- 1DKMS, Tübingen, Germany
- 2DKMS Life Science Lab, Dresden, Germany
- 3University Hospital Carl Gustav Carus, Dresden, Germany
The impact of the highly polymorphic Killer-cell immunoglobulin-like receptor (KIR) gene cluster on the outcome of hematopoietic stem cell transplantation (HCST) is subject of current research. To further understand the involvement of this gene family into Natural Killer (NK) cell-mediated graft-versus-leukemia reactions, knowledge of haplotype structures, and allelic linkage is of importance. In this analysis, we estimate population-specific KIR haplotype frequencies at allele group resolution in a cohort of n = 458 German families. We addressed the polymorphism of the KIR gene complex and phasing ambiguities by a combined approach. Haplotype inference within first-degree family relations allowed us to limit the number of possible diplotypes. Structural restriction to a pattern set of 92 previously described KIR copy number haplotypes further reduced ambiguities. KIR haplotype frequency estimation was finally accomplished by means of an expectation-maximization algorithm. Applying a resolution threshold of ½ n, we were able to identify a set of 551 KIR allele group haplotypes, representing 21 KIR copy number haplotypes. The haplotype frequencies allow studying linkage disequilibrium in two-locus as well as in multi-locus analyses. Our study reveals associations between KIR haplotype structures and allele group frequencies, thereby broadening our understanding of the KIR gene complex.
Introduction
The Killer-cell immunoglobulin-like receptor (KIR) gene cluster is located on the long arm of human chromosome 19 (19q13.4). The family includes the 15 expressed genes KIR2DL1-4, KIR2DL5A, KIR2DL5B, KIR3DL1-3, KIR2DS1-5, and KIR3DS1 and the two pseudogenes KIR2DP1 and KIR3DP1 (1). KIR genes are characterized by a high level of sequence homology among different gene loci on one hand, and multi-layer complexity at the gene and haplotype level on the other hand (2, 3). The KIR gene copy number (CN) of a locus varies from 0 to 3 per haplotype (4). Each of the KIR loci displays high allelic polymorphism. One thousand one hundred ten allele variants and 543 KIR proteins have been named so far [Immuno Polymorphism Database IPD-KIR v2.9.0, December 2019 (5)]. In addition, diversity of the KIR gene region was found to be shaped in a population-specific manner through evolutionary mechanisms (6). Frequent occurrence of hybrid KIR genes (7–14), as well as events of alternative splicing of KIR genes, resulting in potentially functional receptor isoforms (15), have been described.
The high genetic complexity may be rooted in the diverse functions of KIR gene products in the human innate immune system. KIR proteins are transmembrane receptors primarily expressed by Natural Killer (NK) cells. Most KIR are located in the NK cell plasma or endosomal membrane and are known to be involved in the regulation of the immune response to viral infections and cancer or in the governance of histocompatibility during pregnancy (16–18). Bound to their corresponding ligands, which include classical and non-classical human leukocyte antigen (HLA) class I molecules, they convey activating or inhibitory signals to the NK cell (18, 19). KIR3DL1-3, KIR2DL1-3, and KIR2DL5A/B are the KIR receptors with inhibitory potential, the activating receptors are KIR3DS1 and KIR2DS1-5. The integrated signals of KIR and further NK cell receptors regulate NK cell activity between the two extremes of tolerance and killing (16). KIR2DL4 plays a special role in the KIR gene family. This receptor appears to participate in the NK-mediated control of the maternal/fetal interface during pregnancy and is probably not involved in the cancer or infection surveillance (20, 21). However, lack of the KIR2DL4 in maternal NK cells is still compatible with successful pregnancy (22).
In present-day unrelated hematopoietic stem cell transplantation (HSCT) practice, the alleles of particular HLA genes are matched between patient and stem cell donor to avoid undesired immune reactions of donor T-cells against the host organism (23–25). However, as graft-versus-host disease (GvHD) and disease relapse remain serious complications for many patients, there is an ongoing quest to identify further immunogenic factors with the potential to improve the outcome of HSCT. Since NK cells are the first lymphocytes to reconstitute in patients after HSCT (26), efforts have been made to exploit their potential to elicit a rapid and targeted immune response against remaining leukemic cells (27–32). Recent research indicates an impact of donor KIR genotype on the outcome of HSCT, but results remain controversial (33–37).
The knowledge of population-specific allelic haplotype frequencies for HLA genes of the major histocompatibility complex (MHC) offered advantages in the field of unrelated donor HSCT. For instance, all major donor search algorithms utilize HLA haplotype frequencies to estimate the probabilities for listed donors with ambiguous or incomplete HLA typing data to be a match for a specific patient (38, 39). Matching probability analyses using population-specific HLA haplotype frequencies allow targeted planning of donor center recruitment strategies (40). Beyond that, a positive impact on outcome of HSCT is proposed for the additional matching of non-HLA genes located in the MHC region via haplotype matching between patient and donor, but study results are still inconclusive (41–43).
Efficient use of KIR genes in donor selection for successful HSCT accordingly would benefit from knowledge of population-specific KIR haplotype frequencies. However, compared to HLA, KIR haplotype inference from separately typed loci is much more difficult because of the high number of possible diplotypes one unphased KIR genotype is compatible with. For example, two alleles genotyped at one KIR locus may be located either both on the same chromosome or each alone on one of the two chromosomes. These ambiguities and the high number of KIR loci lead to a large amount of possible allelic diplotypes that requires unrealistically large memory for the implementation of a conventional expectation-maximization (EM) algorithm.
A way to cope with the high number of possible allelic diplotypes per individual and to still reproduce the polymorphic nature of KIR haplotypes is to limit the underlying haplotype structure to pre-established patterns (44, 45). Previous research analyzed the haplotype structure of the KIR gene complex at various levels of resolution. Presence or absence of certain KIR loci in individuals was found not to occur at random, but to follow structural patterns. At the level of gene content (presence/absence, P/A) polymorphism, two major groups of KIR haplotypes (A and B) are described (2, 19). A and B haplotypes are characterized and distinguished by the presence of specific sets of KIR genes alongside the four “framework” genes (KIR3DL3, KIR3DP1, KIR2DL4, and KIR3DL2), flanking the centromeric and telomeric regions of the vast majority of all KIR haplotypes. KIR2DS4 is the only activating receptor encoded by A haplotypes, whereas B haplotypes carry up to 5 activating KIR. More detailed analyses of KIR haplotypes and their frequencies in different cohorts were undertaken at gene content level (45–48), copy number level (4, 14, 45, 49, 50) and at (partial) allelic resolution level (44, 48, 49, 51, 52). All studies confirmed the basic concept of A/B haplotypes, but also documented numerous deviations from these structural patterns, caused, e.g., by recombination, gene fusion, deletion, or insertion events.
In our study, we extended the method to limit haplotype structure to pre-established patterns in order to make it applicable to our data obtained by high-throughput KIR genotyping in the context of unrelated HSCT donor registration (53, 54). We applied a three-step approach to estimate population-specific KIR haplotype frequencies at allele group resolution. We analyzed the KIR genotypes in a cohort of n = 458 families in order to reduce phasing and typing ambiguities and thus the number of possible diplotypes for the n = 916 parents. Structural complexity was further confined by restricting possible haplotypes to a set of 92 previously described KIR copy number haplotypes. Haplotype frequencies were then derived using an implementation of the EM algorithm that dealt with remaining ambiguities.
Materials and Methods
KIR and HLA Genotyping
Between October 2016 and April 2019, 2.6 million donors recruited by DKMS were KIR genotyped at allelic resolution by DKMS Life Science Lab in Dresden, Germany, using next generation sequencing methods (53, 55, 56). Genotyping comprises the HLA loci HLA-A, -B, -C, -DRB1, -DQB1, and -DPB1, the KIR loci KIR2DL1-5, KIR3DL1-3, KIR2DS1-5, KIR3DS1, KIR2DP1, and KIR3DP1, as well as ABO (57), RhD, CCR5 (58), and MIC-A/B (59). The KIR genotyping approach delivers both allele group information and copy numbers for every KIR gene (53). DNA was extracted from blood samples or buccal swabs with the informed consent of the donors.
For the analysis of KIR haplotypes, KIR genotyping results were shortened to the first three digits of the allele name, thereby merging alleles with synonymous substitutions within the coding region and non-coding mutations. Allelic ambiguities due to variations outside the typed exons (exons 3, 4, 5, 7, 8, and 9) were grouped and denoted by a trailing “c” (Supplementary Information S1). In the following, 3-digit allelic level and “c”-groups are referred to as “allele group” resolution. Since a clear distinction of genes of the loci KIR2DL5A and KIR2DL5B was not possible without sequence information on exon 1 and promoter region, we treated KIR2DL5A and KIR2DL5B as one locus. We considered 16 KIR loci in total.
Family Selection
Information on family relations is not recorded during DKMS donor recruitment. Data retrieval from the DKMS Germany donor file, demanding consistency of addresses and surnames and a minimal age difference of >20 years between parents and offspring, yielded a pseudonymized set of potential families of self-assessed German origin. The genetic relationship between potential family members was verified on the basis of the respective HLA-A, -B, -C, -DRB1, and -DQB1 typing data. A cohort of 458 HLA-confirmed families with two parents and at least one child were included into our study. Four hundred two of the families had one child, 55 had two, and one family had three children registered with the donor center (ntotal = 1,431).
KIR Data Refinement via Family Information
KIR genes of all members of our family cohort were resolved to allele group level and copy number. Knowledge of family relations was used to review KIR typing when comparison of data between parents and children revealed ambiguities or inconsistencies. For instance, in 1.6% of the 14,656 typed parental KIR loci (916 samples × 16 loci), the allele group typing results of a child apparently did not match the parental genotypes. All but 13 cases, concerning in total 10 sets of parents, could be solved by re-inspection of the sequencing data and application of the family information. The matching status of new and so far unnamed alleles between the family members (464 cases) was verified by comparison of sequencing data and was accordingly considered during haplotype inference. Families with more than one child (n = 56) permitted investigation of potential recombination events by intersecting parental haplotypes deduced via KIR genotyping data of different children.
Copy Number Haplotype Pattern Set (CNPS)
In order to reduce haplotype complexity originating from phasing ambiguities between KIR loci, only KIR genotypes that could be split into two haplotypes that met the structural pattern of 92 previously described KIR copy number haplotypes were permitted. The set of 92 KIR copy number haplotypes used as reference pattern is hereinafter referred to as CNPS. The CNPS included 12 copy number haplotypes described by Pyo et al. (48), 52 by Jiang et al. (4), 27 by Pyo et al. (50), and one by Roe et al. (14) (Supplementary Information S2). Because nomenclature of KIR haplotypes in the different publications in not consistent, we assigned a code name (column “HT code” in Supplementary Information S2) to every copy number haplotype in the CNPS which will be used below.
KIR loci KIR2DL5, KIR2DS3, and KIR2DS5 have experienced a duplication event in the past and can be found in both the centromeric and the telomeric section of particular haplotypes of the KIR gene complex (60, 61). We could not distinguish between the respective centromeric and telomeric variants of the three loci in our analysis. For copy number haplotypes from publications where KIR2DS3 and KIR2DS5 were treated as one single locus and where thus the assignment to one of the loci was ambiguous, we split the haplotype into all possible copy number forms. For example, a haplotype which specifies 2 copies of locus KIR2DS3S5 was split into three allowed copy number haplotypes: one haplotype with one copy of KIR2DS3 and KIR2DS5, each, and two haplotypes with two copies of one of the two genes and none of the other. Copy number haplotypes marked as containing hybrid or fusion gene loci by Pyo et al. (50) and Roe et al. (14) were disregarded.
Workflow
All software required for KIR haplotype frequency (HF) estimation in our approach was written in Perl 5. A schematic overview of the algorithm is shown in Figure 1. Families were analyzed in mother-father-child sets, i.e., a family with two children was analyzed in two separate family sets. Only diplotypes of the 916 parents were allowed to pass into the subsequent KIR HF estimation. Parents whose haplotypes had already counted for frequency estimation via a first family set were flagged to avoid duplication. For each of the mother-father-child constellations of our data set, all possible allele group diplotypes of mother and father were deduced. This was done by locus-wise diplotype inference and subsequent iteration of all possible allele constellations of all loci. The extensive diplotype list was then filtered with the CNPS to exclude all diplotypes that could not be explained by a pair of the allowed copy number haplotypes. In order to limit artifacts from lower-resolution typing data, we restricted the number of possible diplotypes per individual to a maximum of n = 1,000,000. Parents with more possible diplotypes after application of the CNPS were excluded from the subsequent HF estimation, unless they passed this requirement in a family constellation with another child. The most likely set of KIR HFs was finally derived from the parental diplotypes by means of an expectation-maximization (EM) algorithm (62). Haplotypes were initialized with equal frequencies prior to the start of the EM algorithm. The stop criterion, which defines the allowed maximal HF change between consecutive estimations, was set to 5*10−5. HFs were cut after a minimal frequency of f = 1/2n, with n being the number of individuals in the final haplotype estimation, corresponding to the occurrence of at least one haplotype in the sample. The resulting allele group haplotypes were attributed to the corresponding CNPS haplotypes in order to analyze the allelic diversity within the different copy number structures.
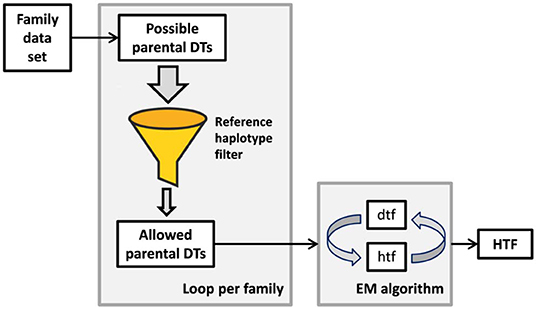
Figure 1. Simplified workflow of our haplotype frequency estimation approach. The number of 16-locus KIR diplotypes per individual is reduced by inference from a family context and subsequent filtering via a set of reference copy number haplotypes. Only diplotypes that can be explained by a pair of reference haplotypes are allowed. Remaining ambiguities are resolved in a conventional expectation-maximization (EM) algorithm. DT, diplotype; dtf, preliminary diplotype frequencies; htf, preliminary haplotype frequencies; HTF, final haplotype frequencies.
HFs were estimated both for the entire KIR gene region and separately for the centromeric and telomeric sections. In order to be able to assign the allele combinations of gene cluster KIR2DL5~KIR2DS3~KIR2DS5 to a centromeric or telomeric location, we included these genes in both estimations of partial KIR HFs.
The approach to reduce the ambiguous nature of the KIR gene complex by means of the CNPS also permits the imputation of allelic haplotypes from large cohorts of individuals without a family context. In order to investigate the additional benefit of phase resolution via family affiliation, allele group diplotypes of all 916 parents of our cohort were determined in a separate workflow without the phasing knowledge of their families. Filtering of the diplotypes via the CNPS and estimation of the allele group KIR HF by the EM algorithm was carried out according to the family workflow described above.
Linkage Disequilibrium
The linkage disequilibrium (LD) coefficient D' was calculated from the entire HF set (before cut below f = 1/2n) for any two-locus combination (63, 64). Significance was tested with Fisher's exact test. P-values were subjected to a Holm-Bonferroni correction for multiple testing. Multi-locus LD analysis was conducted using the normalized entropy difference ε between the observed HFs and those expected under the null hypothesis of linkage equilibrium (64, 65). The value of ε ranges between 0 and 1, with larger values indicating stronger LD. An absent locus (“NEG”) was treated as one allele variant.
Results
KIR Genotyping Resolution of the Family Cohort
91.8% of the 22,896 (1,431 donors × 16 loci) typed loci in our final KIR family data set were determined to allelic resolution, absence, or allele group level. Of this share, 74.7% of the identified copy number equivalents were resolved to allelic level, 21.5% to absence of the locus and 3.8% to broader allele groups, where remaining ambiguities impeded the differentiation between two or more alleles.
The remaining 8.2% of the 22,896 typed loci carried ambiguities either due to unknown phasing of the typed exons (e.g., KIR2DL1*003c + KIR2DL1*004|KIR2DL1*006 + KIR2DL1*010) or to uncertain copy number determination (e.g., KIR2DL3*001 + KIR2DL3*002| KIR2DL3*001 + KIR2DL3*001 + KIR2DL3*002), allowing for multiple valid allele (group) pairs.
Parental Cohort
In the final analysis, KIR data of 790 of the 916 parents was used to estimate HFs of the entire KIR gene region. The 790 individuals originate from 403 of the 458 families. Both parents were included into the HF estimation for 387 families, 16 further families contributed one parent, each.
Reasons for exclusion from the final analysis set were: 97 parents (10.6%) were excluded because no combination of the allowed 92 copy number haplotypes could explain the deduced diplotypes. Twenty parents (2.2%) were excluded because of mismatching to all of their potential children in one KIR locus (nine families) or two KIR loci (one family). Seven further parents (0.8%) were excluded because the number of possible diplotypes after the filtering step exceeded the threshold (n = 1,000,000) of possible KIR diplotypes per individual. Finally, both parents (0.2%) of one family were omitted because the respective diplotype estimations on the basis of the KIR data of two children showed no intersection, but indicated a possible recombination event in the father's chromosomes (Supplementary Information S3). In this case, comparison of the father's different haplotype sets derived from two children revealed an exchange in alleles in locus KIR3DL3.
Consideration of only centromeric or telomeric genes for KIR haplotype estimation in the family context allowed the inclusion of more parents. HFs were calculated from KIR data of 838 (876) individuals for the centromeric (telomeric) loci. The number of individuals excluded because no combination of valid CNPS haplotypes could explain the diplotypes decreased to 6.6% (3.5%). Omission because of mismatching was reduced to 1.7% (0.7%), None of the parents were excluded due to high numbers of possible diplotypes.
When we deduced allele group diplotypes of the 916 parents for the entire KIR gene region without the phasing knowledge of the family relations, the parental cohort that could be included was considerably smaller. Applying the same filtering and configuration values as in the family approach, KIR data of only 438 (47.8%, compared to 790 or 86.2% in the family calculations) individuals passed into the HF estimation. On the one hand, only 6 individuals (0.7% vs. 10.6% in the family approach) were omitted because no valid combination of the reference copy number haplotypes could explain their diplotypes. On the other hand, however, the number of possible diplotypes exceeded the threshold of n = 1,000,000 in 473 parents (51.6 vs. 0.8% in the family approach), demonstrating the efficiency of ambiguity reduction via family information.
KIR Haplotype Frequencies for the Entire Gene Region
KIR HF estimation for the data of the 790 parents resulted in 551 different KIR allele group haplotypes with f ≥ 1/2n, corresponding to a frequency sum of 90.8% (Figure 2, Supplementary Information S4). The 20 most frequent allele group KIR haplotypes are listed in Table 1 and comprise a cumulated frequency of 26.0%.
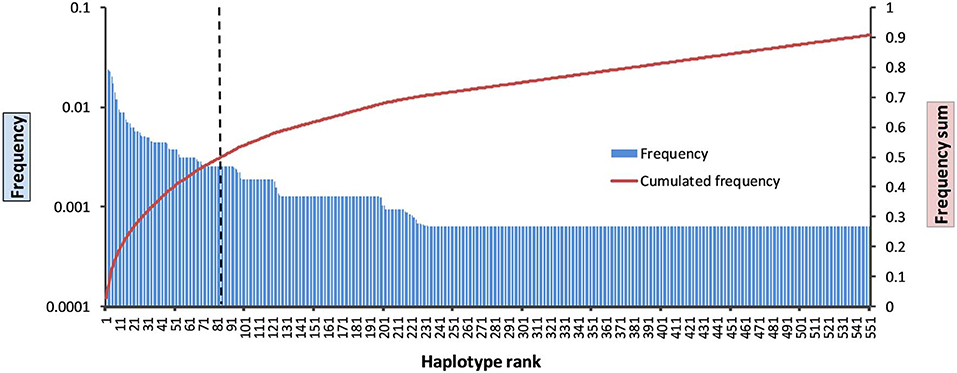
Figure 2. Frequency distribution of the 551 allele group KIR haplotypes above the resolution threshold of f = 1/2n. Blue bars: haplotype frequencies; red line: cumulated frequency; dashed black line: haplotype rank 84 with 50% cumulated haplotype frequency.
Of the 92 permitted reference copy number haplotypes, only 21 are represented in the 551 estimated allele group haplotypes. Table 2 shows identity and frequencies of these CNPS haplotypes. Where the assignment was possible, the A/B haplotype nomenclature established by 48 is indicated. The most frequent of the CNPS haplotypes is P10_01 (cA01~tA01) with f = 59.6%.
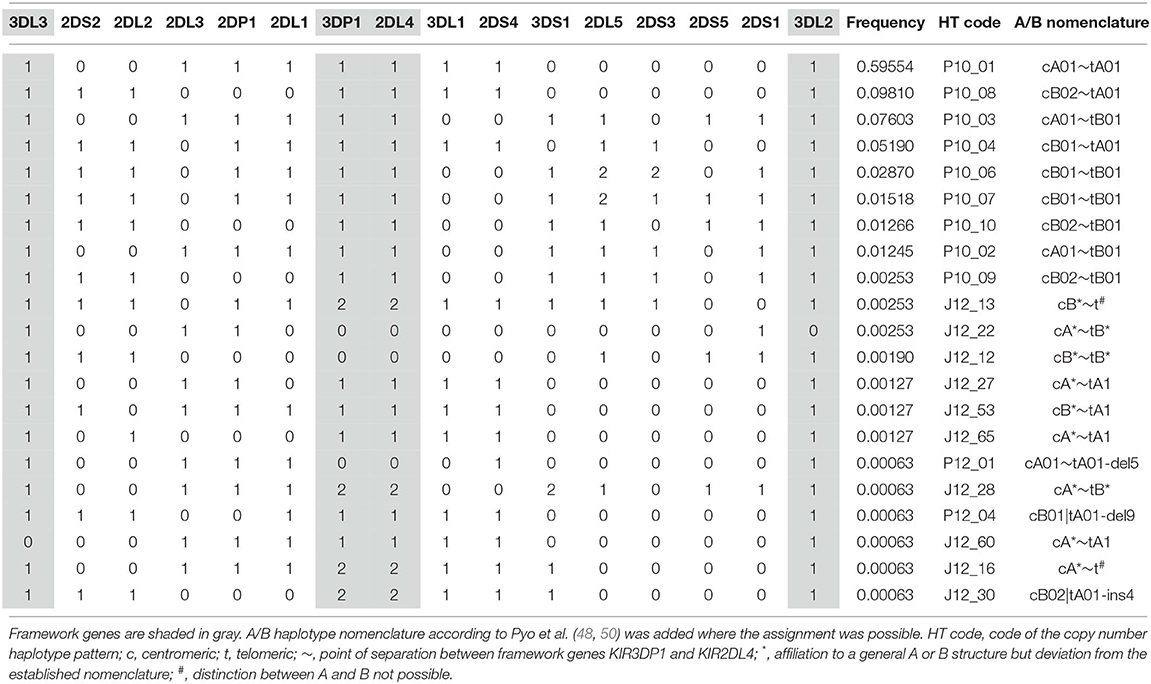
Table 2. List of the 21 KIR copy number haplotypes represented in the 551 allele group KIR haplotypes above the resolution threshold and their frequencies.
Allelic Diversity
We attributed the allele group haplotypes to the respective copy number haplotypes in order to analyze the allelic diversity within the different copy number structures (Supplementary Information S5). However, due to the different frequencies of the copy number haplotypes and thus the different chances to detect allelic diversity, observations in our dataset can only be seen as indications of patterns. For the analysis, we considered only eight copy number haplotypes with a frequency of f ≥ 1% (Table 2). The number of allele group haplotypes per copy number haplotype varies between 8 and 318, with a clear positive correlation between copy number HF and the number of allele group haplotypes. All eight copy number haplotypes are combinations of only three centromeric (cA01, cB01, and cB02) and two telomeric (tA01 and tB01) motifs (in the nomenclature introduced by Pyo et al. (48).
Figure 3 shows for each KIR gene the allele frequencies overall and for each copy number haplotype. Marked differences in overall allelic variability can be observed between the different genes with the highest diversities in the two outermost framework genes KIR3DL3 (n = 29 named alleles) and KIR3DL2 (n = 17), as well as in KIR3DL1 (n = 14). Allelic variability and identity within one locus, however, depends clearly on the respective haplotype motif. Essentially, A haplotype motifs have a higher allelic diversity than B haplotype motifs in both, the centromeric and the telomeric KIR section. In addition, the different structural motifs also correlate with different alleles. For example, in locus KIR3DL2, tB01 haplotypes (P10_02, _03, _06, _07, and _10) are clearly dominated by allele *007 with allele frequencies between 65.0 and 100%. tA01 haplotypes (P10_01, _04, and _08), in contrast, have a much higher allelic variability in KIR3DL2 where allele *007 only reaches a maximum frequency of 0.4%. Similar patterns can be seen in all framework genes and in genes KIR2DP1 and KIR2DL1, which are present in both, A and B haplotypes.
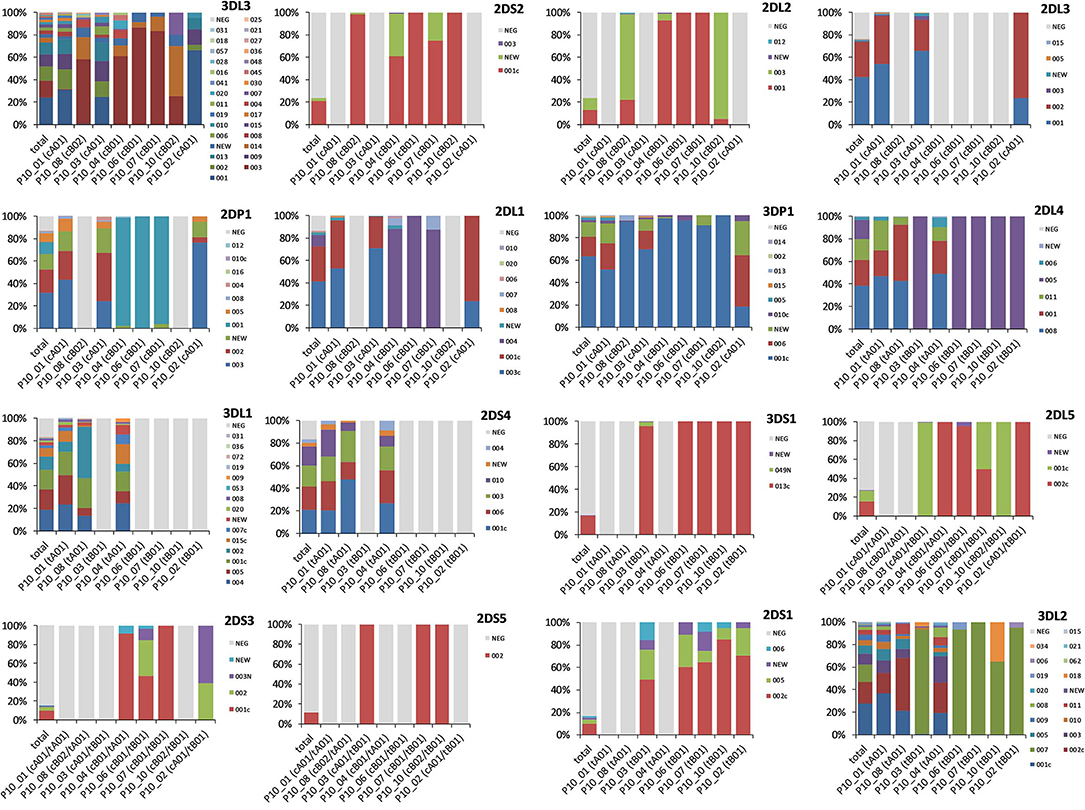
Figure 3. Allele group frequencies per KIR gene. Displayed are, for each of the 16 KIR genes, the allele frequencies for the estimated allele group KIR HF overall (total) and for the 8 CNPS haplotypes with a frequency of f ≥ 1%. The proportion of absence of the respective gene is indicated as “NEG” in light gray. The centromeric or telomeric haplotype structure of the respective CNPS haplotypes in A/B haplotype nomenclature according to Pyo et al. (48, 50) is indicated in brackets in the labeling of the diagram axes.
The allocation of allele group haplotypes to the copy number haplotypes also reveals conserved allele combinations. For instance, the partial haplotype KIR2DL2~KIR2DL3~KIR2DL1 that exhibited linkage disequilibrium also in the LD analysis (see below) displays an interesting pattern of allelic distribution. cB1 haplotypes (P10_04, _06, and _07) are almost exclusively populated with allelic haplotype block 001~NEG~004, while cA01 haplotypes (P10_01, _02, and _03) are dominated by allele group combinations NEG~001~003c and NEG~002~001c. Allele *003 prevails in cB02 haplotypes (P10_08 and _10) with only KIR2DL2 present. A second example is the gene cluster KIR2DL5~KIR2DS3~KIR2DS5. Our typing method does not allow the unambiguous assignment of this gene cluster to a centromeric or telomeric localization. However, the allele distribution of these genes in the different copy number haplotypes clearly reflects a conserved linkage of certain allele group combinations that reveal the position of the cluster in the KIR gene region. On the one hand, P10_04 (cB01~tA01), representing a centromeric localization of the cluster, is almost exclusively composed of KIR2DL5*002c~KIR2DS3*001c. A very small fraction of KIR2DS3 alleles is “NEW”. On the other hand, tB01, the common haplotype structure of P10_02, P10_03, and P10_10, is known to include a telomeric KIR2DL5~KIR2DS3~KIR2DS5 gene cluster. While the exclusive allelic composition in CN haplotypes P10_03 and P10_10 in our data is 2DL5*001c~2DS5*002, P10_02 carries either of the two combinations 2DL5*002c~2DS3*002 and 2DL5*002c~2DS3*003N. The conserved centromeric and telomeric allele combinations in our data set are listed in the Supplementary Information S6.
Linkage Disequilibrium
LD coefficient D' was calculated for all two-locus haplotypes. Altogether, 368 pairs with significant deviation from equilibrium were identified (Supplementary Information S7), 129 of them with positive D'. In a large number of the significant cases of LD, one or both of the “alleles” is the absent locus. Thirty-two pairs of actual alleles in significant LD with D'≥ 0.9 and a HF of f ≥ 0.1 are listed in Table 3. Eleven of the pairs map to the centromeric section of the KIR gene complex, 18 to the telomeric section. The three pairs including loci KIR2DL5 and KIR2DS5 show close linkage to KIR2DL4 and thus are probably located on the telomeric part of the KIR gene cluster.
Figure 4 shows the LD between the 16 analyzed loci of the KIR complex. The normalized entropy difference ε indicates a linkage disequilibrium within the designated centromeric and telomeric parts of the KIR gene complex, but much less between the two parts. For the pair of the two inner framework genes KIR3DP1 and KIR2DL4, the value is close to equilibrium (ε = 0.07). The highest telomeric LD (ε = 0.42) is found for the loci KIR3DL1 and KIR2DS4. In the vast majority of haplotypes, amongst others in the by far most frequent copy number haplotype P10_01(cA01~tA01), those two loci are adjacent and either both present or both absent. In addition, KIR3DL1 is in LD with framework gene KIR2DL4 (ε = 0.38), and KIR2DL4 with KIR2DS4 (ε = 0.39). In the centromeric stretch, the highest LD (ε = 0.37) is found for KIR2DS2 and KIR2DL2, two adjacent and usually concurrent loci. A further block of linkage disequilibrium is formed by loci KIR2DL3, KIR2DP1, and KIR2DL1 (0.32 ≤ ε ≤ 0.36). The three loci KIR2DL5, KIR2DS3, and KIR2DS5 play a special role in the multi-locus LD analysis since our typing method does not allow the separation of KIR2DL5A and KIR2DL5B and thus the direct assignment of the KIR2DL5~KIR2DS3~KIR2DS5 cluster to centromeric or telomeric position on the chromosome. The linkage analysis reveals LD for the two pairs KIR2DL5~KIR2DS3 (ε = 0.32) and KIR2DL5~KIR2DS5 (ε = 0.30), while the two loci KIR2DS3 and KIR2DS5 are almost in linkage equilibrium (ε = 0.01). Overall, the linkage of the three genes of this cluster is higher to loci of the telomeric than to loci of the centromeric region. KIR2DS5 shows no linkage to genes of the centromeric KIR region at all. This is consistent with the conserved centromeric and telomeric allele combinations observed for the KIR2DL5~KIR2DS3~KIR2DS5 cluster, according to which KIR2DS5 is not present in centromeric position.
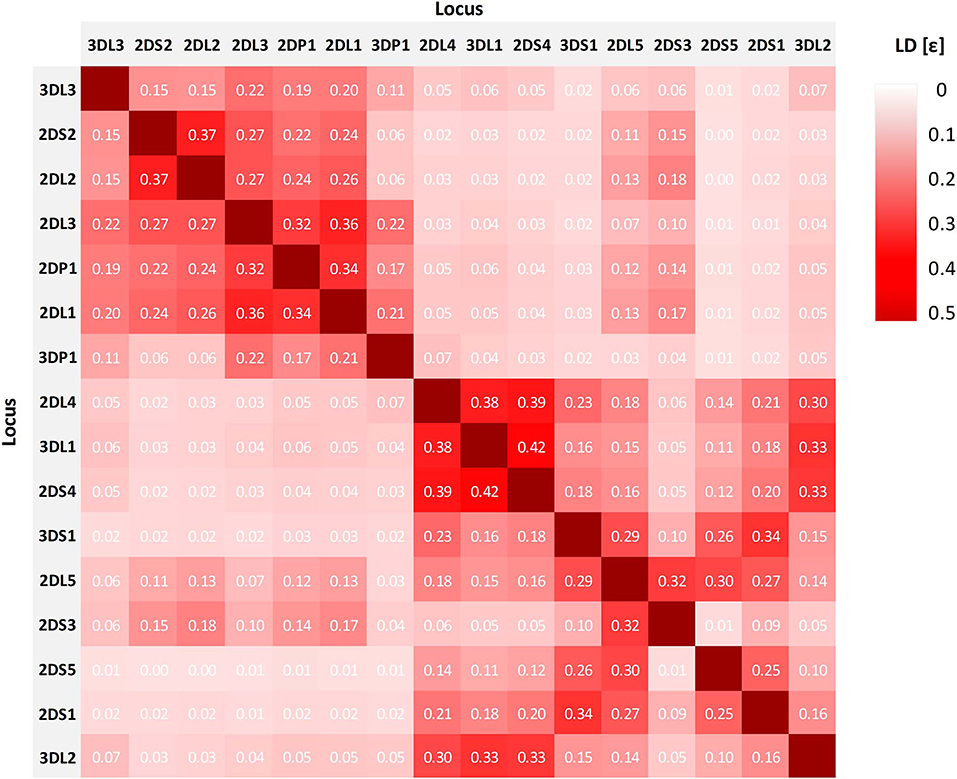
Figure 4. Overall linkage disequilibrium of 16 KIR loci in genomic orientation as designated by the normalized entropy difference ε. The value of ε ranges between 0 and 1, with larger values indicating stronger LD. Values of ε are displayed in the tiles.
Assignment of KIR2DL5~KIR2DS3~KIR2DS5 to Centromeric or Telomeric Position on the Haplotype
The analysis of the allelic diversity of different copy number haplotype structures revealed the association of conserved allele combinations of gene cluster KIR2DL5~KIR2DS3~KIR2DS5 with its localization on the chromosome (Supplementary Information S6). We applied this information to assign centromeric and telomeric positions of the gene cluster in our KIR HFs.
Of the 551 KIR allele group haplotypes, 164 contained the respective genes in a total of 11 different combinations. In 96.3% of the cases, the localization of the KIR2DL5~KIR2DS3~KIR2DS5 genes could be assigned unambiguously. Six cases in two allele combinations included “NEW” alleles in KIR2DS3. For these cases we deduced the position of the clusters in the context of the overall haplotype structure. KIR HFs for the entire gene region with inferred localization of gene clusters KIR2DL5~KIR2DS3~KIR2DS5 are shown in the Supplementary Information S8.
Frequencies of Partial KIR Haplotypes
Estimation of KIR HFs of the centromeric gene region from KIR data of 838 parents resulted in 323 different allele group haplotypes with f ≥ 1/2n (frequency sum 97.1%), representing 22 partial copy number haplotypes (Supplementary Information S9). Frequency of the three structural haplotypes cA01, cB01, and cB02 reached a total of 92.8%. Assignment of cluster KIR2DL5~KIR2DS3~KIR2DS to the centromeric haplotypes was deduced as described above. Interestingly, the partial HFs of the centromeric KIR genes reveal an additional allele combination of the KIR2DL5~KIR2DS3~KIR2DS5 cluster. One haplotype with the combination KIR2DL5*019B~ KIR2DS5*008 was found, the only centromeric occurrence of KIR2DS5 in our study. Its frequency (f = 5.97*10−4) indicates a unique occurrence of the haplotype in our sample.
Accordingly, telomeric KIR HFs, including KIR data of 876 parents yielded 146 allele group haplotypes with f ≥ 1/2n (frequency sum 98.8%). Only 12 partial copy number haplotypes were represented. The frequency of copy number haplotypes tA01, tB01 (KIR2DS3 present), and tB01 (KIR2DS5 present) added up to 96.8% (Supplementary Information S10).
Discussion
We present allele group KIR haplotypes estimated from 790 parents of a cohort of n = 403 German families. The restriction of haplotype structures to a set of copy number haplotypes from previous publications enabled us to cope with the enormous structural ambiguities of the KIR gene complex. Deducing diplotypes within the context of families allowed us to further reduce the number of possible diplotypes per individual. Our approach yielded a set of 551 allele group KIR haplotypes, representing 21 of the 92 CNPS haplotypes.
The results of our study are in good accordance with two other studies on allelic KIR haplotypes. A table comparing our Top20 haplotypes with findings of Vierra-Green et al. (44), Hou et al. (52) can be found in the Supplementary Information S11. Both former studies inferred their allelic HFs from cohorts of European ancestry without a family context and did not include information on the polymorphism of the pseudogene loci KIR2DP1 and KIR3DP1 in their haplotype determination. Haplotype structures were restricted to smaller reference sets of content haplotypes.
Nineteen of our Top 20 allele group KIR haplotypes correspond to allelic haplotypes published by Vierra-Green et al. (44). For the most frequent haplotype in both studies, KIR3DL3*003~KIR2DS2*001c~KIR2DL2*003~KIR3DP1*001c~KIR2DL4*001~KIR3DL1*002~KIR2DS4*001c~KIR3DL2*002c, we estimated a frequency of f = 0.0290, while Vierra-Green et al. determined f = 0.0306. During comparison of the allelic haplotypes it became apparent that several haplotypes in Vierra-Green et al. carried allele KIR2DS4*007 which never occurred in our haplotype set. Analysis of the sequence provided a potential explanation: KIR2DS4*007 differs from KIR2DS4*010 only in the first base of exon 4. As KIR2DS4*010 was not listed in the IPD-KIR database before version 2.2.0 (May 2010), this allele may not have been included in allele typing and interpretation of the former study. Our typing routine distinguishes between the two alleles and we find almost exclusively KIR2DS4*010 in our donors.
In a study published by Hou et al. (52), the centromeric and telomeric stretches of the KIR gene complex were analyzed separately and allelic haplotypes were grouped into different consensus structures. Our estimated allele group KIR haplotypes are in good agreement with their findings (Supplementary Information S7). The centromeric parts of 19 of our Top 20 allele group haplotypes were also described by Hou et al. They could be assigned to four of the five postulated centromeric consensus structures. In the case of the telomeric part, 18 of our Top 20 allele group haplotypes were described in the previous study. They account for 7 out of 8 suggested telomeric haplotype structures.
The comparison of our results to KIR haplotype data of non-European individuals shows clearly less similarities. A recent study on HLA and KIR diversity in individuals from African populations reveals differences in the KIR HFs between the seven populations from Western, Central, and Eastern Africa (66). The African haplotypes furthermore show a different allelic distribution than those of our German cohort. Some of the alleles show exclusive occurrence in one of the geographically distinct cohorts. Alleles 3DL3*001 or 3DL1*002, which are, for example, frequent in our sample, were not observed in the African samples. Vice versa, the African cohorts contain frequent alleles that are not found in our German haplotypes, e.g., 3DL3*005 or 3DL1*017. The centromeric part of only seven and the telomeric part of 10 of our Top 20 allele group haplotypes are present in the African cohorts.
Two levels of linkage are visible in our LD analyses. On the one hand, observed LD in pairs of KIR loci clearly reflect the structural constraints of KIR haplotypes by echoing proximity of loci as given in the different CNPS haplotypes. On the other hand, the linkage of certain allele groups within these structural constraints indicates a non-stochastic distribution of alleles to particular haplotypes. The low deviation from linkage equilibrium between genes of the centromeric and the telomeric gene stretch supports the assumption of a recombination hot spot between KIR3DP1 and KIR2DL4. Our findings on LD confirm data from previous research (44). Our set of 32 allele group LD pairs includes 20 of the 26 two-allele haplotypes that showed significant LD in the former study.
The genetic linkage of alleles within given haplotype structures reflected in the LD analysis is confirmed when allele group haplotypes are clustered by their underlying copy number haplotype. The analysis reveals two interesting results: First, allelic variability in our cohort is found to be higher in A than in B haplotypes. This observation agrees with previous descriptions (48) and is also in accord with findings of lower overall allelic diversity in the activating compared to the inhibitory loci (44, 53). And second, we observe conserved allelic correlations specific to five distinct centromeric and telomeric haplotype motifs (cA01, cB01, cB02, tA01, and tB01). The detected differences in allelic variability and the correlation of certain alleles to A and B haplotype motifs also comprises the framework genes. These results indicate a KIR gene inheritance in closely linked blocks with a recombination hot spot between the genes KIR3DP1 and KIR2DL4. The very particular allelic linkage within the KIR2DL5~KIR2DS3/2DS5 haplotype enabled us to assign this gene cluster to centromeric or telomeric position in our KIR haplotypes even though our typing method did not allow a differentiation between the two loci KIR2DL5A and KIR2DL5B. Beyond that, the observed allele group haplotype patterns of the telomeric and centromeric KIR2DL5~KIR2DS3/2DS5 clusters correspond to those described in previous publications on individuals of European ancestry (52, 60).
The knowledge of family relations in our cohort substantially reduced the number of possible diplotypes per individual. This had two consequences. First, it increased the number of individuals who were excluded from the KIR HF estimation due to the absence of a valid combination of CNPS haplotypes that explained their diplotypes. This demonstrates, on the one hand, the incompleteness of our used CNPS haplotype list. On the other hand, it reveals the limitation of KIR HF estimation without family context, where, in consequence, the same KIR genotypes were described with at least one incorrectly assigned pair of CNPS haplotypes. And second, the reduced number of possible diplotypes per individual provided a reasonable basis for a successful execution of an EM algorithm. Apart from reducing phasing ambiguities, the family context further offers the potential to detect typing inaccuracies and recombination events that would remain hidden otherwise.
Given the multiple constraints we applied to our KIR data in order to estimate allele group KIR HFs, several sources of possible bias to our results have to be considered. One important bias may be caused by the restriction of KIR haplotypes to a limited copy number pattern set. For 10.6% of the parents in our cohort, the deduced diplotypes could not be explained by a pair of haplotypes from the CNPS, indicating further KIR complexity on the copy number level and the need for extension of the CNPS. The estimation of partial (centromeric and telomeric) haplotypes of the same cohort led to a reduction of this percentage of excluded individuals. This indicates that the missing structural diversification of the pattern haplotypes is mostly limited to variation in sub-ranges of the KIR gene complex. Thorough analysis of the diplotypes of the excluded individuals by alternative typing methods beyond the routine high-throughput KIR genotyping in the context of unrelated HSCT donor registration (53) will be of great importance for the future refinement of our approach. Such extended analyses could also detect hybrid genes that are not described in the IPD-KIR database to which our routine typing method is blind, but go beyond the scope of the present study. Further bias may be introduced by sequencing or sequence interpretation errors. However, the impact of this potential bias should be minimal with our approach because the low overall error rate of the NGS high throughput platform was further reduced by verification of the KIR data within the families. The accuracy of the high throughput KIR analysis in a curated sample was found to exceed 99% (53). In the 13 remaining cases of mismatching loci and one presumable case of recombination in our cohort, where typing errors could not be excluded as a cause without re-typing of the respective family members, parents were excluded from the HF estimation. Moreover, the restriction of the cohort to individuals with German origin needs to be treated with some caution. This information was collected via self-assessment of the volunteer stem cell donors during the registration process. The perception of the term “origin” is quite individual (67) and in many cases it is simply difficult to describe an individual's origin by means of one single country code. Finally, family lineage was not known for the individuals of our cohort but deduced from concordance of surname and address and a defined age difference between presumed parents and offspring. However, the verification of genetic relationship via the HLA genes and exclusion of families with any mismatching loci in the KIR genes from the final HF estimation should compensate for lack of direct family information.
In conclusion, our approach yielded a set of 551 allele group KIR haplotype frequencies from a German cohort that is in good accordance with results from other groups. We provide additional data on the diversification of KIR haplotypes by inclusion of allelic polymorphisms of pseudogenes KIR2DP1 and KIR3DP1. The use of family information during diplotype deduction allowed the exclusion of incorrect phasing variants. Our KIR haplotype frequencies reveal relations between KIR copy number haplotypes and allele frequencies, which will be a valuable basis for future research. The application of this approach, e.g., to larger cohorts of different ethnic origin, will further broaden our knowledge and understanding of the very complex nature of the KIR genes.
Research Data
DNA was extracted from blood samples or buccal swabs with the informed consent of the donors. Research data used in this publication was collected from the data subjects and processed on the basis of an informed consent in accordance with the EU Data Protection Regulation (EU-GDPR). Data subjects agreed to their data being processed for scientific studies, in particular with the aim to improving the treatment of patients with blood cancer and other life threatening diseases. The publication itself does not include identifiable personal data.
Data Availability Statement
The raw data supporting the conclusions of this article will be made available by the authors, without undue reservation, to any qualified researcher.
Author Contributions
JSa and US conceived the project. US designed and implemented algorithms, carried out analyses, and wrote the first draft of the manuscript. GS, CM, AH, MK, DS, and JP analyzed and interpreted KIR genotyping data. All authors contributed to manuscript revision, read, and approved the submitted version.
Conflict of Interest
The authors declare that the research was conducted in the absence of any commercial or financial relationships that could be construed as a potential conflict of interest.
Acknowledgments
We thank all the altruistic volunteers who register as potential donors to help strangers in need of a stem cell transplant. We would also like to thank Beatrix Willburger for valuable advice during the implementation of the algorithm. We thank the reviewers for their valuable advice and comments.
Supplementary Material
The Supplementary Material for this article can be found online at: https://www.frontiersin.org/articles/10.3389/fimmu.2020.00429/full#supplementary-material
Abbreviations
CNPS, copy number haplotype pattern set; EM, expectation-maximization; GvHD, graft-versus-host disease; HF, haplotype frequency; HLA, human leukocyte antigen; HSCT, hematopoietic stem cell transplantation; KIR, Killer-cell immunoglobulin-like receptor; LD, linkage disequilibrium; MHC, major histocompatibility complex; NK, natural killer.
References
1. Marsh SGE, Parham P, Dupont B, Geraghty DE, Trowsdale J, Middleton D, et al. Killer-cell immunoglobulin-like receptor (KIR) nomenclature report, 2002. Hum Immunol. (2003) 64:648–54. doi: 10.1016/S0198-8859(03)00067-3
2. Wilson MJ, Torkar M, Haude A, Milne S, Jones T, Sheer D, et al. Plasticity in the organization and sequences of human KIR/ILT gene families. Proc Natl Acad Sci USA. (2000) 97:4778–83. doi: 10.1073/pnas.080588597
3. Middleton D, Gonzelez F. The extensive polymorphism of KIR genes. Immunology. (2010) 129:8–19. doi: 10.1111/j.1365-2567.2009.03208.x
4. Jiang W, Johnson C, Jayaraman J, Simecek N, Noble J, Moffatt MF, et al. Copy number variation leads to considerable diversity for B but not A haplotypes of the human KIR genes encoding NK cell receptors. Genome Res. (2012) 22:1845–54. doi: 10.1101/gr.137976.112
5. Robinson J, Waller MJ, Stoehr P, Marsh SG. IPD–the immuno polymorphism database. Nucleic Acids Res. (2005) 33:D523–6. doi: 10.1093/nar/gki032
6. Augusto DG, Norman PJ, Dandekar R, Hollenbach JA. Fluctuating and geographically specific selection characterize rapid evolution of the human KIR region. Front Immunol. (2019) 10:989. doi: 10.3389/fimmu.2019.00989
7. Shilling HG, Lienert-Weidenbach K, Valiante NM, Uhrberg M, Parham P. Evidence for recombination as a mechanism for KIR diversification. Immunogenetics. (1998) 48:413–6. doi: 10.1007/s002510050453
8. Martin MP, Bashirova A, Traherne J, Trowsdale J, Carrington M. Cutting edge: expansion of the KIR locus by unequal crossing over. J Immunol. (2003) 171:2192–5. doi: 10.4049/jimmunol.171.5.2192
9. Abi-Rached L, Parham P. Natural selection drives recurrent formation of activating killer cell immunoglobulin-like receptor and Ly49 from inhibitory homologues. J Exp Med. (2005) 201:1319–32. doi: 10.1084/jem.20042558
10. Gomez-Lozano N, Estefania E, Williams F, Halfpenny I, Middleton D, Solis R, et al. The silent KIR3DP1 gene (CD158c) is transcribed and might encode a secreted receptor in a minority of humans, in whom the KIR3DP1, KIR2DL4 and KIR3DL1/KIR3DS1 genes are duplicated. Eur J Immunol. (2005) 35:16–24. doi: 10.1002/eji.200425493
11. Norman PJ, Abi-Rached L, Gendzekhadze K, Hammond JA, Moesta AK, Sharma D, et al. Meiotic recombination generates rich diversity in NK cell receptor genes, alleles, and haplotypes. Genome Res. (2009) 19:757–69. doi: 10.1101/gr.085738.108
12. Traherne JA, Martin M, Ward R, Ohashi M, Pellett F, Gladman D, et al. Mechanisms of copy number variation and hybrid gene formation in the KIR immune gene complex. Hum Mol Genet. (2010) 19:737–51. doi: 10.1093/hmg/ddp538
13. Ordonez D, Gomez-Lozano N, Rosales L, Vilches C. Molecular characterisation of KIR2DS2*005, a fusion gene associated with a shortened KIR haplotype. Genes Immun. (2011) 12:544–51. doi: 10.1038/gene.2011.35
14. Roe D, Vierra-Green C, Pyo CW, Eng K, Hall R, Kuang R, et al. Revealing complete complex KIR haplotypes phased by long-read sequencing technology. Genes Immun. (2017). 18:127–34. doi: 10.1038/gene.2017.10
15. Bruijnesteijn J, van der Wiel MKH, de Groot N, Otting N, de Vos-Rouweler AJM, Lardy NM, et al. Extensive alternative splicing of KIR transcripts. Front Immunol. (2018) 9:2846. doi: 10.3389/fimmu.2018.02846
16. Vivier E, Ugolini S, Blaise D, Chabannon C, Brossay L. Targeting natural killer cells and natural killer T cells in cancer. Nat Rev Immunol. (2012) 12:239–52. doi: 10.1038/nri3174
17. Parham P, Moffett A. Variable NK cell receptors and their MHC class I ligands in immunity, reproduction and human evolution. Nat Rev Immunol. (2013) 13:133–44. doi: 10.1038/nri3370
18. Cooley S, Parham P, Miller JS. Strategies to activate NK cells to prevent relapse and induce remission following hematopoietic stem cell transplantation. Blood. (2018) 131:1053–62. doi: 10.1182/blood-2017-08-752170
19. Uhrberg M, Valiante NM, Shum BP, Shilling HG, Lienert-Weidenbach K, Corliss B, et al. Human diversity in killer cell inhibitory receptor genes. Immunity. (1997) 7:753–63. doi: 10.1016/S1074-7613(00)80394-5
20. Faure M, Long EO. KIR2DL4 (CD158d), an NK cell-activating receptor with inhibitory potential. J Immunol. (2002) 168:6208–14. doi: 10.4049/jimmunol.168.12.6208
21. Rajagopalan S, Long EO. KIR2DL4 (CD158d): An activation receptor for HLA-G. Front Immunol. (2012) 3:258. doi: 10.3389/fimmu.2012.00258
22. Gómes-Lozano N, de Pablo R, Puente S, Vilches C. Recognition of HLA-G by the NK cell receptor KIR2DL4 is not essential for human reproduction. Eur J Immunol. (2003) 33:639–44. doi: 10.1002/eji.200323741
23. Lee SJ, Klein J, Haagenson M, Baxter-Lowe LA, Confer DL, Eapen M, et al. High-resolution donor-recipient HLA matching contributes to the success of unrelated donor marrow transplantation. Blood. (2007) 110:4576–83. doi: 10.1182/blood-2007-06-097386
24. Pidala J, Lee SJ, Ahn KW, Spellman S, Wang HL, Aljurf M, et al. Nonpermissive HLA-DPB1 mismatch increases mortality after myeloablative unrelated allogeneic hematopoietic cell transplantation. Blood. (2014) 124:2596–606. doi: 10.1182/blood-2014-05-576041
25. Dehn J, Spellman S, Hurley CK, Shaw BE, Barker JN, Burns LJ, et al. Selection of unrelated donors and cord blood units for hematopoietic cell transplantation: guidelines from NMDP/CIBMTR. Blood. (2019) 134:924–34. doi: 10.1182/blood.2019001212
26. Ullah MA, Hill GR, Tey SK. Functional Reconstitution of natural killer cells in allogeneic hematopoietic stem cell transplantation. Front Immunol. (2016) 7:144. doi: 10.3389/fimmu.2016.00144
27. Ruggeri L, Capanni M, Casucci M, Volpi I, Tosti A, Perruccio K, et al. Role of natural killer cell alloreactivity in HLA-mismatched hematopoietic stem cell transplantation. Blood. (1999) 94:333–9. doi: 10.1182/blood.V94.1.333.413a31_333_339
28. Ruggeri L, Capanni M, Urbani E, Perruccio K, Shlomchik WD, Tosti A, et al. Effectiveness of donor natural killer cell alloreactivity in mismatched hematopoietic transplants. Science. (2002) 295:2097–100. doi: 10.1126/science.1068440
29. Giebel S, Locatelli F, Lamparelli T, Velardi A, Davies S, Frumento G, et al. Survival advantage with KIR ligand incompatibility in hematopoietic stem cell transplantation from unrelated donors. Blood. (2003) 102:814–9. doi: 10.1182/blood-2003-01-0091
30. Giebel S, Locatelli F, Wojnar J, Velardi A, Mina T, Giorgiani G, et al. Homozygosity for human leucocyte antigen-C ligands of KIR2DL1 is associated with increased risk of relapse after human leucocyte antigen-C-matched unrelated donor haematopoietic stem cell transplantation. Br J Haematol. (2005) 131:483–6. doi: 10.1111/j.1365-2141.2005.05797.x
31. Miller JS, Cooley S, Parham P, Farag SS, Verneris MR, McQueen KL, et al. Missing KIR ligands are associated with less relapse and increased graft-versus-host disease (GVHD) following unrelated donor allogeneic HCT. Blood. (2007) 109:5058–61. doi: 10.1182/blood-2007-01-065383
32. Heidenreich S, Kroger N. Reduction of relapse after unrelated donor stem cell transplantation by KIR-based graft selection. Front Immunol. (2017) 8:41. doi: 10.3389/fimmu.2017.00041
33. Cooley S, Trachtenberg E, Bergemann TL, Saeteurn K, Klein J, Le CT, et al. Donors with group B KIR haplotypes improve relapse-free survival after unrelated hematopoietic cell transplantation for acute myelogenous leukemia. Blood. (2009) 113:726–32. doi: 10.1182/blood-2008-07-171926
34. Venstrom JM, Pittari G, Gooley TA, Chewning JH, Spellman S, Haagenson M, et al. HLA-C-dependent prevention of leukemia relapse by donor activating KIR2DS1. N Engl J Med. (2012) 367:805–16. doi: 10.1056/NEJMoa1200503
35. Boudreau JE, Giglio F, Gooley TA, Stevenson PA, Le Luduec J-B, Shaffer BC, et al. KIR3DL1/HLA-B subtypes govern acute myelogenous leukemia relapse after hematopoietic cell transplantation. J Clin Oncol. (2017). doi: 10.1200/JCO.2016.70.7059
36. Schetelig J, Baldauf H, Koster L, Kuxhausen M, Heidenreich F, De Wreede LC, et al. Does donor KIR-genotype impact outcome after unrelated hematopoietic stem cell transplantation for myelodysplastic syndromes or secondary acute myeloid leukemia? Bone Marrow Transplant. (2019) 54:561–2. doi: 10.1038/s41409-019-0559-4
37. Schetelig J, Baldauf H, Heidenreich F, Massalski C, Frank S, Sauter J, et al. External validation of models for KIR2DS1/KIR3DL1-informed Selection of hematopoietic cell donors fails. Blood. (2020). doi: 10.1182/blood.2019002887. [Epub ahead of print].
38. Urban C, Schmidt AH, Hofmann JA. (2020). Hap-E Search 2.0: improving the performance of a probabilistic donor-recipient matching algorithm based on haplotype frequencies. Front Med. 7:32. doi: 10.3389/fmed.2020.00032
39. Bochtler W, Gragert L, Patel ZI, Robinson J, Steiner D, Hofmann JA, et al. A comparative reference study for the validation of HLA-matching algorithms in the search for allogeneic hematopoietic stem cell donors and cord blood units. HLA. (2016) 87:439–48. doi: 10.1111/tan.12817
40. Schmidt AH, Sauter J, Pingel J, Ehninger G. Toward an optimal global stem cell donor recruitment strategy. PLoS ONE. (2014) 9:e86605. doi: 10.1371/journal.pone.0086605
41. Petersdorf EW, Malkki M, Gooley TA, Martin PJ, Guo Z. MHC haplotype matching for unrelated hematopoietic cell transplantation. PLoS Med. (2007) 4:e8. doi: 10.1371/journal.pmed.0040008
42. Joris MM, Lankester AC, von dem Borne PA, Kuball J, Bierings M, Cornelissen JJ, et al. The impact of frequent HLA haplotypes in high linkage disequilibrium on donor search and clinical outcome after unrelated haematopoietic SCT. Bone Marrow Transplant. (2013) 48:483–90. doi: 10.1038/bmt.2012.189
43. Buhler S, Baldomero H, Ferrari-Lacraz S, Nunes JM, Sanchez-Mazas A, Massouridi-Levrat S, et al. High-resolution HLA phased haplotype frequencies to predict the success of unrelated donor searches and clinical outcome following hematopoietic stem cell transplantation. Bone Marrow Transplant. (2019) 54:1701–9. doi: 10.1038/s41409-019-0520-6
44. Vierra-Green C, Roe D, Hou L, Hurley CK, Rajalingam R, Reed E, et al. Allele-level haplotype frequencies and pairwise linkage disequilibrium for 14 KIR loci in 506 European-American individuals. PLoS ONE. (2012) 7:e47491. doi: 10.1371/journal.pone.0047491
45. Vierra-Green C, Roe D, Jayaraman J, Trowsdale J, Traherne J, Kuang R, et al. Estimating KIR haplotype frequencies on a cohort of 10,000 individuals: a comprehensive study on population variations, typing resolutions, and reference haplotypes. PLoS ONE. (2016) 11:e0163973. doi: 10.1371/journal.pone.0163973
46. Hsu KC, Liu XR, Selvakumar A, Mickelson E, O'Reilly RJ, Dupont B. Killer Ig-Like receptor haplotype analysis by gene content: evidence for genomic diversity with a minimum of six basic framework haplotypes, each with multiple subsets. J Immunol. (2002) 169:5118–29. doi: 10.4049/jimmunol.169.9.5118
47. Uhrberg M, Parham P, Wernet P. Definition of gene content for nine common group B haplotypes of the caucasoid population: KIR haplotypes contain between seven and eleven KIR genes. Immunogenetics. (2002) 54:221–9. doi: 10.1007/s00251-002-0463-7
48. Pyo CW, Guethlein LA, Vu Q, Wang R, Abi-Rached L, Norman PJ, et al. Different patterns of evolution in the centromeric and telomeric regions of group A and B haplotypes of the human killer cell Ig-like receptor locus. PLoS ONE. (2010) 5:e15115. doi: 10.1371/journal.pone.0015115
49. Middleton D, Meenagh A, Gourraud PA. KIR haplotype content at the allele level in 77 Northern Irish families. Immunogenetics. (2007) 59:145–58. doi: 10.1007/s00251-006-0181-7
50. Pyo CW, Wang R, Vu Q, Cereb N, Yang SY, Duh FM, et al. Recombinant structures expand and contract inter and intragenic diversification at the KIR locus. BMC Genomics. (2013) 14:89. doi: 10.1186/1471-2164-14-89
51. Shilling HG, Guethlein LA, Cheng NW, Gardiner CM, Rodriguez R, Tyan D, et al. Allelic polymorphism synergizes with variable gene content to individualize human KIR genotype. J Immunol. (2002) 168:2307–15. doi: 10.4049/jimmunol.168.5.2307
52. Hou L, Chen M, Ng J, Hurley CK. Conserved KIR allele-level haplotypes are altered by microvariation in individuals with European ancestry. Genes Immun. (2012) 13:47–58. doi: 10.1038/gene.2011.52
53. Wagner I, Schefzyk D, Pruschke J, Schöfl G, Schöne B, Gruber N, et al. Allele-level KIR genotyping of more than a million samples: workflow, algorithm, and observations. Front Immunol. (2018) 9:2843. doi: 10.3389/fimmu.2018.02843
54. Schmidt AH, Lange V, Hofmann JA, Schetelig J, Pingel J. KIR genotyping data of more than 3 million individuals are available for global unrelated stem cell donor searches. (comment to: Weisdorf D, Cooley S, Wang T, et al. KIR donor selection: feasibility in identifying better donors). Biol Blood Marrow Transplant. (2019) 25:e39–40. doi: 10.1016/j.bbmt.2018.09.020
55. Lange V, Böhme I, Hofmann J, Lang K, Sauter J, Schöne B, et al. Cost-efficient high-throughput HLA typing by MiSeq amplicon sequencing. BMC Genomics. (2014) 15:63. doi: 10.1186/1471-2164-15-63
56. Schöfl G, Lang K, Quenzel P, Böhme I, Sauter J, Hofmann JA, et al. 2.7 million samples genotyped for HLA by next generation sequencing: lessons learned. BMC Genomics. (2017) 18:161. doi: 10.1186/s12864-017-3575-z
57. Lang K, Wagner I, Schöne B, Schöfl G, Birkner K, Hofmann JA, et al. ABO allele-level frequency estimation based on population-scale genotyping by next generation sequencing. BMC Genomics. (2016) 17:374. doi: 10.1186/s12864-016-2687-1
58. Solloch UV, Lang K, Lange V, Böhme I, Schmidt AH, Sauter J. Frequencies of gene variant CCR5-Δ32 in 87 countries based on next-generation sequencing of 1.3 million individuals sampled from 3 national DKMS donor centers. Hum Immunol. (2017) 78:710–7. doi: 10.1016/j.humimm.2017.10.001
59. Klussmeier A, Massalski C, Putke K, Schäfer G, Sauter J, Schefzyk D, et al. High-throughput MICA/B genotyping of over two million samples: workflow and allele frequencies. Front Immunol. (2020) 11:314. doi: 10.3389/fimmu.2020.00314
60. Ordonez D, Meenagh A, Gomez-Lozano N, Castano J, Middleton D, Vilches C. Duplication, mutation and recombination of the human orphan gene KIR2DS3 contribute to the diversity of KIR haplotypes. Genes Immun. (2008) 9:431–7. doi: 10.1038/gene.2008.34
61. Cisneros E, Moraru M, Gómez-Lozano N, López-Botet M, Vilches C. KIR2DL5: an orphan inhibitory receptor displaying complex patterns of polymorphism and expression. Front Immunol. (2012) 3:289. doi: 10.3389/fimmu.2012.00289
62. Excoffier L, Slatkin M. Maximum-likelihood estimation of molecular haplotype frequencies in a diploid population. Mol Biol Evol. (1995) 12:921–7.
63. Lewontin RC. The interaction of selection and linkage. I general considerations; heterotic models. Genetics. (1964) 49:49–67.
64. Nothnagel M, Fürst R, Rohde K. Entropy as a measure for linkage disequilibrium over multilocus haplotype blocks. Hum Hered. (2002) 54:186–98. doi: 10.1159/000070664
65. Okada Y. eLD: Entropy-based linkage disequilibrium index between multiallelic sites. Hum Genome Var. (2018) 5:29. doi: 10.1038/s41439-018-0030-x
66. Nemat-Gorgani N, Guethlein LA, Henn BM, Norberg SJ, Chiaroni J, Sikora M, et al. Diversity of KIR, HLA class I, and their interactions in seven populations of Sub-Saharan Africans. J Immunol. (2019) 202:2636–47. doi: 10.4049/jimmunol.1801586
67. Hollenbach JA, Saperstein A, Albrecht M, Vierra-Green C, Parham P, Norman PJ, et al. Race, ethnicity and ancestry in unrelated transplant matching for the national marrow donor program: a comparison of multiple forms of self-identification with genetics. PLoS ONE. (2015) 10:e0135960. doi: 10.1371/journal.pone.0135960
Keywords: KIR, HSCT, haplotype frequency, donor selection, immunogenetics
Citation: Solloch UV, Schefzyk D, Schäfer G, Massalski C, Kohler M, Pruschke J, Heidl A, Schetelig J, Schmidt AH, Lange V and Sauter J (2020) Estimation of German KIR Allele Group Haplotype Frequencies. Front. Immunol. 11:429. doi: 10.3389/fimmu.2020.00429
Received: 14 November 2019; Accepted: 25 February 2020;
Published: 12 March 2020.
Edited by:
Martin Maiers, National Marrow Donor Program, United StatesReviewed by:
Lisbeth Guethlein, Stanford University, United StatesCarlos Vilches, Hospital Universitario Puerta de Hierro Majadahonda, Spain
Lihua Hou, Georgetown University, United States
Copyright © 2020 Solloch, Schefzyk, Schäfer, Massalski, Kohler, Pruschke, Heidl, Schetelig, Schmidt, Lange and Sauter. This is an open-access article distributed under the terms of the Creative Commons Attribution License (CC BY). The use, distribution or reproduction in other forums is permitted, provided the original author(s) and the copyright owner(s) are credited and that the original publication in this journal is cited, in accordance with accepted academic practice. No use, distribution or reproduction is permitted which does not comply with these terms.
*Correspondence: Ute V. Solloch, c29sbG9jaEBka21zLmRl