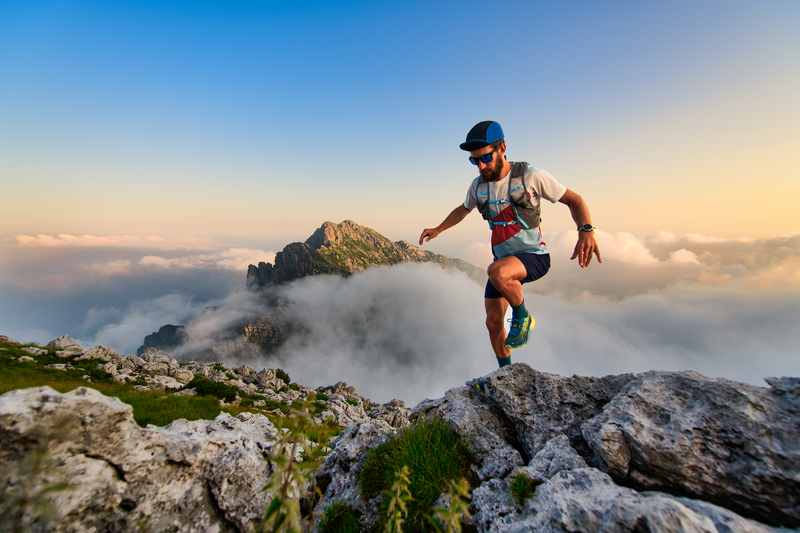
95% of researchers rate our articles as excellent or good
Learn more about the work of our research integrity team to safeguard the quality of each article we publish.
Find out more
ORIGINAL RESEARCH article
Front. Immunol. , 08 January 2020
Sec. T Cell Biology
Volume 10 - 2019 | https://doi.org/10.3389/fimmu.2019.03007
RORγt-expressing Tregs form a specialized subset of intestinal CD4+ Foxp3+ cells which is essential to maintain gut homeostasis and tolerance to commensal microbiota. Recently, c-Maf emerged as a critical factor in the regulation of RORγt expression in Tregs. However, aside from c-Maf signaling, the signaling pathways involved in the differentiation of RORγt+ Tregs and their possible interplay with c-Maf in this process are largely unknown. We show that RORγt+ Treg development is controled by positive as well as negative signals. Along with c-Maf signaling, signals derived from a complex microbiota, as well as IL-6/STAT3- and TGF-β-derived signals act in favor of RORγt+ Treg development. Ectopic expression of c-Maf did not rescue RORγt expression in STAT3-deficient Tregs, indicating the presence of additional effectors downstream of STAT3. Moreover, we show that an inflammatory IFN-γ/STAT1 signaling pathway acts as a negative regulator of RORγt+ Treg differentiation in a c-Maf independent fashion. These data thus argue for a complex integrative signaling network that finely tunes RORγt expression in Tregs. The finding that type 1 inflammation impedes RORγt+ Treg development even in the presence of an active IL-6/STAT3 pathway further suggests a dominant negative effect of STAT1 over STAT3 in this process.
CD4 T cells expressing transcription factor forkhead box P3 (Foxp3) constitute a regulatory lineage of T cells (Treg) which maintains immune tolerance against self-antigens and prevents tissue destruction consequent to excessive immune responses. Tregs can be generated in the thymus from developing CD4+ thymocytes (tTregs) or can result from the differentiation of mature T cells in the periphery (pTregs) (1–3). Recent findings indicate that, similarly to conventional helper T cells, Tregs are phenotypically and functionally heterogeneous. Distinct Treg populations adopt specialized phenotypes through the co-expression of Foxp3 and lineage-defining transcription factors such as PPARγ, BCL6, or RORγt in response to tissue- or inflammatory-driven signals (3).
In particular, RORγt+ Tregs are found in the intestinal tissue of naïve mice. Signals deriving from a complex microbiota as well as STAT3 signaling are necessary to their presence in the intestinal compartment (4, 5). This subset of Tregs has been shown to protect efficiently from intestinal immunopathology in different colitis models (4–7) and to mediate immunological tolerance to the gut pathobiont Helicobacter hepaticus (8). A recent study showed that thymic-derived Tregs also expressed RORγt in lymph nodes following immunization and were able to protect mice from Th17 cell-mediated CNS inflammation (9). Although RORγt expression can be acquired by ex-Tregs during pathogenic Th17 conversion (10), RORγt-expressing Tregs mostly represent a Treg lineage which participates in the immunological tolerance of barrier tissues and protects from autoimmunity (4–9). RORγt+ Tregs can also develop in the tumor microenvironment where they hinder anti-tumor immunity, thus revealing a double-edged function of this Treg subset in immune homeostasis (11). However, despite their importance in physiological and pathological immune responses, the factors driving RORγt+ Treg differentiation are still incompletely defined.
Recent studies reported that transcription factor c-Maf promotes the differentiation of intestinal RORγt+ Tregs (8, 12, 13). Coincidentally, transcriptomic studies conducted on Tregs originating from different tissues revealed a strong enrichment for c-Maf in the intestinal compartment (4). Transcription factor c-Maf, a member of the AP-1 family of basic region/leucine zipper transcription factors, is expressed by distinct CD4+ T cell subsets, including Th17, Th2, Tfh, and Tr1 cells, and is thought to regulate the expression of IL-10, IL-4, and IL-21 through the transactivation of their promoters, downstream of Batf, ICOS, and STAT3 signaling (14–18). Thus, and similarly to what has been previously described for Th17 cells (19), expression of RORγt in Tregs is c-Maf-dependent. However, unlike RORγt expression, which is restricted to gut-associated Tregs in naïve mice, c-Maf is expressed by a wider proportion of Tregs found in distinct organs. Of note, high levels of c-Maf are found in a subset of splenic CD44+ CD62L− effector Tregs driven by ICOS signaling (12). The partial overlapping of RORγt and c-Maf expression along with the presence of a substantial population of c-Maf+ RORγt− Tregs in lymphoid organs therefore suggests that c-Maf is not sufficient per se to drive RORγt+ Treg cell differentiation and supports the existence of complementary signaling pathways.
Herein, extensive analysis of the lymphoid organs and tissues of genetically invalidated mice or mice harboring an altered microbiota revealed that, well beyond the c-Maf/RORγt interplay, multiple signaling pathways cooperate to exert a tight control over RORγt expression in Tregs.
C57BL/6 mice were purchased from Envigo (Horst, The Netherlands). c-Maf-flox mice (C. Birchmeier, Max Delbrück Center for Molecular Medicine, Berlin, Germany) were crossed with CD4-CRE mice (G. Van Loo, Ghent University, Ghent, Belgium) or FOXP3-CRE-YFP mice which were developed by Rudensky (20) and kindly provided by A. Liston (KU Leuven, Leuven, Belgium). IL-6−/− mice were obtained from The Jackson Laboratory (Bar Harbor, ME, USA). STAT3-flox mice were kindly provided by S. Akira (Osaka University, Osaka, Japan); STAT1−/− mice by D.E. Levy (New York University School of Medicine, NYC, USA). Germ-free mice were obtained from the Ghent Germfree and Gnotobiotic mouse facility (Ghent University, Ghent, Belgium) and were compared to SPF control mice. c-Maf-flox, CD4-CRE, FOXP3-CRE-YFP, IL-6−/−, STAT3-flox, STAT1−/− and germ-free mice were bred on a C57BL/6 background. Tgfbr2-flox mice (21) on a NOD background crossed with Foxp3-Cre mice (JAX 008694) were kindly provided by Q. Tang (University of California San Francisco, SF, USA) and were housed and bred at the UCSF Animal Barrier Facility.
All mice were used between 6 and 12 weeks of age. The experiments were carried out in compliance with the relevant laws and institutional guidelines and were approved by the Université Libre de Bruxelles Institutional Animal Care and Use Committee (protocol number CEBEA-4).
The following monoclonal antibodies were purchased from eBioscience: CD278 (ICOS)-biotin, CD304 (Nrp1)-PerCP eF710, c-Maf-EF660, Foxp3-FITC, RORγt-PE, T-bet-PE; or from BD Biosciences: CD25-BB515, CD44-PECy7, CD4-A700, CD4-PB, CD62L-A700, GATA3-PE, RORγt-PECF594, STAT1 (pY701)-A488, STAT3 (pY705)-A647, streptavidin-PECy7.
Live/dead fixable near-IR stain (ThermoFisher) was used to exclude dead cells. For transcription factor staining, cells were stained for surface markers, followed by fixation and permeabilization before nuclear factor staining according to the manufacturer's protocol (FOXP3 staining buffer set from eBioscience). For phosphorylation staining, cells were fixed with formaldehyde and permeabilized with methanol before staining. Flow cytometric analysis was performed on a Canto II (BD Biosciences) or CytoFLEX (Beckman Coulter) and analyzed using FlowJo software (Tree Star).
After removal of Peyer's patches and mesenteric fat, intestinal tissues were washed in HBSS 3% FCS and PBS, cut in small sections and incubated in HBSS 3% FCS containing 2.5 mM EDTA and 72.5 μg/mL DTT for 30 min at 37°C with agitation to remove epithelial cells, and then minced and dissociated in RPMI containing liberase (20 μg/ml, Roche) and DNase (400 μg/ml, Roche) at 37°C for 30 min (small intestine) or 45 min (colon). Leukocytes were collected after a 30% Percoll gradient (GE Healthcare). Lymph nodes, thymus and spleens were mechanically disrupted in culture medium.
After anesthesia, mice were perfused with PBS. Liver and lung samples were digested with collagenase (200 U, Worthington) and DNase I (40 μg/mL, Roche) at 37°C and mechanically disrupted. Leukocytes were collected at the interphase of a 40/70% Percoll gradient.
Wide spectrum antibiotics (ampicillin 1 g/L and neomycin 1 g/L, Sigma-Aldrich; vancomycin 0.5 g/L and metronidazole 1 g/L, Duchefa) were added to the sweetened drinking water of mice treated with antibiotics for 3-4 weeks. Control mice were given sweetened drinking water in parallel.
ME-49 type II T. gondii was kindly provided by Dr. De Craeye (Institut Scientifique de Santé Publique, Belgium) and was used for the production of tissue cysts in C57BL/6 mice, which were inoculated 1-3 months previously with three cysts by gavage. Animals were killed, and the brains were removed. Tissue cysts were counted and mice were infected with 10 cysts by intragastric gavage. Mice were sacrificed at day 8 after infection.
Naive CD4+ T cells were purified from spleen of mice of indicated genotypes. CD4+ T cells were positively selected from organ cell suspensions by magnetic-activated cell sorting using CD4 beads (MACS, Miltenyi) according to the product protocol, and then isolated as CD4+ CD44loCD62LhiCD25− or CD4+ CD44lo CD62Lhi YFP− by FACS. T cells were cultured at 37°C in RPMI supplemented with 5% heat-inactivated FBS (Sigma-Aldrich), 1% non-essential amino acids (Invitrogen), 1 mM sodium pyruvate (Invitrogen), 2 mM L-glutamin (Invitrogen), 500 U/mL penicillin/500 μg/ml streptomycin (Invitrogen), and 50 μM β-mercaptoethanol (Sigma-Aldrich).
To generate iTreg cells, cells were cultured 72 h in 24 or 96 well plates coated with 5 μg/mL anti-CD3 (BioXcell, 145-2c11) at 37°C for 72 h. The culture was supplemented with anti-CD28 (1 μg/mL, BioXcell, 37.51) and TGF-β alone (3 ng/ml, eBioscience), TGF-β and IL-2 (10 ng/mL, Peprotech), or TGF-β, IL-2 and IL-6 (10 ng/mL, eBioscience) for optimal iTreg cell polarization. Acetate (C2, 10 mM), propionate (C3, 0.5 mM), butyrate (C4, 0.125 mM), all from Sigma-Aldrich, and IFN-γ (10 and 100 ng/mL, Peprotech) were also used and added to the culture for the whole duration of the experiment.
Platinum-E retroviral packaging cells (T. Kitamura, University of Tokyo, Tokyo, Japan) were transfected with a c-Maf encoding retroviral plasmid (pMIEG-c-Maf-IRES-GFP) or a control retroviral plasmid (pMIEG-IRES-GFP) to produce retrovirus-containing supernatants. 24 h after activation, naïve CD4 T cells polarized in presence of TGF-β, IL-2, and IL-6, as described above, were infected during a 90-min centrifugation with 1 mL retrovirus-containing supernatant and polybrene. Forty-eight hours later, infected cells were FACS-sorted based on GFP expression and were stimulated with anti-CD3 for 24 h (5 μg/mL, coated) before flow cytometry staining.
Naïve T cells with the phenotype CD4+ CD44lo CD62Lhi YFP− were isolated from the spleen of FOXP3-CRE-YFP mice by FACS after positive enrichment for CD4+ cells using MACS LS columns (Miltenyi) and labeled with carboxyfluorescein diacetate succinimidyl ester (CFSE, ThermoFisher). Treg cells with the phenotype CD4+ FOXP3-CRE-YFP+ were isolated from the mesenteric lymph nodes of Foxp3-CRE-YFP or c-MafTregKO mice by FACS. Splenocytes from wild-type B6 mice were depleted in T cells (anti-CD90.2 beads) using MACS LS columns (Miltenyi). 4 × 104 CFSE-labeled naive T cells were cultured for 72 h with APCs (1 × 105) and soluble anti-CD3 (0.5 μg/mL) in the presence or absence of various numbers of Treg cells as indicated.
RNA was extracted using the TRIzol method (Invitrogen) and reverse transcribed with Superscript II reverse transcriptase (Invitrogen) according to the manufacturer's instructions. Quantitative real-time RT-PCR was performed using the SYBR Green Master mix kit (ThermoFisher). Primer sequences were as follow: Rpl32 (F) ACATCGGTTATGGGAGCAAC; Rrpl32 (R) TCCAGCTCCTTGACATTGT; Il10 (F) CCTGGGTGAGAAGCTGAAGA; Il10 (R) GCTCCACTGCCTTGCTCTTA; Il17a (F) ATCCCTCAAAGCTCAGCGTGTC; Il17a (R) GGGTCTTCATTGCGGTGGAGAG; Il22 (F) CAGCAGCCATACATCGTCAA; Il22 (R) GCCGGACATCTGTGTTGTTA; Tnfa (F) GCCTCCCTCTCATCAGTTCTA; Tnfa (R) GCTACGACGTGGGCTACAG; Rorc (F) TCTACACGGCCCTGGTTCT; Rorc (R) ATGTTCCACTCTCCTCTTCTCTTG.
Publicly available ChIP-seq data (GSE40918) for c-Maf and Stat3 was downloaded and mapped to the mm9 genome using Bowtie2 with sensitive-local predefined parameters. Resulted BAM files were converted to bigwig files and visualized by IGV genome browser.
For unpaired data, statistical difference between groups was determined by an unpaired t test when the sample size was sufficient and both groups passed the normality test, and by a Mann-Whitney test for two-tailed data otherwise. Mutant and control groups did not always have similar standard deviations and therefore an unpaired two-sided Welch's t-test was used. For paired data, a paired t test was used. Error bars represent mean ± SD. No samples were excluded from the analysis.
We first investigated the expression of c-Maf and RORγt in Tregs found in distinct organs. In accordance with previous data (4, 5), we observed that RORγt+ Tregs were mainly present in the small intestine and colon lamina propria and, to a lesser extent, in mesenteric lymph nodes (Figures 1A,B). Of note, a large proportion of Tregs (ranging from 20 to 85%) expressed c-Maf in all the examined organs, with a notable exception for the thymus (Figures 1A,C). In the intestine, both RORγt+ and RORγt− Treg subsets expressed c-Maf, although expression levels were higher in the RORγt+ compartment (Figures 1A,D,E). Strikingly, FACS analysis also revealed that a large fraction of c-Maf+ Tregs do not express RORγt. This was observed in all the examined organs and was most evident in the small intestine lamina propria, where two-thirds of the c-Maf+ Tregs lacked RORγt expression (Figure 1A).
Figure 1. Transcription factor c-Maf is required for the differentiation of RORγt+ Tregs. (A) Representative flow cytometry expression profiles of c-Maf vs. RORγt of Treg cells in the indicated organs of naive C57BL/6 mice (gate CD4+ Foxp3+). (B,C) Histograms showing the frequency of RORγt+ (B) or c-Maf+ (C) Treg cells in the indicated organs. (D,E) Expression profile (D) and median of fluorescence intensity (E) of c-Maf among Foxp3−, Foxp3+ RORγt−, and Foxp3+ RORγt+ CD4 T cells in the indicated organs. (F,G) Representative flow cytometry expression profiles of c-Maf vs. Foxp3 (F, gate CD4+) and GATA3 vs. RORγt (G, gate CD4+ Foxp3+) from mLN and siLP of WT and c-MafTregKO mice. (H–J) Histograms showing the frequency of total Tregs (H), RORγt+ Tregs (I), and GATA3+ Tregs (J) in mLN and siLP of WT and c-MafTregKO mice. Results are representative of at least three independent experiments; histograms in (B,C,E,H–J) represent the mean ± SD of at least five individual mice. Difference between groups is determined by an unpaired t test (H,I) or a Mann-Whitney test for two-tailed data (J). ***p < 0.001; ****p < 0.0001. axLN, axillary lymph nodes; mLN, mesenteric lymph nodes; siLP, small intestine lamina propria; cLP, colon lamina propria.
In lymphoid organs, c-Maf expression was mainly found among effector Tregs, characterized by the expression of high levels of ICOS and CD44 (Figure S1). In contrast to RORγt+ Tregs, which were mostly of peripheral origin, c-Maf+ Tregs were found both in Nrp1+ and Nrp1− Tregs, suggesting that c-Maf+ Tregs can be of thymic or peripheral origin (Figures 2A,B). Thymic c-Maf+ Tregs were enriched in the spleen whereas their peripheral counterparts were enriched in mesenteric lymph nodes and formed the majority of intestinal Tregs (Figure 2C).
Figure 2. c-Mat-expressing Tregs can be of thymic or peripheral origin. (A,B) Representative flow cytometry expression profiles of Nrp1 vs. c-Maf (A) or RORγt (B) of Treg cells in the indicated organs of naïve C57BL/6 mice (gate CD4+ Foxp3+ cells). (C) Pie charts show the relative frequencies of thymic (tTreg) and peripheral (pTreg) Treg cells among c-Maf+ and RORγt+ Treg subsets in the spleen, mLN and siLP. Results are representative of at least three independent experiments.
Treg-specific ablation of c-Maf resulted in increased Treg proportions in the intestine and lymphoid organs (Figures 1F,H and Figure S2A). Despite the wide distribution of c-Maf-expressing Tregs in distinct organs, Treg-conditional ablation of c-Maf did not result in systemic autoimmune disease, nor did it disturb conventional and regulatory T cell homeostasis in lymphoid organs. c-Maf-deficient Tregs also retained their in vitro suppressive capacity (Figure S2B and data not shown). In agreement with previous reports (8, 12), c-MafTregKO mice spontaneously developed intestinal inflammation and showed a near complete loss of RORγt expression in Tregs (Figures 1G,I and Figure S3). They nevertheless expressed normal percentages of intestinal GATA3+ Tregs (Figures 1G,J).
Altogether, these data indicate that c-Maf is required for the differentiation of RORγt+ Tregs and that, contrary to RORγt, c-Maf expression is also found in a large proportion of effector thymic Tregs, located in distinct organs.
The presence of c-Maf+ RORγt− Tregs in different organs and their distinct origins prompted us to further analyze the specific environmental signals responsible for the induction of c-Maf and RORγt expression by Tregs. IL-6/STAT3 and TGF-β signaling has been shown to drive RORγt+ and c-Maf expression in a variety of T cells (5, 22). Mice genetically invalidated for IL-6 (IL-6−/−), STAT3 (Stat3flox/flox-CD4CRE) or TGF-β (Tgfbr2flox/flox-Foxp3CRE) signaling showed normal to even slightly increased frequencies of c-Maf+ Tregs, although RORγt expression was considerably decreased in all the aforementioned conditions (Figure 3; see also Figure S4 for representative FACS plots). Thus, despite being necessary for the expression of RORγt by Tregs, c-Maf is not sufficient, and most likely cooperates with other signaling pathways to induce RORγt expression in Tregs.
Figure 3. IL-6/STAT3 and TGF-β signaling promote RORγt expression in Tregs independently of c-Maf. (A–F) Histograms show the frequency of RORγt+ (A,C,E) or c-Maf+ (B,D,F) cells among Treg cells (gate CD4+ Foxp3+) in indicated organs of WT and IL-6−/− (A,B), STAT3CD4KO (C,D) and TGF- βRIITregKO (E,F) mice. Results are representative of at least three independent experiments; histograms represent the mean ± SD of at least four individual mice. Difference between groups is determined by an unpaired t test or a Mann-Whitney test for two-tailed data (A,B,C siLP, D mLN, E,F). See Figure S4 for representative dot plots. *p < 0.05; **p < 0.01; ****p < 0.0001.
RORγt+ Tregs are absolutely dependent on microbiota for their development and selected bacterial species or bacterial consortia can restore the expression RORγt in Tregs of germ-free mice (4, 5, 23). Analysis of germ-free and antibiotics-treated mice revealed that, in contrast to RORγt expression, which was lost in both cases, c-Maf expression by Tregs was only marginally affected in microbiota-deficient mice (Figures 4A–D).
Figure 4. Microbial signals induce RORγt expression in Tregs but marginally affect c-Maf expression. (A–C) WT mice were treated with wide-spectrum antibiotics or a control solution for 4 weeks. Representative flow cytometry expression profiles of c-Maf vs. RORγt in Treg cells (A) and histograms showing the frequency of RORγt+ (B), and c-Maf+ (C) Tregs in the indicated organs (gate CD4+ Foxp3+). (D) Representative flow cytometry expression profiles of c-Maf vs. RORγt by Treg cells in the indicated organs of germ-free and control mice, (E,F) Naive WT or c-Maf-deficient CD4 T cells were activated in vitro for 72 h in presence of TGF-and small chain fatty acids. Histograms show the expression profiles of c-Maf (E) and RORγt (F) among Treg cells. Results are representative of at least three independent experiments; histograms in (B,C) represent the mean ± SD of at least four individual mice. Difference between groups is determined by an unpaired t test (mLN, siLP) or a Mann-Whitney test for two-tailed data (cLP), *p < 0.05; **p < 0.01; ***p < 0.001.
Short chain fatty acids (SCFA) are gut microbiota-derived bacterial fermentation products, which include acetate, propionate, and butyrate, that regulate the size and function of the colonic Treg pool (24). Treg cells were induced in vitro with TGF-β in the absence or presence of acetate, propionate, or butyrate. In this experimental setting, SCFA did not affect or slightly decreased the differentiation of Foxp3+ Treg cells (Figure S5). Addition of SCFA to the culture medium induced a 4-5-fold upregulation of RORγt expression, while minimally affecting c-Maf expression (Figures 4E,F, left panels). In absence of c-Maf, intermediate levels of RORγt were induced in Tregs treated with SCFA (Figure 4F, right panel). Overall, these observations suggest that microbiota-derived products regulate RORγt expression in Tregs through both c-Maf-dependent and independent pathways.
In presence of TGF-β and IL-2, about 75% of in vitro activated naïve CD4 T cells differentiated into Tregs, as assessed by their Foxp3 expression. Addition of IL-6, a pro-Th17 cytokine, to the TGF-β/IL-2 cytokine cocktail decreased the frequency of induced Tregs but led to the differentiation of a population of double positive c-Maf+ RORγt+ induced Tregs (iTreg17 cells; Figure 5A, lower panels and Figures 5B,C). In agreement with in vivo observations (Figures 1G,I), ablation of c-Maf expression led to a significant reduction of in vitro generated RORγt+ Tregs (Figures 5D,E,H). In the absence of STAT3, the expression of c-Maf and RORγt in iTreg17 was heavily decreased (Figures 5F–I). RT-qPCR analysis confirmed a transcriptional control of Rorc mRNA expression in these experimental settings (Figure S6). Despite showing residual c-Maf expression, STAT3 KO iTregs displayed a more severe down-regulation of RORγt than their c-Maf KO counterparts (90 vs. 50 %; Figure 5J), suggesting a prominent role of STAT3 in RORγt expression in this context. Analysis of STAT3 and c-Maf genome mapping from public ChIPseq databases (25) revealed that both transcription factors bind to the Rorc locus, albeit at distinct preferential sites (Figure 5K).
Figure 5. c-Maf and STAT3 are required for optimal RORγt expression in in vitro polarized Tregs. WT, c-Maf or STAT3-deficient naïve CD4 T cells were activated in vitro in presence of polarizing cytokines for 72 h. Protein expression was then assessed by flow cytometry. (A) Representative flow cytometry expression profiles of Foxp3 vs. CD4 and c-Maf vs. RORγt in the indicated gate by WT Treg cells polarized in vitro in the indicated conditions. (B,C) Histograms show the MFI of c-Maf (B) or RORγt (C) in WT Treg cells in vitro. (D–G) Representative flow cytometry expression profiles of c-Maf vs. RORγt by WT (D,F), c-Maf-deficient (E) and STAT3-deficient (G) Treg cells polarized in presence of TGF-and IL-2 or TGF-, IL-2, and IL-6. (H,I) Graphs show the frequency of RORγt+ cells among WT, c-Maf (H) and STAT3-deficient (I) Tregs polarized in presence of TGF-, IL-2, and IL-6. (J) Proportions of RORγt− expressing cells among CD4+ Foxp3+ Tregs is expressed as a ratio of control. Values from c-Maf or STAT3-KO Tregs were divided by the value from WT Tregs in each experimental data set. (K) Profiles generated from c-Maf and STAT3 ChiP-seq in WT, c-Maf- and STAT3-KO in vitro Th17 cells. Representative IGV tracks showing c-Maf (green), STAT3 (red) binding sites highlighted in gray at the Rorc locus among the indicated cell population. Gene location is indicated at the top of the panel. Y axis indicates the normalized read coverage for each track. Results are representative of at least three independent experiments. Symbols in histograms represent individual mice. Difference between groups is determined by a paired t test. *p < 0.05; ****p < 0.0001.
To determine whether the role of STAT3 in promoting RORγt induction solely relies on c-Maf, we restored c-Maf expression in STAT3-deficient iTreg17 cells. WT and c-Maf KO CD4 T cells were infected with a control-GFP or a c-Maf-GFP encoding retrovirus. Although the c-Maf encoded retrovirus did restore RORγt expression in c-Maf-deficient Tregs and induced optimal levels of c-Maf in STAT3 KO Tregs, it was unable to restore RORγt expression in the latter cells (Figure 6). Collectively, these data indicate that in Tregs, an additional STAT3-driven but cMaf-independent pathway is required to promote optimal RORγt expression.
Figure 6. c-Maf does not rescue RORγt expression in Tregs in the absence of STAT3. WT, c-Maf or STAT3-deficient naïve CD4 T cells were activated in vitro in presence of TGF-β, IL-2, and IL-6 and infected with a control or c-Maf- expressing retrovirus. c-Maf (A) and RORγt (B) expression profiles and MFI as assessed by flow cytometry after 72 h (gate CD4+ Foxp3+). Results are representative of three independent experiments.
While IL-6 promoted RORγt expression in wild type iTregs, it surprisingly led to a marked reduction of this transcription factor in STAT3-deficient iTregs (Figures 5F,G), thus revealing the presence of an inhibitory pathway regulating RORγt expression. Studies by Costa-Pereira et al. have shown that in STAT3 KO fibroblasts, IL-6 signals through STAT1 and has IFNγ-like effects (26). We observed that in contrast to their wild type counterparts, STAT3 KO CD4+ T cells cultured in the presence of IL-6 expressed higher levels of phospho-STAT1 (Figure S7). This prompted us to investigate the consequences of a STAT1/Th1 inflammatory pathway on the differentiation of RORγt+ Tregs.
Infection with Toxoplasma gondii results in a highly Th1-polarized microenvironment leading to altered Treg cell homeostasis (27). In particular, the strong Th1 environment triggered by T. gondii infection has been shown to induce T-bet and IFN-γ expression in Treg cells (27, 28). Analysis of Tregs from the small intestine lamina propria of T. gondii infected C57BL/6 mice confirmed the emergence of a T-bet+ Treg subset (Figures 7A,B, upper panels). Interestingly, T. gondii infection resulted in a reduced frequency of tissue-associated RORγt+ Tregs, despite having minimal effect on Maf expression (Figures 7A,B, middle and lower panels). Th17 cell differentiation was also suppressed during T. gondii infection (Figure S8), consistent with the observation that Th17 cell differentiation and Th1 cell differentiation are mutually suppressive (29, 30). This reciprocal exclusion was also reflected among Tregs, as T-bet and RORγt were expressed in distinct Treg cell subsets in the infected mice (Figure 7C).
Figure 7. Inflammatory Th1 responses opposes RORγt expression in Tregs. (A) Histograms show RORγt, c-Maf and T-bet expression among Tregs in the siLP of WT mice 8 days after infection with Toxoplasma gondii or control mice (gate CD4+ Foxp3+). (B) Frequency of RORγt+, c-Maf+, and T-bet+ cells among Treg cells in the siLP of WT mice 8 days after infection with Toxoplasma gondii or control mice. (C) Representative flow cytometry expression profiles of RORγt vs. T-bet among Treg cells isolated from mLN of WT mice infected or not with Toxoplasma gondii (gate CD4+ Foxp3+). (D) Histograms show RORγt, c-Maf, and T-bet expression in WT and STAT1-deficient in vitro Treg17 cells stimulated with or without IFN-y (100 ng/ml) for 72 h. Results are representative of at least two (A–C) or three (D) independent experiments; histograms represent the mean ± SD of five individual mice. Difference between groups is determined by a Mann-Whitney test for two-tailed data. *p < 0.05.
We next wished to evaluate the effect of Th1-promoting signals on RORγt expression by Tregs, Addition of IFN-γ to the iTreg17 differentiation media led to the selective accumulation of the phosphorylated form of STAT1, without affecting neither phospho-STAT3 accumulation nor c-Maf expression by iTregs (Figure S9 and Figure 7D, upper middle panels). Of note, IFN-γ led to the accumulation of Tbet-expressing Tregs, with a concomitant reduction in the proportions of RORγt+ Tregs (Figure 7D, upper left and right panels). STAT1 KO Tregs were insensitive to the IFN-γ-driven inhibition of RORγt expression (Figure 7D, lower panels). Altogether these results strongly suggest that the IFN-γ/STAT1 signaling pathway negatively regulates RORγt expression even in the presence of an active IL-6/STAT3-driven pathway.
RORγt+ Tregs form a distinct population of regulatory T cells that is crucial to maintain gastrointestinal homeostasis and prevent colitis (4, 5, 7). Whereas, RORγt+ Treg function has been amply documented, the factors driving RORγt+ Treg differentiation remain ill-defined. We show herein that multiple signaling pathways cooperate to exert both positive and negative control over RORγt+ expression by Tregs.
We confirmed and extended previous data showing that transcription factor c-Maf plays a major role for the acquisition of RORγt expression by Tregs (8, 12, 31). However, contrary to RORγt, which is confined to a subset of intestinal Tregs, c-Maf expression is found in a wider proportion of Tregs, located in distinct organs. This observation suggests that c-Maf cooperates with other pathways to induce optimal RORγt expression by these regulatory cells.
We showed that microbial signals, as well as IL-6-, STAT3-, and TGF-β-signaling pathways promote RORγt+ Treg cell differentiation in a non-redundant manner. Constrastingly, blocking any one of these pathways only had minimal effect on c-Maf expression in vivo, suggesting that several redundant pathways cooperate to induce c-Maf expression in Tregs.
Neumann et al. reported that, in addition to the loss of RORγt expression in Tregs, which we also observed, germ-free mice exhibited a near complete loss of c-Maf expression in intestinal Tregs. While we could not reproduce this observation, we observed that Tregs found in germ-free mice expressed slightly reduced levels of c-Maf. Reminding that the RORγt+ population expresses the highest levels of c-Maf among intestinal Tregs, we therefore speculate that RORγt expression in Tregs requires a high c-Maf expression threshold and that even a minimal decrease in c-Maf expression could hinder RORγt expression in microbiota-driven intestinal Tregs.
Short chain fatty acids (SCFA) produced by gut commensal microbes induce functional colonic Tregs and protect against T cell-dependent experimental colitis (24, 32). Depending on the cytokine environment and immunological context, butyrate, acetate, and propionate, the most available SCFA in the gut, can also support IL-10 expression in Th1 and Th17 effector cells, thereby inhibiting colitis caused by pathogenic T cells (33, 34). We show herein that SCFA induce RORγt expression in in vitro differentiated Tregs in a c-Maf-dependent and independent manner. Although SCFA promote peripheral Treg cell generation (24), no significant correlation was observed between any single SCFA and RORγt+ Treg frequency. Moreover, oral supplementation with a mixture of acetate, propionate, and butyrate failed to increase the frequency of mesenteric lymph node RORγt+ Tregs (4, 23). Thus, although our in vitro data showed that SCFA can promote RORγt expression in a pro-Treg culture medium (i.e., in the presence of TGF-β), SCFA alone cannot account for the impact of the microbiota on intestinal RORγt+ Treg development in vivo.
The proportion of RORγt+ Tregs was severely reduced in mice deficient for STAT3 or TGF-β signaling. Rather surprisingly, while STAT3 and TGF-β signaling drive c-Maf expression in Tfh and Th17 cells (25, 35, 36), we found that mice deficient for STAT3 or TGF-β in the T cell compartment harbored normal to even slightly increased numbers of c-Maf+ Tregs, indicating that, while STAT3 and TFG-β seem dispensable for the induction of c-Maf expression, they are both essential to achieve optimal differentiation of RORγt+ Tregs in the intestine.
Besides c-Maf, many genes involved in Th17 differentiation, such as Irf4, Batf, Rora, Ahr, Sox5t, and Hif-1α, are expressed in response to STAT3 activation (19, 37–41). Sox5t is a T cell isoform of Sox5 which induces RORγt expression in Th17 cells via physical interaction with c-Maf (19). As enforced expression of c-Maf was not sufficient to induce Foxp3+ RORγt+ T cell differentiation in absence of STAT3, we speculate that Sox5, or other molecules downstream of STAT3, act together with c-Maf to achieve RORγt expression in Tregs. Interestingly, ChIP-seq data revealed that STAT3 and c-Maf bind with different intensities to distinct sites of the Rorc locus. The inability of c-Maf to compensate STAT3-deficiency in RORγt+ Treg differentiation could therefore also be explained by a direct effect of STAT3 on RORγt expression. This is in agreement with previous data showing that STAT3 binds to intron 1 of Rorc gene and induces chromatin remodeling of the locus (39). Overall, it would seem that STAT3 controls RORγt+ Treg cell fate both through direct activation of the Rorc locus and by regulating the expression of a set of genes, including c-Maf, that is essential for RORγt+ Treg differentiation (Figure S10).
In T cells, IL-6 predominantly signals via STAT3 and to a lesser extent via STAT1. It has been proposed that the accessibility of different STATs within the cell influences the outcome of cytokine signaling (42), as illustrated by the observation that IL-6 acquired the ability to induce the expression of STAT1-dependent genes in STAT3-deficient cells (26, 43). However, Hirahara et al. showed an asymmetric action of STAT3 and STAT1 at the genomic level where much of STAT1 chromatin binding was STAT3-dependent. This challenged the classical view that, in the absence of its major STAT module, a cytokine would acquire an alternative STAT-signaling profile (44). Yet, in the absence of STAT3, and despite a global reduction in STAT1 chromatin binding, some preferential STAT1 binding sites were conserved in a group of IL-6 downregulated genes. With this in mind we hypothesized a negative influence of STAT1 on RORγt expression by Tregs. Indeed, STAT3-deficient T cells showed increased STAT1 phosphorylation in response to IL-6, compatible with a switch from STAT3 to STAT1 signaling in these cells. We further showed that IFN-γ-driven activation of STAT1 opposed RORγt expression in Tregs, without affecting Foxp3 and c-Maf expression or STAT3 phosphorylation. Naïve STAT1 KO mice did not show altered proportions of intestinal RORγt+ Tregs (data not shown), which could be explained by the lack of inflammatory Th1 components at steady state. Indeed, in wild type mice infected with the Th1-prototypic Toxoplasma gondii intestinal parasite, RORγt expression was decreased in intestinal Tregs, confirming the antagonistic role of inflammatory Th1 responses on RORγt expression in Tregs in vivo.
Different integrative pathways have been proposed to explain the functional outcome of multiple STAT signaling in distinct T cell subsets (45). Meyer Zu Horste et al. recently reported that death receptor Fas promotes Th17 cell differentiation and inhibits Th1 cell development by preventing STAT1 activation. In this model, Fas regulated the STAT1 vs. STAT3 balance by binding and sequestering STAT1 (46). Although not formerly excluded, sequestration of STAT3 is unlikely in RORγt+ Tregs as addition of IFN-γ to the iTreg17 culture media did not affect the phosphorylation status of STAT3. Our data rather suggest that Treg cell fate results from the balance of STAT1 and STAT3 driven signals. Gene expression could also conceivably be fine-tuned by the formation of STAT1/STAT3 heterodimers, as proposed for the IL-21 signaling (47). Of interest, patients with loss-of-function STAT3 mutations or with gain-of-function STAT1 mutations show similar susceptibility to fungal infections (48, 49). In the latter group, overactive STAT1 appears to limit STAT3-driven antifungal responses (49).
Further work is required to decipher whether STAT1 interacts with STAT3 or exerts an independent negative role on RORγt+ Treg cell fate. As T-bet is induced in Tregs that develop during Toxoplasma infection or in response to IFN-γ, we can also envision that STAT1 signaling inhibits RORγt expression through T-bet blocking of Runx1-mediated transactivation of Rorc, as previously reported for the Th1/Th17 lineage specification (50). Regardless of the molecular mechanism, the observation that IFN-γ/STAT1 signaling pathway negatively regulates RORγt, even in the presence of an active IL-6/STAT3 pathway, suggests a dominant negative effect of STAT1 over STAT3 in these experimental conditions.
The antagonism between STAT1 and STAT3 seems to be cell type-specific or specific to a certain gene locus, as a cooperation between STAT1 and STAT3 downstream of IL-6 has been described for optimal Bcl6 induction and Tfh differentiation in response to viral infections (51).
Collectively, our data reveal that, beyond the previously established c-Maf/RORγt interplay, multiple signaling pathways cooperate to exert a tight control over RORγt expression in Tregs.
All datasets generated for this study are included in the article/Supplementary Material.
The animal study was reviewed and approved by Université Libre de Bruxelles Institutional Animal Care and Use Committee.
FA conceived and supervised the research program and experiments, and wrote the manuscript. HH performed the experiments, acquired, and analyzed data. SD, FV, AA, YA, HE-K, and GO performed experiments and contributed to the analysis of the data. OL conceived the research program and revised the manuscript.
This work was supported by the European Regional Development Fund (ERDF) and the Walloon Region (Wallonia-Biomed portfolio, 411132-957270), grant from the Fonds Jean Brachet and research credit from the National Fund for Scientific Research, FNRS, Belgium. FA was a Research Associate at the FNRS. YA was recipient of a research fellowship from the FNRS/Télévie. HH was supported by a Belgian FRIA fellowship.
The authors declare that the research was conducted in the absence of any commercial or financial relationships that could be construed as a potential conflict of interest.
We especially thank Muriel Moser for her great support all along this work, for stimulating discussions and for careful revision of the manuscript. We also thank Caroline Abdelaziz and Véronique Dissy for animal care and for technical support. This manuscript has been released as a preprint at bioRxiv (52).
The Supplementary Material for this article can be found online at: https://www.frontiersin.org/articles/10.3389/fimmu.2019.03007/full#supplementary-material
1. Chen W, Jin W, Hardegen N, Lei KJ, Li L, Marinos N, et al. Conversion of peripheral CD4+CD25− naive T cells to CD4+CD25+ regulatory T cells by TGF-beta induction of transcription factor Foxp3. J Exp Med. (2003) 198:1875–86. doi: 10.1084/jem.20030152
2. Whibley N, Tucci A, Powrie F. Regulatory T cell adaptation in the intestine and skin. Nat Immunol. (2019) 20:386–96. doi: 10.1038/s41590-019-0351-z
3. Panduro M, Benoist C, Mathis D. Tissue tregs. Annu Rev Immunol. (2016) 34:609–33. doi: 10.1146/annurev-immunol-032712-095948
4. Sefik E, Geva-Zatorsky N, Oh S, Konnikova L, Zemmour D, McGuire AM, et al. MUCOSAL immunology individual intestinal symbionts induce a distinct population of Rorγ+ regulatory T cells. Science. (2015) 349:8993–7. doi: 10.1126/science.aaa9420
5. Ohnmacht C, Park JH, Cording S, Wing JB, Atarashi K, Obata Y, et al. MUCOSAL immunology the microbiota regulates type 2 immunity through Rorγt+ T cells. Science. (2015) 349:989–93. doi: 10.1126/science.aac4263
6. Lochner M, Ohnmacht C, Presley L, Bruhns P, Si-Tahar M, Sawa S, et al. Microbiota-induced tertiary lymphoid tissues aggravate inflammatory disease in the absence of rorgamma T and LTi cells. J Exp Med. (2011) 208:125–34. doi: 10.1084/jem.20100052
7. Yang B-H, Hagemann S, Mamareli P, Lauer U, Hoffmann U, Beckstette M, et al. Foxp3+ T cells expressing Rorγt represent a stable regulatory T-cell effector lineage with enhanced suppressive capacity during intestinal inflammation. Mucosal Immunol. (2015) 205:1381–93. doi: 10.1038/mi.2015.74
8. Xu M, Pokrovskii M, Ding Y, Yi R, Au C, Harrison OJ, et al. c-Maf-dependent regulatory T cells mediate immunological tolerance to a gut pathobiont. Nature. (2018) 554:373–7. doi: 10.1038/nature25500
9. Kim BS, Lu H, Ichiyama K, Chen X, Zhang YB, Mistry NA, et al. Generation of Rorγt(+) antigen-specific T regulatory 17 cells from Foxp3(+) precursors in autoimmunity. Cell Rep. (2017) 21:195–207. doi: 10.1016/j.celrep.2017.09.021
10. Komatsu N, Okamoto K, Sawa S, Nakashima T, Oh-hora M, Kodama T, et al. Pathogenic conversion of Foxp3+ T cells into Th17 cells in autoimmune arthritis. Nat Med. (2014) 20:62–8. doi: 10.1038/nm.3432
11. Downs-Canner S, Berkey S, Delgoffe GM, Edwards RP, Curiel T, Odunsi K, et al. Suppressive IL-17a(+)Foxp3(+) and ex-Th17 IL-17a(neg)Foxp3(+) T(reg) cells are a source of tumour-associated T(reg) cells. Nat Commun. (2017) 8:14649. doi: 10.1038/ncomms14649
12. Wheaton JD, Yeh CH, Ciofani M. Cutting edge: c-Maf is required for regulatory T cells to adopt Rorγt+ and follicular phenotypes. J Immunol. (2017) 199:3931–6. doi: 10.4049/jimmunol.1701134
13. Imbratta C, Leblond MM, Bouzourène H, Speiser DE, Velin D, Verdeil G. Maf deficiency in T cells dysregulates T(reg) - T(h)17 balance leading to spontaneous colitis. Sci Rep. (2019) 9:6135. doi: 10.1038/s41598-019-42486-2
14. Hiramatsu Y, Suto A, Kashiwakuma D, Kanari H, Kagami S, Ikeda K, et al. C-maf activates the promoter and enhancer of the IL-21 gene, and TGF-beta inhibits c-maf-induced IL-21 production in CD4+ T cells. J Leukoc Biol. (2010) 87:703–12. doi: 10.1189/jlb.0909639
15. Sahoo A, Alekseev A, Tanaka K, Obertas L, Lerman B, Haymaker C, et al. Batf is important for IL-4 expression in T follicular helper cells. Nat Commun. (2015) 6:7997. doi: 10.1038/ncomms8997
16. Apetoh L, Quintana FJ, Pot C, Joller N, Xiao S, Kumar D, et al. The aryl hydrocarbon receptor interacts with c-Maf to promote the differentiation of type 1 regulatory T cells induced by IL-27. Nat Immunol. (2010) 11:854–61. doi: 10.1038/ni.1912
17. Bauquet AT, Jin H, Paterson AM, Mitsdoerffer M, Ho IC, Sharpe AH, et al. The costimulatory molecule ICOS regulates the expression of c-Maf and IL-21 in the development of follicular T helper cells and Th-17 cells. Nat Immunol. (2009) 10:167–75. doi: 10.1038/ni.1690
18. Andris F, Denanglaire S, Anciaux M, Hercor M, Hussein H, Leo O. The transcription factor c-Maf promotes the differentiation of follicular helper T cells. Front Immunol. (2017) 8:480. doi: 10.3389/fimmu.2017.00480
19. Tanaka S, Suto A, Iwamoto T, Kashiwakuma D, Kagami S, Suzuki K, et al. Sox5 and c-Maf cooperatively induce Th17 cell differentiation via Rorγt induction as downstream targets of STAT3. J Exp Med. (2014) 211:1857–74. doi: 10.1084/jem.20130791
20. Rubtsov YP, Rasmussen JP, Chi EY, Fontenot J, Castelli L, Ye X, et al. Regulatory T cell-derived interleukin-10 limits inflammation at environmental interfaces. Immunity. (2008) 28:546–58. doi: 10.1016/j.immuni.2008.02.017
21. Chytil A, Magnuson MA, Wright CV, Moses HL. Conditional inactivation of the TGF-β type Ii receptor using Cre:Lox. Genesis. (2002) 32:73–5. doi: 10.1002/gene.10046
22. Veldhoen M, Hocking RJ, Atkins CJ, Locksley RM, Stockinger B. TGFβ in the context of an inflammatory cytokine milieu supports de novo differentiation of IL-17-producing T cells. Immunity. (2006) 24:179–89. doi: 10.1016/j.immuni.2006.01.001
23. Abdel-Gadir A, Stephen-Victor E, Gerber GK, Noval Rivas M, Wang S, Harb H, et al. Microbiota therapy acts via a regulatory T cell MyD88/Rorγt pathway to suppress food allergy. Nat Med. (2019) 25:1164–74. doi: 10.1038/s41591-019-0461-z
24. Arpaia N, Campbell C, Fan X, Dikiy S, van der Veeken J, deRoos P, et al. Metabolites produced by commensal bacteria promote peripheral regulatory T-cell generation. Nature. (2013) 504:451–5. doi: 10.1038/nature12726
25. Ciofani M, Madar A, Galan C, Sellars M, Mace K, Pauli F, et al. A validated regulatory network for Th17 cell specification. Cell. (2012) 151:289–303. doi: 10.1016/j.cell.2012.09.016
26. Costa-Pereira AP, Tininini S, Strobl B, Alonzi T, Schlaak JF, Is'harc H, et al. Mutational -switch of an IL-6 response to an interferon-γ-like response. Proc Natl Acad Sci USA. (2002) 99:8043–7. doi: 10.1073/pnas.122236099
27. Oldenhove G, Bouladoux N, Wohlfert EA, Hall JA, Chou D, Dos Santos L, et al. Decrease of Foxp3+ treg cell number and acquisition of effector cell phenotype during lethal infection. Immunity. (2009) 31:772–86. doi: 10.1016/j.immuni.2009.10.001
28. Hall AO, Beiting DP, Tato C, John B, Oldenhove G, Lombana CG, et al. The cytokines interleukin 27 and interferon-γ promote distinct treg cell populations required to limit infection-induced pathology. Immunity. (2012) 37:511–23. doi: 10.1016/j.immuni.2012.06.014
29. Harrington LE, Hatton RD, Mangan PR, Turner H, Murphy TL, Murphy KM, et al. Interleukin 17-producing CD4+ effector T cells develop via a lineage distinct from the T helper type 1 and 2 lineages. Nat Immunol. (2005) 6:1123–32. doi: 10.1038/ni1254
30. Park H, Li Z, Yang XO, Chang SH, Nurieva R, Wang YH, et al. A distinct lineage of CD4 T cells regulates tissue inflammation by producing interleukin 17. Nat Immunol. (2005) 6:1133–41. doi: 10.1038/ni1261
31. Neumann C, Blume J, Roy U, Teh PP, Vasanthakumar A, Beller A, et al. C-maf-dependent treg cell control of intestinal Th17 cells and iga establishes host-microbiota homeostasis. Nat Immunol. (2019) 20:471–81. doi: 10.1038/s41590-019-0316-2
32. Furusawa Y, Obata Y, Fukuda S, Endo TA, Nakato G, Takahashi D, et al. Commensal microbe-derived butyrate induces the differentiation of colonic regulatory T cells. Nature. (2013) 504:446–50. doi: 10.1038/nature12721
33. Park J, Kim M, Kang SG, Jannasch AH, Cooper B, Patterson J, et al. Short-chain fatty acids induce both effector and regulatory T cells by suppression of histone deacetylases and regulation of the mtor-s6k pathway. Mucosal Immunol. (2015) 8:80–93. doi: 10.1038/mi.2014.44
34. Sun M, Wu W, Chen L, Yang W, Huang X, Ma C, et al. Microbiota-derived short-chain fatty acids promote TH1 cell IL-10 production to maintain intestinal homeostasis. Nat Commun. (2018) 9:3555. doi: 10.1038/s41467-018-05901-2
35. Rutz S, Noubade R, Eidenschenk C, Ota N, Zeng W, Zheng Y, et al. Transcription factor c-Maf mediates the TGF-[beta]-dependent suppression of IL-22 production in Th17 cells. Nat Immunol. (2011) 12:1238–45. doi: 10.1038/ni.2134
36. Mari N, Hercor M, Denanglaire S, Leo O, Andris F. The capacity of Th2 lymphocytes to deliver B-cell help requires expression of the transcription factor STAT3. Eur J Immunol. (2013) 43:1489–98. doi: 10.1002/eji.201242938
37. Brüstle A, Heink S, Huber M, Rosenplänter C, Stadelmann C, Yu P, et al. The development of inflammatory Th-17 cells requires interferon-regulatory factor 4. Nat Immunol. (2007) 8:958. doi: 10.1038/ni1500
38. Dang EV, Barbi J, Yang HY, Jinasena D, Yu H, Zheng Y, et al. Control of T(h)17/T(reg) balance by hypoxia-inducible factor 1. Cell. (2011) 146:772–84. doi: 10.1016/j.cell.2011.07.033
39. Durant L, Watford WT, Ramos HL, Laurence A, Vahedi G, Wei L, et al. Diverse targets of the transcription factor STAT3 contribute to t cell pathogenicity and homeostasis. Immunity. (2010) 32:605–15. doi: 10.1016/j.immuni.2010.05.003
40. Schraml BU, Hildner K, Ise W, Lee W-L, Smith WA-E, Solomon B, et al. The AP-1 transcription factor batf controls Th17 differentiation. Nature. (2009) 460:405. doi: 10.1038/nature08114
41. Yang XO, Pappu BP, Nurieva R, Akimzhanov A, Kang HS, Chung Y, et al. T helper 17 lineage differentiation is programmed by orphan nuclear receptors RORα and RORγ. Immunity. (2008) 28:29–39. doi: 10.1016/j.immuni.2007.11.016
42. Regis G, Pensa S, Boselli D, Novelli F, Poli V. Ups and downs: the STAT1:STAT3 seesaw of interferon and gp130 receptor signalling. Semin Cell Dev Biol. (2008) 19:351–9. doi: 10.1016/j.semcdb.2008.06.004
43. Schiavone D, Avalle L, Dewilde S, Poli V. The immediate early genes fos and egr1 become STAT1 transcriptional targets in the absence of STAT3. FEBS Lett. (2011) 585:2455–60. doi: 10.1016/j.febslet.2011.06.020
44. Hirahara K, Onodera A, Villarino AV, Bonelli M, Sciumè G, Laurence A, et al. Asymmetric action of STAT transcription factors drives transcriptional outputs and cytokine specificity. Immunity. (2015) 42:877–89. doi: 10.1016/j.immuni.2015.04.014
45. Lin JX, Leonard WJ. Fine-tuning cytokine signals. Annu Rev Immunol. (2019) 37:295–324. doi: 10.1146/annurev-immunol-042718-041447
46. Meyer Zu Horste G, Przybylski D, Schramm MA, Wang C, Schnell A, Lee Y, et al. Fas promotes T helper 17 cell differentiation and inhibits T helper 1 cell development by binding and sequestering transcription factor STAT1. Immunity. (2018) 48:556–69.e7. doi: 10.1016/j.immuni.2018.03.008
47. Wan CK, Andraski AB, Spolski R, Li P, Kazemian M, Oh J, et al. Opposing roles of STAT1 and STAT3 in IL-21 function in CD4+ T cells. Proc Natl Acad Sci USA. (2015) 112:9394–9. doi: 10.1073/pnas.1511711112
48. O'Shea JJ, Holland SM, Staudt LM. JAKs and stats in immunity, immunodeficiency, and cancer. N Engl J Med. (2013) 368:161–70. doi: 10.1056/NEJMra1202117
49. Casanova JL, Holland SM, Notarangelo LD. Inborn errors of human jaks and stats. Immunity. (2012) 36:515–28. doi: 10.1016/j.immuni.2012.03.016
50. Lazarevic V, Chen X, Shim J-H, Hwang E-S, Jang E, Bolm AN, et al. T-bet represses Th17 differentiation by preventing runx1-mediated activation of the gene encoding Rorγt. Nat Immunol. (2011) 12:96–104. doi: 10.1038/ni.1969
51. Choi YS, Eto D, Yang JA, Lao C, Crotty S. Cutting edge: stat1 is required for IL-6 - mediated Bcl6 induction for early follicular helper cell differentiation. J Immunol. (2013) 190:3049–53. doi: 10.4049/jimmunol.1203032
Keywords: Treg subsets, transcription factors, RORγt, cell differentiation, signal transduction, c-Maf
Citation: Hussein H, Denanglaire S, Van Gool F, Azouz A, Ajouaou Y, El-Khatib H, Oldenhove G, Leo O and Andris F (2020) Multiple Environmental Signaling Pathways Control the Differentiation of RORγt-Expressing Regulatory T Cells. Front. Immunol. 10:3007. doi: 10.3389/fimmu.2019.03007
Received: 15 October 2019; Accepted: 09 December 2019;
Published: 08 January 2020.
Edited by:
Remy Bosselut, National Cancer Institute (NCI), United StatesReviewed by:
Bin Li, Shanghai Jiao Tong University School of Medicine, ChinaCopyright © 2020 Hussein, Denanglaire, Van Gool, Azouz, Ajouaou, El-Khatib, Oldenhove, Leo and Andris. This is an open-access article distributed under the terms of the Creative Commons Attribution License (CC BY). The use, distribution or reproduction in other forums is permitted, provided the original author(s) and the copyright owner(s) are credited and that the original publication in this journal is cited, in accordance with accepted academic practice. No use, distribution or reproduction is permitted which does not comply with these terms.
*Correspondence: Fabienne Andris, ZmFuZHJpc0B1bGIuYWMuYmU=
Disclaimer: All claims expressed in this article are solely those of the authors and do not necessarily represent those of their affiliated organizations, or those of the publisher, the editors and the reviewers. Any product that may be evaluated in this article or claim that may be made by its manufacturer is not guaranteed or endorsed by the publisher.
Research integrity at Frontiers
Learn more about the work of our research integrity team to safeguard the quality of each article we publish.