- Department of Pulmonary Medicine, Feinberg School of Medicine, Northwestern University, Chicago, IL, United States
Macrophages are often viewed through the lens of their core functions, but recent transcriptomic studies reveal them to be largely distinct across tissue types. While these differences appear to be shaped by their local environment, the key signals that drive these transcriptional differences remain unclear. Since Wnt signaling plays established roles in cell fate decisions, and tissue patterning during development and tissue repair after injury, we consider evidence that Wnt signals both target and are affected by macrophage functions. We propose that the Wnt gradients present in developing and adult tissues effectively shape macrophage fates and phenotypes. We also highlight evidence that macrophages, through an ability to dispatch Wnt signals, may couple tissue debridement and matrix remodeling with stem cell activation and tissue repair.
Introduction
Macrophages are present in virtually every tissue, playing crucial roles in homeostatic tissue maintenance and coordinating cellular responses to tissue injury [reviewed in (1, 2)]. Most tissues in the steady state contain diverse populations of so-called “tissue-resident” macrophages. These tissue-resident macrophages may differ in their ontogeny, ability to proliferate, and specific micro-niches within the organ. For example, some macrophages populate organs during early embryogenesis (e.g., microglia) or the early postnatal period (e.g., alveolar macrophages in the lung). These macrophages maintain their population via proliferation in situ, thus keeping the tissue micro-niche “occupied” and apparently “closed” to circulating monocytes (3). Circulating monocytes can enter and patrol these closed tissues, but without appropriate differentiation stimuli, fail to differentiate into macrophages, and instead exit via lymphatics (4). In contrast, tissue-resident macrophages in other tissues (e.g., gut, skin and heart) are relatively short-lived and require constant influx and differentiation of monocytes to maintain their population (5, 6). Regardless of these differences, once tissue-resident macrophages are depleted, such as after ionizing radiation or in response to tissue injury, circulating monocytes enter the tissue and differentiate into macrophages to repopulate the “open” niche. The fate of these recruited, monocyte-derived macrophages depends on both the tissue, as well as the type and extent of injury. These macrophages can disappear after injury resolution or adapt to the empty niche and become long-living resident cells by taking on epigenetic, transcriptomic, and functional features of tissue-resident macrophages seeded during development [reviewed in (3)] (Figure 1). Thus, while the local tissue environment is likely critical in shaping macrophage identity and functionality, the specific signals and transcriptional programs they direct remain poorly defined.
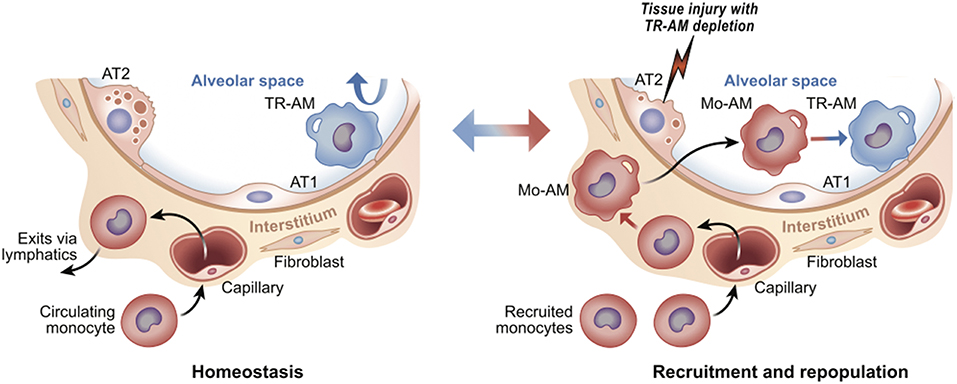
Figure 1. Tissue resident vs. recruited macrophages. Using lung as an example, tissue resident alveolar macrophages (TR-AM) self-renew to maintain surfactant homeostasis; monocytes are not recruited from the circulation (Left). After injury, TR-AMs are depleted and ultimately replaced by circulating monocytes (monocyte-derived alveolar macrophages, or Mo-AM) (Right). Upon injury, Mo-AMs are transcriptionally distinct from TR-AMs. Over time, Mo-AMs become transcriptionally indistinguishable from TR-AMs (7). The alveolar airway surface epithelium is lined by surfactant producing Alveolar Type 2 (AT2) and gas-exchange promoting Type 1 cells (AT1).
Wnt signaling regulates many developmental processes, including cell fate decisions, migration, and the overall spatial patterning of tissues (8). It is one of a number of modular pathway “tools” in the developmental “toolkit” that drives tissue organization, where its contribution to immune biology is just emerging (9–11). The Wnt pathway is organized like many cell-to-cell signaling pathways that induce both transcriptional and behavioral responses. An extracellular Wnt ligand is released by a secretor-cell and received by a responder-cell through a receptor complex generally comprising Frizzled and LRP5/6 family members (12). Depending on the presence or absence of additional co-receptors (elaborated below), Wnt-signaling can activate gene expression via β-catenin (β-cat) paired with a DNA-binding factor from the T cell factor/Lymphocyte enhancer factor (TCF/Lef)-family (13) (Figure 2). This transcriptional mode of Wnt signaling is often referred to as “canonical” signaling, as it was first and remains the most thoroughly defined consequence of Wnt signaling. Wnt receptor activation can alternatively direct diverse cell responses (e.g., planar polarization and cytoskeletal organization) through a number of less defined pathways that are independent of, and often inhibitory to, β-cat transcriptional function, referred to as the “non-canonical” pathway (14, 15). The extent to which a Wnt signal triggered β-cat-dependent vs. -independent responses was historically thought to be due to the particular form of Wnt ligand expressed, where most Wnts (e.g., Wnt3a, Wnt1) activate β-cat, while a smaller subset (e.g., Wnt5a, Wnt11, Wnt4) often antagonize β-cat signaling and/or signal independently of β-cat (16). However, it is now clear that it is the combination of Wnts, receptors, and co-receptors that dictate whether a response will activate β-cat or not (12, 17). Thus, an overriding challenge of understanding Wnt signaling in tissue biology is its complexity. In humans, there are 19 Wnts, 10 Frizzled receptors, a number of co-receptors (Lrp5/6, Lgr4/5/6, Ror1/2, and Derailed/Ryk), as well as secreted factors that both antagonize (e.g., DKKs, Sfrps) or agonize (e.g., R-spondins) Wnt/receptor signaling [reviewed in (18)]. Adding further to complexity, Wnt signaling is used reiteratively by cells and their descendants as tissue development unfolds, such that the target genes activated by Wnts are cell-type and context-dependent (19). It is in this latter context that we focus this review on evidence that macrophages are both a source and recipient of Wnt signals, particularly regarding core macrophage functions. As Wnt ligands are lipid modified (20–22), restricting their solubility, and localized signaling (23), we speculate that short-range Wnt gradients present in developing and adult tissues may play key roles in shaping organ-specific macrophage functions. All Wnt-macrophage studies discussed below are also summarized in Table 1.
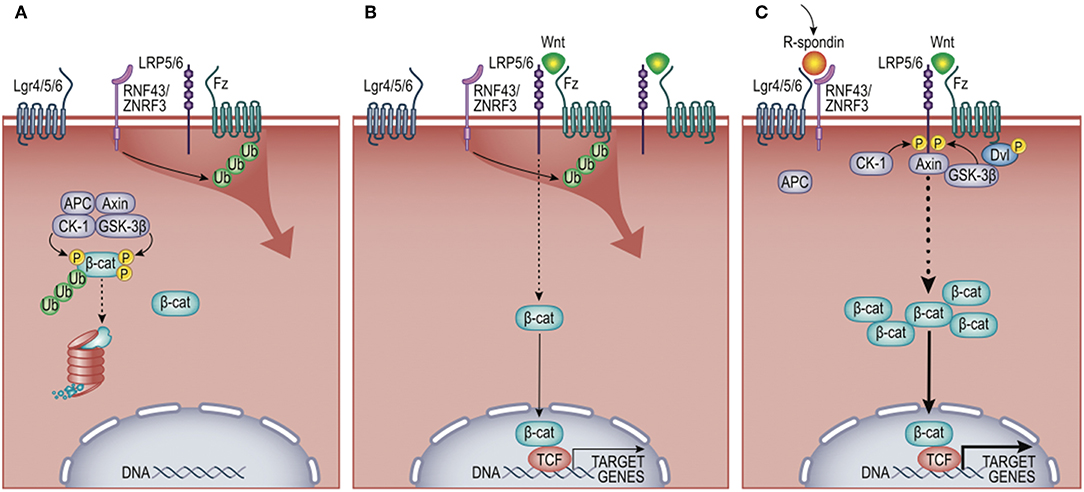
Figure 2. Wnt/β-cat signaling pathway. (A) In the absence of Wnt ligand, cytosolic β-cat is constitutively flagged for degradation by multi-protein complex comprising Adenomatosis Polyposis Coli (APC) protein, Axin, Casein Kinase 1 (CK-1), and Glycogen Synthase Kinase-3 beta (GSK-3β). (B) Wnt engagement of Frizzled (Fz) and low-density lipoprotein receptor-related protein 5 or 6 (Lrp5/6) inhibits β-cat turnover, favoring nuclear translocation and activation of target genes, including the negative feedback regulators zinc and ring finger proteins RNF43/ZNRF3. These E3 ligases antagonize Wnt signals by ubiquitylating Fz receptors, promoting their destruction. (C) R-spondins synergistically promote Wnt signals by binding leucine-rich repeat-containing G-protein coupled receptors (LGR4/5/6) and the E3 ligases RNF43/ZNRF3. This limits the ubiquitylation of Fz receptors, permitting enhanced activation of β-cat target genes. Non-canonical (i.e., β-cat-independent) Wnt signaling is not shown and described elsewhere (14).
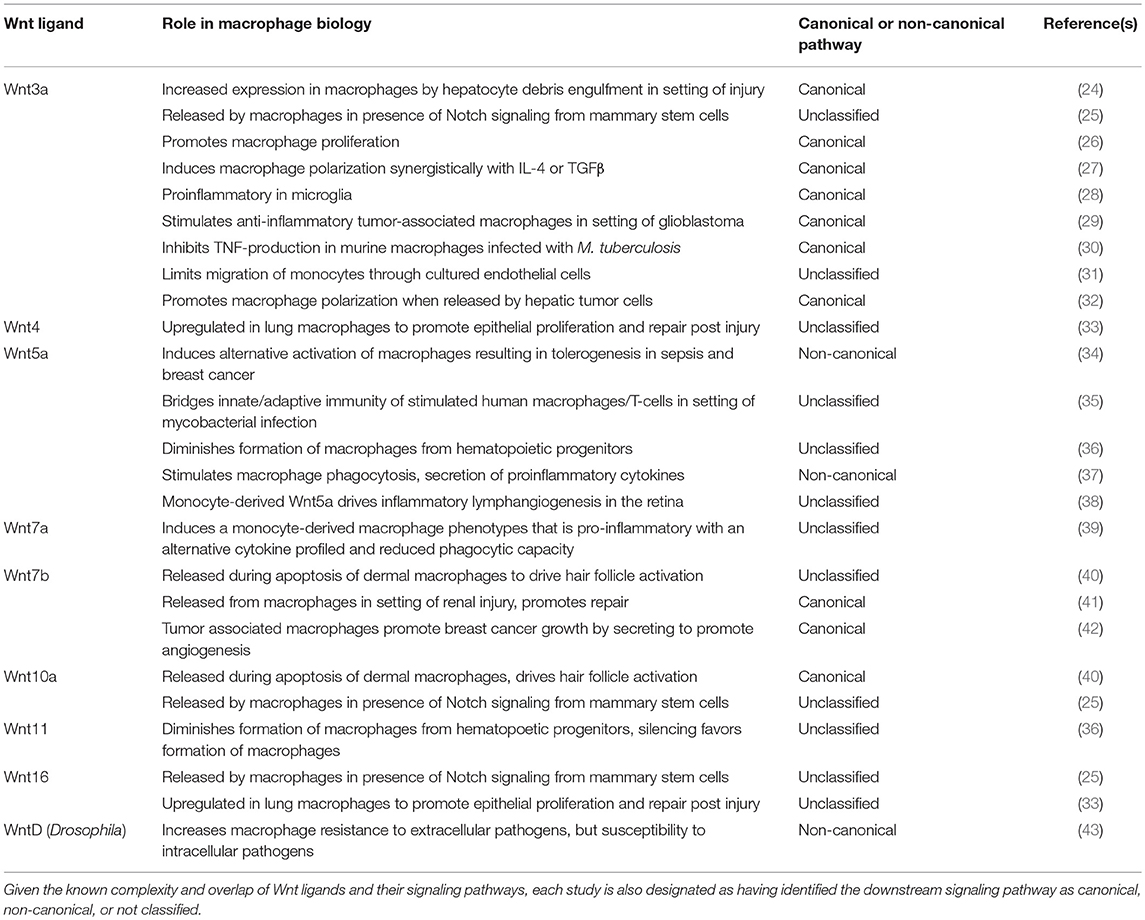
Table 1. Specific Wnt ligand studies mentioned in the text, listed numerically, with elucidated role in macrophage biology.
Wnt Signaling in Macrophage Differentiation and Maintenance
β-Catenin Signaling Directs Macrophage Differentiation
As discussed above, macrophage differentiation is highly contextual. Tissue-resident macrophages originate from embryonic or immediate post-natal tissues, where their transcriptional profile is shaped by their local environment [reviewed in (44)]. Conversely, monocytes arise from bone marrow progenitors throughout lifespan and, in response to tissue injury, become macrophages only after entry into an organ's interstitium. This spatial and environmental complexity makes studying of the pathways involved in monocyte development difficult, especially ex vivo. Nonetheless, in vitro studies suggest that β-cat signaling may promote monocyte differentiation from myeloblasts (45). Myeloblasts are a hematopoietic progenitor that also gives rise to the granulocytic series of blood cell types (e.g., neutrophils, basophils, eosinophils), where granulocyte-macrophage colony stimulating factor (GM-CSF) followed by macrophage colony stimulating factor (M-CSF) promote the production of myeloid cells and are essential for monocyte differentiation [reviewed in (46)]. Using this in vitro system, GM-CSF could direct a β-catenin/T-Cell Factor (β-cat/TCF) transcription program to specify the monocyte lineage. Careful mutational analyses of the beta-subunit of the GM-CSF receptor (GM-CSF-R) revealed molecular strategies that could enhance monocyte/macrophage differentiation at the expense of granulocyte differentiation (45). Microarray analysis of this GM-CSF-R-induced cell-fate switch revealed macrophage differentiation was accompanied by a robust accumulation of β-cat protein, TCF4 mRNA, and a number of β-cat/TCF4 target genes previously identified by chromatin immunoprecipitation (47), including the macrophage-lineage transcription factor, Egr-1 (48). Thus, β-cat/TCF4 signaling can direct monocytic over granulocytic cell fate in a culture system, similar to other binary cell-fate choices β-cat signaling directs throughout development and tissue homeostasis. These data may be consistent with early work showing that transduction of hematopoetic progenitor cell cultures with Wnt5a or Wnt11 expression vectors diminished the formation of macrophages, whereas Wnt11 silencing favored formation of cultures dominated by macrophages (36). Since Wnts5a and 11 are often found to inhibit β-cat signaling across numerous cell types (12), these data support the concept that β-cat signaling may be required for macrophage specification, particularly since removal of all Wnt activity from adult murine bone marrow failed to alter hematopoiesis (49).
Indeed, while GM-CSF-R-mediated upregulation of β-cat protein correlated with an inhibition of the major inhibitory kinase of β-cat, GSK3β (45), it is more likely that GM-CSF signaling is temporally and spatially uncoupled from the activation of β-cat required for macrophage specification. As discussed above, normal macrophage differentiation occurs within the interstitium of tissues, after blood monocyte recruitment, where β-cat activation is more likely to occur downstream of Wnt ligands (50). Given evidence that adult tissues are maintained by tonic Wnt signaling gradients (51–53), it seems likely that extravasating monocytes will find a Wnt-rich environment directing the monocyte-to-macrophage transition. Indeed, the spatial organization of Wnts and Wnt inhibitors may dictate where monocytes will be locally differentiated into macrophages, or even related lineages such as dendritic cells.
While macrophages across tissue types are largely transcriptionally distinct, reflecting the specialized functions of macrophages in each tissue, it is worth noting that a core group of macrophage-associated genes was recently identified (50), some of which show cross-regulation by Wnt/β-cat signaling. For example, the core macrophage factor, Bach1, can negatively regulate β-cat signaling at the level of β-cat/TCF4 interaction (54, 55). In addition, β-cat can either suppress or activate the major macrophage transcription factor CCAAT enhancer-binding protein-α (C/EBPα) (56, 57) depending on its pairing with Lef/TCF-family member isoforms. Thus, while Wnt/β-cat signaling is a plausible switch for the monocyte to macrophage transition across tissue, future mouse genetic studies with Cre-drivers that specifically target monocytes crossed with the β-cat-floxed mouse will be required to formally test this hypothesis.
Wnt Modulation of the Immune Response
Perhaps the earliest evidence linking a Wnt ligand to inflammatory signaling came from the study of embryonic fly development, where WntD (Wnt inhibitor of Dorsal) was found to be a gene target and negative feedback regulator of Toll receptor signaling (58). Specifically, WntD overexpression inhibited the nuclear accumulation and transcriptional activity of Dorsal (an NFkB ortholog). This inhibition was independent of either the inhibitory factor of kappa β (IkB) or β-cat orthologs, suggesting a non-canonical signaling mechanism. Importantly, WntD knock-out flies were immunocompromised and showed altered expression of antimicrobial peptides as well as greater sensitivity to death by Listeria, phenotypes that could be rescued by the loss of Dorsal/NFkB. These data raised the possibility that Wnts found in higher organisms might be similarly upregulated by innate immunity pathways to repress the adverse effects of excessive NFkB signaling.
Although Wnt8 is thought to be the WntD ortholog in higher organisms (59), there is more evidence for other Wnts shaping macrophage immune responses, including Wnt5a (35), Wnt6 (60), and Wnt7a (39). A microarray-assisted screen of human macrophages stimulated with different mycobacterial species found that Wnt5a could be induced by TLR-NFkB signaling, where it drove the enhanced expression of Th1 cytokines, IL12, p40, and INFγ (35). Since the pro-inflammatory effect of Wnt5a appeared to signal independently of the canonical effector, β-cat (61, 62), there was much interest in cross-comparing inflammatory responses initiated by canonical β-cat-activating Wnts, particularly the widely available Wnt3a ligand. Indeed, a number of studies found that Wnt3a/β-cat signaling induced anti-inflammatory cytokine signatures, such as reduced TNFα levels (30), reduced IL6 levels (63, 64), and elevated IL4 targets [Arg1, CD206/MR, Fizz1, YM1 (27); Figure 3]. However, Wnt5a could also generate anti-inflammatory macrophage phenotypes in certain contexts (34, 38). In addition, Wnt3a/β-cat signaling could be pro-inflammatory in microglia (28) and β-cat could pair with a dsRNA nucleic acid sensor protein to induce IFN-β production downstream of Listeria infection (65). Thus, it appears that no uniformly distinct macrophage response may be generated between canonical (β-cat-activating) vs. non-canonical Wnts (66). Whether this reflects differences in Wnt receptor repertoires between macrophage cell lines or subsets, the contextual nature of Wnt signaling and target gene selection, or other technical issues (discussed next), remains unclear.
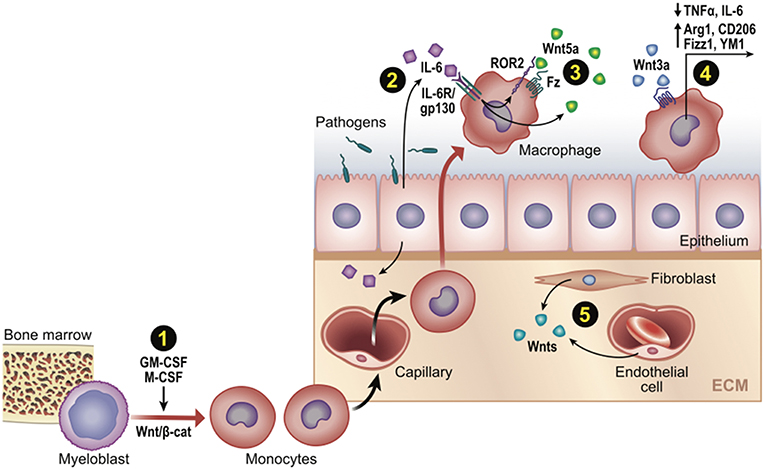
Figure 3. Wnt signaling in monocyte-macrophage development and function post-infection or injury. Schematic representation of Wnt-directed macrophage phenotypes. (1) Growth factors M-CSF and GM-CSF upregulate β-cat/TCF levels, which may promote differentiation of myeloblasts into monocytes in developing if not adult contexts. (2–3) Wnts can be a downstream target of core inflammatory signals (e.g., Wnt5a by IL6/NFkB), where it may promote or limit phagocytic activity. (4) Wnt/β-cat signaling often promotes an anti-inflammatory macrophage phenotype (cytokines/genes shown). (5) Various cell types may be Wnt sources (e.g., fibroblasts, endothelia, as well as epithelia and macrophages).
One complication of studying the downstream effects of particular Wnt ligands is that early “rules” for signaling gave way to exceptions, which are now seen as reflecting the highly contextual nature of this pathway. Early studies found Wnt5a to signal independently of β-cat (67), but we now know that Wnt5a can either activate or inhibit β-cat signaling depending on the presence of distinct cell-state dependent Wnt-receptors and co-receptors (15, 67). For example, when cells express Ror2, a member of the Ror-family of tyrosine kinase receptors, Wnt5a/Ror2 engagement triggers the activation of RhoA, JNK, and NFAT signaling events to control polarized cell adhesion and migration (68) [reviewed in (69)]. Conversely, in the absence of Ror2 and the presence Frizzled 4, Wnt5a can activate β-cat signaling (15). Thus, the expression and or availability of surface receptors is critical to how a particular form of Wnt will signal (12).
Another complication relevant to the study of inflammatory Wnt signaling in macrophages is that β-cat can also be robustly upregulated by the Th2-cytokine, IL4 (70). This increased β-cat is not for nuclear signaling, but rather is coupled to the cell-cell adhesion molecule, E-cadherin. Hence, conditions leading to higher levels of β-cat protein in macrophages do not necessarily mean these cells are responding to canonical Wnt signals. Lastly, other studies raise concerns that commercially available recombinant Wnt proteins bear variable inflammatory activities that cannot be antagonized by established inhibitors, such as sFRP1 (71), or are completely blocked in TLR4−/− macrophages (72). Whether this is due to bona fide lipopolysaccharide (LPS) contamination of recombinant Wnt preparations or overlapping physiochemical properties of LPS and acylated Wnt proteins remains unclear. Thus, the use of recombinant Wnts to interrogate inflammatory pathways should be considered judiciously and verified using forced expression, knock-down or targeted gene loss approaches.
Using these latter approaches, a number of studies clearly confirm roles for β-cat in shaping macrophage activation states. Pro-inflammatory macrophages, originating from newly recruited monocytes, are primed by TLR agonists of bacterial or viral nature and characterized by the production of pro-inflammatory cytokines, high phagocytosis activity and production of reactive oxygen species (ROS). These inflammatory macrophages not only clear pathogens, but also produce tissue damage. Upon phagocytosis of dead and apoptotic cells, these inflammatory monocyte-derived macrophages can convert into pro-repair cells, characterized by increased expression of IL10, Arg1, Tgm2 [reviewed in (3)]. Two recent studies found that tumor-derived Wnt ligands can stimulate an anti-inflammatory tumor-associated-macrophage phenotype via β-cat signaling, which contributes to tumor growth, migration, metastasis, and immunosuppression via elevated expression of Arg1, Sti1, IL10, and decreased expression of IL1b, respectively [(29, 32); Figure 3].
Perhaps the best examples of β-cat signaling driving anti-inflammatory phenotypes have been focused on the dendritic subset of monocyte-derived cells, using Cd11cCre targeted genetic approaches to activate or inhibit β-cat signaling in mice (73–75). These studies independently confirm roles for β-cat in the upregulation of key anti-inflammatory mediators, such as IL-10 and TGFβ, as well as tolerogenic T-cell behaviors relevant to tumor immunosuppression and inflammatory bowel disease (76, 77). Investigation into how distinct macrophage populations contribute to fibrosis revealed that genetic removal of β-cat from either Csf1r- or Cd11c-Cre expressing macrophages attenuates fibrosis in the both kidney (26) or lung injury models (78), through limiting the abundance/maturation of monocyte-recruited macrophages. The former study confirmed that Csfr1-Cre; βcat-floxed mice also show an anti-inflammatory profile (26). While macrophages are weak antigen presenting cells, showing less capacity to travel to lymph nodes for antigen presentation to T-cells than dendritic cells, these data nonetheless raise the possibility that orchestration of anti-tumor immunity and fibrosis resolution may depend on modulated β-cat signaling in both macrophage and dendritic cells. Since most tissue macrophages also express Cd11c and are therefore targeted using this recombinant genetic approach, future studies are required to delineate the precise roles of these subpopulations of the macrophage lineage.
Wnt Signals Control Specific Macrophage Functions
Phagocytosis
Evidence from Drosophila suggests that Wnt signaling may control a cell's response to different pathogens. For example, WntD mutants were more susceptible to the intracellular pathogen, L. monocytogenes, yet more resistant to the extracellular pathogen, S. pneumoniae (43). The loss of WntD is thought to lead to enhanced phagocytic activity and a shift in anti-microbial peptide that favors fast killing of an extracellular pathogen, but at the expense of inadequately controlling cytoplasmic access of an intracellular pathogen. There is some evidence confirming Wnt signals can also affect macrophage phagocytosis in mice, but molecular details remain unclear. In a macrophage cell line, recombinant Wnt5a stimulated phagocytosis of bacteria through a β-cat-independent signaling mechanism (37), and recombinant Wnt7a, but not Wnt1, inhibited phagocytosis in spleen monocyte-derived macrophages, correlating with reduced expression of surface molecules implicated in phagocytic uptake (e.g., CD14, CD11b, CD163, CD206) (39). Importantly, Wnt7a−/− mouse monocyte-derived macrophages showed elevated surface levels of CD11b, which could be reduced by adding back recombinant Wnt7a. Wnt signaling also plays a role in macrophagic phagocytosis in tissue development. Mice deficient in Lrp5, a critical Wnt co-receptor, displayed persistent embryonic eye vascularization due to a failure of macrophage-induced endothelial cell apoptosis (79). Although the precise mechanism is unclear, it is worth noting that in C. elegans, machinery required for the recognition and engulfment of dead cells can also promote apoptosis when caspase activity is compromised. Thus, Wnt-regulated phagocytes may provide a key backup mechanism for removing superfluous cells (80). Lastly, Lrp5 is also important for macrophage phagocytosis and clearance of lipids (e.g., low density lipoproteins), as Lrp5−/− mice are prone to development of atherosclerosis when fed a high fat diet (81, 82). Thus, Wnt receptors and downstream signaling contribute to macrophage phagocytosis in a variety of developmental and disease contexts.
Wnts and Macrophage Adhesion, Migration, and Tissue Recruitment
As Wnt signaling has been linked to numerous cell migratory events from gastrulation to neural crest cell dissemination [reviewed in (83)], it is attractive to consider this signaling module might be conserved in macrophages. Activation of β-cat signaling via Wnt3a (conditioned media) in monocytes (isolated from healthy donor peripheral blood) limited their migration through an endothelial layer of human dermal microvascular endothelial cells, while not affecting their motile capacity or ability to adhere to endothelial cells (31). In other contexts, Wnt co-receptor Lrp5 signaling contributed positively to macrophage motility (84, 85). Whether Wnts are locally secreted by an activated endothelium to modulate the recruitment of monocytes to move across tissue capillary beds remains unknown. Evidence CD11b (an integrin required for tissue migration) is upregulated on the surface of Wnt7a−/− mouse bone marrow-derived macrophages, showing enhanced tissue recruitment relative to controls (39), whereas CD11b is reduced on the surface of Csfr1Cre; β-cat null cells, showing less tissue recruitment than controls (26), strongly suggest that β-cat signaling in macrophages can regulate their entry and/or retention within tissues. Evidence also supports the concept that β-cat signaling in stromal cell populations beneath the vascular bed may direct the expression of chemokines that promote macrophage infiltration [e.g., via Cxcl12 (86)].
Macrophages as a Source of Wnts to Which Parenchymal Cells Respond
Given that macrophages play critical paracrine functions during tissue development and injury repair [reviewed in (87)], it is probably not surprising that numerous studies reveal macrophages as a source of morphogenic Wnt signals to guide stem cell behaviors during these processes.
Tissue Development and Renewal
Skin macrophages can drive the cyclical activation of hair follicle stem cells through an apoptosis-associated release of Wnts7b and 10a within the hair follicle niche (40). Since dermal macrophages have long been appreciated to resorb collagen during hair follicle regression (88–90), it is attractive to consider that the same cell-type which drives matrix scavenging can also dispatch regenerative ligands for the next round of hair growth. Similarly, the mammary gland epithelium forms post-natally during adolescence in a process called branching morphogenesis. For this to occur, epithelial progenitors must bifurcate (divide) and lengthen the terminal end bud into the fat pad, a process that requires macrophage-mediated remodeling of the collagen-rich matrix (91, 92). Recent evidence shows mammary stem cell expression of the Notch ligand, Dll1, activates Notch signaling in macrophages to stimulate Wnt secretion (Wnt3, 10a, and 16) (25). As with dermal macrophages in hair follicle cycling, mammary macrophages coordinate the matrix remodeling required for branching morphogenesis with the delivery of Wnt ligands to expand epithelial progenitors. In the gastrointestinal system, gut macrophages play an important role in signaling an overall readiness of the intestinal epithelium to sample antigens from the gut lumen. Anti-CSF1R approaches that effectively ablate macrophages resulted in perturbed intestinal stem cell abundance and subsequent lineage choices, such as elevated goblet cells and a reduction in M-cells along Peyer's patches, which would limit antigen sampling by sub-epithelial macrophages (93). Further studies of tissue-resident macrophages utilizing Wnts to maintain homeostasis in other adult tissues may reveal further similarities or organ-specific roles.
Repair After Injury
Perhaps the best evidence macrophages are a meaningful source of Wnts come from murine tissue-injury models. Conditional deletion of Wnt7b in Csf1R-expressing macrophages inhibited kidney repair after ischemia/reperfusion injury (41). During chronic liver injury, macrophage engulfment of hepatocyte debris induced Wnt3a expression, which stimulated β-cat signaling in nearby hepatocyte progenitors and their differentiation into hepatocytes (24). Critically, when all active Wnt secretion is prevented in liver macrophages using the WlsCre genetic approach, the normal Wnt gradient that controls liver hepatocyte zonation remains intact, yet liver regeneration after partial hepatectomy was impaired (94). Thus, in the liver, macrophage-derived Wnts are not important for normal tissue homeostasis, but rather for repair after injury. While the injury signals that promote the upregulation of macrophage-derived Wnts remain poorly defined, one recent study found that Cd11c-expressing lung macrophages promote epithelial proliferation and repair post-injury through a Trefoil factor 2-dependent mechanism that leads to the upregulation of Wnts4 and 16 (33). Lastly, it is worth noting that macrophages associated with lung disease can manifest high levels of the Wnt pathway agonist, R-spondin-3 (RSPO3) (95). Therefore, it appears that macrophage-derived Wnts, or factors that synergize with Wnts, may be a general feature of the tissue injury and repair cycle. Indeed, it may be parsimonious for the cell-type required to remove cellular debris and remodel basement membranes to also bring factors which stimulate renewal of the injured tissue.
There is also evidence supporting the concept that adult tissues may display tonic expression of Wnts, which may shape the identity and activity of macrophage sub-populations. For example, using single cell RNA sequencing of human fibrotic lungs, a small group of highly expressed Wnt ligands were apparently restricted to specific cell types, such as WNT2 in fibroblasts, WNT7b in alveolar type 2 and club cells, and WNT7a and WNT3a in type 1 cells (95). If Wnt proteins are secreted from basolateral rather than luminal apical surfaces (as is the case for most growth factors/morphogens), we speculate that during normal tissue homeostasis, interstitial macrophages may be constitutively subjected to a high Wnt environment, whereas alveolar macrophages may occupy a Wnt-free lumenal environment, which may shape their distinct transcriptional profiles (7). Intriguingly, RNA-sequencing of recently characterized population of pro-fibrotic, recruited monocyte-derived alveolar macrophages revealed altered expression of Wnt/β-cat pathway components and target genes (Lam, Gottardi, and Misharin, unpublished). Since deletion of β-cat from CD11c+ alveolar macrophages reduced the number of recruited monocyte-derived alveolar macrophages in the bleomycin model of murine fibrosis (78), we speculate that tissue injury, which may destroy the normal compartmental separation of Wnt-abundant from Wnt-free environments and/or upregulate Wnt expression, may be responsible for the aberrant differentiation state of pro-fibrotic alveolar macrophages.
Cancer Progression
It is well-established macrophages can both drive tumorigenesis and shape anti-tumor immunity [reviewed in (1, 96)]. An early example of the former came from multiphoton intravital imaging of a murine model of breast cancer, which showed epithelial expression of Csf1 promotes the infiltration of Csfr1+ macrophages, while macrophages act as a source of EGF, leading to a mutually reinforcing paracrine signaling loop of CSF-1 and EGF ligands between macrophages and tumor cells that drive tumor invasion and metastasis (97, 98). More recent studies support the idea that tumor associated macrophages may produce Wnts that contribute to tumor cell invasiveness (99–102), although Wnts were not formally removed in these studies. Importantly, genetic removal of Wnt7b from myeloid cells using an inducible Csfr1Cre approach appeared sufficient to reduce mammary tumor burden through inhibition of angiogenesis, possibly due to reduced VEGFa expression in vascular endothelial cells (42).
While evidence for Wnt-expression in macrophages relevant to tissue development, repair, and cancer progression appears clear, it should be noted that the absolute abundance of Wnt expression by macrophages is substantially less than other cell types within the same tissues, as evidenced by various single-cell RNA sequencing data sets that have become available (95) and by studies employing quantitative PCR methods (32, 101). While formal genetic evidence for macrophage-derived Wnts using currently available Cre-drivers exists (42, 103), it is important to recognize that Cre-drivers are often more broadly expressed than appreciated, particularly under injury-induced conditions, and should be considered when interpreting results from such models.
Summary
Growing knowledge at the intersection of macrophage biology and Wnt signaling reveals multiple roles for Wnt signals coming from and within the monocyte-macrophage lineage. Wnt signals received by macrophages are important for their various phagocytic roles, such as modulating the immune response in the setting of infection, tissue repair after injury, malignancy detection, and progression. Conversely, macrophages can be a source of Wnt signals critical for tissue morphogenesis and healing after injury. A key future direction will be to understand how tissue-specific macrophage identities are shaped by their local environment. Since Wnts have been classically shown to act locally, typically only a few cell diameters away from the source of Wnt secretion (23) evidence that adult tissues are maintained by local Wnt signaling niches (51–53) raise the intriguing possibility that such tissue-specific Wnt patterns may contribute to the variety of macrophage-type and behaviors critical to organ development and homeostasis.
Author Contributions
All authors listed have made a substantial, direct and intellectual contribution to the work, and approved it for publication.
Funding
We acknowledge support from Northwestern University's Lung Sciences Training Program T32-HL076139 (to EM) and HL134800 (to CG and AL).
Conflict of Interest Statement
The authors declare that the research was conducted in the absence of any commercial or financial relationships that could be construed as a potential conflict of interest.
Acknowledgments
We thank Sasha Misharin for feedback and Annette Flozak for reading the manuscript.
References
1. Guerriero JL. Macrophages: the road less traveled, changing anticancer therapy. Trends Mol Med. (2018) 24:472–89. doi: 10.1016/j.molmed.2018.03.006
2. Wood W, Martin P. Macrophage functions in tissue patterning and disease: new insights from the fly. Dev Cell. (2017) 40:221–33. doi: 10.1016/j.devcel.2017.01.001
3. Guilliams M, Scott CL. Does niche competition determine the origin of tissue-resident macrophages? Nat Rev Immunol. (2017) 17:451–60. doi: 10.1038/nri.2017.42
4. Jakubzick C, Gautier EL, Gibbings SL, Sojka DK, Schlitzer A, Johnson TE, et al. Minimal differentiation of classical monocytes as they survey steady-state tissues and transport antigen to lymph nodes. Immunity. (2013) 39:599–610. doi: 10.1016/j.immuni.2013.08.007
5. Epelman S, Lavine KJ, Randolph GJ. Origin and functions of tissue macrophages. Immunity. (2014) 41:21–35. doi: 10.1016/j.immuni.2014.06.013
6. Ginhoux F, Guilliams M. Tissue-resident macrophage ontogeny and homeostasis. Immunity. (2016) 44:439–49. doi: 10.1016/j.immuni.2016.02.024
7. Misharin AV, Morales-Nebreda L, Reyfman PA, Cuda CM, Walter JM, McQuattie-Pimentel AC, et al. Monocyte-derived alveolar macrophages drive lung fibrosis and persist in the lung over the life span. J Exp Med. (2017) 214:2387–404. doi: 10.1084/jem.20162152
8. Hoppler S, Moon RT. Wnt Signaling in Development and Disease: Molecular Mechanisms and Biological Functions. Hoboken, NJ: Wiley Blackwell (2014). doi: 10.1002/9781118444122
9. Chae WJ, Bothwell ALM. Canonical and non-canonical Wnt signaling in immune cells. Trends Immunol. (2018) 39:830–47. doi: 10.1016/j.it.2018.08.006
10. Staal FJT, Luis TC, Tiemessen MM. WNT signalling in the immune system: WNT is spreading its wings. Nat Rev Immunol. (2008) 8:581–93. doi: 10.1038/nri2360
11. Swafford D, Manicassamy S. Wnt signaling in dendritic cells: its role in regulation of immunity and tolerance. Discov Med. (2015) 19:303–10.
12. van Amerongen R. Alternative Wnt pathways and receptors. Cold Spring Harb Perspect Biol. (2012) 4:a007914. doi: 10.1101/cshperspect.a007914
13. Loh KM, van Amerongen R, Nusse R. Generating cellular diversity and spatial form: Wnt signaling and the evolution of multicellular animals. Dev Cell. (2016) 38:643–55. doi: 10.1016/j.devcel.2016.08.011
14. Semenov MV, Habas R, Macdonald BT, He X. SnapShot: non-canonical Wnt signaling pathways. Cell. (2007) 131:1378. doi: 10.1016/j.cell.2007.12.011
15. Mikels AJ, Nusse R. Purified Wnt5a protein activates or inhibits β-catenin-TCF signaling depending on receptor context. PLoS Biol. (2006) 4:e115. doi: 10.1371/journal.pbio.0040115
16. Najdi R, Proffitt K, Sprowl S, Kaur S, Yu J, Covey TM, et al. A uniform human Wnt expression library reveals a shared secretory pathway and unique signaling activities. Differentiation. (2012) 84:203–13. doi: 10.1016/j.diff.2012.06.004
17. Grumolato L, Liu G, Mong P, Mudbhary R, Biswas R, Arroyave R, et al. Canonical and noncanonical Wnts use a common mechanism to activate completely unrelated coreceptors. Genes Dev. (2010) 24:2517–30. doi: 10.1101/gad.1957710
18. MacDonald BT, Tamai K, He X. Wnt/β-catenin signaling: components, mechanisms, and diseases. Dev Cell. (2009) 17:9–26. doi: 10.1016/j.devcel.2009.06.016
19. Ramakrishnan AB, Cadigan KM. Wnt target genes and where to find them. F1000Res. (2017) 6:746. doi: 10.12688/f1000research.11034.1
20. Janda CY, Garcia KC. Wnt acylation and its functional implication in Wnt signalling regulation. Biochem Soc Trans. (2015) 43:211–6. doi: 10.1042/BST20140249
21. Speer KF, Sommer A, Tajer B, Mullins MC, Klein PS, Lemmon MA. Non-acylated Wnts can promote signaling. Cell Rep. (2019) 26:875–83. doi: 10.1016/j.celrep.2018.12.104
22. Willert K, Brown JD, Danenberg E, Duncan AW, Weissman IL, Reya T, et al. Wnt proteins are lipid-modified and can act as stem cell growth factors. Nature. (2003) 423:448–52. doi: 10.1038/nature01611
23. Farin HF, Jordens I, Mosa MH, Basak O, Korving JD, Tauriello DV, et al. Visualization of a short-range Wnt gradient in the intestinal stem-cell niche. Nature. (2016) 530:340–3. doi: 10.1038/nature16937
24. Boulter L, Govaere O, Bird TG, Radulescu S, Ramachandran P, Pellicoro A, et al. Macrophage-derived Wnt opposes Notch signaling to specify hepatic progenitor cell fate in chronic liver disease. Nat Med. (2012) 18:572–9. doi: 10.1038/nm.2667
25. Chakrabarti R, Celia-Terrassa T, Kumar S, Hang X, Wei Y, Choudhury A, et al. Notch ligand Dll1 mediates cross-talk between mammary stem cells and the macrophageal niche. Science. (2018) 360:eaan4153. doi: 10.1126/science.aan4153
26. Feng Y, Liang Y, Ren J, Dai C. Canonical Wnt signaling promotes macrophage proliferation during kidney fibrosis. Kidney Dis. (2018) 4:95–103. doi: 10.1159/000488984
27. Feng Y, Ren J, Gui Y, Wei W, Shu B, Lu Q, et al. Wnt/β-catenin-promoted macrophage alternative activation contributes to kidney fibrosis. J Am Soc Nephrol. (2018) 29:182–93. doi: 10.1681/ASN.2017040391
28. Halleskog C, Mulder J, Dahlstrom J, Mackie K, Hortobagyi T, Tanila H, et al. WNT signaling in activated microglia is proinflammatory. Glia. (2011) 59:119–31. doi: 10.1002/glia.21081
29. Matias D, Dubois LG, Pontes B, Rosario L, Ferrer VP, Balca-Silva J, et al. GBM-derived Wnt3a induces M2-like phenotype in microglial cells through Wnt/β-catenin signaling. (2019) Mol Neurobiol. 56:1517–30. doi: 10.1007/s12035-018-1150-5
30. Neumann J, Schaale K, Farhat K, Endermann T, Ulmer AJ, Ehlers S, et al. Frizzled1 is a marker of inflammatory macrophages, and its ligand Wnt3a is involved in reprogramming Mycobacterium tuberculosis-infected macrophages. FASEB J. (2010) 24:4599–612. doi: 10.1096/fj.10-160994
31. Tickenbrock L, Schwable J, Strey A, Sargin B, Hehn S, Baas M, et al. Wnt signaling regulates transendothelial migration of monocytes. J Leukoc Biol. (2006) 79:1306–13. doi: 10.1189/jlb.0905539
32. Yang Y, Ye YC, Chen Y, Zhao JL, Gao CC, Han H, et al. Crosstalk between hepatic tumor cells and macrophages via Wnt/β-catenin signaling promotes M2-like macrophage polarization and reinforces tumor malignant behaviors. Cell Death Dis. (2018) 9:793. doi: 10.1038/s41419-018-0818-0
33. Hung LY, Sen D, Oniskey TK, Katzen J, Cohen NA, Vaughan AE, et al. Macrophages promote epithelial proliferation following infectious and non-infectious lung injury through a Trefoil factor 2-dependent mechanism. Mucosal Immunol. (2019) 12:64–76. doi: 10.1038/s41385-018-0096-2
34. Bergenfelz C, Medrek C, Ekstrom E, Jirstrom K, Janols H, Wullt M, et al. Wnt5a induces a tolerogenic phenotype of macrophages in sepsis and breast cancer patients. J Immunol. (2012) 188:5448–58. doi: 10.4049/jimmunol.1103378
35. Blumenthal A, Ehlers S, Lauber J, Buer J, Lange C, Goldmann T, et al. The Wingless homolog WNT5A and its receptor Frizzled-5 regulate inflammatory responses of human mononuclear cells induced by microbial stimulation. Blood. (2006) 108:965–73. doi: 10.1182/blood-2005-12-5046
36. Brandon C, Eisenberg LM, Eisenberg CA. WNT signaling modulates the diversification of hematopoietic cells. Blood. (2000) 96:4132–41.
37. Maiti G, Naskar D, Sen M. The Wingless homolog Wnt5a stimulates phagocytosis but not bacterial killing. Proc Natl Acad Sci USA. (2012) 109:16600–5. doi: 10.1073/pnas.1207789109
38. Sessa R, Yuen D, Wan S, Rosner M, Padmanaban P, Ge S, et al. Monocyte-derived Wnt5a regulates inflammatory lymphangiogenesis. Cell Res. (2016) 26:262–5. doi: 10.1038/cr.2015.105
39. Wallace J, Lutgen V, Avasarala S, St. Croix B, Winn RA, Al-Harthi L. Wnt7a induces a unique phenotype of monocyte-derived macrophages with lower phagocytic capacity and differential expression of pro- and anti-inflammatory cytokines. Immunology. (2018) 153:203–13. doi: 10.1111/imm.12830
40. Castellana D, Paus R, Perez-Moreno M. Macrophages contribute to the cyclic activation of adult hair follicle stem cells. PLoS Biol. (2014) 12:e1002002. doi: 10.1371/journal.pbio.1002002
41. Lin SL, Li B, Rao S, Yeo EJ, Hudson TE, Nowlin BT, et al. Macrophage Wnt7b is critical for kidney repair and regeneration. Proc Natl Acad Sci USA. (2010) 107:4194–9. doi: 10.1073/pnas.0912228107
42. Yeo EJ, Cassetta L, Qian BZ, Lewkowich I, Li JF, Stefater JA III, et al. Myeloid WNT7b mediates the angiogenic switch and metastasis in breast cancer. Cancer Res. (2014) 74:2962–2973. doi: 10.1158/0008-5472.CAN-13-2421
43. Chambers MC, Lightfield KL, Schneider DS. How the fly balances its ability to combat different pathogens. PLoS Pathog. (2012) 8:e1002970. doi: 10.1371/journal.ppat.1002970
44. Davies LC, Jenkins SJ, Allen JE, Taylor PR. Tissue-resident macrophages. Nat Immunol. (2013) 14:986–95. doi: 10.1038/ni.2705
45. Brown AL, Peters M, D'Andrea RJ, Gonda TJ. Constitutive mutants of the GM-CSF receptor reveal multiple pathways leading to myeloid cell survival, proliferation, and granulocyte-macrophage differentiation. Blood. (2004) 103:507–16. doi: 10.1182/blood-2003-05-1435
46. Metcalf D. The molecular control of cell division, differentiation commitment and maturation in haemopoietic cells. Nature. (1989) 339:27–30. doi: 10.1038/339027a0
47. Yochum GS, McWeeney S, Rajaraman V, Cleland R, Peters S, Goodman RH. Serial analysis of chromatin occupancy identifies β-catenin target genes in colorectal carcinoma cells. Proc Natl Acad Sci USA. (2007) 104:3324–9. doi: 10.1073/pnas.0611576104
48. Brown AL, Salerno DG, Sadras T, Engler GA, Kok CH, Wilkinson CR, et al. The GM-CSF receptor utilizes β-catenin and Tcf4 to specify macrophage lineage differentiation. Differentiation. (2012) 83:47–59. doi: 10.1016/j.diff.2011.08.003
49. Kabiri Z, Numata A, Kawasaki A, Edison, Tenen DG, Virshup DM. Wnts are dispensable for differentiation and self-renewal of adult murine hematopoietic stem cells. Blood. (2015) 126:1086–94. doi: 10.1182/blood-2014-09-598540
50. Gautier EL, Shay T, Miller J, Greter M, Jakubzick C, Ivanov S, et al. Gene-expression profiles and transcriptional regulatory pathways that underlie the identity and diversity of mouse tissue macrophages. Nat Immunol. (2012) 13:1118–28. doi: 10.1038/ni.2419
51. Mah AT, Yan KS, Kuo CJ. Wnt pathway regulation of intestinal stem cells. J Physiol. (2016) 594:4837–47. doi: 10.1113/JP271754
52. Russell JO, Monga SP. Wnt/β-catenin signaling in liver development, homeostasis, and pathobiology. Annu Rev Pathol. (2018) 13:351–78. doi: 10.1146/annurev-pathol-020117-044010
53. Shoshkes-Carmel M, Wang YJ, Wangensteen KJ, Toth B, Kondo A, Massasa EE, et al. Subepithelial telocytes are an important source of Wnts that supports intestinal crypts. Nature. (2018) 557:242–6. doi: 10.1038/s41586-018-0084-4
54. Jiang L, Yin M, Wei X, Liu J, Wang X, Niu C, et al. Bach1 represses Wnt/β-catenin signaling and angiogenesis. Circ Res. (2015) 117:364–75. doi: 10.1161/CIRCRESAHA.115.306829
55. Jiang L, Yin M, Xu J, Jia M, Sun S, Wang X, et al. The transcription factor Bach1 suppresses the developmental angiogenesis of zebrafish. Oxid Med Cell Longev. (2017) 2017:2143875. doi: 10.1155/2017/2143875
56. Karner CM, Esen E, Chen J, Hsu FF, Turk J, Long F. Wnt protein signaling reduces nuclear Acetyl-CoA levels to suppress gene expression during osteoblast differentiation. J Biol Chem. (2016) 291:13028–39. doi: 10.1074/jbc.M115.708578
57. Kennell JA, O'Leary EE, Gummow BM, Hammer GD, MacDougald OA. T-cell factor 4N (TCF-4N), a novel isoform of mouse TCF-4, synergizes with β-catenin to coactivate C/EBPalpha and steroidogenic factor 1 transcription factors. Mol Cell Biol. (2003) 23:5366–75. doi: 10.1128/MCB.23.15.5366-5375.2003
58. Gordon MD, Dionne MS, Schneider DS, Nusse R. WntD is a feedback inhibitor of Dorsal/NF-kappaB in Drosophila development and immunity. Nature. (2005) 437:746–9. doi: 10.1038/nature04073
59. Murat S, Hopfen C, McGregor AP. The function and evolution of Wnt genes in arthropods. Arthropod Struct Dev. (2010) 39:446–52. doi: 10.1016/j.asd.2010.05.007
60. Schaale K, Brandenburg J, Kispert A, Leitges M, Ehlers S, Reiling N. Wnt6 is expressed in granulomatous lesions of Mycobacterium tuberculosis-infected mice and is involved in macrophage differentiation and proliferation. J Immunol. (2013) 191:5182–95. doi: 10.4049/jimmunol.1201819
61. Pereira C, Schaer DJ, Bachli EB, Kurrer MO, Schoedon G. Wnt5A/CaMKII signaling contributes to the inflammatory response of macrophages and is a target for the antiinflammatory action of activated protein C and interleukin-10. Arterioscler Thromb Vasc Biol. (2008) 28:504–10. doi: 10.1161/ATVBAHA.107.157438
62. Sen M, Ghosh G. Transcriptional outcome of Wnt-Frizzled signal transduction in inflammation: evolving concepts. J Immunol. (2008) 181:4441–5. doi: 10.4049/jimmunol.181.7.4441
63. Duan Y, Liao AP, Kuppireddi S, Ye Z, Ciancio MJ, Sun J. β-catenin activity negatively regulates bacteria-induced inflammation. Lab Invest. (2007) 87:613–24. doi: 10.1038/labinvest.3700545
64. Lee H, Bae S, Choi BW, Yoon Y. WNT/β-catenin pathway is modulated in asthma patients and LPS-stimulated RAW264.7 macrophage cell line. Immunopharmacol Immunotoxicol. (2012) 34:56–65. doi: 10.3109/08923973.2011.574704
65. Yang P, An H, Liu X, Wen M, Zheng Y, Rui Y, et al. The cytosolic nucleic acid sensor LRRFIP1 mediates the production of type I interferon via a β-catenin-dependent pathway. Nat Immunol. (2010) 11:487–94. doi: 10.1038/ni.1876
66. Ma B, Hottiger MO. Crosstalk between Wnt/β-catenin and NF-kappaB signaling pathway during inflammation. Front Immunol. (2016) 7:378. doi: 10.3389/fimmu.2016.00378
67. van Amerongen R, Fuerer C, Mizutani M, Nusse R. Wnt5a can both activate and repress Wnt/β-catenin signaling during mouse embryonic development. Dev Biol. (2012) 369:101–14. doi: 10.1016/j.ydbio.2012.06.020
68. Liu Y, Rubin B, Bodine PV, Billiard J. Wnt5a induces homodimerization and activation of Ror2 receptor tyrosine kinase. J Cell Biochem. (2008) 105:497–502. doi: 10.1002/jcb.21848
69. Katoh M, Katoh M. STAT3-induced WNT5A signaling loop in embryonic stem cells, adult normal tissues, chronic persistent inflammation, rheumatoid arthritis and cancer. Int J Mol Med. (2007) 19:273–8. doi: 10.3892/ijmm.19.2.273
70. Van den Bossche J, Bogaert P, van Hengel J, Guerin CJ, Berx G, Movahedi K, et al. Alternatively activated macrophages engage in homotypic and heterotypic interactions through IL-4 and polyamine-induced E-cadherin/catenin complexes. Blood. (2009) 114:4664–74. doi: 10.1182/blood-2009-05-221598
71. Cajanek L, Adlerz L, Bryja V, Arenas E. WNT unrelated activities in commercially available preparations of recombinant WNT3a. J Cell Biochem. (2010) 111:1077–9. doi: 10.1002/jcb.22771
72. Yu CH, Nguyen TT, Irvine KM, Sweet MJ, Frazer IH, Blumenthal A. Recombinant Wnt3a and Wnt5a elicit macrophage cytokine production and tolerization to microbial stimulation via Toll-like receptor 4. Eur J Immunol. (2014) 44:1480–90. doi: 10.1002/eji.201343959
73. Jiang A, Bloom O, Ono S, Cui W, Unternaehrer J, Jiang S, et al. Disruption of E-cadherin-mediated adhesion induces a functionally distinct pathway of dendritic cell maturation. Immunity. (2007) 27:610–24. doi: 10.1016/j.immuni.2007.08.015
74. Liang X, Fu C, Cui W, Ober-Blobaum JL, Zahner SP, Shrikant PA, et al. β-catenin mediates tumor-induced immunosuppression by inhibiting cross-priming of CD8+ T cells. J Leukoc Biol. (2014) 95:179–90. doi: 10.1189/jlb.0613330
75. Manicassamy S, Reizis B, Ravindran R, Nakaya H, Salazar-Gonzalez RM, Wang YC, et al. Activation of β-catenin in dendritic cells regulates immunity versus tolerance in the intestine. Science. (2010) 329:849–53. doi: 10.1126/science.1188510
76. Fu C, Liang X, Cui W, Ober-Blobaum JL, Vazzana J, Shrikant PA, et al. β-catenin in dendritic cells exerts opposite functions in cross-priming and maintenance of CD8+ T cells through regulation of IL-10. Proc Natl Acad Sci USA. (2015) 112:2823–8. doi: 10.1073/pnas.1414167112
77. Hong Y, Manoharan I, Suryawanshi A, Shanmugam A, Swafford D, Ahmad S, et al. Deletion of LRP5 and LRP6 in dendritic cells enhances antitumor immunity. Oncoimmunology. (2016) 5:e1115941. doi: 10.1080/2162402X.2015.1115941
78. Sennello JA, Misharin AV, Flozak AS, Berdnikovs S, Cheresh P, Varga J, et al. Lrp5/β-catenin signaling controls lung macrophage differentiation and inhibits resolution of fibrosis. Am J Respir Cell Mol Biol. (2017) 56:191–201. doi: 10.1165/rcmb.2016-0147OC
79. Kato M, Patel MS, Levasseur R, Lobov I, Chang BH, Glass DA, et al. Cbfa1-independent decrease in osteoblast proliferation, osteopenia, and persistent embryonic eye vascularization in mice deficient in Lrp5, a Wnt coreceptor. J Cell Biol. (2002) 157:303–14. doi: 10.1083/jcb.200201089
80. Hoeppner DJ, Hengartner MO, Schnabel R. Engulfment genes cooperate with ced-3 to promote cell death in Caenorhabditis elegans. Nature. (2001) 412:202–6. doi: 10.1038/35084103
81. Borrell-Pages M, Carolina Romero J, Badimon L. LRP5 and plasma cholesterol levels modulate the canonical Wnt pathway in peripheral blood leukocytes. Immunol Cell Biol. (2015) 93:653–61. doi: 10.1038/icb.2015.41
82. Borrell-Pages M, Romero JC, Badimon L. LRP5 deficiency down-regulates Wnt signalling and promotes aortic lipid infiltration in hypercholesterolaemic mice. J Cell Mol Med. (2015) 19:770–7. doi: 10.1111/jcmm.12396
83. Schambony A, Wedlich D. Wnt signaling and cell migration. In: Madame Curie Bioscience Database. Austin, TX: Landes Bioscience (2013).
84. Borrell-Pages M, Romero JC, Crespo J, Juan-Babot O, Badimon L. LRP5 associates with specific subsets of macrophages: molecular and functional effects. J Mol Cell Cardiol. (2016) 90:146–56. doi: 10.1016/j.yjmcc.2015.12.002
85. Borrell-Pages M, Romero JC, Juan-Babot O, Badimon L. Wnt pathway activation, cell migration, and lipid uptake is regulated by low-density lipoprotein receptor-related protein 5 in human macrophages. Eur Heart J. (2011) 32:2841–50. doi: 10.1093/eurheartj/ehr062
86. Akcora BO, Storm G, Bansal R. Inhibition of canonical WNT signaling pathway by β-catenin/CBP inhibitor ICG-001 ameliorates liver fibrosis in vivo through suppression of stromal CXCL12. Biochim Biophys Acta Mol Basis Dis. (2018) 1864:804–18. doi: 10.1016/j.bbadis.2017.12.001
87. Varol C, Mildner A, Jung S. Macrophages: development and tissue specialization. Annu Rev Immunol. (2015) 33:643–75. doi: 10.1146/annurev-immunol-032414-112220
88. Parakkal PF. Involvement of macrophages in collagen resorption. J Cell Biol. (1969) 41:345–54. doi: 10.1083/jcb.41.1.345
89. Parakkal PF. Role of macrophages in collagen resorption during hair growth cycle. J Ultrastruct Res. (1969) 29:210–7. doi: 10.1016/S0022-5320(69)90101-4
90. Parakkal PF. Ultrastructural changes of the basal lamina during the hair growth cycle. J Cell Biol. (1969) 40:561–4. doi: 10.1083/jcb.40.2.561
91. Ingman WV, Wyckoff J, Gouon-Evans V, Condeelis J, Pollard JW. Macrophages promote collagen fibrillogenesis around terminal end buds of the developing mammary gland. Dev Dyn. (2006) 235:3222–9. doi: 10.1002/dvdy.20972
92. Van Nguyen A, Pollard JW. Colony stimulating factor-1 is required to recruit macrophages into the mammary gland to facilitate mammary ductal outgrowth. Dev Biol. (2002) 247:11–25. doi: 10.1006/dbio.2002.0669
93. Sehgal A, Donaldson DS, Pridans C, Sauter KA, Hume DA, Mabbott NA. The role of CSF1R-dependent macrophages in control of the intestinal stem-cell niche. Nat Commun. (2018) 9:1272. doi: 10.1038/s41467-018-03638-6
94. Yang J, Mowry LE, Nejak-Bowen KN, Okabe H, Diegel CR, Lang RA, et al. β-catenin signaling in murine liver zonation and regeneration: a Wnt-Wnt situation! Hepatology. (2014) 60:964–76. doi: 10.1002/hep.27082
95. Reyfman PA, Walter JM, Joshi N, Anekalla K, McQuattie-Pimentel AC, Chiu S, et al. Single-cell transcriptomic analysis of human lung provides insights into the pathobiology of pulmonary fibrosis. Am J Respir Crit Care Med. (2019) 199:1517–36. doi: 10.1101/296608
96. Cheung KJ, Ewald AJ. Illuminating breast cancer invasion: diverse roles for cell-cell interactions. Curr Opin Cell Biol. (2014) 30:99–111. doi: 10.1016/j.ceb.2014.07.003
97. Lin EY, Nguyen AV, Russell RG, Pollard JW. Colony-stimulating factor 1 promotes progression of mammary tumors to malignancy. J Exp Med. (2001) 193:727–40. doi: 10.1084/jem.193.6.727
98. Wyckoff J, Wang W, Lin EY, Wang Y, Pixley F, Stanley ER, et al. A paracrine loop between tumor cells and macrophages is required for tumor cell migration in mammary tumors. Cancer Res. (2004) 64:7022–9. doi: 10.1158/0008-5472.CAN-04-1449
99. Debebe A, Medina V, Chen CY, Mahajan IM, Jia C, Fu D, et al. Wnt/β-catenin activation and macrophage induction during liver cancer development following steatosis. Oncogene. (2017) 36:6020–9. doi: 10.1038/onc.2017.207
100. Linde N, Casanova-Acebes M, Sosa MS, Mortha A, Rahman A, Farias E, et al. Macrophages orchestrate breast cancer early dissemination and metastasis. Nat Commun. (2018) 9:21. doi: 10.1038/s41467-017-02481-5
101. Ojalvo LS, Whittaker CA, Condeelis JS, Pollard JW. Gene expression analysis of macrophages that facilitate tumor invasion supports a role for Wnt-signaling in mediating their activity in primary mammary tumors. J Immunol. (2010) 184:702–12. doi: 10.4049/jimmunol.0902360
102. Pukrop T, Klemm F, Hagemann T, Gradl D, Schulz M, Siemes S, et al. Wnt 5a signaling is critical for macrophage-induced invasion of breast cancer cell lines. Proc Natl Acad Sci USA. (2006) 103:5454–9. doi: 10.1073/pnas.0509703103
Keywords: macrophages, monocytes, beta catenin, Wnt signaling, immunity
Citation: Malsin ES, Kim S, Lam AP and Gottardi CJ (2019) Macrophages as a Source and Recipient of Wnt Signals. Front. Immunol. 10:1813. doi: 10.3389/fimmu.2019.01813
Received: 25 April 2019; Accepted: 18 July 2019;
Published: 31 July 2019.
Edited by:
Elodie Segura, Institut Curie, FranceReviewed by:
Jeffrey M. Rosen, Baylor College of Medicine, United StatesYifan Zhan, Walter and Eliza Hall Institute of Medical Research, Australia
Copyright © 2019 Malsin, Kim, Lam and Gottardi. This is an open-access article distributed under the terms of the Creative Commons Attribution License (CC BY). The use, distribution or reproduction in other forums is permitted, provided the original author(s) and the copyright owner(s) are credited and that the original publication in this journal is cited, in accordance with accepted academic practice. No use, distribution or reproduction is permitted which does not comply with these terms.
*Correspondence: Anna P. Lam, YS1sYW00QG5vcnRod2VzdGVybi5lZHU=; Cara J. Gottardi, Yy1nb3R0YXJkaUBub3J0aHdlc3Rlcm4uZWR1