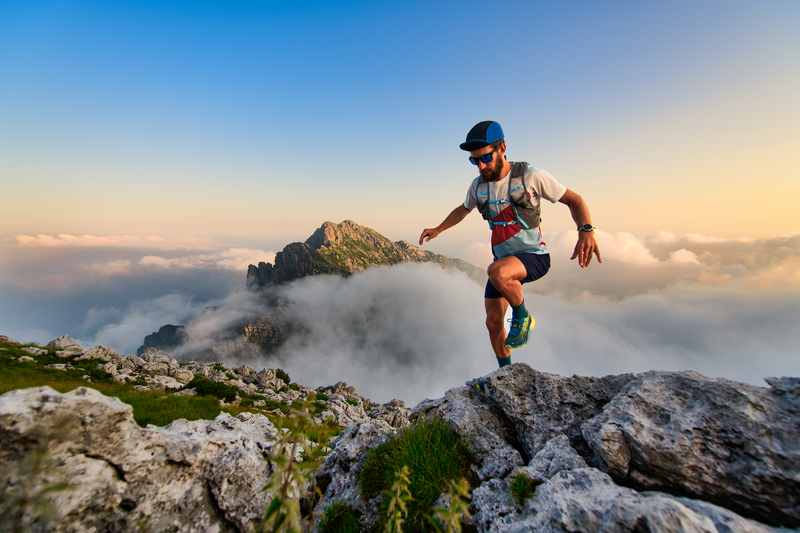
95% of researchers rate our articles as excellent or good
Learn more about the work of our research integrity team to safeguard the quality of each article we publish.
Find out more
ORIGINAL RESEARCH article
Front. Immunol. , 22 August 2019
Sec. Comparative Immunology
Volume 10 - 2019 | https://doi.org/10.3389/fimmu.2019.01991
This article is part of the Research Topic Vaccines and Immunostimulants for Finfish View all 21 articles
Temperature is one of the major factors that affect the outbreak of infectious disease. Lines of evidences have shown that virulence factors can be controlled by thermo-sensors in bacterial pathogens. However, how temperature influences host's responses to the pathogen is still largely unexplored, and the study of this might pave the way to develop strategies to manage pathogenic bacterial infection. In the present study, we show that finfish Carassius carassius, the crucian carp that is tolerant to a wide range of temperatures, is less susceptible to bacterial infection when grown in 20°C than in 30°C. The different responses of C. carassius to bacterial infection could be partially explained by the distinct metabolisms under the specific temperatures: C. carassius shows elevated tricarboxylic acid cycle (TCA cycle) but decreased taurine and hypotaurine metabolism as well as lower biosynthesis of unsaturated fatty acids at 30°C. The decreased abundance of palmitate, threonine, and taurine represents the most characteristic metabolic feature. Consistently, exogenous palmitate, threonine, or taurine enhances the survival of C. carassius to bacterial infection at 30°C in a dose-dependent manner. This effect could be attributed to the inhibition on the TCA cycle by the three metabolites. This notion is further supported by the fact that low concentration of malonate, a succinate dehydrogenase inhibitor, increases the survival of C. carassius at 30°C as well. On the other hand, addition of the three metabolites rescued the decreased expression of pro-inflammatory cytokines including TNF-α1, TNF-α2, IL-1β1, IL-1β2, and lysozyme at 30°C. Taken together, our results revealed an unexpected relationship between temperature and metabolism that orchestrates the immune regulation against infection by bacterial pathogens. Thus, this study shed light on the modulation of finfish physiology to fight against bacterial infection through metabolism.
Climate is one of the most important environmental factors that influences the spread of communicable diseases prone to their epidemic (1). The outbreak of bacterial infectious disease depends on not only the pathogenicity of the bacteria but also many environmental factors, including the temperature changes. In aquaculture, infections mostly occur in spring and fall when the water temperature is between 22 and 30°C (2). The outbreak of bacterial infectious diseases depends on at least two determining factors: (1) the multiple strategies of pathogens to sense the environmental perturbations and fluctuations as cues to adjust their growth, development and pathogenesis. It is well established that the elevated temperature promotes the expression of virulence genes like type III secretion system, temperature-sensitive hemagglutinin, adhesins, and other virulence regulators in the species of Edwardsiella, Vibrio, and Aeromonas (2–6); (2) the fish's immune responses to pathogens that affected by residential water temperature. It has long been shown that the elevated temperature negatively affected fish's immune responses to vaccination and reduced the phagocytosis to bacterial pathogens (7). However, the interplay between temperature and host immune response is still largely unexplored (8, 9).
In aquaculture, infectious disease caused by bacteria is the major cause of mortalities that result in huge economic loss (10). A good research model to investigate the relationship between temperature and host immune responses might contribute to the control of infectious diseases in aquaculture and is thus highly needed. Crucian carp Carassius auratus is one of the most extensively cultured freshwater fish throughout the world, reaching 2.2 million tons in the year of 2010 (11). Furthermore, crucian carp is a potential model to study genome evolution and physiological adaptation as it has exhibited a striking capacity to cope with the low level of oxygen and a wide range of ambient temperatures (11, 12). The capability of crucian carp to adapt to versatile environments suggests that it is an ideal model to investigate the impacts of environmental factors on the immune responses of fish to pathogens (13).
Edwardsiella tarda, a zoonotic Gram-negative pathogen, infects a broad range of hosts including fish, amphibians, reptiles, and mammals, and causes edwardsiellosis (14–16). E. tarda represents a critical pathogen that causes huge economic and biomasses loss in aquaculture (17, 18). The outbreak of edwardsiellosis can lead to massive fish death in a short period. The infected fish shows abnormal swimming behavior, including spiral movement and floating near the water surface (19–22). Epidemiological investigation of the outbreak of E. tarda indicates that infection usually occurs when environmental conditions are imbalanced, such as higher water temperature, poor water quality, and high organic content (23, 24). Teleost, including crucian carps and striped bass, are ectotherm, whose body temperature is largely influenced by the water temperature. Consistently, it has different physiology or metabolism at different environmental conditions (25–28). Since metabolism is linked to immunity, it is not astonishing that bacterial infections occur more frequently at certain environmental conditions (29–32). However, the interplay among water temperature, metabolism and immunity is not well understood. The elucidation of such relationship might be useful for the development of a new strategy to fight against bacterial infection at high temperatures.
Metabolomics provides a “top-down,” integrated view of biochemistry in complex organisms that could be used to profile the metabolic response to internal and external environments. Crucial biomarkers identified from the metabolomics data could be used to reprogram the metabolome (33, 34). Antibiotic-resistant bacteria and antibiotic-sensitive bacteria have a distinct metabolome, and the crucial metabolic biomarkers can reprogram the antibiotic-resistant bacteria to become sensitive to antibiotics again, shedding light on a new strategy to manage bacterial antibiotic-resistance (33, 35–38). Similarly, tilapia showed distinct responses to Streptococcus agalactiae infection when grown at 20, 25, and 30°C. The metabolomics profiling of liver extract from tilapia grown at different temperatures showed that they have different metabolic responses. L-Proline, one of the crucial biomarkers, decreases along with the increasing temperatures. Interestingly, exogenous L-Proline promotes the survival of tilapias to S. agalactiae at 30°C (39). These results indicate that metabolic modulation is a novel approach to promote fish survival against bacterial infection at higher temperature (40–42). However, the underlying mechanism is still unknown.
In the present study, we adopted crucian carp and E. tarda as the research model to investigate the interplay of temperature, metabolism, and immunity. By challenging crucian carp with E. tarda, we found that crucian carp grown at 20°C, the optimum temperature, was more resistant to bacterial infection than those grown at 30°C. The profiling of the metabolome in fish grown at these two different environmental conditions identified three crucial biomarkers, palmitate, threonine, and taurine, which can be used to reprogram the metabolome of crucian carp and make the fish less susceptible to bacterial infection. More importantly, these three metabolites increase the expression of innate immune genes that are known to promote inflammation to kill pathogens.
To investigate how temperature affects host's susceptibility to bacterial infection, crucian carps Carassius carassius, cultured at 20 or 30°C, were challenged with 1 × 105 CFU E. tarda EIB202. Upon infection by E. tarda, the cumulative survival rates of C. carassius grown at 20°C were 76%, compared to 30% grown at 30°C (Figure 1). This result indicates that crucian carps cultured at 30°C are more susceptible to E. tarda infection than those cultured at 20°C.
Figure 1. The survival of crucian carp to E. tarda infection cultured at 20 and 30°C. C. carassius (n = 30 per group) were acclimated at 20 or 30°C for 7 days before bacterial challenge. For bacterial infection, C. carassius were injected with 10 μl 1 × 105 CFU/fish E. tarda or 10 μl saline solution as negative control. Accumulative death was monitored for a total of 15 days.
Hosts adjusted their metabolism to adapt to different temperatures that have profound effects on their capability to cope with bacterial infection (39). We apply GC-MS-based metabolomics to investigate the metabolic differences between the C. carassius that were acclimated at 20 and 30°C for 7 days. Spleens are removed, homogenized, and the metabolites were extracted for non-targeted metabolomic analysis (Figure S1). For each spleen sample, two technical replicates were adopted. In total, 48 GC-MS data sets were generated. After data processing, 56 metabolites with different abundances were identified, followed by categorization into carbohydrates (35%), lipids (27%), amino acids (24%), nucleotides (7%), and others (7%) (Figure 2A). Among the 56 metabolites, 33 metabolites were reduced in the C. carassius grown at 30°C. Interestingly, the abundance of the metabolites belonging to the category of lipids were all decreased in fish cultured at 30°C (Figure 2B). A heat map of the metabolites of different abundances was generated and was shown in Figure 2C. Z-score varied between −17 and 24 at 30°C as compared to 20°C. The abundance of 17 metabolites were increased and 33 metabolites were decreased from samples in fish grown at 30°C (Figure 2D). These data suggest that C. carassius mounts metabolic shift when cultured at different temperatures.
Figure 2. C. carassius cultured at 20 and 30°C had a different metabolism. (A) Categories of the different metabolites. Fifty six metabolites with different abundance were searched against in KEGG for their categories, and the pie chart was generated in Excel 2010 (Microsoft, USA). (B) The number of metabolites of different abundance in each category as shown in (A). (C) Heat map of unsupervised hierarchical clustering of different metabolites (row). Yellow and blue indicate increase and decrease of the metabolites scaled to mean and standard deviation of row metabolite level, respectively, (see color scale) (D) Z scores (standard deviation from average) of metabolites identified from 30 to 20°C, which are corresponding to the data shown in (C). Each point represents one technical repeat of metabolite.
Enriched metabolic pathways are crucial for the understanding of the key events during metabolic shift (43). Thus, the identified 56 metabolites with different abundance were analyzed by pathway enrichment analysis using an online software (http://www.metaboanalyst.ca). Thirteen metabolic pathways were identified. Valine, leucine, and isoleucine biosynthesis was the pathway mostly affected, followed by glycine, serine and threonine metabolism, alanine, aspartate and glutamate metabolism, methane metabolism, arginine and proline metabolism, taurine and hypotaurine metabolism, and TCA cycle. (Figure 3A). Among the 13 enriched metabolic pathways, three pathways, namely, taurine and hypotaurine metabolism, TCA cycle, and biosynthesis of unsaturated fatty acids, were of special interest because the abundance of metabolites in taurine and hypotaruine metabolism and biosynthesis of unsaturated fatty acids were all decreased, while the abundance of metabolites in the TCA cycle was increased in fish grown at 30°C (Figure 3B). These results indicate that C. carassius compromises taurine and hypotaurine metabolism and biosynthesis of unsaturated fatty acids but boosts the TCA cycle to cope with the temperature stress. This adaptation may make the fish more susceptible to bacterial infection.
Figure 3. Pathway enrichment analysis of metabolites with different abundance. (A) Pathway enrichment analysis of different metabolites. Metabolites demonstrating different abundance were analyzed by pathway enrichment analysis using online software (http://www.metaboanalyst.ca). Significant enriched pathways are selected to plot (p < 0.05). (B) Abundance of metabolites in the enriched pathways listed in (A). The metabolites in fish grown at 30°C were compared to that at 20°C. Different metabolites highlighted in yellow and blue indicate increased and decreased abundance, respectively.
To identify the crucial metabolites cultured at 20 and 30°C, orthogonal partial least square discriminant analysis (OPLS-DA) was conducted to recognize the sample patterns. The two groups were distributed in two quarters. Component t[1] separated 30°C group from 20°C group (Figure 4A) and Component t[1] differentiated variability within groups. Discriminating variables were shown as S-plot (Figure 4B) where cut-off values were set as grater or equal to 0.05 and 0.5 for absolute value of covariance p and correlation p(corr), respectively. Nineteen biomarkers screened by component t[1] were shown in Figure 4B (font in red). Among the 19 metabolites, the abundance of 9 metabolites were decreased in the group grown at 20°C, in which taurine, threonine, and palmitic acid showed the most significant difference. Taurine and palmitic acid are important components belonging to the taurine and hypotaurine metabolism and the biosynthesis of unsaturated fatty acids, respectively. Threonine belongs to the pathway with the second most impact (Figure 3A). Thus, taurine, palmitic acid, and threonine were the crucial biomarkers that distinguish the group cultured at 20 or 30°C. The scatter map of taurine, threonine, and palmitic acid were shown in Figure 4C.
Figure 4. Identification of crucial biomarkers. (A) The PCA analysis of metabolites with difference abundance in fish grown at the 30 and 20°C to investigate intergroup difference. Each dot represents the technique replicates in the plot. The metabolites with difference abundance in fish grown at 30 and 20°C were separated by the independent factors t[1]. (B) S-plot, generated by OPLS-DA, to identify different metabolites of intragroup as from t[1] in (A). Each triangle represents individual metabolite. The potential biomarkers are highlighted in red for metabolites whose p value is ≥0.05 and 0.5 for absolute value of covariance p and correlation p (corr), respectively. (C) Crucial biomarkers represent the metabolism of fish grown between 30 and 20°C. The abundance of the three crucial biomarkers, taurine, threonine, and palmitic acid, are compared between 20 and 30°C and presented as scatter plot. Each dot represents individual replicate. *p < 0.05; **p < 0.01.
We constructed the metabolic pathways affected by the water temperature in Figure 5A, where metabolites with increased and decreased abundance are highlighted in red and blue, respectively. Our results showed that the abundances of all the identified metabolites of the TCA were increased (Figures 3B, 5A), implying that TCA cycle may play important roles in addition to taurine, threonine, and palmitic acid. To explore this possibility, we measured the activity of the enzymes of the TCA cycle, including pyruvate dehydrogenase (PDH), succinate dehydrogenase (SDH), α-ketoglutarate dehydrogenase (α-KGDH), and malic dehydrogenase (MDH). As shown in Figure 5B, the activities of PDH, SDH, α-KGDH, and MDH were higher in 30°C than those in 20°C: 7.18 ± 1.01 U/mg vs. 9.66 ± 1.34 U/mg for PDH; 25.99 ± 3.86 U/mg vs. 35.73 ± 1.86 U/mg for SDH; 8.27 ± 1.11 U/mg vs. 11.41 ± 1.14 U/mg for α-KGDH; and 16.41 ± 2.03 U/mg vs. 21.55 ± 1.69 U/mg for MDH, respectively (the percentage change and statistical significance were listed in Table 1). The increased abundance of the key metabolites in the TCA cycle, including succinate, malate, citrate, and fumarate, indicates that TCA cycle is upregulated at 30°C as to 20°C.
Figure 5. Metabolic network of metabolites of different abundance, and activity of the TCA cycle. (A) Integrated metabolic network in relation to different metabolites. Different metabolites are searched against KEGG, and the metabolic network was reconstructed with ChemDraw Pro 18. The red and blue stand for the increased and decreased metabolites in fish grown at 30°C, respectively. (B)The enzymatic activity of PDH, α-KGDH, SDH, and MDH of spleen from fish grown at 30 and 20°C were quantified. Spleens were removed, lysed and extracted for enzyme analysis, and the data were shown as histogram. The significant differences are analyzed by non-parametric Kruskal-Wallis one-way analysis with Dunn multiple comparison post hoc test. **p < 0.01 indicates statistic significant.
We hypothesized that the increase of taurine, threonine, and palmitic acid in C. carassius may promote fish survival of bacterial challenge at 30°C. To test this, fish was intraperitoneally injected with taurine (50, 100, and 200 μg), threonine (125, 250, and 500 μg), or palmitic acid (3.5, 7, and 14 μg), followed by the challenge with E. tarda. Our results showed that the three metabolites had a protective effect on bacterial infection in a dose-dependent manner (Figure 6A).
Figure 6. Crucial biomarkers modulate the metabolism of crucian carps and promote their survival against bacterial infections. (A) The survival of crucian carps in the presence of crucial biomarkers upon E. tarda infection. C. carassius was treated with saline control or different dose crucial biomarkers at 30°C for 3 days, followed by bacterial challenge through intraperitoneal injection (1 × 105 CFU). The accumulative fish death was monitored for a total of 15 days' post-infection (n = 30 per group). (B) Activity of PDH, alpha-KGDH, SDH, and MDH of spleen in the presence of crucial biomarkers (200 μg taurine, 500 μg threonine, or 14 μg palmitic acid plus 10% BSA). Values are means ± SEM(n = 6 per group), and statistic difference is analyzed with non-parametric Kruskal-Wallis one-way analysis with Dunn multiple comparison post hoc test. **p < 0.01. (C) Percent survival of crucial carps in the presence of malonate. C. carassius was treated with malonate at different doses for 12 h followed by E. tarda challenge through intraperitoneal injection (1 × 105 CFU). The accumulative fish death was monitored for a total of 15 days post-infection (n = 30 per group).
To further address the metabolic basis of how the three metabolites protect fish against bacterial infection, we measured the enzymatic activities of PDH, SDH, α-KGDH, MDH in the TCA cycle after metabolite administration. Exogenous administration of taurine and palmitic acid significantly reduced the enzymatic activity of PDH in fish (from 6.18 ± 0.25 U/mg to 5.01 ± 0.52 U/mg by taurine; from 6.41 ± 0.63 U/mg to 4.87 ± 0.52 U/mg by palmitic acid), α-KGDH (from 7.05 ± 0.17 U/mg to 4.81 ± 0.72 U/mg by taurine; from 6.09 ± 0.57 U/mg to 2.51 ± 0.31 U/mg by palmitic acid), SDH (from 28.56 ± 2.00 U/mg to 16.91 ± 1.75 U/mg by taurine; from 30.80 ± 0.52 U/mg to 12.90 ± 0.79 U/mg by palmitic acid), and MDH (from 19.29 ± 0.95 U/mg to 15.0 ± 1.15 U/mg by taurine; from 18.04 ± 0.87 U/mg to 13.38 ± 0.81 U/mg by palmitic acid) (Figure 6B; Tables 2, 3). While exogenous threonine decreased the activity of α-KGDH from 7.05 ± 0.17 to 5.73 ± 0.61, and of SDH from 28.56 ± 2.00 to 21.77 ± 1.89 U/mg, but had no effect on the activities of PDH and MDH (Figure 6B; Table 4). These results suggested that exogenous taurine and palmitic acid may downregulate TCA cycle to promote C. carassius survival from E. tarda infection at 30°C. To further validate the role of attenuated TCA cycle in fish survival, we treated C. carassius with a low concentration of malonate, an inhibitor of SDH, and found that fish survival was increased in 20% (Figure 6C). Therefore, the attenuation of the TCA cycle by taurine and palmitic acid, threonine or inhibitors of SDH promotes fish survival from bacterial infection at 30°C.
Table 3. Enzyme activity of PDH, α-KGDH, SDH, and MDH of spleen at 30°C in the presence of palmitic acid.
To investigate whether the crucial biomarkers exert their effects through adjusting the fish immunity, we quantified the transcriptional level of 13 innate immune genes, including TNF-α1, TNF-α2, IL-1β1, IL-1β2, lysozyme, nfkbiab, IL-11, TLR9, TLR2, IFN-γ1-1, IFN-γ1-2, and complement component C3. Interestingly, the transcriptional level of TNF-α1, TNF-α2, IL-1β1, IL-1β2, and lysozyme were decreased when C. carassius was cultured at 30°C, but they can be quickly increased upon treatment with taurine, palmitic acid, and threonine (Figures 7A,B). The transcriptional expressions of TLR2, IFN-γ1-1, IFN-γ1-2, and C3 were also further boosted when the metabolites were supplemented (Figures 7A,B). Furthermore, all of these three metabolites downregulated the expression of IL-11 (Figures 7A,B). These results indicated that the three crucial biomarkers regulate innate immune response to fight against the infection at 30°C.
Figure 7. Crucial biomarkers modulate the innate immune responses of fish at 30°C. (A,B) qRT-PCR for cytokine genes of C. carassius treated with control (saline or 10% BSA) or crucial biomarkers (200 μg taurine, 500 μg threonine, or 14 μg palmitic acid plus 10% BSA) for 3 days following E. tarda challenge through intraperitoneal injection (1 × 103 CFU). The spleens were collected 12 h post-injection for RNA extraction and qRT-PCR. Values are means ± SEM from six biological replicates. *p < 0.05; **p < 0.01.
Environmental temperature affects fish host immune responses to microbial infection (44). Several endemic diseases of salmonids are more prevalent and difficult to control with the increase of water temperature (44). One reason is that the increase in water temperatures are likely to enhance the susceptibility of fish to disease and the likelihood of disease emergence (44). That temperature shift alters the immune status of Cyprinus carpio advanced fingerlings, while persistent sub-lethal exposure to chlorine augments temperature induced immunosuppression (26). Multiple acute temperature stress affects leucocyte populations and antibody responses in the common carp, Cyprinus carpio L. (27). Water temperature affects metabolism and metabolome in fish (39, 45). L-Proline, a crucial biomarker identified from temperature shift, increased the survival of tilapias infected by Streptococcus agalactiae in high water temperature (39). Our results further demonstrated that water temperature alters crucian carp metabolism, orchestrating innate immunity to cope with bacterial infection. Insights gained on the metabolome-mediated mechanisms may provide new therapeutic methods for the treatment of infections or preventive measures against the future outbreaks of bacterial infection.
The first core finding in the present study showed that high temperature disrupts the normal flow of the TCA cycle, making the host vulnerable to bacterial infection. The activated TCA cycle is a characteristic metabolic feature at high temperature as confirmed by the elevated activities of enzymes in pyruvate metabolism and the TCA cycle. More importantly, the inhibition of the TCA cycle promoted fish survival during bacterial infection. Therefore, the enhanced TCA cycle is critical for crucian carps to survive at higher water temperature.
The second core finding shows that the crucial biomarkers not only promote the survival of crucian carps cultured at 30°C, but also rescue the expression of innate immune genes that are repressed at 30°C. Specifically, palmitic acid, threonine, and taurine restored the expressions of TNF-α1, TNF-α2, IL-1β1, IL-1β2, and lysozyme, which were reduced at 30°C. In addition, nfkbiab, TLR9, TLR3, IL-11, and C3 were also regulated by the three metabolites. Therefore, our data provide direct evidence that these crucial metabolites, identified from water temperature-mediated metabolomes, regulate innate immune response. Among the genes of innate immunity, only 13 genes were selected as they are the only genes available for crucian carps in GeneBank, categorized to B-Jellyroll cytokines (TNF-α1 and TNF -α2), β-Trefoil cytokines (IL-1β1 and IL-1β2), Type I α helical cytokines (IL-11), Type II α helical cytokines (IFN-γ1-1 and IFN-γ1-2), TLR family (TLR 2, TLR 3, and TLR 9), lysozyme, complement system (C3), and nfkbiab (46). The function of B-Jellyroll cytokines, also called the TNF superfamily cytokines in fish, is similar to their mammalian counterparts that are expressed at early stage of bacterial infection (47). TNF-α plays a critical role in regulating inflammation by promoting phagocytosis, increasing the expression of immune genes and generation of bacterial-killing reactive oxygen species, and leukocyte homing, proliferation and migration in fish like trout (47–49). IL-1β, which belongs to the β-Trefoil cytokines, was the first interleukin identified in boy and cartilaginous fish (50). It is usually produced once the cells are activated by pattern recognition receptors (51). IL-1β plays a diverse pro-inflammatory roles that increases the number of phagocytes, phagocytic, and lysozyme activity, modulating the IL-17 family for antimicrobial activity and enhancing antibody production when co-administrated with bacterial vaccine (52–56). The TLR family are conserved cell membrane receptors that recognize pathogen-associated molecular pattern or damage-associated molecular patterns, and they function in fish species to initiate an immune response (57–59). The complement system and lysozyme are innate molecules to target bacterial cells for cell lysis by disrupting the cell membrane. They mount a non-specific immune response and represent maternal immunity from fish embryo stage, and thus are important for fish survival during bacterial infection (60). Therefore, the innate immune response was compromised by crucian carp at high temperature, but it can be rescued through metabolic reprogramming.
In addition, palmitate, threonine, and taurine protect crucian carps from E. tarda infection at 30°C, possibly by inhibition of the TCA cycle. More importantly, the three metabolites exhibit similar impacts on the TCA cycle and innate immune responses. These results suggest that the TCA cycle related to the innate immunity.
In summary, the present study identifies important metabolites that could promote the fish survival upon bacterial infection at 30°C, a higher water temperature than their optimum water temperature. We showed that crucian carps grown at 30°C demonstrated a metabolome that is characterized by the enhanced TCA cycle but a reduced abundance of palmitate, threonine, and taurine. Importantly, exogenous palmitate, threonine, and taurine slightly inhibit the TCA cycle and restore the altered innate immune responses repressed at the higher temperature. These results indicate that higher water temperature reduces the survival of crucian carps via metabolism, which can be reprogramed through crucial biomarkers.
This study was conducted in accordance with the recommendations in the Guide for the Care and Use of Laboratory Animals of the National Institutes of Health and maintained according to the standard protocols. All experiments were approved by the Institutional Animal Care and Use Committee of Sun Yat-sen University (Animal welfare Assurance Number: 16).
Edwardsiedlla tarda EIB202 is a virulent strain isolated from the outbreak of farmed turbot and is a generous gift from Dr. Yuanxin Zhang (61). EIB202 was grown in Luria-Bertani (LB) broth or 1% LB agar plate.
Crucian carp, C. carassius, (body length: 3 ± 0.5 cm, body weight: 2 ± 0.2 g) was obtained from Crucian Carp Breeding Corporation (Guangzhou, P.R. China). C. carassius was free of Edwardsiedlla infection through microbiological detection. C. carassius was normally reared in 50 L water tanks equipped with Closed Recirculating Aquaculture Systems, and the maintaining physico-chemical parameters were: dissolved oxygen: 6–7 mg/L, carbon dioxide content: <10 mg/L, pH value: 7.0–7.5, nitrogen content: 1–2 mg/L, and nitrite content: 0.1–0.3 mg/L. These animals were cultured under this condition for 2 weeks before experimental manipulation and were fed twice daily with commercial fish feed (38% crude protein, 6% crude fat, and 16% crude ash related to wet matter, 7% crude fiber and 8% moisture, based on NRC recommendations, at a ratio of 3% of body weight per day) on a 12 h/12 h rhythm of light and darkness photoperiod always. The tank was cleaned once a day by siphoning up the food debris and feces. To accommodate C. carassius to different temperatures, the water temperature was gradually changed until the fish were adapted to constant temperature at 20 or 30°C. The water quality was monitored regularly.
To prepare E. tarda EIB202 for bacterial infection, a single colony was picked up from agar plate and grown in LB medium overnight. The overnight culture was diluted into fresh LB medium at 1:100 and grown with shaking at 200 rpm at 30°C until OD600 of the culture reached 1.0. Cells were pelleted by centrifugation and washed with 0.85% saline solution three times and suspended in saline solution.
For bacterial infection, each C. carassius cultured at 20 or 30°C was intraperitoneally injected with 10 μl 1 × 105 CFU bacterial suspension or saline only (n = 30 for each treatment). These crucian carps were observed for symptoms twice daily for 15 days for accumulative death.
Sample preparation was carried out as described previously (32). C. carassius cultured at 20 or 30°C were euthanized in ice slush (5 parts ice/1 part water, 0–4°C) for at least 10 min following cessation of gill movement, and left in the ice water for a total of 20 min after cessation of all movement to ensure death by hypoxia following the guidelines of NIH. C. carassius were rinsed with distilled water and then wiped thoroughly with sterilized gauze. Spleens were removed ascetically, where 25 mg of spleens were cut and immensed immediately in 1 mL cold methanol. Then, the samples were sonicated for 5 min at 10W-power setting at Ultrasonic Processor (JY92-IIDN, SCIENTZ), followed by centrifugation at 12,000 × g in 4°C for 10 min. The supernatant was collected and 10 μl 0.1 mg/ml ribitol (Sigma) was added as the internal standard. The supernatant was concentrated in a rotary vacuum centrifuge device (LABCONCO). The dried polar extracts were incubated with 80 μl methoxy amination hydrochloride (20 mg/ml pyridine) for 90 min at 37°C, followed by an addition of 80 μl N-methyl-N-trimethylsilyltrifluoroacetamide (MSTFA) (Sigma) and incubated for another 30 min at 37°C. Finally, the resulted samples were cooled down to room temperature prior to mass spectrometry analysis.
GC-MS analysis was carried out with a variation on the two-stage techniques as described previously (32). In brief, 1 μL derivatized sample was injected into a DBS-MS column using splitless injection, and analysis was carried out by Agilent 7890A GC equipped with an Agilent 5975C VL MSD detector (Agilent Technologies). The initial temperature of the GC oven was held at 85°C for 5 min followed by an increase to 270°C at a rate of 15°C min−1 then held for 5 min. Helium was used as carrier gas and the flow was kept at 1 mL min−1 constantly. The MS was operated in a range of 50–600 m/z. For each sample, two technical replicates were prepared to confirm the reproducibility of the reported procedures.
C. carassius (n = 330) was randomly divided into five groups, including three metabolite groups (n = 90 per group), and two control groups (n = 30 per group), and acclimatized for 7 days at 30°C. For the three metabolites groups, C. carassius was intraperitoneally injected with taurine (Sigma) at three doses (50, 100, or 200 μg per fish; n = 30 for each dose), threonine (Sigma) at three doses (125, 250, or 500 μg per fish; n = 30 for each dose), or palmitic acid (Sigma) at three doses (3.5, 7.0, or 14.0 μg per fish; n = 30 for each dose). For the two control groups, they were either injected with saline only (n = 30) as a control to taurine and threonine groups, or with 10% bovine serum albumin (Sigma) as a control to palmitic acid group. The injection was conducted once daily for 3 days. Afterwards, C. carassius was challenged by intraperitoneal injection of E. tarda EIB202 with a dose of 10 μl 1 × 105 CFU/fish, and the fish mortality was observed for 15 days for accumulative death.
To quantify the transcriptional level of innate immune genes by qRT-PCR, C. carassius was intraperitoneally injected with taurine (200 μg per fish; n = 20), threonine (500 μg per fish; n = 20), or palmitic acid (14 μg per fish; n = 20) once daily for 3 days. Then, C. carassius were challenged with E. tarda EIB202 with a dose of 10 μl 1 × 103 CFU per fish. The spleens were aseptically removed and collected 12 h post-infection as stated above for RNA isolation.
Enzyme activities were measured as described previously (37, 62). In brief, spleens freshly removed from fish were rinsed with pre-cooled 1 × PBS, resuspended in lysate buffer, and disrupted by sonication for 5 mins at 10W power setting. Following centrifugation, the supernatant, defined as the total protein, was transferred to a new tube, and the protein concentration in the supernatant was determined by Bradford assay. The total proteins of 100 μg were applied for pyruvate dehydrogenase or α-ketoglutarate dehydrogenase assay, and 250 μg of total proteins for succinate dehydrogenase or malate dehydrogenase assays. The enzymatic assay was conducted by mixing an equal volume of the protein sample and reaction buffer to a final volume of 200 μL in 96-well plate. The plate was further incubated at 37°C for 15 min, and the absorbance was read at 570 nm on a microplate reader. The enzyme activity was calculated by plotting against the standard curve. The reaction mixtures are as follows: PDH reaction mixture: 0.5 mM 3-(4,5-di methyl thiazol-2-yl)-2,5-diphenyltetrazolium bromide (MTT) (Sangon Biotech), 1 mM MgCl2 (Sigma), 6.5 mM phenazine methosulfate (PMS) (Sangon Biotech), 0.2 mM thiamine pyrophosphate (TPP) (Sangon Biotech), and 2 mM sodium pyruvate (Sigma) and 50 mM phosphate buffered saline (PBS) (Invitrogen); KGDH reaction mixture: 0.5 mM MTT, 1 mM MgCl2, 6.5 mM PMS, 0.2 mM TPP, 2 mM α-ketoglutaric acid sodium salt (Sigma) and 50 mM PBS; SDH reaction mixture: 0.5 mM MTT, 13 mM PMS, 5 mM sodium succinate (Sigma), and 50 mM PBS; MDH reaction mixture: 0.5 mM MTT, 13 mM PMS, 50 mM malic acid disodium salt (Sigma), and 50 mM PBS.
Total RNA was isolated with Trizol (Invitrogen, USA) and quantified by Nanodrop (Thermo Scientific). Reverse transcription-PCR (qRT-PCR) was carried out using Prime-ScriptTM RT reagent Kit with gDNA eraser (Takara, Japan) with 1 μg of total RNA according to manufacturer's instructions. The experiment was performed in six biological replicates.
qRT-PCR was performed with a LightCycler-480 (Roche, Germany) using SYBR Premix Ex Taq II Kits (Takara, Japan) following the manufacture's instruction. The experiment was performed in six biological replicates. Primers for each gene were listed in Table 5, and each primer pair was specific. Actin, tubulin and GAPDH genes were chosen as the internal control. The relative expression of each gene was determined by comparative threshold cycle method (2−ΔΔCT method).
For the GC-MS data analysis, metabolites from the GC-MS spectra were identified by searching against National Institute of Standards and Technology (NIST) library used the software of NIST MS search 2.0. The resulting data matrix was normalized using the concentrations of added internal standards, which were subsequently removed so that the data could be used for modeling consisted of the extracted compound. Peak areas of all identified metabolites were normalized by ribitol as internal standard. Statistical difference of metabolites in each sample was obtained by Kruskal–Wallis test and Mann–Whitney test using SPSS 23.0 (IBM Corp, USA). Z-score and hierarchical clustering were used to analyze the normalization area. Hierarchical clustering was completed in the R platform (https://cran.r-project.org). Multivariate statistical analysis included principal component analysis (PCA) and orthogonal partial least square discriminant analysis (OPLS-DA) implemented with SIMCA 12.0 (Umetrics, Umeå, Sweden).
For the data analysis of qRT-PCR and enzyme activity, non-parametric Kruskal-Wallis one-way analysis with Dunn multiple comparison post hoc test was used, SPSS 23.0, p < 0.05 was considered significant.
All datasets generated for this study are included in the manuscript and/or the Supplementary Files.
This study was conducted in accordance with the recommendations in the Guide for the Care and Use of Laboratory Animals of the National Institutes of Health and maintained according to the standard protocols. All experiments were approved by the Institutional Animal Care and Use Committee of Sun Yat-sen University (Animal welfare Assurance Number: 16).
BP conceptualized and designed the project and wrote the manuscript. MJ and ZC performed the experiments. MJ and JZ performed the data analysis. BP, MJ, ZC, and JZ interpreted the data. All the authors reviewed the manuscript.
This work was sponsored by grants from the National Key R&D Research program of China (2016YFD0501307), NSFC project (31822058, 31672656, 31872602), and the Fundamental Research Funds for the Central Universities (18lgzd14).
The authors declare that the research was conducted in the absence of any commercial or financial relationships that could be construed as a potential conflict of interest.
The Supplementary Material for this article can be found online at: https://www.frontiersin.org/articles/10.3389/fimmu.2019.01991/full#supplementary-material
2. Shapiro RS, Cowen LE. Thermo control of microbial development and virulence: molecular mechanism of microbial temperature sensing. mBio. (2012) 3:e00238-12. doi: 10.1128/mBio.00238-12
3. Hu YH, Zhou HZ, Jin QW, Zhang J. The serine protease autotransporter Tsh contributes to the virulence of Edwarsiella tarda. Vet Microbiol. (2016) 189:68–74. doi: 10.1016/j.vetmic.2016.04.021
4. Awan F, Dong Y, Wang N, Liu J, Ma K, Liu Y. The fight for invincibility: environmental stress response mechanism and Aeromonas hydrophila. Microb Pathog. (2018) 116:135–45. doi: 10.1016/j.micpath.2018.01.023
5. Huang L, Guo L, Xu L, Qin Y, Zhao L, Su Y, et al. The role of rpoS in the regulation of Vibrio alginolyticus virulence and the response to diverse stresses. J Fish Dis. (2019) 42:703–12. doi: 10.1111/jfd.12972
6. Yao Z, Guo Z, Wang Y, Li W, Fu Y, Lin Y, et al. Integrated succinylome and metabolome profiling reveals crucial role of S-ribosylhomocystein lysase in quorum sensing and metabolism of Aeromonas hydrophila. Mol Cell Proteomics. (2019) 18:200–15. doi: 10.1074/mcp.RA118.001035
7. Avtalion RR, Willian Clem L. Environmental control of the immune response in fish. Crit Rev Environ Sci Tech. (1981) 11:163–88. doi: 10.1080/10643388109381687
8. Greenspan SE, Bower DS, Roznik EA, Pike DA, Marantelli G, Alford RA, et al. Infection increases vulnerability to climate change via effects on host thermal tolerance. Sci Rep. (2017) 7:9349. doi: 10.1038/s41598-017-09950-3
9. Hector TE, Sqro CM, Hall MD. Pathogen exposure disrupts an organism's ability to cope with thermal stress. Glob Change Biol. (2019) 1–13. doi: 10.1111/gcb.14713
10. Wang Y, Wang X, Ali F, Li Z, Fu Y, Yang X, et al. Comparative extracellular proteomics of Aeromonas hydrophila reveals iron-regulated secreted proteins as potential vaccine candidates. Front Immunol. (2019) 10:256. doi: 10.3389/fimmu.2019.00256
11. Liao XL, Cheng L, Xu P, Lu GQ, Wachholtz M, Sun XW, et al. Transcriptome analysis of crucian carp (Carassius auratus), an important aquaculture and hypoxia-tolerant species. PLoS ONE. (2013) 8:e62308. doi: 10.1371/journal.pone.0062308
12. Sollid J, Weber RE, Nilsson GE. Temperature alters the respiratory surface area of crucian carp Carassius carassius and goldfish Carassius auratus. J Exp Biol. (2005) 208:1109–16. doi: 10.1242/jeb.01505
13. Rhee JS, Jeong CB, Kim DH, Kim IIC, Lee YS, Lee CL, et al. (2014). Immune gene discovery in the crucian carp Carassius auratus. Fish Shellfish Immunol. 36:240–51. doi: 10.1016/j.fsi.2013.11.009
14. Liu XJ, Zhu WC, Su YB, Guo C, Zeng ZH, Li H, et al. Characterization of ampicillin-stressed proteomics and development of a direct method for detecting drug-binding proteins in Edwardsiella tarda. J Proteomics. (2015) 116:97–105. doi: 10.1016/j.jprot.2014.12.018
15. Ling SH, Wang XH, Xie L, Lim TM, Leung KY. Use of green fluorescent protein (GFP) to study the invasion pathways of Edwardsiella tarda in in vivo and in vitro fish models. Microbiology. (2000) 146:7–19. doi: 10.1099/00221287-146-1-7
16. Xu T, Zhang XH. Edwardsiella tarda: an intriguing problem in aquaculture. Aquaculture. (2014) 431:129–35. doi: 10.1016/j.aquaculture.2013.12.001
17. Li Z, Liu X, Liu J, Zhang K, Yu H, He Y, et al. Transcriptome profiling based on protein-protein interaction networks provides a core set of genes for understanding blood immune response mechanisms against Edwardsiella tarda infection in Japanese flounder (Paralichthys olivaceus). Dev Comp Immunol. (2018) 78:100–13. doi: 10.1016/j.dci.2017.09.013
18. Katharios P, Kalatzis PG, Kokkari C, Pavlidis M, Wang Q. Characterization of a highly virulent Edwardsiella anguillarum strain isolated from Greek aquaculture, and a spontaneously induced prophage therein. Front Microbiol. (2019) 10:141. doi: 10.3389/fmicb.2019.00141
19. Meyer FP, Bullock GL. Edwardsiella tarda, a new pathogen of channel catfish (Ictalurus punctatus). Appl Microbiol. (1973) 25:155–6.
20. Miyazaki T, Kaige N. Comparative histopathology of edwardsiellosis in fishes. Fish Pathol. (1985) 20:219–27. doi: 10.3147/jsfp.20.219
21. John AM, Prakash JA, Simon EG, Thomas N. Edwardsiella tarda sepsis with multiple liver abscesses in a patient with Cushing's syndrome. Indian J Med Microbiol. (2012) 30:352–4. doi: 10.4103/0255-0857.99503
22. Miniero DY, Xavier de Oliverir MG, Paulo Vieira Cunha M, Soares Franco L, Pulecio Santos SL, Zanolli Moreno L, et al. Edwardsiella tarda outbreak affecting fishes and aquatic birds in Brazil. Vet Q. (2018) 38:99–105. doi: 10.1080/01652176.2018.1540070
23. Woo PTK, Bruno DW. Fish diseases and disorders. Volume 3, viral, bacterial and fungal infections. In: Evans JJ, Klesius PH, Plumb JA, Shoemaker CA, editors. Edwardsiella septicaemias. 2nd ed. Wallingford: CABI International (2010). p. 512–534.
24. Park SB, Aoki T, Jung TS. Pathogenesis of and strategies for preventing Edwardsiella tarda infection in fish. Vet Res. (2012) 43:67. doi: 10.1186/1297-9716-43-67
25. Kim JH, Park HJ, Kim KW, Hwang IK, Kim DH, Oh CW, et al. Growth performance, oxidative stress, and non-specific immune responses in juvenile sablefish, Anoplopoma fimbria, by changes of water temperature and salinity. Fish Physiol Biochem. (2017) 43:1421–31. doi: 10.1007/s10695-017-0382-z
26. Verma AK, Pal AK, Manush SH, Das T, Dalvi RS, Chandrachoodan PP, et al. Persistent sub-lethal chlorine exposure augments temperature induced immunosuppression in Cyprinus carpio advanced fingerlings. Fish Shellfish Immunol. (2007) 22:547–55. doi: 10.1016/j.fsi.2006.08.001
27. Engelsma MY, Hougee S, Nap D, Hofenk M, Rombout JH, van Muiswinkel WB, et al. Multiple acute temperature stress affects leucocyte populations and antibody responses in common carp, Cyprinus carpio L. Fish Shellfish Immunol. (2003) 15:397–410. doi: 10.1016/S1050-4648(03)00006-8
28. Le Morvan C, Deschaux P, Troutaud D. Effects and mechanisms of environmental temperature on carp (Cyprinus carpio)anti-DNP antibody response and non-specific cytotoxic cell activity: a kinetic study. Dev Comp Immunol. (1996) 20:331–40. doi: 10.1016/S0145-305X(96)00027-4
29. Zhao XL, Wu CW, Peng XX, Li H. Interferon-α2b against microbes through promoting biosynthesis of unsaturated fatty acids. J Proteome Res. (2014) 13:4155–63. doi: 10.1021/pr500592x
30. Chen XH, Liu SR, Peng B, Li D, Cheng ZX, Zhu JX, et al. Exogenous L-valine promotes phagocytosis to kill multidrug-resistant bacterial pathogens. Front Immunol. (2017) 8:207. doi: 10.3389/fimmu.2017.00207
31. Zeng ZH, Du CC, Liu SR, Li H, Peng XX, Peng B. Glucose enhances tilapia against Edwardsiella tarda infection through metabolome reprogramming. Fish Shellfish Immunol. (2017) 61:34–43. doi: 10.1016/j.fsi.2016.12.010
32. Jiang M, Gong QY, Lai SS, Cheng ZX, Chen ZG, Zheng J, et al. Phenylalanine enhances innate immune response to clear ceftazidime-resistant Vibrio alginolyticus in Danio rerio. Fish Shellfish Immunol. (2019) 84:912–9. doi: 10.1016/j.fsi.2018.10.071
33. Peng B, Su YB, Li H, Han Y, Guo C, Tian YM, et al. Exogenous alanine or/and glucose plus kanamycin kills antibiotic-resistant bacteria. Cell Metab. (2015) 21:249–61. doi: 10.1016/j.cmet.2015.01.008
34. Su YB, Peng B, Han Y, Li H, Peng XX. Fructose restores susceptibility of multidrug-resistant Edwardsiella tarda to kanamycin. J Proteome Res. (2015) 14:1612–20. doi: 10.1021/pr501285f
35. Cheng ZX, Ma YM, Li H, Peng XX. N-acetylglucosamine enhances survival ability of tilapias infected by Streptococcus iniae. Fish Shellfish Immunol. (2014) 40:524–30. doi: 10.1016/j.fsi.2014.08.008
36. Su YB, Peng B, Li H, Cheng ZX, Zhang TT, Zhu JX, et al. The pyruvate cycle increases aminoglycosides efficacy and provides respiratory energy in bacteria. Proc Natl Acad Sci USA. (2018) 115:E1578–87. doi: 10.1073/pnas.1714645115
37. Yang J, Zeng ZH, Yang MJ, Cheng ZX, Peng XX, Li H. NaCl promotes antibiotic resistance by reducing redox states in Vibrio alginolyticus. Environ Microbiol. (2018) 20:4022–36. doi: 10.1111/1462-2920.14443
38. Liu SR, Peng XX, Li H. Metabolic mechanism of ceftazidime resistance in Vibrio alginolyticus. Infect Drug Resist. (2019) 12:417–29. doi: 10.2147/IDR.S179639
39. Zhao XL, Han Y, Ren ST, Ma YM, Li H, Peng XX. L-proline increases survival of tilapias infected by Streptococcus agalactiae in higher water temperature. Fish Shellfish Immunol. (2015) 44:33–42. doi: 10.1016/j.fsi.2015.01.025
40. Ma YM, Yang MJ, Wang S, Li H, Peng XX. Liver functional metabolomics discloses an action of L-leucine against Streptococcus iniae infection in tilapias. Fish Shellfish Immunol. (2015) 45:414–21. doi: 10.1016/j.fsi.2015.04.037
41. Chen XH, Zhang BW, Li H, Peng XX. Myo-inositol improves the host's ability to eliminate balofloxacin-resistant Escherichia coli. Sci Rep. (2015) 5:10720. doi: 10.1038/srep10720
42. Wu CW, Zhao XL, Wu XJ, Wen C, Li H, Chen XH, et al. (2015). Exogenous glycine and serine promote growth and antifungal activity of Penicillium citrinum W1 from the south-west Indian Ocean. FEMS Microbiol. Letter. 362:fnv040. doi: 10.1093/femsle/fnv040
43. Maudsley S, Chadwick W, Wang L, Zhou Y, Martin B, Park SS. Bioinformatic approaches to metabolic pathway analysis. Methods Mol Biol. (2011) 756:99–130. doi: 10.1007/978-1-61779-160-4_5
44. Marcos-López M, Gale P, Oidtmann BC, Peeler EJ. Assessing the impact of climate change on disease emergence in freshwater fish in the United Kingdom. Transbound Emerg Dis. (2010) 57:293–304. doi: 10.1111/j.1865-1682.2010.01150.x
45. Gandar A, Laffaille P, Canlet C, Tremblay-Franco M, Gautier R, Perrault A, et al. Adaptive response under multiple stress exposure in fish: from the molecular to individual level. Chemosphere. (2017) 188:60–72. doi: 10.1016/j.chemosphere.2017.08.089
46. Zou J, Secombes CJ. The function of fish cytokines. Biology. (2016) 5:E23. doi: 10.3390/biology5020023
47. Zou J, Peddie S, Scapigliati G, Zhang Y, Bols NC, Ellis AE, et al. Functional characterization of the recombinant tumor necrosis factors in rainbow trout, Oncorhynchus mykiss. Dev Comp Immunol. (2003) 27:813–22. doi: 10.1016/S0145-305X(03)00077-6
48. Boecke A, Sieger D, Neacsu CD, Kashkar H, Krönke M. Factor associated with neutral sphingomyelinase activity mediates navigational capacity of leukocytes responding to wounds and infection: live imaging studies in zebrafish larvae. J Immunol. (2012) 189:1559–66. doi: 10.4049/jimmunol.1102207
49. Lu XJ, Chen Q, Yang GJ, Chen J. The TNFα converting enzyme (TACE) from ayu (Plecoglossus altivelis) exhibits TNFα shedding activity. Mol Immunol. (2015) 63:497–504. doi: 10.1016/j.molimm.2014.10.010
50. Bird S, Wang T, Zou J, Cunningham C, Secombes CJ. The first cytokine sequence within cartilaginous fish: IL-1 beta in the small spotted catshark (Scyliorhinus canicula). J Immunol. (2002) 168:3329–40. doi: 10.4049/jimmunol.168.7.3329
51. Vojtech LN, Scharping N, Woodson JC, Hansen JD. Roles of inflammatory caspases during processing of zebrafish interleukin-1β in Francisella noatunensis infection. Infect Immun. (2012) 80:2878–85. doi: 10.1128/IAI.00543-12
52. Hong S, Peddie S, Campos-Pérez JJ, Zou J, Secombes CJ. The effect of intraperitoneally administered recombinant IL-1beta on immune parameters and resistance to Aeromonas salmonicida in the rainbow trout (Oncorhynchus mykiss). Dev Comp Immunol. (2003) 27:801–12. doi: 10.1016/S0145-305X(03)00056-9
53. Wang X, Li C, Thongda W, Luo Y, Beck B, Peatman E. Characterization and mucosal responses of interleukin 17 family ligand and receptor genes in channel catfish Ictalurus punctatus. Fish Shellfish Immunol. (2014) 38:47–55. doi: 10.1016/j.fsi.2014.02.020
54. Taechavasonyoo A, Hirono I, Kondo H. The immune-adjuvant effect of Japanese flounder Paralichthys olivaceus IL-1β. Dev Comp Immunol. (2013) 41:564–8. doi: 10.1016/j.dci.2013.07.003
55. Yamasaki M, Araki K, Maruyoshi K, Matsumoto M, Nakayasu C, Moritomo T, et al. Comparative analysis of adaptive immune response after vaccine trials using live attenuated and formalin-killed cells of Edwardsiella tarda in ginbuna crucian carp (Carassius auratus langsdorfii). Fish Shellfish Immunol. (2015) 45:437–42. doi: 10.1016/j.fsi.2015.04.038
56. Cheng ZX, Chu X, Wang SN, Peng XX, Li H. Six genes of ompA family shuffling for development of polyvalent vaccines against Vibrio alginolyticus and Edwardsiella tarda. Fish Shellfish Immunol. (2018) 75:308–15. doi: 10.1016/j.fsi.2018.02.022
57. Matzinger P. The danger model: a renewed sense of self. Science. (2002) 296:301–5. doi: 10.1126/science.1071059
58. Medzhitov R. Recognition of microorganisms, activation of the immune response. Nature. (2007) 449:819–26. doi: 10.1038/nature06246
59. Rebl A, Goldammer T, Seyfert HM. Toll-like receptor signaling in body fish. Vet Immunol Immunopathol. (2010) 134:139–50. doi: 10.1016/j.vetimm.2009.09.021
60. Wang Z, Zhang S. The role of lysozyme and complement in the antibacterial activity of zebrafish (Danio rerio) egg cytosol. Fish Shellfish Immunol. (2010) 29:773–7. doi: 10.1016/j.fsi.2010.07.002
61. Wang QY, Yang MJ, Xiao JF, Wu HZ, Wang X, Lv YZ, et al. Genome sequence of the versatile fish pathogen Edwardsiella tarda provides insights into its adaptation to braod host ranges and intracellular niches. PLos ONE. (2009) 4:e7646. doi: 10.1371/journal.pone.0007646
Keywords: water temperature, bacterial infection, Carassius carassius, metabolome, innate immunity
Citation: Jiang M, Chen Z, Zheng J and Peng B (2019) Metabolites-Enabled Survival of Crucian Carps Infected by Edwardsiella tarda in High Water Temperature. Front. Immunol. 10:1991. doi: 10.3389/fimmu.2019.01991
Received: 30 April 2019; Accepted: 06 August 2019;
Published: 22 August 2019.
Edited by:
Carolina Tafalla, Instituto Nacional de Investigación y Tecnología Agraria y Alimentaria (INIA), SpainReviewed by:
Caroline Fossum, Swedish University of Agricultural Sciences, SwedenCopyright © 2019 Jiang, Chen, Zheng and Peng. This is an open-access article distributed under the terms of the Creative Commons Attribution License (CC BY). The use, distribution or reproduction in other forums is permitted, provided the original author(s) and the copyright owner(s) are credited and that the original publication in this journal is cited, in accordance with accepted academic practice. No use, distribution or reproduction is permitted which does not comply with these terms.
*Correspondence: Bo Peng, cGVuZ2IyNkBtYWlsLnN5c3UuZWR1LmNu
Disclaimer: All claims expressed in this article are solely those of the authors and do not necessarily represent those of their affiliated organizations, or those of the publisher, the editors and the reviewers. Any product that may be evaluated in this article or claim that may be made by its manufacturer is not guaranteed or endorsed by the publisher.
Research integrity at Frontiers
Learn more about the work of our research integrity team to safeguard the quality of each article we publish.