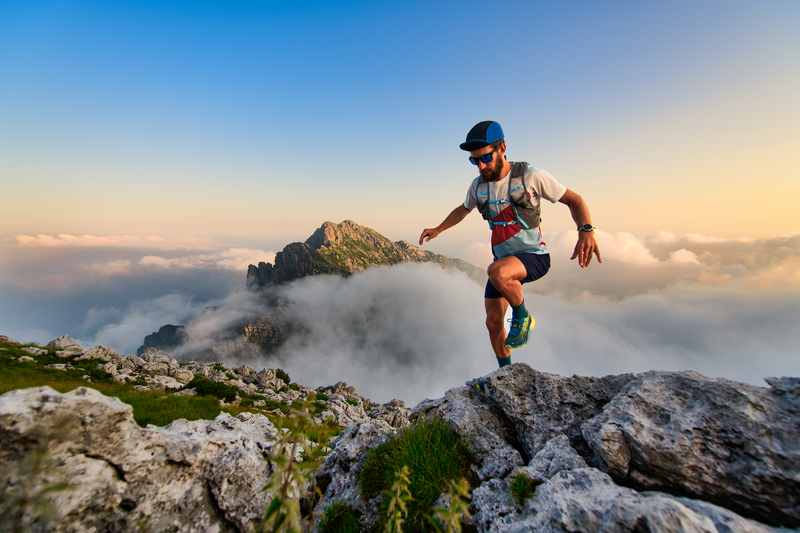
95% of researchers rate our articles as excellent or good
Learn more about the work of our research integrity team to safeguard the quality of each article we publish.
Find out more
REVIEW article
Front. Immunol. , 25 June 2019
Sec. NK and Innate Lymphoid Cell Biology
Volume 10 - 2019 | https://doi.org/10.3389/fimmu.2019.01416
This article is part of the Research Topic Circuits of Resident Immunity Regulating Tissue Adaptation and Organ Homeostasis View all 12 articles
The lungs, a special site that is frequently challenged by tumors, pathogens and other environmental insults, are populated by large numbers of innate immune cells. Among these, natural killer (NK) cells are gaining increasing attention. Recent studies have revealed that NK cells are heterogeneous populations consisting of distinct subpopulations with diverse characteristics, some of which are determined by their local tissue microenvironment. Most current information about NK cells comes from studies of NK cells from the peripheral blood of humans and NK cells from the spleen and bone marrow of mice. However, the functions and phenotypes of lung NK cells differ from those of NK cells in other tissues. Here, we provide an overview of human and mouse lung NK cells in the context of homeostasis, pathogenic infections, asthma, chronic obstructive pulmonary disease (COPD) and lung cancer, mainly focusing on their phenotype, function, frequency, and their potential role in pathogenesis or immune defense. A comprehensive understanding of the biology of NK cells in the lungs will aid the development of NK cell-based immunotherapies for the treatment of lung diseases.
The lungs comprise mucosae that are constantly exposed to environmental and autologous stimuli, and they are sites of high incidence of primary and metastatic tumors (1). Accordingly, a rapid and efficient immune response that prevents tumorigenesis and pathogen invasion without leading to excessive inflammation is needed to maintain pulmonary homeostasis. As a type of innate immune cell, natural killer (NK) cells are regarded as the host's first line of defense against tumors and viral infection (2). Moreover, involvement of NK cells in various lung diseases, such as lung cancer, chronic obstructive pulmonary disease (COPD) and asthma, as well as infections, has been documented (Table 1) (34, 35, 37–39).
In humans, NK cells are usually defined as CD3−CD56+ cells, and they are divided into two main subsets with different functions and maturation statuses: CD56brightCD16− and CD56dimCD16+. The CD56dimCD16+ NK cells are known as a highly differentiated subset with killer cell immunoglobulin-like receptor (KIR) expression, potent cytotoxicity and the capacity to induce antibody-dependent cellular cytotoxicity (ADCC), while the less mature CD56brightCD16− NK cells lack KIR expression but are the major producers of cytokines (40–42). In mice, NK cells do not express CD56 and have historically been defined as CD3−NK1.1+ cells (and, more recently, CD3−NKp46+ cells). However, type 1 innate lymphoid cells (ILC1s) and some subsets of type 3 innate lymphoid cells (ILC3s) also express NK1.1 and NKp46 and are easily confused with NK cells (43, 44). No equivalent subsets to the human NK cell subsets have been established to date among mouse NK cells. Mouse NK cells are divided into four subsets from the most immature to the most mature, according to the expression of CD27 and CD11b: CD27−CD11b−, CD27+CD11b−, CD27+CD11b+, and CD27−CD11b+ (45– 47).
NK cell functions are modulated by the balance between activating and inhibitory signals delivered by receptors expressed on the NK cell surface (Table 2). Abnormal cells (including cancer cells and infected cells) activate NK cells via lack of ligands of NK cell inhibitory receptors (missing self) or increased expression of ligands of NK cell activating receptors (induced self) (48–50). In addition, cytokines such as interleukin (IL)-12, IL-15, IL-18, and type I interferon (IFN), as well as Toll-like receptor (TLR) ligands, are powerful activators of NK cell functions (51, 52). Activated NK cells then function in various environments mainly through cytotoxicity and cytokine production. Recent findings have revealed that the functions and phenotypes of NK cells vary depending on their local microenvironments (53), mainly due to the distinct cytokines, cellular composition and foreign stimuli of various tissues. In this review, we provide an overview of the current understanding and gaps in knowledge regarding NK cells in the lungs.
Lung NK cells are generally thought to originate and develop in the bone marrow, and then migrate to the lungs (54). In human lungs, NK cells, accounting for about 10–20% of the lymphocytes, are located in the parenchyma and are not detected outside the parenchyma (Figure 1) (1). In mice, lung NK cells account for about 10% of the lymphocytes, and this percentage is higher than the percentages in other tissues (liver, peripheral blood, spleen, bone marrow, thymus and lymph node) (55, 56). Moreover, the number of mouse lung NK cells is second only to the number of spleen NK cells (55).
Figure 1. Lung NK cells in homeostasis. NK cells account for 10–20% of lymphocytes in the human and mouse lungs, and these cells are located in the lung parenchyma. (A) NK cells in the lungs have a more mature phenotype compared to those in other tissues. In mice, lung NK cells express high levels of the mature markers NKp46, CD49b, CD11b, and Ly49s. In humans, lung NK cells are mostly composed of the CD56dimCD16+ subset, and highly differentiated CD57+NKG2A− NK cells are present at a higher frequency in the lungs than in matched peripheral blood. (B) The vast majority of lung NK cells are circulating, and the existence of a small percentage of seemingly tissue-resident NK cells in the lungs remains to be confirmed. (C,D) Lung NK cells are hypofunctional in homeostasis, and their cytotoxicity and IFN-γ production levels are lower than those of NK cells in the spleen and peripheral blood. IFN, interferon; NK, natural killer; PB, peripheral blood.
Human lung NK cells are mostly composed of the CD56dimCD16+ subset. In addition, KIR-expressing NK cells and highly differentiated CD57+NKG2A− NK cells are found at higher frequencies in the lungs than in matched peripheral blood. These findings indicate that human lung NK cells have a well-differentiated phenotype (1). Even as early as the human fetal period, the frequency of KIR-expressing and differentiated NK cells is highest in the lungs compared to other tissues (57). A more mature phenotype is also observed for mouse lung NK cells. The most mature subset, CD27−CD11b+ NK cells, is found at a higher frequency among the lung NK cells (>70%) than those in the liver, peripheral blood, spleen, bone marrow and lymph nodes. Moreover, NK cells in the mouse lung express higher levels of the mature markers CD49b, CD122, CD43, Ly49s, and CD11b, but lower levels of the immature marker CD51, than NK cells in other tissues (56, 58).
Despite the well-differentiated phenotype, both human and mouse lung NK cells are hypofunctional in homeostasis. Human lung NK cells are hyporesponsive to stimulation by target cells (irrespective of priming with IFN-α) compared with peripheral blood NK cells (1). This may be caused by suppressive effects of alveolar macrophages and soluble factors in the epithelial lining fluid of the lower respiratory tract (59). Similarly, in mice, lung NK cells exhibit lower cytotoxicity toward targets compared with spleen NK cells when stimulated by IL-2 or IL-2/IL-12/IL-18 (58). In addition, in mice, the expression intensity of molecules associated with activation (NKp46, NKG2D, and CD69) is lower, and the expression of inhibitory receptors (NKG2A and CD94) is higher, on lung NK cells than on NK cells in the spleen and bone marrow. This indicates that lung NK cells are subject to tighter restrictions in the steady state (56). As the lungs comprise mucosal surfaces that are constantly exposed to environmental and autologous antigens, the dominance of hypofunctional NK cells may contribute to pulmonary homeostasis.
CD49a, CD69, and CD103 are regarded as markers of tissue-resident NK cells (53, 60–63). The fact that the vast majority of lung NK cells in mice are non-tissue-resident cells has been demonstrated by a parabiotic mouse model and the very low expression of CD49a and CD69 (35, 64, 65). Although the majority of human lung NK cells are CD56dim with a non-tissue-resident phenotype (1), a small but distinct CD49a+ lung NK cell subset (which largely involves CD56bright NK cells) has recently been identified (66). These CD56brightCD49a+ lung NK cells strongly co-express CD103 and CD69 and these cells are not found among the CD56bright NK cells in the peripheral blood, implying that CD56brightCD49a+ lung NK cells may be tissue-resident cells (66). However, the circulating and tissue-resident characteristics of human lung NK cells still need to be further investigated using humanized mice and “multi-omics” analyses (67).
There is increasing evidence that NK cells are involved in lung immune responses to respiratory pathogens. As an important type of innate immune cell, NK cells can respond rapidly to invading pathogens and clear them efficiently. On the other hand, NK cells may cause uncontrolled inflammation and pathological damage in some cases.
NK cells are innate immune cells that confer early immunity in acute viral infections and, accordingly, patients with genetic deficiencies that cause the loss of functions of NK cells are subjected to recurrent viral infection (39, 68, 69). However, the rapid immune response mediated by NK cells may sometimes occur at the cost of excessive inflammation. The lungs are continually exposed to various respiratory viruses such as influenza viruses. The evidence of involvement of NK cells in influenza infection dates back to 1982. Ennis et al. (70) demonstrated that individuals infected with influenza viruses exhibit increased peripheral blood NK cell activity in association with interferon (now known as IFN-γ) induction. In mouse models, depletion of systemic or lung NK cells increases the morbidity and mortality of mice during the early course of medium-dose influenza infection (3, 4), indicating a protective role of NK cells. In contrast, depletion of systemic NK cells improves the survival of mice infected with high-dose influenza viruses by alleviating lung immunopathology (5, 6). These findings uncover a dual role for mouse NK cells in influenza infection, providing protection or contributing to pathogenesis, depending on the virus dose.
During influenza infections, NK cells are activated by infected cells via contact-dependent mechanisms (71), and by cytokines such as IL-12, IL-2 and type I IFN, which are derived from infected cells and possibly from other cell types (71–76). In addition to these conventional recognition modes during viral infection, the NK cell receptors NKp44 (which is only expressed on human NK cells) and NKp46 can bind to influenza haemagglutinins (HAs). This allows NK cells to directly recognize influenza viruses and lyse influenza virus-infected cells (77–80). Recent studies have found that influenza vaccines induce the immune memory of human NK cells (81). Similarly, in mice, influenza infection also induces memory-like NK cells, which protect the mice against secondary influenza infection. Intriguingly, these memory-like NK cells reside in the liver rather than in the lungs (82–84), and NK cell-mediated recall responses are not dependent on the NKp46-HA interaction (85).
In mice, NK cells quickly accumulate in the lungs within the first few days of influenza infection (56, 86). These activated lung NK cells then contribute to viral clearance through IFN-γ production, activation of adaptive immune cells, ADCC and cytotoxic lysis. More recently, Kumar et al. (87) reported that conventional NKp46+NK1.1+CD127−RORγt− NK cells in the bronchoalveolar lavage fluid (BALF), trachea and lung tissue produce IL-22 during influenza infection, which facilitates tissue regeneration and prevents excessive lung inflammation. These findings indicate the multiple roles of NK cells in response to influenza viruses.
Due to the difficulties of obtaining lung tissues from humans infected with influenza viruses, most studies exploring the responses of human NK cells to influenza viruses use peripheral blood NK cells (73, 88–90), and less is known about human lung NK cells in influenza infections. Recently, Cooper et al. (66) utilized a lung explant model to characterize human lung NK cells during the early course of influenza infection. The lung NK cells responded quickly upon ex vivo influenza infection of lung explants, with upregulation of CD107a by 24 h after infection. Compared with CD56brightCD49a− NK cells, CD56brightCD49a+ lung NK cells, which possibly represent a tissue-resident and trained NK cell subset, express higher levels of CD107a. Recent studies have shown that some activated CD56dimCD16+ NK cells lose CD16 expression through ADAM17-mediated shedding and become CD56dimCD16− NK cells (91). However, the expression of CD107a on CD56bright and CD56dim NK cells is comparable, and there is no difference in expression between CD56dimCD16−CD49a+ and CD56dimCD16−CD49a− NK cells (66). Although granzyme B and IFN-γ are induced in lung explants after influenza infection, and enhanced IFN-γ responses are detected in peripheral blood NK cells following influenza vaccination (66, 73, 88, 90), there is no direct evidence that granzyme B and IFN-γ are released by lung NK cells. Thus, the immune responses of human lung NK cells in influenza infection remain to be further explored.
Despite the potent antiviral function of NK cells, recurrent influenza infections are common, suggesting that influenza viruses employ complex strategies to evade NK cell-mediated immunosurveillance (92). First, influenza viruses replicate rapidly before NK cells accumulate robustly in the lungs, providing sufficient time for virus dissemination (93). Second, mutation of influenza HA may impair the capacity of NK cells to recognize and lyse infected cells (94). Third, activation of NK cells can be inhibited by influenza HA in a dose-dependent manner (95, 96). On the other hand, when the levels of HA are too low for NK cell recognition, NK cells may not be activated sufficiently to clear viruses (93, 97). Fourth, influenza viruses can directly infect NK cells and induce apoptosis, leading to decreased NK cell cytotoxicity (98).
NK cells are generally regarded as important contributors to the host defense against tumors and viruses, but recent studies have shown that NK cells also play a role in resisting bacterial infections.
Tuberculosis is a leading cause of bacterial infections worldwide. M. tuberculosis (MTb) maintains a latent state in most infected individuals, and active disease usually progresses slowly, manifesting later in life (99). In vitro studies demonstrate that human peripheral blood NK cells can be activated by MTb-infected monocytes, and this is mediated by NKG2D recognition of ULBP1 and by NKp46 recognition of vimentin (100, 101). Moreover, human NK cells can directly recognize MTb by the binding of TLR2 and NKp44 to peptidoglycan and unknown components of MTb cell walls, respectively, and then become activated (102–104).
A study in immunocompetent mice showed that activated NK cells with upregulated CD69, IFN-γ, and perforin accumulated in the lungs in the early stage after aerosol infection with MTb, but depletion of NK cells did not influence the course of infection (105). Nevertheless, another study in T cell-deficient mice demonstrated that NK cells mediated early defense against MTb infections via IFN-γ (19, 106). Given that mice infected with MTb progress directly to active disease without experiencing latency, these reports indicate the redundant role of NK cells in the active stages of MTb infection. In humans, NK cells in the peripheral blood stimulated with MTb or live M. bovis Bacillus Calmette-Guerin (BCG) upregulate IFN-γ expression (107, 108). More recently, Chowdhury et al. (109) conducted a long-term study on a cohort of South African adolescents and found that the frequency of NK cells in the peripheral blood can inform disease progression, therapeutic responses and lung inflammation of patients with active tuberculosis. Pleural fluid, which is the excess fluid that collects around the lungs of pulmonary tuberculosis patients, may be closer to the pulmonary milieus than peripheral blood. The pleural fluid is enriched with IFN-γ-producing CD56bright NK cells due to selective apoptosis of cytotoxic CD56dim NK cells induced by soluble factors present in tuberculous effusions (110). Together, these findings in mice and humans suggest that NK cells may function at the site of active MTb infections mainly through IFN-γ production rather than cytotoxic lysis. Although Chowdhury et al. (109) showed that peripheral blood NK cells from individuals with latent tuberculosis infection display elevated cytotoxicity and increased frequency, whether cytotoxic lysis is employed by NK cells in the defense against MTb, especially latent MTb, remains to be further researched.
K. pneumoniae is an important cause of nosocomial pneumonia and is infamous for multidrug resistance. In vitro studies have shown that human NK cells can be activated by TLR2 recognition of recombinant protein A, the pathogen-associated molecular pattern expressed by K. pneumoniae (111). However, whether NK cells can recognize live K. pneumoniae and lyse K. pneumoniae after this direct recognition is unclear. In mice, lung NK cells promote host defense against K. pneumoniae by IL-22 and IFN-γ production (13, 14). On the other hand, Wang et al. (112) demonstrated that K. pneumoniae pre-infection alleviated influenza virus-induced death and acute lung injury by inhibiting lung NK cell expansion. These findings suggest a complex role of NK cells in response to various pathogens. Thus, accurate and in-depth research into NK cells in different infection conditions is needed and this will contribute to the development of effective interventions for lung infections.
Asthma and COPD are very common and serious chronic inflammatory diseases of the lungs that may lead to pulmonary fibrosis (113, 114), and NK cells are implicated in both diseases.
Asthma is a chronic airway inflammatory disease, and the majority of cases involve allergic asthma which is typically characterized by type 2 immune responses (114). Asthma can be induced and exacerbated by many factors, such as environmental pollutants, allergens, obesity and viral infections.
Current reports on NK cells in asthmatic patients seem to be somewhat contradictory, both regarding the numbers and functions of NK cells. An early study showed that the percentage of peripheral blood NK cells increases in asthmatic children during acute exacerbations relative to asthmatic children who are in a stable state after prednisolone therapy (115). Nevertheless, Barning et al. (29) and Duvall et al. (116) demonstrated that asthmatic patients have fewer NK cells in both the peripheral blood and BALF than healthy individuals, and the loss of NK cells is increased in patients with severe asthma. The CD56dim NK cell subset (but not the CD56bright NK cell subset) is selectively lost in the peripheral blood of asthmatic patients, whereas BALF NK cells are skewed toward a CD56dim phenotype in asthmatic patients. With regard to the functions of NK cells, there is evidence that NK cells can facilitate inflammation resolution by inducing eosinophil apoptosis (29). In healthy individuals and patients with mild asthma, NK cells in the peripheral blood can induce the apoptosis of eosinophils efficiently. In contrast, despite displaying a more activated phenotype, the cytotoxicity of peripheral blood NK cells from patients with severe asthma is impaired, and the decreased cytotoxicity can be exacerbated by corticosteroids (29, 116). These results indicate the attenuated capacity of NK cells to resolve inflammation in severe asthma. In contrast, earlier studies reported that NK cell cytotoxicity is elevated in the peripheral blood of patients with asthma compared to healthy individuals, and it declines immediately after acute antigen challenge (117, 118). On the other hand, Wei et al. (37) showed that there were increased IL-4+ NK cells in the peripheral blood of asthmatic patients compared to healthy individuals, and IL-4+ NK cells decreased when the patients recovered owing to erythromycin treatment. This implies a role for NK cells in promoting IgE-mediated ongoing allergic inflammation.
The contradictory results have also been observed in mouse models. The ovalbumin (OVA)-induced asthmatic mouse model is widely used in the study of allergic asthma, and many studies have demonstrated an important role for NK cells at all stages of asthma using this model. OVA sensitization and challenge does not change the total number of NK cells in the lungs, but it selectively increases the number of immature NK cells in the lung draining lymph nodes, as well as upregulating the expression of CD86 on NK cells in both the lungs and lung draining lymph nodes (119). Lack of NK cells either throughout life or just prior to sensitization leads to decreased type 2 cytokine secretion, decreased OVA-specific IgE production, and decreased pulmonary eosinophil infiltration (30, 31). Furthermore, adoptive transfer of OVA-specific T cells from sensitized wild-type (WT) mice, but not mice lacking NK cells, can induce the development of asthma in allergen-challenged RAG−/− mice (31). These results indicate that NK cells are essential for allergic sensitization, and that NK cell-mediated initiation of the type 2 response is probably involved in this process. However, once mice have been sensitized, NK cells may not regulate the established type 2 response but instead they may promote pulmonary eosinophilia, as evidenced by the fact that NK cell depletion during allergen challenge significantly reduces BALF eosinophilia without altering airway hyperresponsiveness or serum OVA-specific IgE levels (119). Nevertheless, Haworth et al. (32) found that depletion of NK cells at the peak of inflammation delays the clearance of airway CD4+ T cells and eosinophils. Taken together, these findings suggest that besides the pathologic role of NK cells in allergic sensitization and inflammation promotion, NK cells also provide protection by contributing to the resolution of allergic lung inflammation in mice with OVA-induced asthma. In house dust mite (HDM)-induced asthma mouse models, HDM exposure leads to the accumulation of NK cells in the BALF and lung draining lymph nodes, as well as the activation of NK cells in the lungs (120). Farhadi et al. (33) have shown that NK cells play a critical role in the pathogenesis of HDM-induced asthma via NKG2D and granzyme B. However, a more recent study demonstrated that NK cells are not required for the development of HDM-induced asthmatic disease (120).
There is evidence that viral infection is associated with the development of asthma, and NK cells have been shown to play an important regulatory role in this setting. In mice with pre-existing allergic inflammation and asthma, the induction of asthma-activated NK cells confers more potent protection against influenza infection (121). Nevertheless, NK cells activated by the viral mimic polyinosinic:polycytidylic acid (poly(I:C)) exacerbate OVA-induced asthma via IL-17a production (122). However, when mice are infected with respiratory syncytial viruses and then subjected to allergic sensitization, NK cells inhibit viral- and bystander allergen-specific type 2 responses, possibly through IFN-γ production (7). Recent studies have reported the presence of an altered microbial composition in patients with asthma, and airway dysbiosis is relevant to the clinical features in these individuals (123, 124). Airway colonization by Haemophilus influenzae and Streptococcus pneumonia at 1 month of age was associated with an increased odds ratio of childhood asthma (125). Although NK cells produced higher levels of IFN-γ during H. influenzae and S. pneumonia infections (15, 23), colonization by H. influenzae and S. pneumonia did not inhibit asthma, in contrast to the anti-asthma role of NK cells during respiratory syncytial virus infections. This may be because H. influenzae and S. pneumonia activate other cell types and pathways involved in asthma occurrence and exacerbations. Moreover, the children were also colonized by many other bacteria, and the integrated effect on NK cells caused by diverse bacteria may lead to variable consequences. Thus, the exact associations between NK cells, airway bacteria and asthma need further study.
The abovementioned contradictory results may be influenced by the fact that asthma-associated factors (such as viral and bacterial infections, obesity, allergens, other environmental insults and corticosteroids) may directly affect NK cell functions and the fact that NK cell-depleting antibodies may impair natural killer T cells (antibodies against NK1.1) or some granulocytes and subsets of T cells in certain conditions (antibodies against asialo-GM1) (126–128). In the future, investigations of the exact roles of NK cells in asthma could be enhanced by using improved tools to specifically deplete lung NK cells, generating transgenic mice that temporarily lack lung NK cells, establishing novel humanized asthmatic mouse models, and carrying out large-scale univariate analyses in asthmatic patients.
COPD, caused mainly by cigarette smoking (CS) and biomass fuel, is a common worldwide healthcare issue (129). Chronic inflammation drives the irreversible airway obstruction in COPD, eventually resulting in a decline in lung function (130). Unlike asthma, COPD typically involves the infiltration of neutrophils, Th1 cells and CD8+ T cells (130). NK cells are also thought to be responsible for the progression of COPD. Although the number of NK cells in the peripheral blood, BALF and lung parenchyma of COPD patients are the same as in smokers without COPD (34, 131), CD57+ cells in pulmonary lymphoid follicles have been reported to be significantly increased in COPD patients compared to in smokers without COPD (132). CS enhances the IL-15 trans-presentation of dendritic cells to induce NK cell priming (133). NK cells exhibit hyperresponsiveness in COPD, as evidenced by the findings that CD16+ NK cells kill autologous lung CD326+ epithelial cells and that NK cells from CS-exposed mice produce higher levels of IFN-γ upon stimulation with cytokines or TLR ligands (poly(I:C), ssRNA40, or ODN1826) (34, 134, 135). An imbalance of activating and inhibitory signaling contributes to NK cell hyperresponsiveness. NK cells from CS-exposed mice show greater cytotoxic activity in response to the NKG2D ligand RAE-1 (134). Moreover, CS-induced lung inflammation is impaired in NKG2D-deficient mice, revealing the critical role of NKG2D in COPD development (134). In addition, the inhibitory receptor CD94 has been found to be decreased on NK cells of COPD patients, which may be related to increased granzyme B production (131). The state of NK cells in the CS-induced COPD mouse model cannot completely recapitulate that in patients with COPD. BALF NK cells displayed comparable cytotoxic potential between current smokers with COPD and ex-smokers with COPD, suggesting that the alterations of NK cells are not solely caused by CS and that other factors such as genetics and infections may contribute. In contrast, the hyperresponsiveness of NK cells was lost following smoking cessation in the CS-induced COPD mouse model, which indicated the limitation of using this model to study COPD and that a better mouse model is urgently needed.
Bronchial colonization by potentially pathogenic microorganisms is frequently found in COPD, and COPD exacerbations are closely associated with viral and bacterial infections (136–138). An NK cell-related mechanism may contribute to enhanced lung inflammation during influenza-induced COPD exacerbations. In a CS-induced COPD mouse model, after influenza infection, NK cells were found to produce more IFN-γ (135). Microbiome analyses of sputum samples from patients with COPD exacerbations demonstrated an alteration in bacterial diversity, with an overrepresentation of the Proteobacteria phylum, which includes most of the bacteria considered to be pathogenic (139). Although chronic colonization by Pseudomonas aeruginosa has been found in COPD patients suffering from exacerbations, in most cases, the exacerbations are due to other pathogenic microorganisms (140, 141). Given that lung NK cells in mice infected with P. aeruginosa produced increased IFN-γ through NKG2D-mediated activation (17), a phenotype similar to influenza-induced exacerbations, it would be fascinating to investigate the precise effect of P. aeruginosa-only infections on COPD exacerbations utilizing murine experiments and to explore the role of NK cell-related NKG2D and IFN-γ in this situation. In addition, colonization by H. influenzae, which can lead to NK cell activation (23), has also been reported in COPD patients (142), but the exact interaction between NK cells and H. influenzae in COPD is yet to be determined. Collectively, local hyperresponsive NK cells are responsible for smoke-induced lung inflammation, leading to accelerated progression of COPD. Therefore, targeting NK cells may represent a new strategy for treating COPD.
Lung cancer is the leading cause of death related to cancer worldwide (143). It is classified into non-small-cell lung cancer (NSCLC; ~80%) and small-cell lung cancer (SCLC; ~20%). NK cells are cytotoxic lymphocytes that were originally identified based on their ability to kill cancer cells, and their potent antitumor effects have been confirmed in numerous tumor types including lung cancer (144, 145). The localization of NK cells in lung cancer is similar in humans and mice; NK cells are located mostly in the invasive margin surrounding the tumor lesions, with rare direct contact with cancer cells (35, 146, 147).
The most direct evidence of an anti-lung cancer role for NK cells comes from Kras-driven spontaneous lung cancer and cancer cell-injection experiments in mice, in which mice lacking NK cells were generated by Nfil3 knockout or administration of antibodies against NK1.1 or asialo-GM1. The lung tumor burden was found to be significantly increased in the mice lacking NK cells (35, 36). However, the robust protective role of NK cells against tumors is limited to the early stage of lung cancer, at least in Kras-driven lung cancer in mice, because NK cells become dysfunctional during the late stage. In mice, NK cell dysfunction in the lung cancer microenvironment mainly manifests as attenuated cytotoxicity, diminished responsiveness and impaired viability (Figure 2) (35).
Figure 2. NK cell dysfunction in lung cancer. NK cells in the lung cancer microenvironment display attenuated cytotoxicity, impaired viability and a distinct phenotype, with downregulated expression of NKG2D, DNAM1, CD16, CD27, NKp30, NKp44, and NKp80 and upregulated expression of NKG2A, KIR2DL1, and KIR2DL2. Two mechanisms are involved in NK cell dysfunction in the lung cancer microenvironment. First, aberrant FBP1 expression in NK cells leads to dysfunction by inhibiting their viability and glycolysis. Second, increased microRNA-183 reduces DPA12 expression in NK cells and thus suppresses NK cells. The initiation of both mechanisms may be associated with tumor microenvironment-derived TGF-β. TGF-β, transforming growth factor-β; FBP1, fructose-1,6-bisphosphatase; IFN, interferon; NK, natural killer; DAP12, DNAX activating protein of12 kDa; TGFβR, transforming growth factor β receptor.
Similar phenomena have been observed in NK cells in tumor tissues of patients with NSCLC. NK cells isolated from tumors in these patients have a decreased cell number, a distinctive receptor expression pattern (downregulated expression of NKp30, NKp80, CD16, DNAM1, ILT2, KIR2DL1, and KIR2DL2, but upregulated expression of NKp44, NKG2A, CD69, and HLA-DR), impaired IFN-γ production and CD107a degranulation, lower cytotoxicity, and a proangiogenic phenotype compared with non-tumoral NK cells (146–149). Moreover, NK cells infiltrating NSCLC are enriched with the CD56brightCD16− subset (146), which is a minor subset among non-tumoral lung NK cells. The enrichment of CD56brightCD16− NK cells is probably due to the exclusion of CD16+ NK cells from lung tumor lesions, because the frequency of CD16− NK cells among leukocytes in lung tissues is comparable in tumor and non-tumor tissues (149). However, whether the loss of CD16+ NK cells is caused by the impaired viability or failure to infiltrate tumor lesions, and how CD56brightCD16− NK cells, which express high levels of the tissue-resident marker CD69 (53), maintain their survival and residency in the lung cancer environment, remain to be determined.
Little is known about NK cells in patients with SCLC. Limited information has shown that, compared to the peripheral blood NK cells of healthy individuals, the peripheral blood NK cells of patients with SCLC are present at the same frequency, exhibit weakened cytotoxicity, and have downregulated NKp46 and perforin expression (150). So far, no studies have reported on the status of NK cells in the tumor microenvironment in patients with SCLC, and this needs to be further investigated.
Platonova et al. (147) found that NK cell infiltration is not correlated with clinical outcomes in NSCLC, similar to the finding by Platonova et al. (147), we recently observed that NK cell depletion in late-stage Kras-driven mouse lung cancer does not influence tumor development (35). The limited prognostic significance of NK cells in NSCLC may be caused by intratumoral NK cell dysfunction in patients who mainly have intermediate- or advanced-stage tumors. Thus, both intratumoral NK cell functions and cell densities may be critical to clinical outcomes in NSCLC. A deeper understanding of the mechanisms associated with NK cell dysfunction in the lung cancer microenvironment will contribute to the development of NK cell-based lung cancer immunotherapy.
NK cell dysfunction in tumor microenvironments can generally be caused by tumor cells, myeloid-derived suppressor cells, macrophages, Tregs and platelets in a contact-dependent manner or via secretion of soluble factors such as transforming growth factor (TGF)-β, IL-10, indoleamine-2,3-dioxygenase, prostaglandin E2, and adenosine (145, 151). However, NK cell characteristics may be affected by their tissue localization, and each tumor type has a unique microenvironment composed of diverse immune cells (53). Whether the abovementioned mechanisms are applicable to NK cell dysfunction in lung cancer is yet to be fully investigated. Among these mechanisms, in Kras-driven lung cancer in mice, TGF-β may be involved in FBP1 upregulation in NK cells, and FBP1-mediated glycolysis inhibition and FBP1-mediated impaired viability have been confirmed to induce NK cell dysfunction (35). Additionally, Donatelli et al. (152) demonstrated that TGF-β-inducible microRNA-183 silenced human NK cells via DNAX-activating protein of 12 kDa (DAP12) depletion. Moreover, higher levels of TGF-β in the human lung cancer microenvironment and reduced DAP12 expression in tumor-associated NK cells were observed simultaneously, further indicating another TGF-β-involved mechanism associated with NK cell dysfunction.
NK cell dysfunction favors tumor immunoevasion, so focusing on restoring NK cell functions represents important potential strategies for inhibiting lung cancer. These strategies include activating NK cells using IL-2/IL-12/IL-15/IL-18, blocking inhibitory receptors on NK cells by targeting NKG2A, KIR2DL1, and KIR2DL2, enhancing NK cell glycolysis by inhibiting FBP1 and altering the immunosuppressive microenvironment by neutralizing TGF-β.
Although the biology of NK cells has been well-documented, most studies have focused on peripheral blood NK cells in humans, and bone marrow and spleen NK cells in mice, and less is known about NK cells in the lungs. Recently, the concepts of tissue-resident NK cells and tissue microenvironments have attracted investigators' attention. This has raised the issue that NK cells may be profoundly affected by their local tissue microenvironment, and the characteristics of NK cells in distinct tissues have gradually been uncovered. Our current knowledge about NK cells in the lungs is from studies on WT and transgenic mice and studies comparing healthy individual samples and patient samples (predominately lung tumor resection samples and, less frequently, BALF and sputum samples). The present review shows that NK cells in the lungs appear to be conserved between humans and mice regarding several aspects, including the high degree of differentiation, hypofunction and tissue localization during homeostasis, and responses to tumors and influenza infections. Thus, elaborate mouse models that closely mimic human disease have helped to understand the biology of NK cells in the lungs, such as the Kras-driven lung cancer mouse model and influenza viral infection mouse model. However, for some common lung diseases such as tuberculosis, the lack of a good mouse model and the difficulty in obtaining lung tissue from patients had led to a lack of understanding of NK cells in these conditions. Moreover, as mouse NK cells do not express CD56 and KIRs, which are important for human NK cells, the characteristics and functions of different human lung NK cell subsets subdivided by these molecules remain unclear.
Over the past decade, ILCs, which include NK cells, ILC1s, ILC2s, ILC3s, and lymphoid tissue-inducer cells, have emerged as an important cell population with potent roles in host defense in mucosal tissues including lung tissues (153, 154). Generally, ILC1s respond to tumors and intracellular pathogens such as viruses, ILC2s react to extracellular helminths and allergens and ILC3s resist extracellular microbes such as fungi and bacteria; some of these effects have been demonstrated in the lungs (154–158). Although other ILCs in the lungs are far less abundant than NK cells, ILC1s and parts of ILC3s are easily confused with NK cells (43, 44), so previous conclusions about lung NK cells may be influenced by the effects of other ILCs. Thus, it is necessary to exclude other ILCs to further investigate the exact characteristics of lung NK cells.
With regard to lung NK cell research, several interesting issues remain to be solved: (i) whether lung-resident NK cells are present in the lungs in the context of homeostasis and/or disorders; (ii) if so, how lung-resident NK cells function in certain conditions; (iii) the differences and connections between NK cells and ILCs in the lungs; (iv) why memory-like NK cells induced by influenza virus infection are present in the liver rather than in the lungs; (v) whether memory NK cells can form and be maintained in the lungs (as the lungs are frequently challenged by tumors and pathogens); and (vi) how to establish immunocompetent mouse models that can closely mimic human lung diseases. In the future, advanced technologies and tools, such as humanized mice, omics analyses and living microscopy imaging, may be needed to further study lung NK cells. With deeper knowledge of the biology of lung NK cells, effective therapeutic strategies based on NK cells are expected to be applied to treat lung diseases.
JC wrote the manuscript. HW designed the review and revised the manuscript.
This work was supported by the key project of the National Natural Science Foundation of China (81330071, 81788101, 91442202, and 81602491), The Strategic Priority Research Program of the Chinese Academy of Sciences (CAS grants XDA12020217, XDPB10) and Postdoctoral Innovative Talent Support Program.
The authors declare that the research was conducted in the absence of any commercial or financial relationships that could be construed as a potential conflict of interest.
1. Marquardt N, Kekalainen E, Chen P, Kvedaraite E, Wilson JN, Ivarsson MA, et al. Human lung natural killer cells are predominantly comprised of highly differentiated hypofunctional CD69−CD56dim cells. J Allergy Clin Immunol. (2017) 139:1321–30.e1324. doi: 10.1016/j.jaci.2016.07.043
2. Vivier E, Tomasello E, Baratin M, Walzer T, Ugolini S. Functions of natural killer cells. Nat Immunol. (2008) 9:503–10. doi: 10.1038/ni1582
3. Stein-Streilein J, Guffee J. In vivo treatment of mice and hamsters with antibodies to asialo GM1 increases morbidity and mortality to pulmonary influenza infection. J Immunol. (1986) 136:1435–41.
4. Nogusa S, Ritz BW, Kassim SH, Jennings SR, Gardner EM. Characterization of age-related changes in natural killer cells during primary influenza infection in mice. Mech Ageing Dev. (2008) 129:223–30. doi: 10.1016/j.mad.2008.01.003
5. Abdul-Careem MF, Mian MF, Yue G, Gillgrass A, Chenoweth MJ, Barra NG, et al. Critical role of natural killer cells in lung immunopathology during influenza infection in mice. J Infect Dis. (2012) 206:167–77. doi: 10.1093/infdis/jis340
6. Zhou G, Juang SW, Kane KP. NK cells exacerbate the pathology of influenza virus infection in mice. Eur J Immunol. (2013) 43:929–38. doi: 10.1002/eji.201242620
7. Kaiko GE, Phipps S, Angkasekwinai P, Dong C, Foster PS. NK cell deficiency predisposes to viral-induced Th2-type allergic inflammation via epithelial-derived IL-25. J Immunol. (2010) 185:4681–90. doi: 10.4049/jimmunol.1001758
8. Hussell T, Openshaw PJ. Intracellular IFN-gamma expression in natural killer cells precedes lung CD8+ T cell recruitment during respiratory syncytial virus infection. J Gen Virol. (1998) 79 (Pt 11):2593–601. doi: 10.1099/0022-1317-79-11-2593
9. Hussell T, Openshaw PJ. IL-12-activated NK cells reduce lung eosinophilia to the attachment protein of respiratory syncytial virus but do not enhance the severity of illness in CD8 T cell-immunodeficient conditions. J Immunol. (2000) 165:7109–15. doi: 10.4049/jimmunol.165.12.7109
10. Li F, Zhu H, Sun R, Wei H, Tian Z. Natural killer cells are involved in acute lung immune injury caused by respiratory syncytial virus infection. J Virol. (2012) 86:2251–8. doi: 10.1128/JVI.06209-11
11. Reading PC, Whitney PG, Barr DP, Smyth MJ, Brooks AG. NK cells contribute to the early clearance of HSV-1 from the lung but cannot control replication in the central nervous system following intranasal infection. Eur J Immunol. (2006) 36:897–905. doi: 10.1002/eji.200535710
12. Reading PC, Whitney PG, Barr DP, Wojtasiak M, Mintern JD, Waithman J, et al. IL-18, but not IL-12, regulates NK cell activity following intranasal herpes simplex virus type 1 infection. J Immunol. (2007) 179:3214–21. doi: 10.4049/jimmunol.179.5.3214
13. Xu X, Weiss ID, Zhang HH, Singh SP, Wynn TA, Wilson MS, et al. Conventional NK cells can produce IL-22 and promote host defense in Klebsiella pneumoniae pneumonia. J Immunol. (2014) 192:1778–86. doi: 10.4049/jimmunol.1300039
14. Ivin M, Dumigan A, de Vasconcelos FN, Ebner F, Borroni M, Kavirayani A, et al. Natural killer cell-intrinsic type I IFN signaling controls Klebsiella pneumoniae growth during lung infection. PLoS Pathog. (2017) 13:e1006696. doi: 10.1371/journal.ppat.1006696
15. Elhaik-Goldman S, Kafka D, Yossef R, Hadad U, Elkabets M, Vallon-Eberhard A, et al. The natural cytotoxicity receptor 1 contribution to early clearance of Streptococcus pneumoniae and to natural killer-macrophage cross talk. PLoS ONE. (2011) 6:e23472. doi: 10.1371/journal.pone.0023472
16. Kerr AR, Kirkham LA, Kadioglu A, Andrew PW, Garside P, Thompson H, et al. Identification of a detrimental role for NK cells in pneumococcal pneumonia and sepsis in immunocompromised hosts. Microbes Infect. (2005) 7:845–52. doi: 10.1016/j.micinf.2005.02.011
17. Borchers MT, Harris NL, Wesselkamper SC, Zhang S, Chen Y, Young L, et al. The NKG2D-activating receptor mediates pulmonary clearance of Pseudomonas aeruginosa. Infect Immun. (2006) 74:2578–86. doi: 10.1128/IAI.74.5.2578-2586.2006
18. Wesselkamper SC, Eppert BL, Motz GT, Lau GW, Hassett DJ, Borchers MT. NKG2D is critical for NK cell activation in host defense against Pseudomonas aeruginosa respiratory infection. J Immunol. (2008) 181:5481–9. doi: 10.4049/jimmunol.181.8.5481
19. Feng CG, Kaviratne M, Rothfuchs AG, Cheever A, Hieny S, Young HA, et al. NK cell-derived IFN-differentially regulates innate resistance and neutrophil response in T cell-deficient hosts infected with Mycobacterium tuberculosis. J Immunol. (2006) 177:7086–93. doi: 10.4049/jimmunol.177.10.7086
20. Byrne P, McGuirk P, Todryk S, Mills KH. Depletion of NK cells results in disseminating lethal infection with Bordetella pertussis associated with a reduction of antigen-specific Th1 and enhancement of Th2, but not Tr1 cells. Eur J Immunol. (2004) 34:2579–88. doi: 10.1002/eji.200425092
21. Yoshihara R, Shiozawa S, Fujita T, Chihara K. Gamma interferon is produced by human natural killer cells but not T cells during Staphylococcus aureus stimulation. Infect Immun. (1993) 61:3117–22.
22. Small CL, McCormick S, Gill N, Kugathasan K, Santosuosso M, Donaldson N, et al. NK cells play a critical protective role in host defense against acute extracellular Staphylococcus aureus bacterial infection in the lung. J Immunol. (2008) 180:5558–68. doi: 10.4049/jimmunol.180.8.5558
23. Miyazaki S, Ishikawa F, Shimizu K, Ubagai T, Edelstein PH, Yamaguchi K. Gr-1high polymorphonuclear leukocytes and NK cells act via IL-15 to clear intracellular Haemophilus influenzae in experimental murine peritonitis and pneumonia. J Immunol. (2007) 179:5407–14. doi: 10.4049/jimmunol.179.8.5407
24. Li J, Dong X, Zhao L, Wang X, Wang Y, Yang X, et al. Natural killer cells regulate Th1/Treg and Th17/Treg balance in chlamydial lung infection. J Cell Mol Med. (2016) 20:1339–51. doi: 10.1111/jcmm.12821
25. Morrison BE, Park SJ, Mooney JM, Mehrad B. Chemokine-mediated recruitment of NK cells is a critical host defense mechanism in invasive aspergillosis. J Clin Invest. (2003) 112:1862–70. doi: 10.1172/JCI18125
26. Park SJ, Hughes MA, Burdick M, Strieter RM, Mehrad B. Early NK cell-derived IFN-{gamma} is essential to host defense in neutropenic invasive aspergillosis. J Immunol. (2009) 182:4306–12. doi: 10.4049/jimmunol.0803462
27. Lipscomb MF, Alvarellos T, Toews GB, Tompkins R, Evans Z, Koo G, et al. Role of natural killer cells in resistance to Cryptococcus neoformans infections in mice. Am J Pathol. (1987) 128:354–61.
28. Kawakami K, Koguchi Y, Qureshi MH, Miyazato A, Yara S, Kinjo Y, et al. IL-18 contributes to host resistance against infection with Cryptococcus neoformans in mice with defective IL-12 synthesis through induction of IFN-gamma production by NK cells. J Immunol. (2000) 165:941–7. doi: 10.4049/jimmunol.165.2.941
29. Barnig C, Cernadas M, Dutile S, Liu X, Perrella MA, Kazani S, et al. Lipoxin A4 regulates natural killer cell and type 2 innate lymphoid cell activation in asthma. Sci Transl Med. (2013) 5:174ra126. doi: 10.1126/scitranslmed.3004812
30. Korsgren M, Persson CGA, Sundler F, Bjerke T, Hansson T, Chambers BJ, et al. Natural killer cells determine development of allergen-induced eosinophilic airway inflammation in mice. J Exp Med. (1999) 189:553–62. doi: 10.1084/jem.189.3.553
31. Mathias CB, Guernsey LA, Zammit D, Brammer C, Wu CA, Thrall RS, et al. Pro-inflammatory role of natural killer cells in the development of allergic airway disease. Clin Exp Allergy. (2014) 44:589–601. doi: 10.1111/cea.12271
32. Haworth O, Cernadas M, Levy BD. NK cells are effectors for resolvin E1 in the timely resolution of allergic airway inflammation. J Immunol. (2011) 186:6129–35. doi: 10.4049/jimmunol.1004007
33. Farhadi N, Lambert L, Triulzi C, Openshaw PJM, Guerra N, Culley FJ. Natural killer cell NKG2D and granzyme B are critical for allergic pulmonary inflammation. J Allergy Clin Immunol. (2014) 133:827–35.e823. doi: 10.1016/j.jaci.2013.09.048
34. Freeman CM, Stolberg VR, Crudgington S, Martinez FJ, Han MK, Chensue SW, et al. Human CD56+ cytotoxic lung lymphocytes kill autologous lung cells in chronic obstructive pulmonary disease. PLoS ONE. (2014) 9:e103840. doi: 10.1371/journal.pone.0103840
35. Cong J, Wang X, Zheng X, Wang D, Fu B, Sun R, et al. Dysfunction of natural killer cells by FBP1-induced inhibition of glycolysis during lung cancer progression. Cell Metab. (2018) 28:243–55.e245. doi: 10.1016/j.cmet.2018.06.021
36. Takeda K, Nakayama M, Sakaki M, Hayakawa Y, Imawari M, Ogasawara K, et al. IFN-gamma production by lung NK cells is critical for the natural resistance to pulmonary metastasis of B16 melanoma in mice. J Leukoc Biol. (2011) 90:777–85. doi: 10.1189/jlb.0411208
37. Wei H, Zhang J, Xiao W, Feng J, Sun R, Tian Z. Involvement of human natural killer cells in asthma pathogenesis: natural killer 2 cells in type 2 cytokine predominance. J Allergy Clin Immunol. (2005) 115:841–7. doi: 10.1016/j.jaci.2004.11.026
38. Culley FJ. Natural killer cells in infection and inflammation of the lung. Immunology. (2009) 128:151–63. doi: 10.1111/j.1365-2567.2009.03167.x
39. Hammer Q, Ruckert T, Romagnani C. Natural killer cell specificity for viral infections. Nat Immunol. (2018) 19:800–8. doi: 10.1038/s41590-018-0163-6
40. Lanier LL, Le AM, Civin CI, Loken MR, Phillips JH. The relationship of CD16 (Leu-11) and Leu-19 (NKH-1) antigen expression on human peripheral blood NK cells and cytotoxic T lymphocytes. J Immunol. (1986) 136:4480–6.
41. Cooper MA, Fehniger TA, Turner SC, Chen KS, Ghaheri BA, Ghayur T, et al. Human natural killer cells: a unique innate immunoregulatory role for the CD56bright subset. Blood. (2001) 97:3146–51. doi: 10.1182/blood.V97.10.3146
42. Fauriat C, Long EO, Ljunggren HG, Bryceson YT. Regulation of human NK-cell cytokine and chemokine production by target cell recognition. Blood. (2010) 115:2167–76. doi: 10.1182/blood-2009-08-238469
43. Narni-Mancinelli E, Chaix J, Fenis A, Kerdiles YM, Yessaad N, Reynders A, et al. Fate mapping analysis of lymphoid cells expressing the NKp46 cell surface receptor. Proc Natl Acad Sci USA. (2011) 108:18324–9. doi: 10.1073/pnas.1112064108
44. Tomasello E, Yessaad N, Gregoire E, Hudspeth K, Luci C, Mavilio D, et al. Mapping of NKp46+ cells in healthy human lymphoid and non-lymphoid tissues. Front Immunol. (2012) 3:344. doi: 10.3389/fimmu.2012.00344
45. Kim S, Iizuka K, Kang HS, Dokun A, French AR, Greco S, et al. In vivo developmental stages in murine natural killer cell maturation. Nat Immunol. (2002) 3:523–8. doi: 10.1038/ni796
46. Hayakawa Y, Huntington ND, Nutt SL, Smyth MJ. Functional subsets of mouse natural killer cells. Immunol Rev. (2006) 214:47–55. doi: 10.1111/j.1600-065X.2006.00454.x
47. Hayakawa Y, Smyth MJ. CD27 dissects mature NK cells into two subsets with distinct responsiveness and migratory capacity. J Immunol. (2006) 176:1517–24. doi: 10.4049/jimmunol.176.3.1517
48. Diefenbach A, Raulet DH. Innate immune recognition by stimulatory immunoreceptors. Curr Opin Immunol. (2003) 15:37–44. doi: 10.1016/S0952-7915(02)00007-9
49. Lanier LL. NK cell recognition. Annu Rev Immunol. (2005) 23:225–74. doi: 10.1146/annurev.immunol.23.021704.115526
50. Raulet DH, Vance RE. Self-tolerance of natural killer cells. Nat Rev Immunol. (2006) 6:520–31. doi: 10.1038/nri1863
51. Walzer T, Dalod M, Robbins SH, Zitvogel L, Vivier E. Natural-killer cells and dendritic cells: “l'union fait la force”. Blood. (2005) 106:2252–8. doi: 10.1182/blood-2005-03-1154
52. Guillerey C, Chow MT, Miles K, Olver S, Sceneay J, Takeda K, et al. Toll-like receptor 3 regulates NK cell responses to cytokines and controls experimental metastasis. Oncoimmunology. (2015) 4:e1027468. doi: 10.1080/2162402X.2015.1027468
53. Björkström NK, Ljunggren H-G, Michaëlsson J. Emerging insights into natural killer cells in human peripheral tissues. Nat Rev Immunol. (2016) 16:310–20. doi: 10.1038/nri.2016.34
54. Trinchieri G. Biology of natural killer cells. Adv Immunol. (1989) 47:187–376. doi: 10.1016/S0065-2776(08)60664-1
55. Gregoire C, Chasson L, Luci C, Tomasello E, Geissmann F, Vivier E, et al. The trafficking of natural killer cells. Immunol Rev. (2007) 220:169–82. doi: 10.1111/j.1600-065X.2007.00563.x
56. Wang J, Li F, Zheng M, Sun R, Wei H, Tian Z. Lung natural killer cells in mice: phenotype and response to respiratory infection. Immunology. (2012) 137:37–47. doi: 10.1111/j.1365-2567.2012.03607.x
57. Ivarsson MA, Loh L, Marquardt N, Kekäläinen E, Berglin L, Björkström NK, et al. Differentiation and functional regulation of human fetal NK cells. J Clin Invest. (2013) 123:3889–901. doi: 10.1172/JCI68989
58. Michel T, Poli A, Domingues O, Mauffray M, Theresine M, Brons NH, et al. Mouse lung and spleen natural killer cells have phenotypic and functional differences, in part influenced by macrophages. PLoS ONE. (2012) 7:e51230. doi: 10.1371/journal.pone.0051230
59. Robinson BW, Pinkston P, Crystal RG. Natural killer cells are present in the normal human lung but are functionally impotent. J Clin Invest. (1984) 74:942–50. doi: 10.1172/JCI111513
60. Koopman LA, Kopcow HD, Rybalov B, Boyson JE, Orange JS, Schatz F, et al. Human decidual natural killer cells are a unique NK cell subset with immunomodulatory potential. J Exp Med. (2003) 198:1201–12. doi: 10.1084/jem.20030305
61. Peng H, Jiang X, Chen Y, Sojka DK, Wei H, Gao X, et al. Liver-resident NK cells confer adaptive immunity in skin-contact inflammation. J Clin Invest. (2013) 123:1444–56. doi: 10.1172/JCI66381
62. Montaldo E, Vacca P, Chiossone L, Croxatto D, Loiacono F, Martini S, et al. Unique Eomes+ NK cell subsets are present in uterus and decidua during early pregnancy. Front Immunol. (2015) 6:646. doi: 10.3389/fimmu.2015.00646
63. Lugthart G, Melsen JE, Vervat C, van Ostaijen-Ten Dam MM, Corver WE, Roelen DL, et al. Human lymphoid tissues harbor a distinct CD69+CXCR6+ NK cell population. J Immunol. (2016) 197:78–84. doi: 10.4049/jimmunol.1502603
64. Sojka DK, Plougastel-Douglas B, Yang L, Pak-Wittel MA, Artyomov MN, Ivanova Y, et al. Tissue-resident natural killer (NK) cells are cell lineages distinct from thymic and conventional splenic NK cells. eLife. (2014) 3:01659. doi: 10.7554/eLife.01659
65. Gasteiger G, Fan X, Dikiy S, Lee SY, Rudensky AY. Tissue residency of innate lymphoid cells in lymphoid and nonlymphoid organs. Science. (2015) 350:981–5. doi: 10.1126/science.aac9593
66. Cooper GE, Ostridge K, Khakoo SI, Wilkinson TMA, Staples KJ. Human CD49a+ lung natural killer cell cytotoxicity in response to influenza A virus. Front Immunol. (2018) 9:1671. doi: 10.3389/fimmu.2018.01671
67. Zhou Y, Xu X, Tian Z, Wei H. “Multi-omics” analyses of the development and function of natural killer cells. Front Immunol. (2017) 8:1095. doi: 10.3389/fimmu.2017.01095
68. Orange JS. Human natural killer cell deficiencies and susceptibility to infection. Microbes Infect. (2002) 4:1545–58. doi: 10.1016/S1286-4579(02)00038-2
69. Orange JS, Brodeur SR, Jain A, Bonilla FA, Schneider LC, Kretschmer R, et al. Deficient natural killer cell cytotoxicity in patients with IKK-γ/NEMO mutations. J Clin Invest. (2002) 109:1501–9. doi: 10.1172/JCI0214858
70. Ennis FA, Meager A, Beare AS, Qi YH, Riley D, Schwarz G, et al. Interferon induction and increased natural killer-cell activity in influenza infections in man. Lancet. (1981) 2:891–3. doi: 10.1016/S0140-6736(81)91390-8
71. Siren J, Sareneva T, Pirhonen J, Strengell M, Veckman V, Julkunen I, et al. Cytokine and contact-dependent activation of natural killer cells by influenza A or Sendai virus-infected macrophages. J Gen Virol. (2004) 85 (Pt 8):2357–64. doi: 10.1099/vir.0.80105-0
72. Monteiro JM, Harvey C, Trinchieri G. Role of interleukin-12 in primary influenza virus infection. J Virol. (1998) 72:4825–31.
73. He X-S, Draghi M, Mahmood K, Holmes TH, Kemble GW, Dekker CL, et al. T cell–dependent production of IFN-γ by NK cells in response to influenza A virus. J Clin Invest. (2004) 114:1812–9. doi: 10.1172/JCI22797
74. Hwang I, Scott JM, Kakarla T, Duriancik DM, Choi S, Cho C, et al. Activation mechanisms of natural killer cells during influenza virus infection. PLoS ONE. (2012) 7:e51858. doi: 10.1371/journal.pone.0051858
75. Arimori Y, Nakamura R, Yamada H, Shibata K, Maeda N, Kase T, et al. Type I interferon plays opposing roles in cytotoxicity and interferon-gamma production by natural killer and CD8 T cells after influenza A virus infection in mice. J Innate Immun. (2014) 6:456–66. doi: 10.1159/000356824
76. Stegemann-Koniszewski S, Behrens S, Boehme JD, Hochnadel I, Riese P, Guzman CA, et al. Respiratory influenza A virus infection triggers local and systemic natural killer cell activation via toll-like receptor 7. Front Immunol. (2018) 9:245. doi: 10.3389/fimmu.2018.00245
77. Arnon TI, Lev M, Katz G, Chernobrov Y, Porgador A, Mandelboim O. Recognition of viral hemagglutinins by NKp44 but not by NKp30. Eur J Immunol. (2001) 31:2680–9. doi: 10.1002/1521-4141(200109)31:9<2680::AID-IMMU2680>3.0.CO;2-A
78. Mandelboim O, Lieberman N, Lev M, Paul L, Arnon TI, Bushkin Y, et al. Recognition of haemagglutinins on virus-infected cells by NKp46 activates lysis by human NK cells. Nature. (2001) 409:1055–60. doi: 10.1038/35059110
79. Arnon TI, Achdout H, Lieberman N, Gazit R, Gonen-Gross T, Katz G, et al. The mechanisms controlling the recognition of tumor- and virus-infected cells by NKp46. Blood. (2004) 103:664–72. doi: 10.1182/blood-2003-05-1716
80. Ho JW, Hershkovitz O, Peiris M, Zilka A, Bar-Ilan A, Nal B, et al. H5-type influenza virus hemagglutinin is functionally recognized by the natural killer-activating receptor NKp44. J Virol. (2008) 82:2028–32. doi: 10.1128/JVI.02065-07
81. Dou Y, Fu B, Sun R, Li W, Hu W, Tian Z, et al. Influenza vaccine induces intracellular immune memory of human NK cells. PLoS ONE. (2015) 10:e0121258. doi: 10.1371/journal.pone.0121258
82. Li T, Wang J, Wang Y, Chen Y, Wei H, Sun R, et al. Respiratory influenza virus infection induces memory-like liver NK cells in mice. J Immunol. (2017) 198:1242–52. doi: 10.4049/jimmunol.1502186
83. Peng H, Sun R. Liver-resident NK cells and their potential functions. Cell Mol Immunol. (2017) 14:890–4. doi: 10.1038/cmi.2017.72
84. Wang X, Peng H, Tian Z. Innate lymphoid cell memory. Cell Mol Immunol. (2019) 16:423–9. doi: 10.1038/s41423-019-0212-6
85. Paust S, Gill HS, Wang BZ, Flynn MP, Moseman EA, Senman B, et al. Critical role for the chemokine receptor CXCR6 in NK cell-mediated antigen-specific memory of haptens and viruses. Nat Immunol. (2010) 11:1127–35. doi: 10.1038/ni.1953
86. Stein-Streilein J, Bennett M, Mann D, Kumar V. Natural killer cells in mouse lung: surface phenotype, target preference, and response to local influenza virus infection. J Immunol. (1983) 131:2699–704.
87. Kumar P, Thakar MS, Ouyang W, Malarkannan S. IL-22 from conventional NK cells is epithelial regenerative and inflammation protective during influenza infection. Mucosal Immunol. (2012) 6:69–82. doi: 10.1038/mi.2012.49
88. He XS, Holmes TH, Zhang C, Mahmood K, Kemble GW, Lewis DB, et al. Cellular immune responses in children and adults receiving inactivated or live attenuated influenza vaccines. J Virol. (2006) 80:11756–66. doi: 10.1128/JVI.01460-06
89. Draghi M, Pashine A, Sanjanwala B, Gendzekhadze K, Cantoni C, Cosman D, et al. NKp46 and NKG2D recognition of infected dendritic cells is necessary for NK cell activation in the human response to influenza infection. J Immunol. (2007) 178:2688–98. doi: 10.4049/jimmunol.178.5.2688
90. Long BR, Michaelsson J, Loo CP, Ballan WM, Vu BA, Hecht FM, et al. Elevated frequency of gamma interferon-producing NK cells in healthy adults vaccinated against influenza virus. Clin Vaccine Immunol. (2008) 15:120–30. doi: 10.1128/CVI.00357-07
91. Romee R, Foley B, Lenvik T, Wang Y, Zhang B, Ankarlo D, et al. NK cell CD16 surface expression and function is regulated by a disintegrin and metalloprotease-17 (ADAM17). Blood. (2013) 121:3599–608. doi: 10.1182/blood-2012-04-425397
92. Schultz-Cherry S. Role of NK cells in influenza infection. In: Oldstone MBA, Compans RW, editors. Influenza Pathogenesis and Control, Vol. II. Cham: Springer International Publishing (2015). p. 109–20. doi: 10.1007/82_2014_403
93. Guo H, Kumar P, Malarkannan S. Evasion of natural killer cells by influenza virus. J Leukoc Biol. (2011) 89:189–94. doi: 10.1189/jlb.0610319
94. Owen RE, Yamada E, Thompson CI, Phillipson LJ, Thompson C, Taylor E, et al. Alterations in receptor binding properties of recent human influenza H3N2 viruses are associated with reduced natural killer cell lysis of infected cells. J Virol. (2007) 81:11170–8. doi: 10.1128/JVI.01217-07
95. Ali SA, Rees RC, Oxford J. Modulation of human natural killer cytotoxicity by influenza virus and its subunit protein. Immunology. (1984) 52:687–95.
96. Mao H, Tu W, Liu Y, Qin G, Zheng J, Chan PL, et al. Inhibition of human natural killer cell activity by influenza virions and hemagglutinin. J Virol. (2010) 84:4148–57. doi: 10.1128/JVI.02340-09
97. Stein-Streilein J, Witte PL, Streilein JW, Guffee J. Local cellular defenses in influenza-infected lungs. Cell Immunol. (1985) 95:234–46. doi: 10.1016/0008-8749(85)90311-9
98. Mao H, Tu W, Qin G, Law HK, Sia SF, Chan PL, et al. Influenza virus directly infects human natural killer cells and induces cell apoptosis. J Virol. (2009) 83:9215–22. doi: 10.1128/JVI.00805-09
99. Shen H, Chen ZW. The crucial roles of Th17-related cytokines/signal pathways in M. tuberculosis infection. Cell Mol Immunol. (2018) 15:216–25. doi: 10.1038/cmi.2017.128
100. Vankayalapati R, Garg A, Porgador A, Griffith DE, Klucar P, Safi H, et al. Role of NK cell-activating receptors and their ligands in the lysis of mononuclear phagocytes infected with an intracellular bacterium. J Immunol. (2005) 175:4611–7. doi: 10.4049/jimmunol.175.7.4611
101. Garg A, Barnes PF, Porgador A, Roy S, Wu S, Nanda JS, et al. Vimentin expressed on Mycobacterium tuberculosis-infected human monocytes is involved in binding to the NKp46 receptor. J Immunol. (2006) 177:6192–8. doi: 10.4049/jimmunol.177.9.6192
102. Esin S, Batoni G, Counoupas C, Stringaro A, Brancatisano FL, Colone M, et al. Direct binding of human NK cell natural cytotoxicity receptor NKp44 to the surfaces of mycobacteria and other bacteria. Infect Immun. (2008) 76:1719–27. doi: 10.1128/IAI.00870-07
103. Marcenaro E, Ferranti B, Falco M, Moretta L, Moretta A. Human NK cells directly recognize Mycobacterium bovis via TLR2 and acquire the ability to kill monocyte-derived DC. Int Immunol. (2008) 20:1155–67. doi: 10.1093/intimm/dxn073
104. Esin S, Counoupas C, Aulicino A, Brancatisano FL, Maisetta G, Bottai D, et al. Interaction of Mycobacterium tuberculosis cell wall components with the human natural killer cell receptors NKp44 and Toll-like receptor 2. Scand J Immunol. (2013) 77:460–9. doi: 10.1111/sji.12052
105. Junqueira-Kipnis AP, Kipnis A, Jamieson A, Juarrero MG, Diefenbach A, Raulet DH, et al. NK cells respond to pulmonary infection with Mycobacterium tuberculosis, but play a minimal role in protection. J Immunol. (2003) 171:6039–45. doi: 10.4049/jimmunol.171.11.6039
106. Liu CH, Liu H, Ge B. Innate immunity in tuberculosis: host defense vs pathogen evasion. Cell Mol Immunol. (2017) 14:963–75. doi: 10.1038/cmi.2017.88
107. Gerosa F, Baldani-Guerra B, Nisii C, Marchesini V, Carra G, Trinchieri G. Reciprocal activating interaction between natural killer cells and dendritic cells. J Exp Med. (2002) 195:327–33. doi: 10.1084/jem.20010938
108. Feinberg J, Fieschi C, Doffinger R, Feinberg M, Leclerc T, Boisson-Dupuis S, et al. Bacillus Calmette Guerin triggers the IL-12/IFN-gamma axis by an IRAK-4- and NEMO-dependent, non-cognate interaction between monocytes, NK, and T lymphocytes. Eur J Immunol. (2004) 34:3276–84. doi: 10.1002/eji.200425221
109. Chowdhury RR, Vallania F, Yang Q, Lopez Angel CJ, Darboe F, Penn-Nicholson A, et al. A multi-cohort study of the immune factors associated with M. tuberculosis infection outcomes. Nature. (2018) 560:644–8. doi: 10.1038/s41586-018-0439-x
110. Schierloh P, Yokobori N, Aleman M, Musella RM, Beigier-Bompadre M, Saab MA, et al. Increased susceptibility to apoptosis of CD56dimCD16+ NK cells induces the enrichment of IFN- -producing CD56bright cells in tuberculous pleurisy. J Immunol. (2005) 175:6852–60. doi: 10.4049/jimmunol.175.10.6852
111. Chalifour A, Jeannin P, Gauchat JF, Blaecke A, Malissard M, N'Guyen T, et al. Direct bacterial protein PAMP recognition by human NK cells involves TLRs and triggers alpha-defensin production. Blood. (2004) 104:1778–83. doi: 10.1182/blood-2003-08-2820
112. Wang J, Li F, Sun R, Gao X, Wei H, Tian Z. Klebsiella pneumoniae alleviates influenza-induced acute lung injury via limiting NK cell expansion. J Immunol. (2014) 193:1133–41. doi: 10.4049/jimmunol.1303303
113. Wilson MS, Wynn TA. Pulmonary fibrosis: pathogenesis, etiology and regulation. Mucosal Immunol. (2009) 2:103–21. doi: 10.1038/mi.2008.85
114. Barnes PJ. Targeting cytokines to treat asthma and chronic obstructive pulmonary disease. Nat Rev Immunol. (2018) 18:454–66. doi: 10.1038/s41577-018-0006-6
115. Lin S-J, Chang L-Y, Yan D-C, Huang Y-J, Lin T-J, Lin T-Y. Decreased intercellular adhesion molecule-1 (CD54) and L-selectin (CD62L) expression on peripheral blood natural killer cells in asthmatic children with acute exacerbation. Allergy. (2003) 58:67–71. doi: 10.1034/j.1398-9995.2003.t01-1-23697.x
116. Duvall MG, Barnig C, Cernadas M, Ricklefs I, Krishnamoorthy N, Grossman NL, et al. Natural killer cell-mediated inflammation resolution is disabled in severe asthma. Sci Immunol. (2017) 2:5446. doi: 10.1126/sciimmunol.aam5446
117. Jira M, Antosova E, Vondra V, Strejcek J, Mazakova H, Prazakova J. Natural killer and interleukin-2 induced cytotoxicity in asthmatics. Allergy. (1988) 43:294–8. doi: 10.1111/j.1398-9995.1988.tb00903.x
118. Di Lorenzo G, Esposito Pellitteri M, Drago A, Di Blasi P, Candore G, Balistreri C, et al. Effects of in vitro treatment with fluticasone propionate on natural killer and lymphokine-induced killer activity in asthmatic and healthy individuals. Allergy. (2001) 56:323–7. doi: 10.1034/j.1398-9995.2001.00879.x
119. Ple C, Barrier M, Amniai L, Marquillies P, Bertout J, Tsicopoulos A, et al. Natural killer cells accumulate in lung-draining lymph nodes and regulate airway eosinophilia in a murine model of asthma. Scand J Immunol. (2010) 72:118–27. doi: 10.1111/j.1365-3083.2010.02419.x
120. Haspeslagh E, van Helden MJ, Deswarte K, De Prijck S, van Moorleghem J, Boon L, et al. Role of NKp46+ natural killer cells in house dust mite-driven asthma. EMBO Mol Med. (2018) 10:e8657. doi: 10.15252/emmm.201708657
121. Ishikawa H, Sasaki H, Fukui T, Fujita K, Kutsukake E, Matsumoto T. Mice with asthma are more resistant to influenza virus infection and NK cells activated by the induction of asthma have potentially protective effects. J Clin Immunol. (2012) 32:256–67. doi: 10.1007/s10875-011-9619-2
122. Lunding LP, Webering S, Vock C, Behrends J, Wagner C, Holscher C, et al. Poly(inosinic-cytidylic) acid-triggered exacerbation of experimental asthma depends on IL-17A produced by NK cells. J Immunol. (2015) 194:5615–25. doi: 10.4049/jimmunol.1402529
123. Marri PR, Stern DA, Wright AL, Billheimer D, Martinez FD. Asthma-associated differences in microbial composition of induced sputum. J Allergy Clin Immunol. (2013) 131:346–52.e341–3. doi: 10.1016/j.jaci.2012.11.013
124. Huang YJ, Nariya S, Harris JM, Lynch SV, Choy DF, Arron JR, et al. The airway microbiome in patients with severe asthma: associations with disease features and severity. J Allergy Clin Immunol. (2015) 136:874–84. doi: 10.1016/j.jaci.2015.05.044
125. Bisgaard H, Hermansen MN, Buchvald F, Loland L, Halkjaer LB, Bonnelykke K, et al. Childhood asthma after bacterial colonization of the airway in neonates. N Engl J Med. (2007) 357:1487–95. doi: 10.1056/NEJMoa052632
126. Lee U, Santa K, Habu S, Nishimura T. Murine asialo GM1+CD8+ T cells as novel interleukin-12-responsive killer T cell precursors. Jpn J Cancer Res. (1996) 87:429–32. doi: 10.1111/j.1349-7006.1996.tb00241.x
127. Slifka MK, Pagarigan RR, Whitton JL. NK markers are expressed on a high percentage of virus-specific CD8+ and CD4+ T cells. J Immunol. (2000) 164:2009–15. doi: 10.4049/jimmunol.164.4.2009
128. Nishikado H, Mukai K, Kawano Y, Minegishi Y, Karasuyama H. NK cell-depleting anti-asialo GM1 antibody exhibits a lethal off-target effect on basophils in vivo. J Immunol. (2011) 186:5766–71. doi: 10.4049/jimmunol.1100370
129. Rabe KF, Watz H. Chronic obstructive pulmonary disease. Lancet. (2017) 389:1931–40. doi: 10.1016/S0140-6736(17)31222-9
130. Barnes PJ. Immunology of asthma and chronic obstructive pulmonary disease. Nat Rev Immunol. (2008) 8:183–92. doi: 10.1038/nri2254
131. Hodge G, Mukaro V, Holmes M, Reynolds PN, Hodge S. Enhanced cytotoxic function of natural killer and natural killer T-like cells associated with decreased CD94 (Kp43) in the chronic obstructive pulmonary disease airway. Respirology. (2013) 18:369–76. doi: 10.1111/j.1440-1843.2012.02287.x
132. Olloquequi J, Montes JF, Prats A, Rodriguez E, Montero MA, Garcia-Valero J, et al. Significant increase of CD57+ cells in pulmonary lymphoid follicles of COPD patients. Eur Respir J. (2011) 37:289–98. doi: 10.1183/09031936.00201509
133. Finch DK, Stolberg VR, Ferguson J, Alikaj H, Kady MR, Richmond BW, et al. Lung dendritic cells drive natural killer cytotoxicity in chronic obstructive pulmonary disease via IL-15Ralpha. Am J Respir Crit Care Med. (2018) 198:1140–50. doi: 10.1164/rccm.201712-2513OC
134. Motz GT, Eppert BL, Wortham BW, Amos-Kroohs RM, Flury JL, Wesselkamper SC, et al. Chronic cigarette smoke exposure primes NK cell activation in a mouse model of chronic obstructive pulmonary disease. J Immunol. (2010) 184:4460–9. doi: 10.4049/jimmunol.0903654
135. Wortham BW, Eppert BL, Motz GT, Flury JL, Orozco-Levi M, Hoebe K, et al. NKG2D mediates NK cell hyperresponsiveness and influenza-induced pathologies in a mouse model of chronic obstructive pulmonary disease. J Immunol. (2012) 188:4468–75. doi: 10.4049/jimmunol.1102643
136. Celli BR, Barnes PJ. Exacerbations of chronic obstructive pulmonary disease. Eur Respir J. (2007) 29:1224–38. doi: 10.1183/09031936.00109906
137. Sethi S, Murphy TF. Infection in the pathogenesis and course of chronic obstructive pulmonary disease. N Engl J Med. (2008) 359:2355–65. doi: 10.1056/NEJMra0800353
138. Marin A, Monso E, Garcia-Nunez M, Sauleda J, Noguera A, Pons J, et al. Variability and effects of bronchial colonisation in patients with moderate COPD. Eur Respir J. (2010) 35:295–302. doi: 10.1183/09031936.00126808
139. Garcia-Nunez M, Millares L, Pomares X, Ferrari R, Perez-Brocal V, Gallego M, et al. Severity-related changes of bronchial microbiome in chronic obstructive pulmonary disease. J Clin Microbiol. (2014) 52:4217–23. doi: 10.1128/JCM.01967-14
140. Millares L, Ferrari R, Gallego M, Garcia-Nunez M, Perez-Brocal V, Espasa M, et al. Bronchial microbiome of severe COPD patients colonised by Pseudomonas aeruginosa. Eur J Clin Microbiol Infect Dis. (2014) 33:1101–11. doi: 10.1007/s10096-013-2044-0
141. Garcia-Nunez M, Marti S, Puig C, Perez-Brocal V, Millares L, Santos S, et al. Bronchial microbiome, PA biofilm-forming capacity and exacerbation in severe COPD patients colonized by P. aeruginosa Future Microbiol. (2017) 12:379–92. doi: 10.2217/fmb-2016-0127
142. Rosell A, Monso E, Soler N, Torres F, Angrill J, Riise G, et al. Microbiologic determinants of exacerbation in chronic obstructive pulmonary disease. Arch Intern Med. (2005) 165:891–7. doi: 10.1001/archinte.165.8.891
143. Bray F, Ferlay J, Soerjomataram I, Siegel RL, Torre LA, Jemal A. Global cancer statistics 2018: GLOBOCAN estimates of incidence and mortality worldwide for 36 cancers in 185 countries. CA Cancer J Clin. (2018) 68:394–424. doi: 10.3322/caac.21492
144. Malmberg KJ, Carlsten M, Bjorklund A, Sohlberg E, Bryceson YT, Ljunggren HG. Natural killer cell-mediated immunosurveillance of human cancer. Semin Immunol. (2017) 31:20–9. doi: 10.1016/j.smim.2017.08.002
145. Chiossone L, Dumas PY, Vienne M, Vivier E. Natural killer cells and other innate lymphoid cells in cancer. Nat Rev Immunol. (2018) 18:671–88. doi: 10.1038/s41577-018-0061-z
146. Carrega P, Morandi B, Costa R, Frumento G, Forte G, Altavilla G, et al. Natural killer cells infiltrating human nonsmall-cell lung cancer are enriched in CD56bright CD16− cells and display an impaired capability to kill tumor cells. Cancer. (2008) 112:863–75. doi: 10.1002/cncr.23239
147. Platonova S, Cherfils-Vicini J, Damotte D, Crozet L, Vieillard V, Validire P, et al. Profound coordinated alterations of intratumoral NK cell phenotype and function in lung carcinoma. Cancer Res. (2011) 71:5412–22. doi: 10.1158/0008-5472.CAN-10-4179
148. Bruno A, Focaccetti C, Pagani A, Imperatori AS, Spagnoletti M, Rotolo N, et al. The proangiogenic phenotype of natural killer cells in patients with non-small cell lung cancer. Neoplasia. (2013) 15:133–7. doi: 10.1593/neo.121758
149. Lavin Y, Kobayashi S, Leader A, Amir ED, Elefant N, Bigenwald C, et al. Innate immune landscape in early lung adenocarcinoma by paired single-cell analyses. Cell. (2017) 169:750–5.e717. doi: 10.1016/j.cell.2017.04.014
150. Al Omar SY, Marshall E, Middleton D, Christmas SE. Increased killer immunoglobulin-like receptor expression and functional defects in natural killer cells in lung cancer. Immunology. (2011) 133:94–104. doi: 10.1111/j.1365-2567.2011.03415.x
151. Morvan MG, Lanier LL. NK cells and cancer: you can teach innate cells new tricks. Nat Rev Cancer. (2016) 16:7–19. doi: 10.1038/nrc.2015.5
152. Donatelli SS, Zhou JM, Gilvary DL, Eksioglu EA, Chen X, Cress WD, et al. TGF-beta-inducible microRNA-183 silences tumor-associated natural killer cells. Proc Natl Acad Sci USA. (2014) 111:4203–8. doi: 10.1073/pnas.1319269111
153. Artis D, Spits H. The biology of innate lymphoid cells. Nature. (2015) 517:293–301. doi: 10.1038/nature14189
154. Vivier E, Artis D, Colonna M, Diefenbach A, Di Santo JP, Eberl G, et al. Innate lymphoid cells: 10 years on. Cell. (2018) 174:1054–66. doi: 10.1016/j.cell.2018.07.017
155. Van Maele L, Carnoy C, Cayet D, Ivanov S, Porte R, Deruy E, et al. Activation of Type 3 innate lymphoid cells and interleukin 22 secretion in the lungs during Streptococcus pneumoniae infection. J Infect Dis. (2014) 210:493–503. doi: 10.1093/infdis/jiu106
156. Monticelli LA, Buck MD, Flamar AL, Saenz SA, Tait Wojno ED, Yudanin NA, et al. Arginase 1 is an innate lymphoid-cell-intrinsic metabolic checkpoint controlling type 2 inflammation. Nat Immunol. (2016) 17:656–65. doi: 10.1038/ni.3421
157. Vashist N, Trittel S, Ebensen T, Chambers BJ, Guzman CA, Riese P. Influenza-activated ILC1s contribute to antiviral immunity partially influenced by differential GITR expression. Front Immunol. (2018) 9:505. doi: 10.3389/fimmu.2018.00505
Keywords: natural killer cells, lung, homeostasis, inflammation, infection, lung cancer
Citation: Cong J and Wei H (2019) Natural Killer Cells in the Lungs. Front. Immunol. 10:1416. doi: 10.3389/fimmu.2019.01416
Received: 22 March 2019; Accepted: 04 June 2019;
Published: 25 June 2019.
Edited by:
Arthur Mortha, University of Toronto, CanadaReviewed by:
Michele Ardolino, University of Ottawa, CanadaCopyright © 2019 Cong and Wei. This is an open-access article distributed under the terms of the Creative Commons Attribution License (CC BY). The use, distribution or reproduction in other forums is permitted, provided the original author(s) and the copyright owner(s) are credited and that the original publication in this journal is cited, in accordance with accepted academic practice. No use, distribution or reproduction is permitted which does not comply with these terms.
*Correspondence: Haiming Wei, dXN0Y3dobUB1c3RjLmVkdS5jbg==
Disclaimer: All claims expressed in this article are solely those of the authors and do not necessarily represent those of their affiliated organizations, or those of the publisher, the editors and the reviewers. Any product that may be evaluated in this article or claim that may be made by its manufacturer is not guaranteed or endorsed by the publisher.
Research integrity at Frontiers
Learn more about the work of our research integrity team to safeguard the quality of each article we publish.