, M, Gonzalez de la Aleja, A, Nicolas-Avila, JA, et al. Phagocytosis imprints heterogeneity in tissue-resident macrophages. J Exp Med. (2017) 214:1281–96. doi: 10.1084/jem.20161375
99. Han, CZ, and Ravichandran, KS. Metabolic connections during apoptotic cell engulfment. Cell. (2011) 147:1442–5. doi: 10.1016/j.cell.2011.12.006
100. Hochreiter-Hufford, A, and Ravichandran, KS. Clearing the dead: apoptotic cell sensing, recognition, engulfment, and digestion. Cold Spring Harb Perspect Biol. (2013) 5:a008748. doi: 10.1101/cshperspect.a008748
101. Green, DR, Oguin, TH, and Martinez, J. The clearance of dying cells: table for two. Cell Death Differ. (2016) 23:915–26. doi: 10.1038/cdd.2015.172
102. Morioka, S, Perry, JSA, Raymond, MH, Medina, CB, Zhu, Y, Zhao, L, et al. Efferocytosis induces a novel SLC program to promote glucose uptake and lactate release. Nature. (2018) 563:714–8. doi: 10.1038/s41586-018-0735-5
103. Freemerman, AJ, Zhao, L, Pingili, AK, Teng, B, Cozzo, AJ, Fuller, AM, et al. Myeloid Slc2a1-deficient murine model revealed macrophage activation and metabolic phenotype are fueled by GLUT1. J Immunol. (2019) 202:1265–86. doi: 10.4049/jimmunol.1800002
104. Jiang, H, Shi, H, Sun, M, Wang, Y, Meng, Q, Guo, P, et al. PFKFB3-driven macrophage glycolytic metabolism is a crucial component of innate antiviral defense. J Immunol. (2016) 197:2880–90. doi: 10.4049/jimmunol.1600474
105. Yurdagul, A Jr., Subramanian, M, Wang, X, Crown, SB, Ilkayeva, OR, Darville, L, et al. Macrophage metabolism of apoptotic cell-derived arginine promotes continual efferocytosis and resolution of injury. Cell Metab. (2020) 31:518–33.e10. doi: 10.1016/j.cmet.2020.01.001
106. Wang, Y, Subramanian, M, Yurdagul, A Jr., Barbosa-Lorenzi, VC, Cai, B, de Juan-Sanz, J, et al. Mitochondrial fission promotes the continued clearance of apoptotic cells by macrophages. Cell. (2017) 171:331–45.e22. doi: 10.1016/j.cell.2017.08.041
107. A-Gonzalez, N, and Hidalgo, A. Nuclear receptors and clearance of apoptotic cells: stimulating the macrophage’s appetite. Front Immunol. (2014) 5:211. doi: 10.3389/fimmu.2014.00211
108. A-Gonzalez, N, Bensinger, SJ, Hong, C, Beceiro, S, Bradley, MN, Zelcer, N, et al. Apoptotic cells promote their own clearance and immune tolerance through activation of the nuclear receptor LXR. Immunity. (2009) 31:245–58. doi: 10.1016/j.immuni.2009.06.018
109. Mukundan, L, Odegaard, JI, Morel, CR, Heredia, JE, Mwangi, JW, Ricardo-Gonzalez, RR, et al. PPAR-delta senses and orchestrates clearance of apoptotic cells to promote tolerance. Nat Med. (2009) 15:1266–72. doi: 10.1038/nm.2048
110. Rebe, C, Raveneau, M, Chevriaux, A, Lakomy, D, Sberna, AL, Costa, A, et al. Induction of transglutaminase 2 by a liver X receptor/retinoic acid receptor alpha pathway increases the clearance of apoptotic cells by human macrophages. Circ Res. (2009) 105:393–401. doi: 10.1161/CIRCRESAHA.109.201855
111. Toth, B, Garabuczi, E, Sarang, Z, Vereb, G, Vamosi, G, Aeschlimann, D, et al. Transglutaminase 2 is needed for the formation of an efficient phagocyte portal in macrophages engulfing apoptotic cells. J Immunol. (2009) 182:2084–92. doi: 10.4049/jimmunol.0803444
112. Morimoto, K, Janssen, WJ, Fessler, MB, McPhillips, KA, Borges, VM, Bowler, RP, et al. Lovastatin enhances clearance of apoptotic cells (efferocytosis) with implications for chronic obstructive pulmonary disease. J Immunol. (2006) 176:7657–65. doi: 10.4049/jimmunol.176.12.7657
113. Lee, YJ, Kim, MJ, Yoon, YS, Choi, YH, Kim, HS, and Kang, JL. Simvastatin treatment boosts benefits of apoptotic cell infusion in murine lung fibrosis. Cell Death Dis. (2017) 8:e2860. doi: 10.1038/cddis.2017.260
114. Venkateswaran, A, Laffitte, BA, Joseph, SB, Mak, PA, Wilpitz, DC, Edwards, PA, et al. Control of cellular cholesterol efflux by the nuclear oxysterol receptor LXR alpha. Proc Natl Acad Sci USA. (2000) 97:12097–102. doi: 10.1073/pnas.200367697
Keywords: phagocytosis, efferocytosis, DEL-1, immunometabolism, inflammation resolution, integrins
Citation: Kourtzelis I, Hajishengallis G and Chavakis T (2020) Phagocytosis of Apoptotic Cells in Resolution of Inflammation. Front. Immunol. 11:553. doi: 10.3389/fimmu.2020.00553
Received: 12 January 2020; Accepted: 11 March 2020;
Published: 31 March 2020.
Edited by:
Esther M. Lafuente, Complutense University of Madrid, SpainReviewed by:
Amiram Ariel, University of Haifa, IsraelCopyright © 2020 Kourtzelis, Hajishengallis and Chavakis. This is an open-access article distributed under the terms of the Creative Commons Attribution License (CC BY). The use, distribution or reproduction in other forums is permitted, provided the original author(s) and the copyright owner(s) are credited and that the original publication in this journal is cited, in accordance with accepted academic practice. No use, distribution or reproduction is permitted which does not comply with these terms.
*Correspondence: Ioannis Kourtzelis, aW9hbm5pcy5rb3VydHplbGlzQHlvcmsuYWMudWs=
Disclaimer: All claims expressed in this article are solely those of the authors and do not necessarily represent those of their affiliated organizations, or those of the publisher, the editors and the reviewers. Any product that may be evaluated in this article or claim that may be made by its manufacturer is not guaranteed or endorsed by the publisher.
Research integrity at Frontiers
Learn more about the work of our research integrity team to safeguard the quality of each article we publish.