- 1Department of Pediatrics, UC Davis School of Medicine, Sacramento, CA, United States
- 2Division of Cardiovascular Medicine, Department of Internal Medicine, UC Davis Health System, Sacramento, CA, United States
- 3Department of Veterans Affairs, Northern California Health Care System, Mather, CA, United States
- 4Department of Hospital Medicine, Children’s National Health System, Washington, DC, United States
Background: In extremely premature infants, postnatal growth restriction (PNGR) is common and increases the risk of developing bronchopulmonary dysplasia (BPD) and pulmonary hypertension (PH). Mechanisms by which poor nutrition impacts lung development are unknown, but alterations in the gut microbiota appear to play a role. In a rodent model, PNGR plus hyperoxia causes BPD and PH and increases intestinal Enterobacteriaceae, Gram-negative organisms that stimulate Toll-like receptor 4 (TLR4). We hypothesized that intestinal dysbiosis activates intestinal TLR4 triggering systemic inflammation which impacts lung development.
Methods: Rat pups were assigned to litters of 17 (PNGR) or 10 (normal growth) at birth and exposed to room air or 75% oxygen for 14 days. Half of the pups were treated with the TLR4 inhibitor TAK-242 from birth or beginning at day 3. After 14 days, pulmonary arterial pressure was evaluated by echocardiography and hearts were examined for right ventricular hypertrophy (RVH). Lungs and serum samples were analyzed by western blotting and immunohistochemistry.
Results: Postnatal growth restriction + hyperoxia increased pulmonary arterial pressure and RVH with trends toward increased plasma IL1β and decreased IκBα, the inhibitor of NFκB, in lung tissue. Treatment with the TLR4 inhibitor attenuated PH and inflammation.
Conclusion: Postnatal growth restriction induces an increase in intestinal Enterobacteriaceae leading to PH. Activation of the TLR4 pathway is a promising mechanism by which intestinal dysbiosis impacts the developing lung.
Introduction
Pulmonary hypertension (PH) is an increase in pulmonary vascular resistance resulting in a decrease in pulmonary blood flow and right ventricular hypertrophy (RVH). The incidence of PH among extremely premature infants (birth weight less than 1000 g) is as high as 18% and increases to 25–40% among premature infants with bronchopulmonary dysplasia (BPD), a chronic lung disease (1, 2). In this population, PH is associated with very high morbidity and 50% mortality (3). PH is often not diagnosed until the patient develops severe right ventricular dysfunction. Current screening methods are unreliable, and no early biomarkers of PH exist.
A large cohort study found that 79% of premature infants with gestational age <27 weeks displayed poor growth after birth (post-natal growth restriction, PNGR) (4). PNGR is associated with a sustained elevation in C-reactive protein (5) and increases the risk of PH, BPD and other diseases of prematurity including necrotizing enterocolitis (NEC), an inflammatory disease of the intestines (6–8). In a recent prospective study of PH, among extremely preterm infants with BPD (mean gestational age at birth 26 weeks) evaluated at 36 to 38 weeks corrected gestational age, 13/44 (30%) patients with PH had a history of NEC, while only 8/115 (7%) patients without PH had a history of NEC [adjusted odds ratio 5.5 (95% confidence intervals 1.9, 15.4)] (9), suggesting an association between inflammation in the gut and pulmonary vascular disease. A meta-analysis confirmed a strong association between NEC and PH particularly among infants with BPD (RR 3.4 with 95% confidence intervals 1.1 and 10.2) (10).
Similar to preterm infants, rats are born in the saccular stage of lung development. Neonatal rats exposed to hyperoxia (75–95% O2) for 14 days develop PH, RVH, pulmonary vascular remodeling, and alveolar simplification characteristic of preterm infants with BPD (11). We have shown in a novel rodent model that PNGR, achieved by increasing litter size from 10 to 17 pups, triggers PH and amplifies the adverse effects of hyperoxia at 2 weeks of age (12, 13). This age is roughly equivalent to a human infant at 6–12 months (14), a common time of death for premature infants with PH.
Associations between nutrition, the intestinal microbiota and immune responses in distant sites such as the lung, brain and liver have prompted study of the gut-lung, gut-brain, and gut-liver axes. We recently reported that PNGR, but not hyperoxia, alters the intestinal microbiota in rats at 14 days (15). Partially correcting the dysbiosis with a probiotic strain of Lactobacillus reuteri attenuates PNGR-induced PH (15). We also reported an increase in pro-inflammatory Gram-negative Enterobacteriaceae in the distal small bowel of rat pups exposed to both PNGR and hyperoxia (15). Recognition of lipopolysaccharide (LPS) in the cell wall of Enterobacteriaceae by the host Toll-like receptor (TLR)4 is important in the pathogenesis of NEC, and both inhibition of TLR4 and manipulation of the intestinal microbiota with probiotic organisms prevents this disease (16, 17). TLR4 signaling is also important in lung injury and inflammation (18). In a rodent model of NEC, lung injury is prominent and can be attenuated by deletion of TLR4 from the pulmonary epithelium (19). Furthermore, activation of TLR4 in the NEC model induced expression of chemokine ligand 25 (CC25) resulting in recruitment of Th17 cells to the lungs (20). From these studies we hypothesized that TLR4 –induced inflammation in the intestines is an important mechanism by which PNGR-associated dysbiosis triggers PH in rat pups. The goal of this study was to investigate the role of TLR4 in the developing gut-lung axis.
Materials and Methods
Animals
The animal protocol was approved by the Institutional Animal Care and Use Committee at UC Davis. Timed-pregnant Sprague Dawley dams at E14-E16 were ordered from Charles River Laboratories (Wilmington, MA, United States). Rats were housed in plastic cages with a 12 h dark:light cycle and allowed to feed ad libitum with a standard diet (2018 Teklad from Harlan). After birth, pups were pooled and randomly assigned to litters of 10 pups (normal litter size, N) or 17 pups (restricted litter size, R). Additionally, pups were randomly assigned to cages maintained in room air (A) or exposed to 75% oxygen (O) in a plexiglass chamber (Biospherix, Lacona, NY, United States) continuously, and dams were rotated with the appropriate control or PNGR dam every 24 h. As we have shown previously, the pups tolerate hyperoxia for 14 days without mortality (12). Some pups in each group were injected subcutaneously with the TLR4 inhibitor TAK-242 (Cayman Chemicals, Ann Arbor, MI, United States) (Resatorvid, 3 mg/kg/day from birth) or with vehicle alone (5% ethanol). The dose was chosen based on a previous study in a mouse sepsis model (21). At postnatal day 14, the pups were analyzed by echocardiography, weighed and euthanized for tissue harvest. Pups were euthanized by exposure to CO2 followed by cardiac puncture and exsanguination, and plasma was collected by centrifugation in heparin-treated tubes (Thermo Fisher Scientific) and stored at −80°C. Hearts and lungs, were snap-frozen in liquid nitrogen and stored at −80°C. The intestinal microbiota was not evaluated for this series of experiments, but has previously been reported for this model (15).
Echocardiography
At day 14, echocardiography was performed on pups under light anesthesia with isoflurane using a VisualSonics VIVO 2100 in vivo ultrasound imaging system (VisualSonics, Toronto, ON, Canada) to determine the ratio of the pulmonary acceleration time (PAT) to the total ejection time (ET) a marker of PH as previously described (12).
Measurement of Right Ventricular Hypertrophy (RVH)
Fulton’s index [the weight of the right ventricle (RV) divided by the weight of the left ventricle (LV) + septum] was determined to assess RVH. Additionally, RV and LV + septum weights were normalized to body weight (22).
Plasma IL-1b was quantified using the Rat IL-1 beta Platinum ELISA kit (Thermo Fisher Scientific, Waltham, MA, United States) according to the manufacturer’s instructions.
Western blots were performed on lung tissue as previously described (12). Briefly, lung tissue was suspended in RIPA buffer containing protease and phosphatase inhibitors and sonicated on ice. Protein content was determined by the Bradford method and Western blotting performed using 1:500 dilution of mouse anti-IκB-α antibody (sc-1643, Santa Cruz Biotechnology, Dallas, TX, United States) at 4°C overnight followed by a 60 min incubation with an anti-mouse secondary antibody conjugated to horseradish peroxidase (Santa Cruz). Blots were then probed for β-actin (ab6276, 1:4000, Abcam, Cambridge, MA, United States) for 60 min at room temperature. Chemiluminescence generated by Super Signal West Femto substrate (Thermo Fisher Scientific) was detected and quantified using a Kodak Image Station and software. Signals were normalized to β-actin and expressed as fold change relative to OR animals.
Statistical Analysis
Data are presented as means ± SEM. “N” represents the number of animals in each group. Groups were compared with one-way ANOVA (Stata 12.1, College Station, TX, United States). If the F test was significant, a Scheffe post hoc test was performed. The independent variables were considered significant at p < 0.05.
Results
Our previous study identified an increase in Enterobacteriaceae in the distal small bowel of rat pups exposed to PNGR and hyperoxia (15). To determine if activation of TLR4 by Enterobacteriaceae is involved in the development of PH in these rats, we first determined the efficacy of the TLR4 antagonist TAK-242 to attenuate PH. Increased pulmonary artery pressure results in RVH. As we have shown previously (12), PNGR and hyperoxia alone increase Fulton’s index (the ratio of RV weight to LV + septum weight) with a further increase in Fulton’s index when both are combined (Figure 1A). Daily treatment with TAK-242 attenuated RVH in pups exposed to PNGR with and without hyperoxia, but not in pups exposed to hyperoxia alone (Figure 1A).
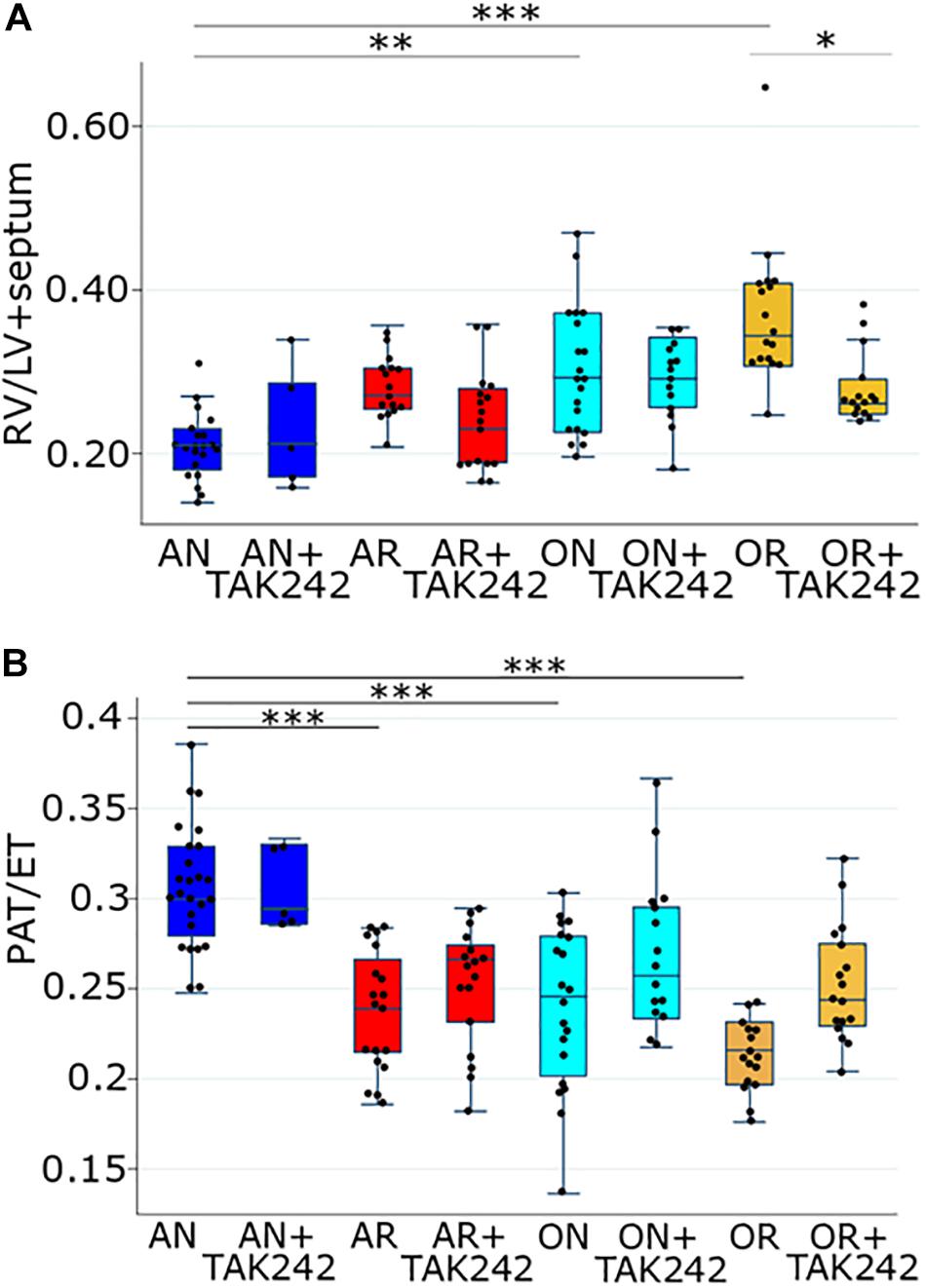
Figure 1. (A) Fulton’s index (right ventricular weight divided by the combined weight of the left ventricle and the intraventricular septum) in the four groups of the PNGR model with and without treatment with the TLR4 inhibitor TAK-242. One way ANOVA p < 0.001, AN vs. AR p = 0.2, AN vs. ON p = 0.01, AN vs. OR p < 0.001, OR vs. OR + TAK242 p = 0.04 (Scheffe). Comparing just the AN vs. AR (not considering the other groups), using a t-test, p < 0.001. Number of animals AN = 21, AN + TAK242 = 5, AR = 16, AR + TAK242 = 17, ON = 20, ON + TAK242 = 14, OR = 17, OR + TAK242 = 15. (B) Ratio of PAT to total ejection time (PAT/ET) in the four groups of the PNGR model with and without treatment with the TLR4 inhibitor TAK-242. One way ANOVA p < 0.001, AN vs. AR p < 0.001, AN vs. ON p < 0.001, AN vs. OR p < 0.001, OR vs. OR + TAK242 p = 0.18 (Scheffe). Comparing just the OR vs. OR + TAK242 (not considering the other groups), using a t-test, p < 0.001. Number of animals AN = 25, AN + TAK242 = 5, AR = 19, ARTAK242 = 17, ON = 20, ON + TAK242 = 15, OR = 16, OR + TAK242 = 17. Asterisks in both A and B are from the Scheffe post hoc test: ∗ p < 0.05, ∗∗ p < 0.01, ∗∗∗ p < 0.001. AN = room air, normal litter size; AR = room air, growth-restricted litter size; ON = hyperoxia, normal litter size; OR = hyperoxia, growth-restricted litter size.
The ratio of the PAT to total ejection time (PAT/ET) detected by echocardiography decreases with increased pulmonary artery pressures. As we have shown previously (12), PAT/ET ratios were significantly decreased in pups exposed to PNGR or hyperoxia alone, and were decreased further in pups exposed to both (Figure 1B). Daily treatment with TAK-242 attenuated the decrease in PAT/ET ratios in pups exposed to hyperoxia with PNGR, but not in pups exposed to PNGR or hyperoxia alone (Figure 1B).
We opted to focus the remaining experiments on the PNGR and hyperoxia group for four reasons: (1) we have previously demonstrated that intestinal dysbiosis is most severe in the PNGR and hyperoxia group with the largest increases in Enterobacteriaceae, (2) this group consistently has the most severe phenotype in our model, (3) this group had a significant attenuation of both RVH and PAT/ET ratio, with TLR4 inhibition and (4) this group most closely reflects extremely premature infants at the highest risk for PH (those with BPD and poor postnatal growth). We next looked at circulating levels of the cytokine IL-1β, a downstream component of TLR4-induced inflammatory responses. A strong trend toward higher plasma levels was seen in pups exposed to PNGR and hyperoxia relative to controls, while daily treatment with TAK-242 trended toward decreased circulating IL-1β (Figure 2).
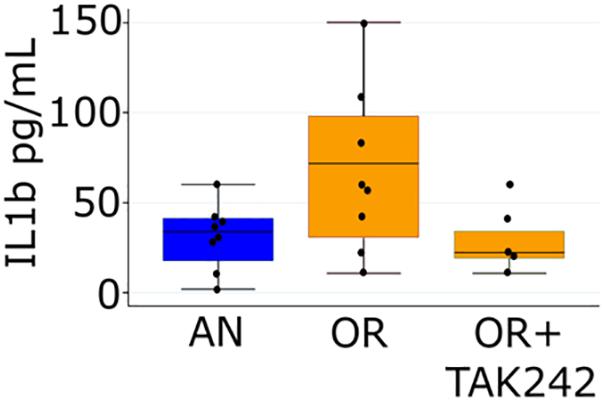
Figure 2. Plasma IL-1β measured by ELISA at day 14 in pups from the air, normal litter size group and the hyperoxia, PNGR group with and without treatment with the TLR4 inhibitor TAK-242. One way ANOVA p = 0.046, AN vs. OR p = 0.08 and OR vs. OR + TAK242 p = 0.12 (Scheffe). Number of animals: AN = 8, OR = 8, OR + TAK242 = 5.
We quantified levels of IκBα protein in lung as a marker of lung inflammation. IκBα is an inhibitory protein of the key pro-inflammatory transcription factor NFκB, and decreases in IκBα indicate an increase in NFκB-mediated inflammation. IκBα protein trended to a decrease in lungs from rats exposed to PNGR and hyperoxia relative to controls, while daily treatment with TAK-242 significantly increased IκBα levels (Figure 3).
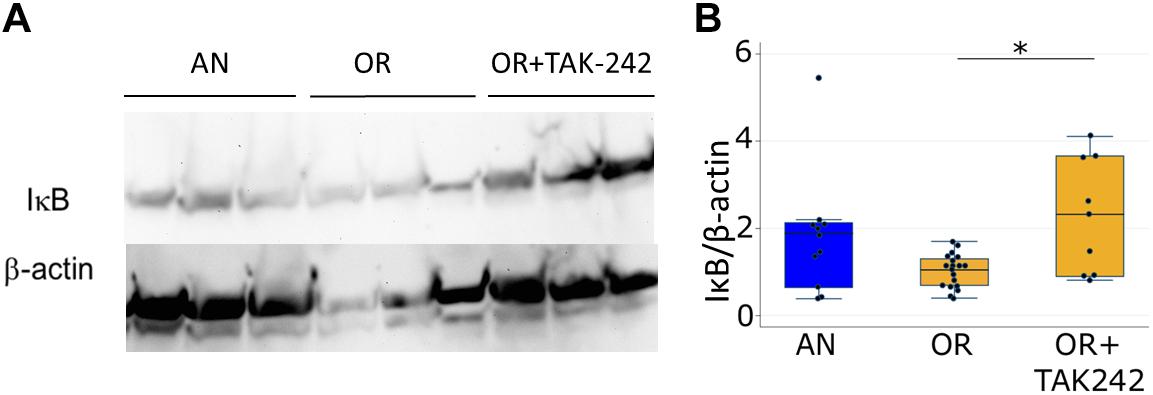
Figure 3. Lung IκBα measured by western blotting at 14 days in pups from the air, normal litter size group and the hyperoxia, PNGR group with and without treatment with the TLR4 inhibitor TAK-242. (A) Representative blot (B). Each band was normalized to β-actin and to OR (each point represents a fold change compared to the mean OR value) One way ANOVA p < 0.01, AN vs. OR p = 0.11 and OR vs. OR + TAK-242 p < 0.02 (∗Scheffe). Number of animals: AN = 11, OR = 19, OR + TAK242 = 9.
In our proposed pathway, intestinal dysbiosis precedes and initiates TLR4 signaling. This raises the possibility that delayed treatment, either to alter the intestinal microbiota or to inhibit TLR4 targeting may be effective in attenuating PH, an advantage in the management of a disease that is not apparent in the premature infant in the first days and weeks of life. To test this hypothesis, we performed additional experiments in which the pups were divided into the four groups on day 1 as usual, but the intervention was not begun until day of life 3. Delaying treatment with TAK-242 until postnatal day 3 still led to significantly increased PAT/ET on day 14 in pups exposed to PNGR and hyperoxia (Figure 4) indicating attenuated PH.
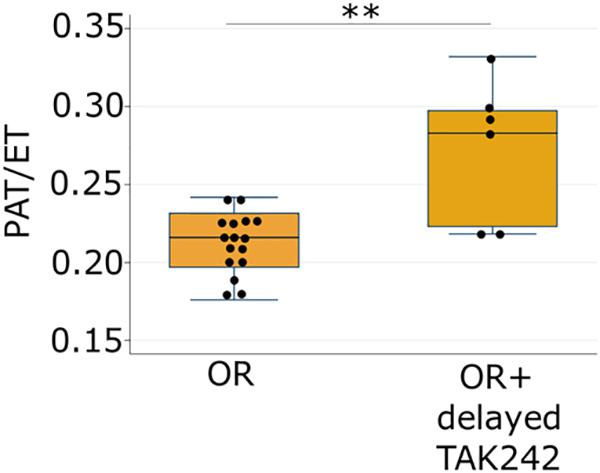
Figure 4. Ratio of pulmonary acceleration time to total ejection time (PAT/ET) in pups exposed to hyperoxia and PNGR model with and without delayed treatment (day 3) with the TLR4 inhibitor TAK-242. ∗∗p < 0.01. Number of animals: OR = 16, OR + delayed TAK242 = 6.
Discussion
Postnatal growth restriction is common with very premature birth and increases the risk of BPD and PH. Retrospective cohort studies have demonstrated associations between decreased caloric intake in the first weeks of life and BPD in very preterm infants (6, 7, 23), and limited studies of aggressive nutrition in the first weeks of life have shown benefit in decreasing BPD in this same population (24). NEC is also a risk factor for PH in very preterm infants both with and without BPD (9), supporting the hypothesis that inflammation in the gut impacts the developing lung vasculature.
Many rodent models of PH involve exposing neonatal pups to hyperoxia, although our model including a component of PNGR more closely approximates clinical conditions in extremely premature infants. As such it is a powerful tool to investigate the underlying mechanisms whereby the most vulnerable extremely low birth weight premature infants (those with poor growth receiving supplemental oxygen) are at greatest risk of developing cardiovascular diseases. From these data and our previously published study (15) we hypothesized that PNGR combined with hyperoxia triggers intestinal dysbiosis including elevated Enterobacteriaceae. Blooms of Enterobacteriaceae have been identified just prior to the onset of necrotizing enterocolitis in premature infants (25) and are a signature of dysbiosis in many disease processes (26). We further hypothesized that the resultant activation of TLR4 by Enterobacteriaceae in the intestines triggers an inflammatory response including elevated circulating IL-1β. This transduces the inflammatory signal to the lungs activating NFκB, leading to PH and RVH (Figure 5). The present study identifying a role for TLR4 signaling in PH induced by PNGR and hyperoxia supports this hypothesis. We previously demonstrated that the probiotic L. reuteri DSM 17938 reverses dysbiosis and attenuates PH and RVH (15), and inhibiting TLR4 signaling likewise attenuates PH and RVH as we show here.
Furthermore, our data suggest that delayed targeting of TLR4 signaling is still effective in attenuating PH. We do not yet know the windows of benefit for either probiotic administration or TLR4 inhibition for successful attenuation of PH in our model, but a potential treatment strategy for preterm infants may involve early probiotic treatment with subsequent targeting of TLR4 signaling in high risk infants.
Studies investigating the gut-lung axis have prompted the hypothesis that intestinal dysbiosis is an important driver of systemic inflammation (27). These associations are particularly important in preterm neonates with immature gut and lung immune responses. Studies of human milk are particularly relevant in this population. Human milk feeding decreases the risk of NEC (28). Meta-analyses suggest a benefit in feeding mother’s own milk (29) and pasteurized donor human milk (30) in the prevention of BPD. In organoids derived from the terminal ileum of mouse pups, human milk exosomes have been shown to attenuate LPS induced activation of TLR4 (31). Human milk oligosaccharides interact with TLR4 on the surface of dendritic cells inducing immune tolerance through increased generation of regulatory T cells and attenuation of LPS-induced expression of IL6 and TNFα (32). It is also possible that TLR4 is important in maintenance of stem cells in the developing gut and lung. In the developing intestinal tract, intestinal stem cells express TLR4 which regulates proliferation and apoptosis (33). In a lung injury model, deletion of TLR4 impairs the renewal capacity of lung stem cells (34). Conversely, in a model of neonatal PH triggered by intra-amniotic injection of PBS, human mesenchymal stem cells decrease expression of TLR4, NFκB, and TNFα in the heart and attenuate PH (35).
Toll-like receptors are important in recognition of pathogen-associated molecular patterns and triggering of innate immune responses in both the gut and the lung, as demonstrated in the studies of TLR4 in NEC-associated lung injury noted in the introduction. TLR4 activates IL-1β transcription via NFκB (36). The role of TLR4 in regulating pulmonary vasculogenesis has also been explored. Adult TLR4-deficient mice do not develop PH when exposed to hypoxia (37). Stimulation of TLR4 on platelets leads to platelet activation and aggregation exacerbating PH (and as a result selective knockout of TLR4 on platelets is protective) (38). The increase in Enterobacteriaceae in our PNGR model suggests a potential role for TLR4 in the intestine and/or the lung in the resultant PH. Probiotic microbes impact host immune responses including downregulation of TLR4 (39), chemokines and cytokines (40–46), suppression of T-helper 2 responses (47) decrease in intestinal permeability (48–51), alteration of intestinal motility (52, 53), and production of short chain fatty acids (54, 55).
Lung inflammation is involved in the development of PH in humans and animal models (56). We believe the current study is the first to demonstrate a potential role for an inflammatory response initiating in the intestines in PNGR/hyperoxia-induced PH. Our studies do not rule out the possibility of a TLR4 response induced in the lung following simultaneous exposure to PNGR and hyperoxia; direct measurement of TLR4 in both the gut and lung would be valuable to address this possibility. Activation of lung TLR4 using aerosolized LPS results in elevated IL-1β in bronchiolar lavage fluid in mice (57), while treatment with the anti-inflammatory molecule dioscin suppresses various pro-inflammatory molecules including TLR4, IL-1β and NFκB in the lungs of rats injected with LPS (58). Our current study indicated that subcutaneous TAK-242 is effective at attenuating PH in our model. Further studies comparing the efficacy of TAK-242 delivered intranasally or via gavage may identify where TLR4 is activated in rats exposed to PNGR and hyperoxia. Measurement of IL1β in both intestinal and lung tissue in this model would be of value in future studies.
In the current study we demonstrate that IκBα levels were decreased in the lungs of rats exposed to PNGR and hyperoxia, while TAK-242 prevented this decrease. Increased NFκB activity is evident in explanted lungs of patients with idiopathic PH (59), and an NFκB decoy delivery into lungs prevents monocrotaline-induced NFκB activity and PH in rats (59) suggesting that elevated lung NFκB activity plays a central role in the pathogenesis of PH. We have previously shown a decrease in IκB in the lungs and pulmonary arteries in a lamb model of persistent PH of the newborn suggesting a potential role for NFκB-target genes in pulmonary vascular remodeling (60). Direct measurement of NFκB in lung tissue in this model would be of value.
In summary, we show that TLR4 inhibition attenuated PH, RVH, and decreased lung IκBα activity in rat pups exposed to PNGR and hyperoxia with a trend toward decreasing elevated circulating IL-1β. Further elucidation of the underlying mechanisms may identify crucial spatial (intestinal and pulmonary) and temporal targets to improve clinical outcomes of low birth weight preterm infants at risk of developing PH.
Data Availability Statement
The datasets generated for this study are available on request to the corresponding author.
Ethics Statement
The animal study was reviewed and approved by the UC Davis Institutional Animal Care and Use Committee.
Author Contributions
SW contributed to study design and data analysis and wrote initial draft. KG, KH, SM, and AH performed the analyses. CW and PT performed the animal experiments. RS, SL, NC, and MU contributed to study design and data analysis. All authors approved the final manuscript.
Funding
This work was supported by the National Institutes of Health: R21 HD096241-01 to MU, the Children’s Miracle Network to SW, National Institutes of Health R01 HL HL085727, R01 HL085844, R01 HL137228, S10 OD010389 shared equipment grant, VA Merit Review Grant I01 BX000576 and I01 CX001490 to NC, and Postdoctoral Fellowships from NIH T32 Training Grant in Basic & Translational Cardiovascular Science (T32 HL86350) and NIH F32 HL149288 to PT.
Conflict of Interest
The authors declare that the research was conducted in the absence of any commercial or financial relationships that could be construed as a potential conflict of interest.
References
1. Berkelhamer SK, Mestan KK, Steinhorn RH. Pulmonary hypertension in bronchopulmonary dysplasia. Semin Perinatol. (2013) 37:124–31. doi: 10.1053/j.semperi.2013.01.009
2. Check J, Gotteiner N, Liu X, Su E, Porta N, Steinhorn R, et al. Fetal growth restriction and pulmonary hypertension in premature infants with bronchopulmonary dysplasia. J Perinatol. (2013) 33:553–7. doi: 10.1038/jp.2012.164
3. Khemani E, McElhinney DB, Rhein L, Andrade O, Lacro RV, Thomas KC, et al. Pulmonary artery hypertension in formerly premature infants with bronchopulmonary dysplasia: clinical features and outcomes in the surfactant era. Pediatrics. (2007) 120:1260–9.
4. Natarajan G, Johnson YR, Brozanski B, Farrow KN, Zaniletti I, Padula MA, et al. Postnatal weight gain in preterm infants with severe bronchopulmonary dysplasia. Am J Perinatol. (2014) 31:223–30. doi: 10.1055/s-0033-1345264
5. Cuestas E, Aguilera B, Cerutti M, Rizzotti A. Sustained neonatal inflammation is associated with poor growth in infants born very preterm during the first year of life. J Pediatr. (2019) 205:91–7. doi: 10.1016/j.jpeds.2018.09.032
6. Uberos J, Lardon-Fernandez M, Machado-Casas I, Molina-Oya M, Narbona-Lopez E. Nutrition in extremely low birth weight infants: impact on bronchopulmonary dysplasia. Minerva Pediatr. (2016) 68:419–26.
7. Malikiwi AI, Lee YM, Davies-Tuck M, Wong FY. Postnatal nutritional deficit is an independent predictor of bronchopulmonary dysplasia among extremely premature infants born at or less than 28 weeks gestation. Early Hum Dev. (2019) 131:29–35. doi: 10.1016/j.earlhumdev.2019.02.005
8. Klevebro S, Lundgren P, Hammar U, Smith LE, Bottai M, Domellof M, et al. Cohort study of growth patterns by gestational age in preterm infants developing morbidity. BMJ Open. (2016) 6:e012872. doi: 10.1136/bmjopen-2016-012872
9. Weismann CG, Asnes JD, Bazzy-Asaad A, Tolomeo C, Ehrenkranz RA, Bizzarro MJ. Pulmonary hypertension in preterm infants: results of a prospective screening program. J Perinatol. (2017) 37:572–7. doi: 10.1038/jp.2016.255
10. Arjaans S, Zwart EAH, Ploegstra MJ, Bos AF, Kooi EMW, Hillege HL, et al. Identification of gaps in the current knowledge on pulmonary hypertension in extremely preterm infants: a systematic review meta-analysis. Paediatr Perinat Epidemiol. (2018) 32:258–67. doi: 10.1111/ppe.12444
11. Koppel R, Han RN, Cox D, Tanswell AK, Rabinovitch M. Alpha 1-antitrypsin protects neonatal rats from pulmonary vascular and parenchymal effects of oxygen toxicity. Pediatr Res. (1994) 36:763–70.
12. Wedgwood S, Warford C, Agvateesiri SC, Thai P, Berkelhamer SK, Perez M, et al. Postnatal growth restriction augments oxygen-induced pulmonary hypertension in a neonatal rat model of bronchopulmonary dysplasia. Pediatr Res. (2016) 80:894–902. doi: 10.1038/pr.2016.164
13. La Frano MR, Fahrmann JF, Grapov D, Fiehn O, Pedersen TL, Newman JW, et al. Metabolic perturbations of postnatal growth restriction and hyperoxia-induced pulmonary hypertension in a bronchopulmonary dysplasia model. Metabolomics. (2017) 13:32.
15. Wedgwood S, Warford C, Agvatisiri SR, Thai PN, Chiamvimonvat N, Kalanetra KM, et al. The developing gut-lung axis: postnatal growth restriction, intestinal dysbiosis, and pulmonary hypertension in a rodent model. Pediatr Res. (2019) doi: 10.1038/s41390-019-0578-2 [Epub ahead of print].
16. Vongbhavit K, Underwood MA. Prevention of necrotizing enterocolitis through manipulation of the intestinal microbiota of the premature infant. Clin Ther. (2016) 38:716–32.
17. Hackam DJ, Sodhi CP. Toll-like receptor-mediated intestinal inflammatory imbalance in the pathogenesis of necrotizing enterocolitis. Cell Mol Gastroenterol Hepatol. (2018) 6:229–38.e1. doi: 10.1016/j.jcmgh.2018.04.001
18. Hu R, Xu H, Jiang H, Zhang Y, Sun Y. The role of TLR4 in the pathogenesis of indirect acute lung injury. Front Biosci. (2013) 18:1244–55. doi: 10.2741/4176
19. Jia H, Sodhi CP, Yamaguchi Y, Lu P, Martin LY, Good M, et al. Pulmonary epithelial TLR4 activation leads to lung injury in neonatal necrotizing enterocolitis. J Immunol. (2016) 197:859–71. doi: 10.4049/jimmunol.1600618
20. Jia H, Sodhi CP, Yamaguchi Y, Lu P, Ladd MR, Werts A, et al. Toll like receptor 4 mediated lymphocyte imbalance induces nec-induced lung injury. Shock (Augusta Ga). (2019) 52:215–23. doi: 10.1097/SHK.0000000000001255
21. Takashima K, Matsunaga N, Yoshimatsu M, Hazeki K, Kaisho T, Uekata M, et al. Analysis of binding site for the novel small-molecule TLR4 signal transduction inhibitor TAK-242 and its therapeutic effect on mouse sepsis model. Br J Pharmacol. (2009) 157:1250–62. doi: 10.1111/j.1476-5381.2009.00297.x
22. Ladha F, Bonnet S, Eaton F, Hashimoto K, Korbutt G, Thebaud B. Sildenafil improves alveolar growth and pulmonary hypertension in hyperoxia-induced lung injury. Am J Respir Crit Care Med. (2005) 172:750–6.
23. Wemhoner A, Ortner D, Tschirch E, Strasak A, Rudiger M. Nutrition of preterm infants in relation to bronchopulmonary dysplasia. BMC Pulm Med. (2011) 11:7. doi: 10.1186/1471-2466-11-7
24. Panagiotounakou P, Sokou R, Gounari E, Konstantinidi A, Antonogeorgos G, Grivea IN, et al. Very preterm neonates receiving “aggressive” nutrition and early nCPAP had similar long-term respiratory outcomes as term neonates. Pediatr Res. (2019) 86:742–8. doi: 10.1038/s41390-019-0514-5
25. Mai V, Young CM, Ukhanova M, Wang X, Sun Y, Casella G, et al. Fecal microbiota in premature infants prior to necrotizing enterocolitis. PLoS One. (2011) 6:e20647. doi: 10.1371/journal.pone.0020647
26. Litvak Y, Byndloss MX, Tsolis RM, Baumler AJ. Dysbiotic proteobacteria expansion: a microbial signature of epithelial dysfunction. Curr Opin Microbiol. (2017) 39:1–6. doi: 10.1016/j.mib.2017.07.003
27. Budden KF, Gellatly SL, Wood DL, Cooper MA, Morrison M, Hugenholtz P, et al. Emerging pathogenic links between microbiota and the gut-lung axis. Nat Rev. (2017) 15:55–63. doi: 10.1038/nrmicro.2016.142
28. Miller J, Tonkin E, Damarell RA, McPhee AJ, Suganuma M, Suganuma H, et al. A systematic review and meta-analysis of human milk feeding and morbidity in very low birth weight infants. Nutrients. (2018) 10:707. doi: 10.3390/nu10060707
29. Villamor-Martinez E, Pierro M, Cavallaro G, Mosca F, Villamor E. Mother’s own milk and bronchopulmonary dysplasia: a systematic review and meta-analysis. Front Pediatr. (2019) 7:224. doi: 10.3389/fped.2019.00224
30. Villamor-Martinez E, Pierro M, Cavallaro G, Mosca F, Kramer BW, Villamor E. Donor human milk protects against bronchopulmonary dysplasia: a systematic review and meta-analysis. Nutrients (2018) 10:238. doi: 10.3390/nu10020238
31. Gao R, Zhang R, Qian T, Peng X, He W, Zheng S, et al. A comparison of exosomes derived from different periods breast milk on protecting against intestinal organoid injury. Pediatr Surg Int. (2019) 35:1363–8. doi: 10.1007/s00383-019-04562-6
32. Xiao L, van De Worp WR, Stassen R, van Maastrigt C, Kettelarij N, Stahl B, et al. Human milk oligosaccharides promote immune tolerance via direct interactions with human dendritic cells. Eur J Immunol. (2019) 49:1001–14. doi: 10.1002/eji.201847971
33. Neal MD, Sodhi CP, Jia H, Dyer M, Egan CE, Yazji I, et al. Toll-like receptor 4 is expressed on intestinal stem cells and regulates their proliferation and apoptosis via the p53 up-regulated modulator of apoptosis. J Biol Chem. (2012) 287:37296–308. doi: 10.1074/jbc.M112.375881
34. Liang J, Zhang Y, Xie T, Liu N, Chen H, Geng Y, et al. Hyaluronan and TLR4 promote surfactant-protein-C-positive alveolar progenitor cell renewal and prevent severe pulmonary fibrosis in mice. Nat Med. (2016) 22:1285–93. doi: 10.1038/nm.4192
35. Chou HC, Lin W, Chen CM. Human mesenchymal stem cells attenuate pulmonary hypertension induced by prenatal lipopolysaccharide treatment in rats. Clin Exp Pharmacol Physiol. (2016) 43:906–14. doi: 10.1111/1440-1681.12604
37. Young KC, Hussein SM, Dadiz R, deMello D, Devia C, Hehre D, et al. Toll-like receptor 4-deficient mice are resistant to chronic hypoxia-induced pulmonary hypertension. Exp Lung Res. (2010) 36:111–9. doi: 10.3109/01902140903171610
38. Bauer EM, Chanthaphavong RS, Sodhi CP, Hackam DJ, Billiar TR, Bauer PM. Genetic deletion of toll-like receptor 4 on platelets attenuates experimental pulmonary hypertension. Circ Res. (2014) 114:1596–600. doi: 10.1161/CIRCRESAHA.114.303662
39. Yao P, Tan F, Gao H, Wang L, Yang T, Cheng Y. Effects of probiotics on tolllike receptor expression in ulcerative colitis rats induced by 2,4,6trinitrobenzene sulfonic acid. Mol Med Rep. (2017) 15:1973–80. doi: 10.3892/mmr.2017.6226
40. Izumi H, Minegishi M, Sato Y, Shimizu T, Sekine K, Takase M. Bifidobacterium breve alters immune function and ameliorates DSS-induced inflammation in weanling rats. Pediatr Res. (2015) 78:407–16. doi: 10.1038/pr.2015.115
41. Philippe D, Favre L, Foata F, Adolfsson O, Perruisseau-Carrier G, Vidal K, et al. Bifidobacterium lactis attenuates onset of inflammation in a murine model of colitis. World J Gastroenterol. (2011) 17:459–69. doi: 10.3748/wjg.v17.i4.459
42. Lin YP, Thibodeaux CH, Pena JA, Ferry GD, Versalovic J. Probiotic Lactobacillus reuteri suppress proinflammatory cytokines via c-Jun. Inflamm Bowel Dis. (2008) 14:1068–83. doi: 10.1002/ibd.20448
43. Liu Y, Fatheree NY, Mangalat N, Rhoads JM. Lactobacillus reuteri strains reduce incidence and severity of experimental necrotizing enterocolitis via modulation of TLR4 and NF-kappaB signaling in the intestine. Am J Physiol Gastrointest liver Physiol. (2012) 302:G608–17. doi: 10.1152/ajpgi.00266.2011
44. Mirpuri J, Sotnikov I, Myers L, Denning TL, Yarovinsky F, Parkos CA, et al. Lactobacillus rhamnosus (LGG) regulates IL-10 signaling in the developing murine colon through upregulation of the IL-10R2 receptor subunit. PLoS One. (2012) 7:e51955. doi: 10.1371/journal.pone.0051955
45. Borthakur A, Bhattacharyya S, Kumar A, Anbazhagan AN, Tobacman JK, Dudeja PK. Lactobacillus acidophilus alleviates platelet-activating factor-induced inflammatory responses in human intestinal epithelial cells. PLoS One. (2013) 8:e75664. doi: 10.1371/journal.pone.0075664
46. Liu Y, Fatheree NY, Mangalat N, Rhoads JM. Human-derived probiotic Lactobacillus reuteri strains differentially reduce intestinal inflammation. Am J Physiol Gastrointest Physiol. (2010) 299:G1087–96. doi: 10.1152/ajpgi.00124.2010
47. Inoue Y, Iwabuchi N, Xiao JZ, Yaeshima T, Iwatsuki K. Suppressive effects of Bifidobacterium breve strain M-16V on T-helper type 2 immune responses in a murine model. Biol Pharm Bull. (2009) 32:760–3.
48. Putaala H, Salusjarvi T, Nordstrom M, Saarinen M, Ouwehand AC, Bech Hansen E, et al. Effect of four probiotic strains and Escherichia coli O157:H7 on tight junction integrity and cyclo-oxygenase expression. Res Microbiol. (2008) 159:692–8. doi: 10.1016/j.resmic.2008.08.002
49. Patel RM, Myers LS, Kurundkar AR, Maheshwari A, Nusrat A, Lin PW. Probiotic bacteria induce maturation of intestinal claudin 3 expression and barrier function. Am J Pathol. (2012) 180:626–35. doi: 10.1016/j.ajpath.2011.10.025
50. Lin PW, Nasr TR, Berardinelli AJ, Kumar A, Neish AS. The probiotic Lactobacillus GG may augment intestinal host defense by regulating apoptosis and promoting cytoprotective responses in the developing murine gut. Pediatr Res. (2008) 64:511–6. doi: 10.1203/PDR.0b013e3181827c0f
51. Yan F, Polk DB. Characterization of a probiotic-derived soluble protein which reveals a mechanism of preventive and treatment effects of probiotics on intestinal inflammatory diseases. Gut Microbes. (2012) 3:25–8. doi: 10.4161/gmic.19245
52. Kunze WA, Mao YK, Wang B, Huizinga JD, Ma X, Forsythe P, et al. Lactobacillus reuteri enhances excitability of colonic AH neurons by inhibiting calcium-dependent potassium channel opening. J Cell Mol Med. (2009) 13:2261–70. doi: 10.1111/j.1582-4934.2009.00686.x
53. Wu RY, Pasyk M, Wang B, Forsythe P, Bienenstock J, Mao YK, et al. Spatiotemporal maps reveal regional differences in the effects on gut motility for Lactobacillus reuteri and rhamnosus strains. Neurogastroenterol Motil. (2013) 25:e205–14. doi: 10.1111/nmo.12072
54. Arboleya S, Salazar N, Solis G, Fernandez N, Hernandez-Barranco AM, Cuesta I, et al. Assessment of intestinal microbiota modulation ability of Bifidobacterium strains in vitro fecal batch cultures from preterm neonates. Anaerobe. (2013) 19:9–16. doi: 10.1016/j.anaerobe.2012.11.001
55. Kumar A, Alrefai WA, Borthakur A, Dudeja PK. Lactobacillus acidophilus counteracts enteropathogenic E. coli-induced inhibition of butyrate uptake in intestinal epithelial cells. Am J Physiol Gastrointest Liver Physiol. (2015) 309:G602–7. doi: 10.1152/ajpgi.00186.2015
56. Kumar R, Graham B. How does inflammation contribute to pulmonary hypertension? Eur Respir J. (2018) 51:1702403. doi: 10.1183/13993003.02403-2017
57. Eltom S, Belvisi MG, Yew-Booth L, Dekkak B, Maher SA, Dubuis ED, et al. TLR4 activation induces IL-1beta release via an IPAF dependent but caspase 1/11/8 independent pathway in the lung. Respir Res. (2014) 15:87. doi: 10.1186/s12931-014-0087-0
58. Zeng H, Yang L, Zhang X, Chen Y, Cai J. Dioscin prevents LPS-induced acute lung injury through inhibiting the TLR4/MyD88 signaling pathway via upregulation of HSP70. Mol Med Rep. (2018) 17:6752–8. doi: 10.3892/mmr.2018.8667
59. Kimura S, Egashira K, Chen L, Nakano K, Iwata E, Miyagawa M, et al. Nanoparticle-mediated delivery of nuclear factor kappaB decoy into lungs ameliorates monocrotaline-induced pulmonary arterial hypertension. Hypertension. (2009) 53:877–83. doi: 10.1161/HYPERTENSIONAHA.108.121418
Keywords: intestinal dysbiosis, TLR4, pulmonary hypertension, bronchopulmonary dysplasia, premature infant, Enterobacteriaceae
Citation: Wedgwood S, Gerard K, Halloran K, Hanhauser A, Monacelli S, Warford C, Thai PN, Chiamvimonvat N, Lakshminrusimha S, Steinhorn RH and Underwood MA (2020) Intestinal Dysbiosis and the Developing Lung: The Role of Toll-Like Receptor 4 in the Gut-Lung Axis. Front. Immunol. 11:357. doi: 10.3389/fimmu.2020.00357
Received: 16 November 2019; Accepted: 14 February 2020;
Published: 05 March 2020.
Edited by:
Duc Ninh Nguyen, University of Copenhagen, DenmarkReviewed by:
Chung-Ming Chen, Taipei Medical University, TaiwanClaudia Nold, Hudson Institute of Medical Research, Australia
David Hackam, Johns Hopkins Medicine, United States
Copyright © 2020 Wedgwood, Gerard, Halloran, Hanhauser, Monacelli, Warford, Thai, Chiamvimonvat, Lakshminrusimha, Steinhorn and Underwood. This is an open-access article distributed under the terms of the Creative Commons Attribution License (CC BY). The use, distribution or reproduction in other forums is permitted, provided the original author(s) and the copyright owner(s) are credited and that the original publication in this journal is cited, in accordance with accepted academic practice. No use, distribution or reproduction is permitted which does not comply with these terms.
*Correspondence: Mark A. Underwood, bXVuZGVyd29vZEB1Y2RhdmlzLmVkdQ==