- 1Systems Biology of Reproduction Momentum Research Group, Institute of Enzymology, Research Centre for Natural Sciences, Hungarian Academy of Sciences, Budapest, Hungary
- 2Department of Immunology, Eotvos Lorand University, Budapest, Hungary
- 3Perinatology Research Branch, Eunice Kennedy Shriver National Institute of Child Health and Human Development, National Institutes of Health, U.S. Department of Health and Human Services, Bethesda, MD and Detroit, MI, United States
- 4Department of Obstetrics and Gynecology, University of Michigan, Ann Arbor, MI, United States
- 5Department of Epidemiology and Biostatistics, Michigan State University, East Lansing, MI, United States
- 6Center for Molecular Medicine and Genetics, Wayne State University, Detroit, MI, United States
- 7Structural Biophysics Research Group, Institute of Enzymology, Research Centre for Natural Sciences, Hungarian Academy of Sciences, Budapest, Hungary
- 8Department of Genetics, Cell and Immunobiology, Semmelweis University, Budapest, Hungary
- 9TeleMarpe Ltd, Tel Aviv, Israel
- 10Maternity Private Clinic of Obstetrics and Gynecology, Budapest, Hungary
- 11Department of Obstetrics and Gynecology, Wayne State University School of Medicine, Detroit, MI, United States
- 12Department of Computer Science, Wayne State University College of Engineering, Detroit, MI, United States
- 13Department of Physiology, Wayne State University School of Medicine, Detroit, MI, United States
- 14Division of Obstetrics and Gynecology, Maternity Department “D”, Faculty of Health Sciences, Soroka University Medical Center, School of Medicine, Ben Gurion University of the Negev, Beer-Sheva, Israel
- 15Department of Obstetrics and Gynecology, Semmelweis University, Budapest, Hungary
- 16Department of Biomedicine, University and University Hospital Basel, Basel, Switzerland
- 17First Department of Pathology and Experimental Cancer Research, Semmelweis University, Budapest, Hungary
Galectins are potent immunomodulators that regulate maternal immune responses in pregnancy and prevent the rejection of the semi-allogeneic fetus that also occurs in miscarriages. We previously identified a gene cluster on Chromosome 19 that expresses a subfamily of galectins, including galectin-13 (Gal-13) and galectin-14 (Gal-14), which emerged in anthropoid primates. These galectins are expressed only by the placenta and induce the apoptosis of activated T lymphocytes, possibly contributing to a shifted maternal immune balance in pregnancy. The placental expression of Gal-13 and Gal-14 is decreased in preeclampsia, a life-threatening obstetrical syndrome partly attributed to maternal anti-fetal rejection. This study is aimed at revealing the effects of Gal-13 and Gal-14 on T cell functions and comparing the expression of these galectins in placentas from healthy pregnancies and miscarriages. First-trimester placentas were collected from miscarriages and elective termination of pregnancies, tissue microarrays were constructed, and then the expression of Gal-13 and Gal-14 was analyzed by immunohistochemistry and immunoscoring. Recombinant Gal-13 and Gal-14 were expressed and purified, and their effects were investigated on primary peripheral blood T cells. The binding of Gal-13 and Gal-14 to T cells and the effects of these galectins on apoptosis, activation marker (CD25, CD71, CD95, HLA-DR) expression and cytokine (IL-1β, IL-6, IL-8, IL-10, IFNγ) production of T cells were examined by flow cytometry. Gal-13 and Gal-14 are primarily expressed by the syncytiotrophoblast at the maternal-fetal interface in the first trimester, and their placental expression is decreased in miscarriages compared to first-trimester controls. Recombinant Gal-13 and Gal-14 bind to T cells in a population- and activation-dependent manner. Gal-13 and Gal-14 induce apoptosis of Th and Tc cell populations, regardless of their activation status. Out of the investigated activation markers, Gal-14 decreases the cell surface expression of CD71, Gal-13 increases the expression of CD25, and both galectins increase the expression of CD95 on T cells. Non-activated T cells produce larger amounts of IL-8 in the presence of Gal-13 or Gal-14. In conclusion, these results show that Gal-13 and Gal-14 already provide an immunoprivileged environment at the maternal-fetal interface during early pregnancy, and their reduced expression is related to miscarriages.
Introduction
The mechanisms sustaining maternal immune tolerance to the semi-allogeneic fetus while shielding against microbial infections during pregnancy as well as the changes and interplay of maternal, fetal, and placental immune responses during pregnancy are of major interest in reproductive research (1–41). These immune tolerance mechanisms are complex and dynamic given that implantation involves decidual inflammation; the second trimester of pregnancy is characterized by a predominantly anti-inflammatory milieu in the womb, while at the end of the third trimester, the initiation of parturition requires a transition toward physiologic pro-inflammatory responses (42–44). Recent evolutionary evidence has shown that the pro-inflammatory implantation reaction in humans, as in all eutherian mammals, is derived from an inflammatory attachment reaction in the uterus of the ancestral therian mammals that directly leads to parturition, and that a key innovation in eutherian mammals was the shift from this inflammatory attachment reaction to the non-inflammatory mid-pregnancy period, which allowed an extended period of intimate placentation (45, 46). Although the molecular changes of this evolutionary shift in uterine immune responses are not yet explored in detail, these may include the placental expression of molecules that down-regulate maternal immune responses (47–67). This is substantiated by the fact that the dysregulated expression of immunoregulatory molecules at the maternal-fetal interface and the consequent disturbances in maternal-fetal immune regulation and pro-inflammatory processes are associated with the development of the great obstetrical syndromes, including miscarriage (68–71), preterm labor (72–80), or preeclampsia (81–87).
Regulation of the immune system is mediated by a complex network of cellular and molecular interactions, including glycan recognition by endogenous lectins (61, 88–90). Galectins, a subfamily of lectins specifically bind β-galactoside-containing glycoconjugates, also on immune cell surfaces where they modify immune responses by cross-linking receptors (61, 89, 91–93). Galectins have pleiotropic functions given their binding to a diverse set of cell surface ligands on immune and other cells including trophoblasts (94, 95). In mammals, 19 galectins have been identified, of which 13 are expressed in human tissues (56, 61, 92, 93). Studies of past decades began the exploration of the diverse functions of human galectins, primarily galectins-1, -3, and -9, in innate and adaptive immune responses including the regulation of leukocyte homing, adhesion, apoptosis, pathogen sensing, and immune signaling, also observed in reproductive processes (52, 96–102). Of major interest, several human galectins have an abundant expression at the maternal-fetal interface (31, 52, 53, 56, 58, 97, 103–109), and galectins-13, -14, and -16 are solely expressed by the human placenta (53, 56, 58, 61). These three galectins are expressed from a gene cluster on Chromosome 19 that had emerged in anthropoid primates (53, 56, 61, 110).
We recently started to explore the biological functions of Chromosome 19 galectins in pregnancy (53). Galectin-13 and galectin-14 (Gal-13 and Gal-14), originally described as placental protein 13 (PP13) (111) and placental protein 13-like (PPL13) (112), respectively, are strongly expressed in the syncytiotrophoblast at the lining of the maternal-fetal interface (53, 106, 110, 113, 114). The expression of these galectins is dependent on trophoblast differentiation, and this developmentally regulated process in the trophoblast emerged during primate evolution (110). Of importance, Gal-13 is secreted from the syncytiotrophoblast into the maternal circulation, and low Gal-13 concentration in the maternal circulation in the first trimester was found in women who subsequently developed preterm preeclampsia (115–122), a severe obstetrical syndrome with a strong systemic immune dysregulation (51, 82, 86, 123–128) that already exists in the first trimester (129). Our studies have also shown that the placental expression of Gal-13 and Gal-14 is down-regulated in preterm preeclampsia (81, 110, 113, 129), where the placental pathology and the pro-inflammatory changes are similar to that of miscarriage (130–135).
Since we and our collaborators have shown that Gal-13 and Gal-14 induce the apoptosis of pre-activated T lymphocytes (53) and that Gal-13 increased IL-1α and IL-6 secretion from peripheral blood mononuclear cells (PBMCs) in pregnant women (114), an unanswered question remained: are Gal-13 and Gal-14 critical regulators of immune processes at the early maternal-fetal interface that can be considerably dysregulated in miscarriage? Therefore, we investigated the placental expression of Gal-13 and Gal-14 in miscarriage and also the effects of Gal-13 and Gal-14 on human T lymphocyte functions, which may play a critical role in immune tolerance and rejection. Indeed, we show herein that these placenta-specific galectins moderate adaptive immune responses and are down-regulated in miscarriages, suggesting that their reduced expression is related to the immunopathology of miscarriage.
Materials and Methods
Study Groups, Clinical Definitions, and Sample Collection
Placental tissue samples, collected from Caucasian women, were processed immediately after sample collection as previously described (81, 136), fixed in 10% neutral-buffered formalin, and were then embedded in paraffin (FFPE). First- (n = 40) and third- (n = 2) trimester placentas were collected prospectively at the Maternity Private Department, Semmelweis University (Budapest, Hungary). Pregnancies were dated according to ultrasound scans collected between 5 and 13 weeks of gestation. Patients with a twin gestation were excluded. Women were enrolled in two groups: those who underwent elective termination of pregnancy (control, n = 30) and those who miscarried their pregnancy (cases, n = 10) (Table 1). Miscarriage was defined according to the American College of Obstetricians and Gynecologists Practice Bulletin, as a non-viable, intrauterine pregnancy with a gestational sac containing an embryo or fetus without fetal heart activity within the first 12 6/7 weeks of gestation (137).
Clinical samples and data collection were approved by the Health Science Board of Hungary (ETT-TUKEB 4834-0/2011-1018EKU). Written informed consent was obtained from women prior to sample collection and the experiments conformed to the principles set out in the World Medical Association Declaration of Helsinki. Specimens and data were stored anonymously.
Histopathologic Evaluation of the Placentas
Five-micrometers-thick sections were cut from FFPE tissue blocks and stained with hematoxylin and eosin for histopathological evaluation at the 1st Department of Pathology and Experimental Cancer Research, Semmelweis University. The sections were examined using light microscopy by a perinatal pathologist blinded to the clinical information. Histopathologic changes were defined according to published criteria (136, 138, 139).
Tissue Microarray Construction, and Galectin-13 and Galectin-14 Immunostainings
As previously described (140–143), representative areas were selected for the construction of tissue microarrays (TMAs), which contained 2 mm cores in diameter. To investigate protein expressions, two TMAs were created, using an automated tissue arrayer (TMA Master II, 3DHISTECH Ltd.), to contain one block of each first-trimester (n = 40) placenta as well as a positive control (third-trimester healthy placenta) and a negative control (liver) in triplicate.
Five-micrometers-thick sections were cut from TMAs and placed on silanized slides. After deparaffinization and rehydration, antigen retrieval was performed using citrate buffer (10 mM Sodium citrate, 0.05% Tween 20, pH = 6) for 5 min at 100°C in a pressure cooker. Endogen peroxidase blocking was performed using 10% H2O2 for 20 min. Immunostaining was carried out using the Novolink Polymer Detection System (Novocastra Laboratories), according to the manufacturer's protocol, as detailed in Supplementary Table 1. Slides were blocked for 10 min with Protein Block. To evaluate Gal-13 expression, slides were incubated with anti-galectin-13 mouse monoclonal antibody (clone 215-28-3) in 1% BSA-TBS for 60 min at 37°C. To evaluate Gal-14 expression, slides were incubated with anti-galectin-14 recombinant human antibody in 1% BSA-TBS for 60 min at room temperature. In the case of Gal-14 staining, after three washes with Tris buffer saline with 0.05% Tween 20 (TBST), slides were incubated with anti-His6 mouse monoclonal antibody for 30 min at room temperature. In both circumstances, subsequent steps were the same. Briefly, after three washes with TBST and Post Primary treatment (30 min, at room temperature), Novolink Polymer was used as the secondary antibody for 30 min at room temperature. This was followed by three washes with TBST, and then the sections were developed using 3,3′-diaminobenzidine (DAB, Novolink) in 1:20 dilution. Finally, sections were counterstained with hematoxylin, and these were mounted with DPX Mountant (Sigma-Aldrich) after dehydration.
Evaluation of Immunostainings
Gal-13 or Gal-14 immunostained placental TMAs were digitally scanned by a high-resolution bright field slide scanner (Pannoramic Scan, 3DHISTECH Ltd.), and cytoplasmic staining in the syncytiotrophoblast was evaluated on virtual slides using Pannoramic Viewer 1.15.4 (3DHISTECH Ltd.) by two examiners blinded to the clinical information. All villi were scored semi-quantitatively. The intensity of immunostaining was graded from 0 to 3. The average intensity was determined for each core as the representative data for that core. By averaging immunoscores of the cores, the overall intensity score was assigned to each placenta and then to each patient group.
Expression and Purification of Recombinant Galectin-13 and Galectin-14
Recombinant Gal-13/Gal-14 was expressed as previously described (53) with modifications. Expression plasmids used earlier (53) were modified by the N-terminal insertion of maltose-binding protein (MBP) tag on these galectins. These modified plasmids, containing either full-length Gal-13 or Gal-14 as well as N-terminal maltose-binding protein (MBP)- and C-terminal His6-tags, were transformed into ClearColi BL21 (DE3) (Lucigen). For protein expression, cells were grown in LB-Miller broth to OD600 = 0.6 at 37°C, induced with 0.4 mM isopropyl β-D-1-thiogalactopyranoside (IPTG), and further grown for 4 h at 30°C. The following purification steps were applied: affinity purification on MBPTrap HP column (GE Healthcare Life Sciences); size exclusion chromatography (Superdex 200 Increase SEC column, GE Healthcare Life Sciences) for elimination of aggregates (only for Gal-14); MBP cleavage by Tobacco Etch virus (TEV) protease [expressed and purified according to Kapust et al. (144)]; affinity chromatography on HisTrap HP columns (GE Healthcare Life Sciences); desalting and buffer exchange on Bio-Gel P-6 Desalting Cartridge (Bio-Scale Mini, Bio-Rad). All steps were carried out in the presence of 1 mM dithiothreitol (DTT). Finally, Gal-13 and Gal-14 in PBS, supplemented with 1 mM DTT, were aliquoted and stored at −80°C.
Checking the Purity and Carbohydrate Binding Properties of Recombinant Gal-13 and Gal-14
The purity of the recombinant galectins was verified by heating the samples in Laemmli buffer for 10 min at 70°C, followed by 15% SDS polyacrylamide gel electrophoresis (SDS-PAGE) (Bio-Rad). After gel electrophoresis, recombinant galectins were either subjected to Coomassie blue staining (Supplementary Figure 1A) or transferred to nitrocellulose membranes. Membranes were blocked with 5% non-fat dry milk in TBST for 1 h, and then these were incubated overnight at 4°C with primary antibodies to Gal-13 (clone 27-3-2) or Gal-14 in TBST with 5% BSA. After repeated washing with TBST, blots were incubated for 1 h with HRP-goat anti-mouse IgG antibody (ThermoFisher Scientific) for Gal-13 or with HRP-goat anti-human IgG F(ab')2 antibody (Bio-Rad) for Gal-14 (Supplementary Table 2). After repeated washing with TBST, protein bands were visualized by enhanced chemiluminescence (ECL; Amersham International) (Supplementary Figure 1B).
To determine their functional activity, the binding of purified galectins to asialofetuin (ASF), a naturally occurring multivalent glycoprotein serving as a ligand for several galectins, was assayed by ELISA (145, 146). Briefly, ASF (50 μL of 10 μg/mL bovine ASF in sodium carbonate buffer pH = 9.6) was immobilized in microtiter plates overnight. After blocking residual binding sites with BSA (5% in PBS-Tween, PBST) for 2 h, different amounts of Gal-13 or Gal-14 were incubated for 1 h in PBST with 0.5% BSA. Washing with PBS was done three times between the incubation steps. Bound galectins were detected by incubation with anti-His6-HRP antibody in PBST with 0.5% BSA (Biolegend, 1:1,000) and by the subsequent conversion of 3,3′5,5′-tetramethylbenzidine (TMB; Sigma-Aldrich) with a readout at 450 nm (reference filter: 620 nm). The reaction was stopped by 4N H2SO4 (Supplementary Figure 1C). Additionally, 50 μg/mL recombinant galectins were pre-incubated with gentle rotation on lactose-agarose beads (Sigma-Aldrich) for 1 h at room temperature prior to performing ELISA to also check for lactose inhibition. The inhibition was moderate for Gal-13 and weak for Gal-14, as we found differential binding of Gal-13 and Gal-14 to lactose and other carbohydrates in a previous study (53).
Isolation of Primary Immune Cells
Blood samples were obtained from a donor pool of non-pregnant, healthy, human females (n = 18 in total, n = 4–8 per assay, median age: 29.5) who were in the pre-ovulatory phase. PBMCs were isolated by Ficoll-Hypaque (Sigma-Aldrich) density-gradient centrifugation and washed in RPMI 1640 medium (ThermoFisher Scientific) before experimentations. T cells were isolated from PBMCs with the Dynabeads untouched human T cell kit (ThermoFisher Scientific) according to the manufacturer's protocol. PBMCs or T lymphocytes were kept in RPMI 1640 medium supplemented with 10% FBS and gentamycin or were activated for 48/72 h with the Dynabeads human T-Activator CD3/CD28 (ThermoFisher Scientific), according to the manufacturer's instructions, before treatment with Gal-13 or Gal-14 for 24 h.
Binding of Gal-13 and Gal-14 to Peripheral Blood T Cells
Fresh PBMCs from three donors, or PBMCs activated or not with human T-Activator for 72 h, were used for the Gal-13/Gal-14 binding study. To measure the binding of recombinant Gal-13 or Gal-14 to the surface of T cells, 2 ×105 PBMCs were initially washed in PBS containing 1% BSA. Recombinant Gal-13 or Gal-14 (4 μM), which we conjugated with CF488 fluorophore using the Mix-n-Stain CF488 kit (Sigma-Aldrich) according to the technical bulletin, was added to the cells, and samples were incubated for 45 min on ice. After washing, Fc receptors were blocked with human FcR blocking reagent (Miltenyi Biotec) for 5 min on ice. Anti-CD3-APC, anti-CD4-PerCP, and anti-CD8-APC/Fire750 antibodies (Biolegend) were applied to discriminate between T lymphocyte populations. All antibodies and reagents are listed in Supplementary Table 3. Flow cytofluorimetric measurements were carried out on a CytoFLEX device (Beckman Coulter) by collecting data from 50,000 cells. Data were analyzed using FlowJo v10 software (FlowJo, LLC).
Apoptosis Assay
PBMCs (5 ×105), previously activated or not with human T-Activator for 48 h, were incubated for 24 h on tissue culture plates with 0.25 or 4 μM recombinant Gal-13 or Gal-14 in RPMI 1640 medium supplemented with 10% FBS. The 105 cells were stained with anti-CD3-APC and anti-CD8-FITC antibodies, as described above. Cells were then incubated in 100 μL of annexin-binding buffer containing phycoerythrin-conjugated Annexin V (Annexin V-PE) and 7-amino-actinomycin D (7-AAD) (Annexin-V Apoptosis Detection Kit, ThermoFisher Scientific; Supplementary Table 3) for 15 min at room temperature in the dark. After incubation, 400 μL annexin binding buffer was added, and samples were measured immediately on a FACSCalibur cytofluorimeter using Cell Quest software (BD Biosciences). The Annexin V-PE−/7-AAD− population was regarded as normal, while the Annexin V-PE+/7-AAD− and Annexin V-PE+/7-AAD+ populations were taken as measurements of early and late apoptotic cells, respectively. Data were analyzed using FlowJo v10 software.
Flow Cytometry Measurement of Activation Markers
The PBMCs (5 ×105), previously activated or not with human T-Activator for 72 h, were incubated for 24 h on tissue culture plates with 4 μM recombinant Gal-13 or Gal-14 in RPMI 1640 medium supplemented with 10% FBS. To examine cell surface markers, 2 ×105 PBMCs were initially washed in PBS containing 1% FBS. Fc receptors were blocked with human FcR blocking reagent for 5 min on ice; then, specific antibodies to mid-late and late activation markers CD25 (Interleukin-2 Receptor alpha, IL-2Rα), CD71 (Transferrin Receptor, TfR), CD95 (Fas Cell Surface Death Receptor, Fas), and HLA-DR (Human Leukocyte Antigen, DR isotype; member of MHC-II) were added to the cells. Anti-CD3-APC and anti-CD8-FITC antibodies were added simultaneously and samples were incubated for 20 min on ice. All antibodies are listed in Supplementary Table 3. After washing, cells were measured in a CytoFLEX flow cytofluorimeter. A total of 20,000 cells were collected and data were analyzed using FlowJo v10 software.
Measurement of Cytokine Production by Bead Array
The T lymphocytes were isolated as described above. The 5 ×105 cells, previously activated or not with human T-Activator for 72 h, were incubated for 24 h on tissue culture plates with 4 μM recombinant Gal-13 or Gal-14 in RPMI 1640 medium supplemented with 10% FBS. Supernatants were collected in all cases, centrifuged at 400 g for 10 min, aliquoted and stored at −80°C until use. LEGENDplex bead-based immunoassays (Biolegend) were applied to measure the concentration of IL-8, IL-10, IFNγ, IL-1β, and IL-6 cytokines in cell culture supernatants of T cells, according to the manufacturer's instruction. Beads were measured in a FACSCalibur flow cytofluorimeter and data were analyzed using FlowJo v10 software.
Statistical Analysis
Statistical analysis was performed using GraphPad Prism 5.0 (GraphPad Software). An unpaired t-test with or without Welch's correction was used to analyze demographic data. An unpaired t-test was also used to analyze Gal-13 and Gal-14 immunostainings when comparing first-trimester control and miscarriage groups. The Fisher's exact test was performed to test the distribution of Gal-13 or Gal-14 immunoscores between the control and miscarriage groups. Repeated ANOVA tests with Tukey's post-hoc test were used for the analysis of galectin binding and CD4:CD8 ratio upon different treatments. One sample t-test was used to compare apoptosis of the Gal-13- and Gal-14-treated groups to the PBS-DTT-treated group, and to analyze the binding of Gal-13 and Gal-14 to ASF with or without lactose pre-treatment. Repeated ANOVA tests with Dunnett's post-hoc test were used to compare the non-treated group with Gal-13/Gal-14-treated groups in activation marker expression and cytokine production studies. Results were considered statistically significant at *p < 0.05, **p < 0.01, and ***p < 0.001.
Results
Gal-13 and Gal-14 Are Expressed in First-Trimester Placentas and Their Expression Is Decreased in Miscarriage
Immunostainings of TMAs revealed that Gal-13 (Figures 1A,B) and Gal-14 (Figures 1E,F) are predominantly expressed in the cytoplasm of the syncytiotrophoblast of chorionic villi in the first trimester, and there were no stainings in the cytotrophoblasts and villous stroma, similar to later stages of pregnancy (53, 81, 106, 110, 113). Moreover, chorionic villi exhibited more intense syncytiotrophoblast cytoplasmic staining in the first trimester than in the third trimester (Supplementary Figure 2). The specificity of the galectin antibodies was confirmed by previous studies and by the lack of Gal-13 and Gal-14 immunostaining of human livers on our TMAs.
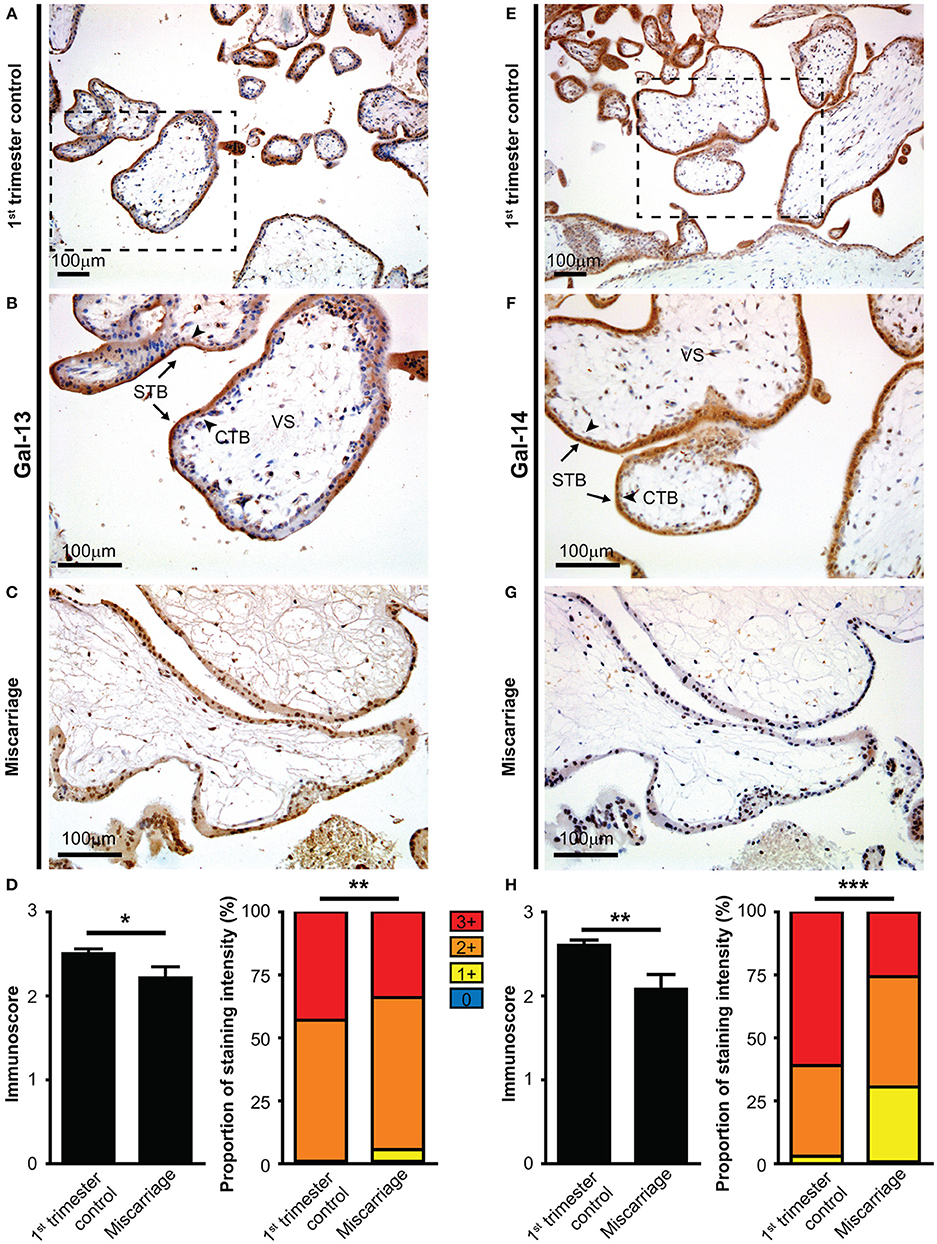
Figure 1. The syncytiotrophoblast expresses galectin-13 and galectin-14 in the first-trimester placenta, which is decreased in miscarriage. Five-micrometers-thick first-trimester placental sections from normal pregnancy (A,B,E,F) or from miscarriage (C,G) were stained for Gal-13 (A–C) or Gal-14 (E–G) by specific monoclonal antibodies. Chorionic villi exhibited intense syncytiotrophoblast cytoplasmic staining (arrows, STB), while the villus stroma (VS) and cytotrophoblasts were negative (arrowheads, CTB). Representative images, hematoxylin counterstain, 100x (A,E) and 200x (B,C,F,G) magnifications. Gal-13 (D) and Gal-14 (H) immunoscores (mean ± SEM) and proportion of staining intensities in control placentas (n = 30) and placentas with miscarriage (n = 10) are displayed on left and right graphs, respectively (Gal-13: ntotalvillus = 775 and ntotalvillus = 106, respectively; Gal-14: ntotalvillus = 797 and ntotalvillus = 121, respectively). Unpaired t-test was used for the comparison of the mean immunoscores of the two groups. Fisher's exact test was performed to test the frequency difference of Gal-13 or Gal-14 immunostaining between control and miscarriage groups (*p < 0.05, **p < 0.01, ***p < 0.001).
Next, we examined whether the expression of Gal-13 and Gal-14 is dysregulated in first-trimester placentas obtained from women who miscarried, as a potential sign of fetal rejection. There was no significant change in Gal-13 or Gal-14 immunoscores with gestational age in control placentas (R2 = 0.0078 for Gal-14; R2 = 10−5 for Gal-13). However, the average immunoscore of syncytiotrophoblast decreased by 11.5% for Gal-13 (p = 0.027, Figures 1A–D) and by 20% (p = 0.001) for Gal-14 (Figures 1E–H) in miscarriages compared to gestational age-matched controls. Also, there was a significant difference in the distribution of Gal-13 and Gal-14 immunoscores (p = 0.002 and p < 0.001, respectively) between the disease and control groups (Figures 1D,H).
Gal-13 and Gal-14 Bind to Peripheral Blood T Cells
As Gal-13 and Gal-14 are released from the placenta into the maternal circulation, where they may regulate maternal T lymphocytes (53), we further characterized their effects on T cell populations. First, we examined the binding of fluorescent Gal-13 or Gal-14 to primary T cells. We found that Gal-13 and Gal-14 bound to freshly isolated T lymphocytes, either to helper (Th: 3.4 ± 0.9% and 5.1 ± 1%, respectively) or cytotoxic T (Tc: 3.9 ± 0.8% and 7.7 ± 2.2%, respectively) cells (Figures 2A,B, Supplementary Figure 3). Upon activation, binding of Gal-13 to Th and Tc cells was increased by 21% (p < 0.001) and 42% (p < 0.001), respectively (Figure 2B, Supplementary Figure 3B). Binding of Gal-14 was increased by 20% (p < 0.01) and by 30% (p < 0.001) to activated Th and Tc cells, respectively, compared to non-activated ones (Figure 2B, Supplementary Figure 3B). Of note, both galectins tended to bind more to Tc over Th lymphocytes, a difference that reached statistical significance in the case of activated cells (p < 0.001 for Gal-13 and p < 0.05 for Gal-14).
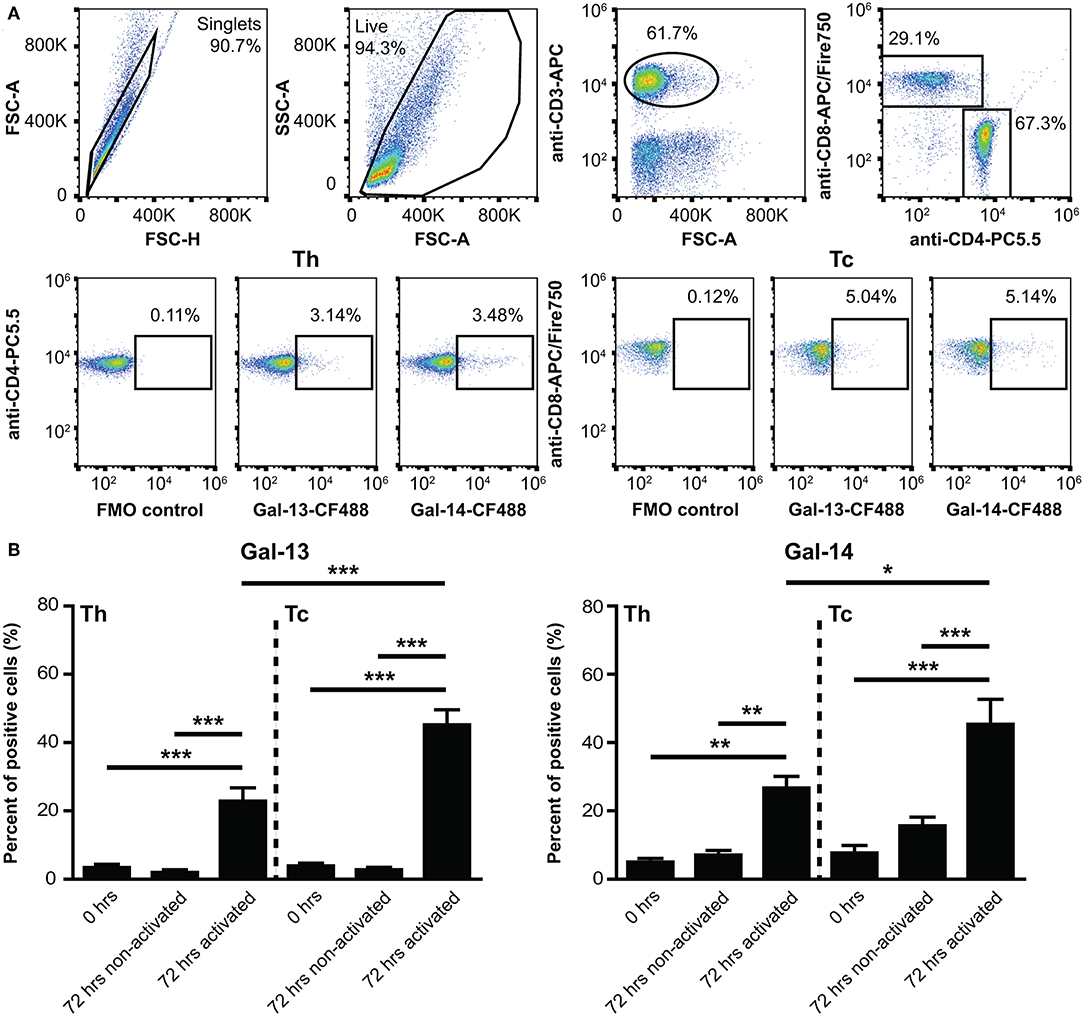
Figure 2. Binding of galectin-13 and galectin-14 to T lymphocytes is population- and activation-dependent. (A) Detection of Gal-13 or Gal-14 binding to human T lymphocytes was achieved by flow cytometry with the following cells: freshly isolated PBMCs (0 h) or PBMCs, kept in culture for 72 h in the presence (72 h activated) or absence (72 h non-activated) of anti-CD3/CD28 microbeads. Cells were incubated with 4 μM Gal-13-CF488 or Gal-14-CF488 for 45 min on ice. PBMCs were also stained for CD3 (anti-CD3-APC), CD4 (anti-CD4-PC5.5), and CD8 (anti-CD8-APC/Fire750), in order to distinguish between helper (Th) and cytotoxic (Tc) T lymphocytes. The gating strategy is shown in (A). (B) Graphs show the percentage of cells, to which Gal-13 (left) or Gal-14 (right) were bound, as mean ± SEM. Repeated ANOVA with Tukey's post-hoc test was used for the comparison of groups (*p < 0.05, **p < 0.01, ***p < 0.001). Four non-pregnant female donors were included in each group. FMO, Fluorescence minus one; PBMCs, peripheral blood mononuclear cells.
Gal-13 and Gal-14 Increase Apoptosis of Non-activated and Activated T Cells
Since certain galectins can induce the apoptosis of T cells depending on T cell subsets and their activation status (147–149), we investigated the effects of Gal-13 and Gal-14 on various T cell populations, either in an activated or a non-activated state. Flow cytometry results, overall, show that 4 μM, but not 0.25 μM, of Gal-13 or Gal-14 increased apoptosis of T lymphocytes either pre-activated or not (Figures 3A,B, Supplementary Figure 4A). Gal-13 increased apoptosis of both non-activated and pre-activated T cells by 5.3% (p = 0.010) and 9%, (p = 0.011), respectively. Gal-14 increased apoptosis of pre-activated T cells by 8.9% (p = 0.040) compared to PBS-DTT treated cells. We further analyzed Th and Tc lymphocytes separately, based on CD3 and CD8 expression. Tc cell apoptosis was increased for both galectins regardless of the activation state (Gal-13, non-activated: 3.9%, p = 0.002; Gal-13, pre-activated: 8.2%, p = 0.022; Gal-14, non-activated: 11%, p = 0.001; Gal-14, pre-activated: 11.2%, p = 0.032), while Gal-13 increased apoptosis rate (8.3%, p = 0.031) of non-activated Th cells (Figures 3A,B). The proportion of early apoptotic (Annexin V+ 7-AAD−) T lymphocytes, Th cells, and Tc cells as well did not change upon Gal-13 or Gal-14 treatment (Supplementary Figure 4B).
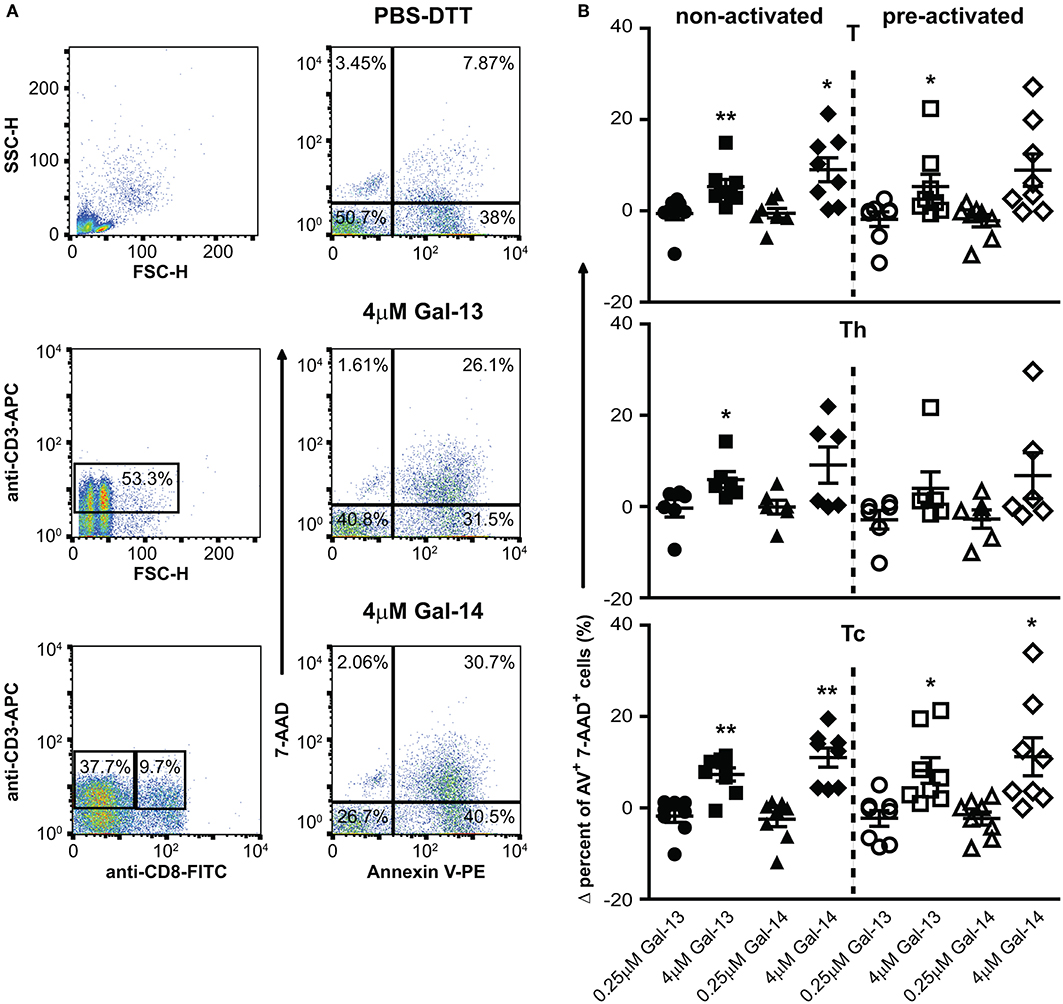
Figure 3. Galectin-13 and galectin-14 increase apoptosis of T lymphocytes. (A) PBMCs were kept in culture for 48 h in the presence (activated) or absence (non-activated) of anti-CD3/CD28 microbeads, then treated with Gal-13 or Gal-14 for 24 h. To detect apoptosis of T lymphocytes by flow cytometry, cells were stained with Annexin V-PE and 7-AAD and were also stained for CD3 (anti-CD3-APC), and CD8 (anti-CD8-FITC), in order to distinguish between helper (Th) and cytotoxic (Tc) T cells, respectively. (A) The gating strategy (left: CD3+ T gate, CD3+CD8+ Tc gate, CD3+CD8− Th gate) and representative Annexin V and 7-AAD dot plots of vehicle- (PBS-DTT), Gal-13- or Gal-14-treated T cells (CD3+) are displayed. (B) Graphs show the Δ percentage of double positive (Annexin V-PE+ 7-AAD+) cells as mean ± SEM. One sample t-test was used for the comparison of galectin-treated groups with the PBS-DTT-treated group of non-activated or pre-activated cells (*p < 0.05, **p < 0.01). Six-eight non-pregnant female donors were included in each group. PBMCs, Peripheral blood mononuclear cells.
Gal-13 and Gal-14 Treatment Alters Cell Surface Expression of T Cell Activation Markers
Next, we investigated the impact of Gal-13 and Gal-14 on the expression of well-known activation markers—CD25 (IL-2Rα), CD71 (TfR), CD95 (Fas), and HLA-DR (MHC-II)—of T lymphocytes. Interestingly, Gal-13 treatment increased both the percentage of CD95 positive cells and the cell surface expression of CD95 on Th (% control: 11.3 ± 5.2%, Gal-13: 19.6 ± 7%, p < 0.05; RMFI control: 1.7 ± 0.2, Gal-13: 2.2 ± 0.2, p < 0.01) and Tc (% control: 6.1 ± 1.7%, Gal-13: 13.7 ± 3.7%, p < 0.05; RMFI control: 1.4 ± 0.1, Gal-13: 1.7 ± 0.2, p < 0.01) lymphocytes. Gal-14 treatment increased the percentage of CD95 positive cells (18.7 ± 8.5%, p < 0.05) and the cell surface expression of CD95 on Th (2.1 ± 0.3, p < 0.05) but not on Tc lymphocytes (Figure 4A, Supplementary Figure 5B).
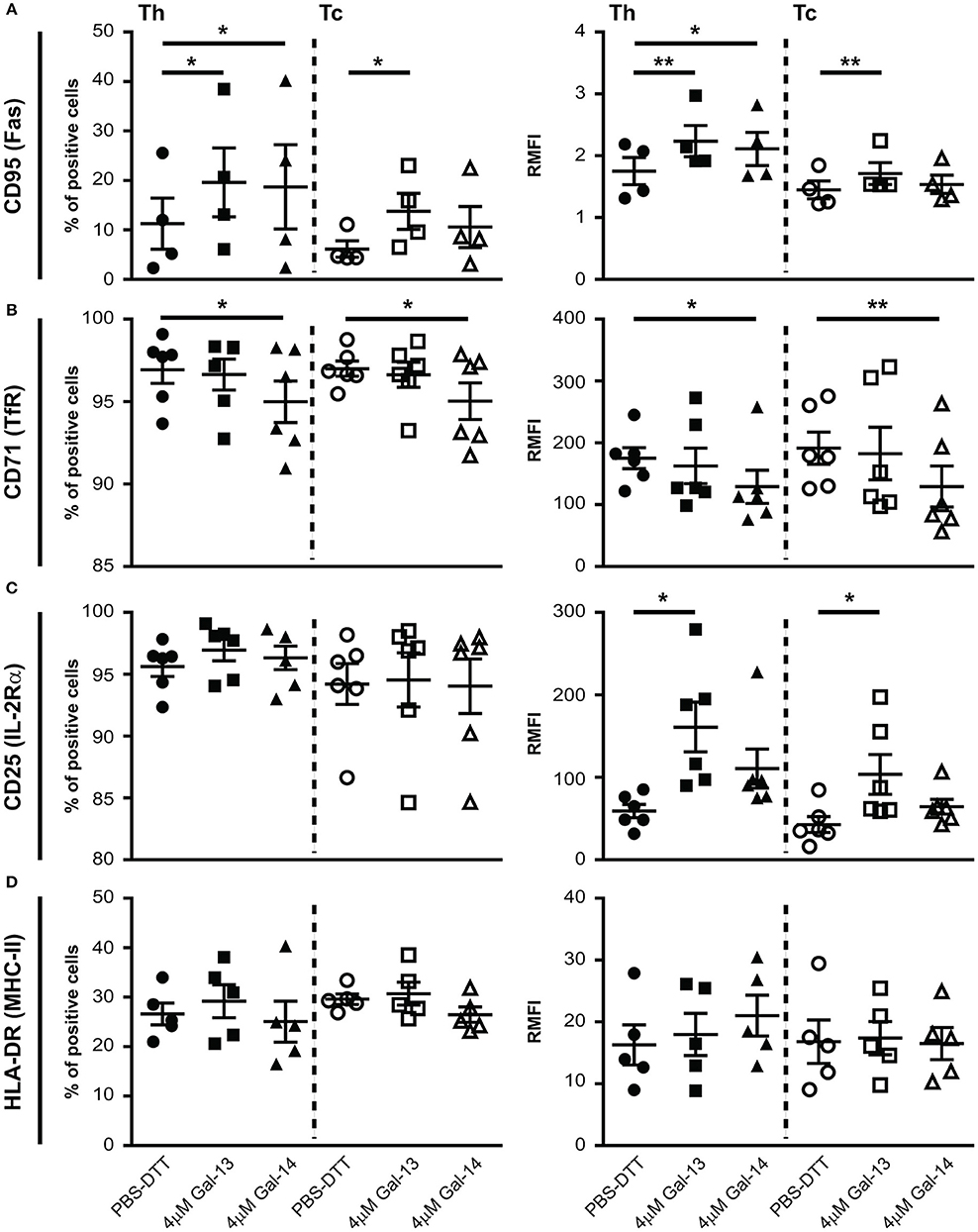
Figure 4. Galectin-13 and galectin-14 affect cell surface expression of activation markers on T lymphocytes. PBMCs were kept in culture for 72 h in the presence of anti-CD3/CD28 microbeads, then treated with Gal-13 or Gal-14 for 24 h. To detect cell surface expression of CD95 (A), CD71 (B), CD25 (C), HLA-DR (D) activation markers on T lymphocytes by flow cytometry, cells were stained with anti-CD25-PE and anti-CD71-PerCP/5.5, or anti-CD95-PE and anti-HLA-DR-PerCP/5.5. Cells were also stained for CD3 (anti-CD3-APC), and CD8 (anti-CD8-FITC) in order to distinguish between helper (Th) and cytotoxic (Tc) T cells. Left graphs show the percentage of positive cells and right graphs show relative median fluorescence intensity (RMFI) values (mean ± SEM). RMFI was calculated by dividing specific median fluorescence intensity with the median fluorescence intensity of the isotype control. Repeated ANOVA with Dunnett's post-hoc test was used for comparison of the non-treated group with Gal-13/Gal-14-treated groups (*p < 0.05, **p < 0.01). Four-six non-pregnant female donors were included in each group. HLA-DR, Human leukocyte antigen DR isotype; PBMCs, peripheral blood mononuclear cells.
The percentage of cells expressing CD71 and the cell surface expression of CD71 decreased upon Gal-14, but not Gal-13 treatment on both Th (% control: 96.9 ± 0.8%, Gal-14: 91 ± 1.3%, p < 0.05; RMFI control: 175.1 ± 17, Gal-14: 129 ± 6.1, p < 0.05) and Tc (% control: 97 ± 0.5%, Gal-14: 95 ± 1.1%, p < 0.05; RMFI control: 191.4 ± 26, Gal-14: 129.3 ± 33.2, p < 0.01) lymphocytes (Figure 4B, Supplementary Figure 5B).
Neither the percentage of CD25 nor of HLA-DR positive cells changed upon galectin treatment (Figures 4C,D, Supplementary Figure 5B). However, cell surface expression of CD25 increased upon Gal-13 treatment (Th RMFI control: 59.1 ± 8.1, Gal-13: 161 ± 30, p < 0.05; Tc RMFI control: 42.7 ± 9.6, Gal-13: 103.5 ± 24, p < 0.05), and tended to increase upon Gal-14 (RMFI Th: 110.6 ± 23.7; Tc: 64.4 ± 9.1) treatment in both T cell populations (Figure 4C). Of note, treatment of non-activated cells with Gal-13 or Gal-14 did not change the expression of these activation markers (Supplementary Figure 5A).
Gal-13 and Gal-14 Induce Il-8 Secretion of T Cells
Next, we sought to explore whether T lymphocytes contribute to the altered cytokine production, previously measured in PBMCs (114). IL-1β and IL-6 concentrations were below the detection limit in cell culture supernatants (data not shown). Surprisingly, IL-8 production was increased upon treatment with either Gal-13 (260.7 ± 78 pg/mL, p < 0.01) or Gal-14 (237.4 ± 73.5 pg/mL, p < 0.05) compared to the control (10.6 ± 9.2 pg/mL), when T cells were not activated. In the case of activation through CD3 and CD28, galectins could not further increase IL-8 production (Figure 5). Neither IL-10 nor IFNγ production changed upon galectin treatment (Figure 5).
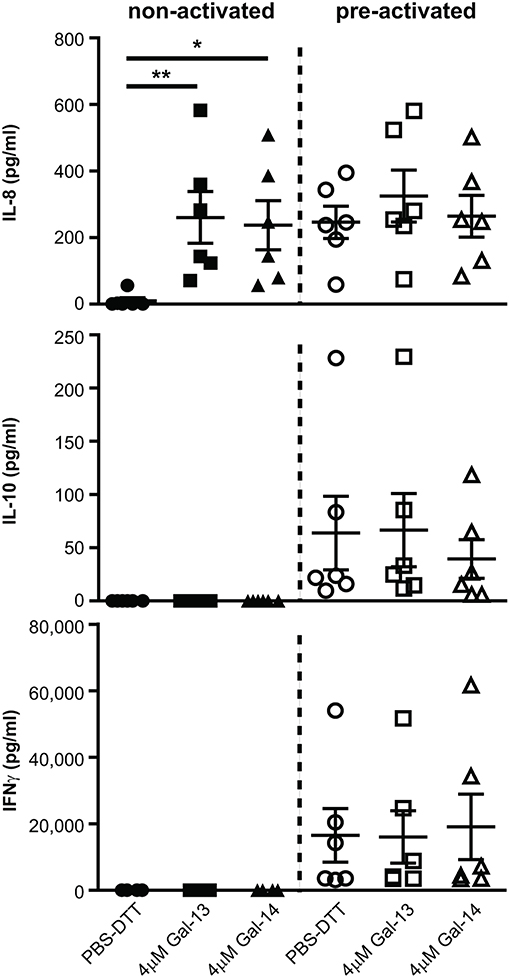
Figure 5. Galectin-13 and galectin-14 induce IL-8 production in resting T lymphocytes. T lymphocytes after negative selection were kept in culture for 72 h in the presence (pre-activated) or absence (non-activated) of anti-CD3/CD28 microbeads, then treated with 4 μM Gal-13 or Gal-14 for 24 h. Supernatants were collected and IL-8, IL-10, and IFNγ cytokines were measured by flow cytometry using LEGENDplex assays. Graphs show calculated cytokine concentrations as mean ± SEM. Repeated ANOVA with Dunnett's post-hoc test was used for the comparison of the non-treated group with Gal-13/Gal-14-treated groups (*p < 0.05, **p < 0.01). Six non-pregnant female donors were included in each group.
Discussion
Principal Findings of the Study
(1) Gal-13 and Gal-14 are mainly expressed by the syncytiotrophoblast at the maternal-fetal interface in the first trimester, stronger than in the third trimester of pregnancy; (2) the syncytiotrophoblastic expression of both Gal-13 and Gal-14 is down-regulated in miscarriages compared to first trimester control placentas; (3) recombinant Gal-13 and Gal-14 differentially bind to peripheral blood T cell populations, predominantly to Tc over Th cells; (4) Gal-13 and Gal-14 induce the apoptosis of both T cell populations regardless of their activation status; (5) Gal-14 decreases the cell surface expression of CD71, Gal-13 and Gal-14 increase the cell surface expression of CD95 and Gal-13 increases the cell surface expression of CD25 on T cells; and (6) non-activated T cells produce larger amounts of IL-8 in the presence of Gal-13 or Gal-14.
Placental Galectin-13 and Galectin-14 Expression Is Decreased in Miscarriage
This is the first study to characterize the simultaneous expression of Gal-13 and Gal-14 in first-trimester placentas in healthy and complicated pregnancies. We found that these placenta-specific galectins are mainly expressed by the syncytiotrophoblast at the lining of the maternal-fetal interface, similar to these galectins that are expressed in the placenta in the third trimester (53, 106, 110, 113). The expression of these galectins in the syncytiotrophoblast is developmentally regulated during trophoblast differentiation by transcription factors binding to non-coding elements upfront of these galectin genes on Chromosome 19, a process that emerged in anthropoid primates (110). This is particularly interesting from an immunological point of view given that these anthropoids had a long gestation, which necessitated additional immune tolerance mechanisms at their maternal-fetal interface to prevent fetal rejection (53). In this latter evolutionary and immune functional study, we proposed that the emergence of these galectins in anthropoid primates provided additional immune tolerance toward the fetus. Previous studies on placentas delivered by women with preeclampsia, a severe obstetrical syndrome with an immune rejection component, revealed the down-regulation of Gal-13 and Gal-14 placental expression, suggesting that this phenomenon may be linked to altered immune tolerance (81, 110, 113, 129).
Herein, we report for the first time that the placental expression of Gal-13 and Gal-14 is also decreased in first-trimester miscarriages. As most of these cases have a normal karyotype, our finding suggests that altered maternal-fetal immune tolerance is not closely associated with chromosomal abnormalities but can be a separate underlying mechanism for miscarriages. Indeed, recent publications presented that miscarriages are associated with immune etiologies (150), where the development of the fetus and placenta is affected by either auto- or alloimmune rejection-type activity (151). Of interest, the expression of other galectins, although in modest extent, is also decreased at the maternal-fetal interface in spontaneous and recurrent miscarriages, including Gal-1, Gal-2, Gal-7, Gal-9, and Gal-10 (152–154). Furthermore, in good accordance, serum concentrations of Gal-1 and Gal-9 were also found to be decreased in miscarriage (99, 154, 155). An elegant study revealed that Gal-1 has pivotal functions supporting maternal-fetal immune tolerance and its decreased expression leads to fetal loss in a mouse model. Gal-1 prevents fetal loss and restores tolerance through multiple mechanisms, including the induction of tolerogenic dendritic cells, which, in turn, promotes the expansion of IL-10-secreting regulatory T cells in vivo (107). On the other hand, Gal-9 was found to exert its functions in non-pregnant and pregnant states on NK cells, T cells, and B cells (101, 102, 147, 156–159). In addition, Gal-9 promotes trophoblast invasion in a Tim-3 dependent manner (154). Consistently, a higher proportion of decidual T cells that express activation markers (CD25, and CD69) was found in spontaneous abortion than in elective termination of pregnancy, and decidual lymphocytes from spontaneous abortion increased the apoptosis of trophoblast cells (160). Since Gal-13 and Gal-14 are only expressed in anthropoid primates, it is not possible to investigate the role of these proteins in knock-out mammalian models in vivo. Nevertheless, we can conclude that several galectins, including Gal-13 and Gal-14, potentially act in concert and play a role in maintaining pregnancy and that their lower expression at the maternal-fetal interface in early pregnancy may lead to an immune imbalance that interferes with implantation, trophoblast invasion, and placentation, leading to fetal rejection and miscarriages.
Galectin-13 and Galectin-14 Promote Apoptosis of T Cells
The majority of the galectin family regulates adaptive immune responses through the induction of T cell apoptosis, which then leads to a shift in the innate/adaptive, Th1/Th2, and Th17/Treg immune balances (161–163). Similarly, we reported that exogenously added Gal-13 and Gal-14 are able to induce the apoptosis of activated T cells to a similar extent as Gal-1 (53). In accord with these findings, the interesting study from Kliman et al. (114) showed that Gal-13 is secreted from the syncytiotrophoblast and forms perivenous aggregates in the decidual extracellular matrix in the first trimester. These Gal-13 aggregates, found around decidual veins, were associated with T cell-, neutrophil-, and macrophage-containing “decidual zones of necrosis” (ZONEs), in which apoptotic T cells were also found. Based on these findings in normal pregnancies, and also based on cases in which fewer ZONEs and apoptotic T cells were found in association with very low serum Gal-13 levels, the authors hypothesized that Gal-13 is a key placental protein that downregulates maternal immune responses in the first-trimester decidua to avoid rejection of invasive trophoblasts at the maternal-fetal interface, and that low Gal-13 expression leads to heightened immune responses and impaired trophoblast invasion. It is possible that in cases where this mechanism is very defective (e.g., due to the concerted downregulation of Gal-13, Gal-14, Gal-1, and other immunoregulatory molecules in the placenta or decidua), pregnancies will be miscarried.
To better elucidate the role of Gal-13/Gal-14 in the regulation of T cells, we further characterized the pro-apoptotic effect of these galectins on T lymphocytes. We found that both Gal-13 and Gal-14 increased the rate of late-apoptotic T lymphocytes with ~5–10%, which was not affected by the activation status of the cells, while other galectins promote the apoptosis of only activated leukocytes (164, 165). Our result suggests that Gal-13 and Gal-14 have a basic pro-apoptotic activity on T cells. Of note, Tc cells bound more galectins than Th cells and Tc cells were more susceptible to Gal-13/Gal-14 induced apoptosis than Th cells, which may be related to the differential glycosylation pattern on these two T cell populations. This phenomenon has not been deeply explored in other galectins, which, however, were studied for their effects on different Th subsets. For example, Gal-1 selectively induced the apoptosis of pro-inflammatory Th1 and Th17 cell subsets, but not of naïve, Th2, or Treg cells (166). Moreover, Gal-9 induced the apoptosis of Th1 cells (147) in a Tim-3 dependent manner. Our results warrant further characterization of the pro-apoptotic effects of Gal-13 and Gal-14 on different Th subsets and determination of glycophenotype on T cell populations.
Apoptotic cell death in activated T cells is mediated by signaling through the activation marker CD95 (Fas), following binding to its ligand CD95L/FasL (167). In addition, another T cell activation marker CD25 (Il-2Rα), important for T cell proliferation, is also involved in this process by increasing the expression of CD95 (168). Herein, we found that the cell surface expression of CD95 and CD25 are increased upon Gal-14 and/or Gal-13 treatment. This is important since activated T cells are more prone to apoptosis (169); thus, Gal-13 and Gal-14 may increase the sensitivity of activated T cells to die by the activation-induced cell death. This is concordant with an earlier study in which Gal-1 increased the percentage of Th1 cells expressing CD95, although the Gal-1-mediated apoptosis of these cells was independent of CD95 (149). Interestingly, we found decreased expression of another activation marker (CD71) on T cells treated with Gal-14. This is seemingly contradictory, however, CD71 transiently associates with the TCR in response to TCR engagement (170) and is an essential factor for proliferating T cells (171, 172). Thus, galectins may inhibit T cell proliferation, which still needs to be tested in later studies.
Galectin-13 and Galectin-14 Regulate Cytokine Production of T Cells
Several galectins have been shown to alter cytokine production of immune cells. For example, Gal-1 induces IL-10 production in Treg cells (107, 173) and Gal-9 promotes IL-2 and IFNγ production in T cells (164). Herein, we found in the applied experimental settings that Gal-13 and Gal-14 did not alter most pro-inflammatory (IFNγ, IL-1β, and IL-6) or anti-inflammatory (IL-10) cytokine production of T cells; however, both of these galectins induced IL-8 production in non-activated T cells. This is particularly interesting since IL-8 exerts a pro-angiogenic effect on endothelial cells by decreasing the apoptosis of endothelial cells and increasing their proliferation and capillary formation (174). In addition, a novel neutrophil population was identified by recent studies in second-trimester human deciduas, which promoted in vitro angiogenesis in an IL-8-dependent manner (175, 176). Furthermore, decidual NK cell subsets release significant amounts of pro-angiogenic factors, such as VEGF and IL-8, necessary for spiral artery formation during decidualization (177–179). Of note, the pro-angiogenic effects of other galectins during gestation have been discovered, as reviewed recently (180). Therefore, it is tempting to speculate that Gal-13 and Gal-14 may induce angiogenesis at the maternal-fetal interface through increasing IL-8 production of T cells. This finding is related to the in vivo vasodilator effect of Gal-13 (181–184). In this context, reduced Gal-13 and Gal-14 expression may play a role in the disturbed vascular changes in preterm preeclampsia (58, 185–194). All our data discussed above support the idea that Gal-13 and Gal-14 also have immunoregulatory and vascular effects, as found for galectin-1 or galectin-3 (195, 196). Since the immunomodulatory effects of Gal-13 and Gal-14 could be observed on a broad scale, changing the habit of adaptive immune cells may affect innate immune cells, as well. More experiments are warranted in this direction to comprehensively elucidate the effects of these galectins at the maternal-fetal interface.
Strengths and Limitations of the Study
The strengths of the study are as follows: (1) strict clinical definitions and homogenous patient groups; (2) standardized, quick placental sample collection during pregnancy terminations; (3) standardized histopathological examination of the placentas based on international criteria; (4) protein expression profiling on placentas with tissue microarray and immunostaining followed by semiquantitative immunoscorings and statistical analysis; (5) expression and purification of large amounts of recombinant galectins with standardized methods; and (6) an array of functional experiments with primary cells and recombinant proteins.
Limitations of the study are as follows: (1) the relatively modest number of cases in each patient group due to the strict clinical and histopathological inclusion criteria used for patient enrollment. On the other hand, this was one of the most important strengths of our study; (2) for in vitro experiments, only non-pregnant donors were included in the study given the conditions in our patient recruitment. However, this was also a value of our study, since we used a “naïve” population of immune cells to test the effects of Gal-13 and Gal-14, while experiments with PBMCs isolated from the peripheral circulation or decidua of pregnant women pre-exposed to placental Gal-13/Gal-14 might not have revealed the true effects of these molecules. Nevertheless, our results warrant further characterization of the effects of Gal-13 and Gal-14 on peripheral blood and decidual leukocytes isolated from pregnant women, as pregnancy hormones, especially estrogen and progesterone, may impact glycosylation pattern and galectin-biding capacity of these cells; (3) Gal-13 and Gal-14 concentrations applied in our in vitro experiments were supraphysiologic, similar to experimental settings in previous studies on the functional effects of galectins (7, 165). The use of higher galectin concentrations is due to the fact that blood concentrations of galectins do not reflect their effective local/cell surface concentrations. In fact, these studies usually applied recombinant galectins between 10 and 100 μg/mL, representing a reasonable range of the local galectin concentration expected in the tissues (197). Another technical reason to use higher galectin concentrations is to prevent their subunit dissociation in the solvent that contains DTT (198); and (4) the evaluation of blood concentrations of Gal-13/Gal-14, which may change in parallel with their placental dysregulation in miscarriages, as also seen in the case of Gal-1 (99, 153, 155, 184), was beyond the scope of this study, but our results warrant further investigation.
Concluding Remarks
The causes and consequences of the down-regulation of placental Gal-13 and Gal-14 expression in miscarriages still have to be uncovered by later functional studies. This work suggests that Gal-13 and Gal-14 down-regulate adaptive immune responses at the maternal-fetal interface through T cell apoptosis, and that their impaired expression leads to fetal rejection in miscarriages. Another process in which these galectins may function is angiogenesis, which is altered in both miscarriage and preeclampsia, in which Gal-13 and Gal-14 expression is decreased. Since galectins have pleiotropic functions on various immune and non-immune cells given their promiscuous binding to various cell surface receptors via glycan binding, we envision that both actions may be functional in human pregnancy. In conclusion, our results suggest that Gal-13 and Gal-14 provide an immunoprivileged environment at the maternal-fetal interface, already in early pregnancy, either through down-regulating maternal immune responses or via the support of placental development, and their reduced expression is related to the immune pathology of miscarriages.
Data Availability
All datasets generated for this study are included in the manuscript and/or the Supplementary Files.
Ethics Statement
Clinical sample and data collection were approved by the Health Science Board of Hungary (ETT-TUKEB 4834-0/2011-1018EKU). Written informed consent was obtained from women prior to sample collection and the experiments conformed to the principles set out in the World Medical Association Declaration of Helsinki. Specimens and data were stored anonymously.
Author Contributions
AB, IH, SH, JM, SR, EP, and NT conceptualized study and designed research. AB, DC, KJ, AK, EP, KP, NS, and ET performed research. SH, PH, HM, EP, ZP, RR, NT, and PZ contributed new reagents, analytic tools, and clinical specimens. AB, DC, OE, JM, EP, SR, AT, and NT analyzed and interpreted data. All authors contributed to the writing of the paper.
Funding
This research was supported by the Perinatology Research Branch, Division of Obstetrics and Maternal-Fetal Medicine, Division of Intramural Research, Eunice Kennedy Shriver National Institute of Child Health and Human Development, National Institutes of Health, US Department of Health and Human Services (NICHD/NIH/DHHS); Federal funds from NICHD/NIH/DHHS under Contract No. HHSN275201300006C; grants from the Hungarian National Science Fund (OTKA-PD 104398 to AB and OTKA-K 124862 to NT), from the Hungarian Academy of Sciences (Momentum LP2014-7/2014 to NT), and from the Hungarian National Research, Development and Innovation Fund (ÚNKP-18-3-IV-SE-14 to AK and FIEK_16-1-2016-0005 to NT).
Conflict of Interest Statement
HM is the CEO and Chairman of TeleMarpe Ltd. and is a consultant of Hy Laboratories.
The remaining authors declare that the research was conducted in the absence of any commercial or financial relationships that could be construed as a potential conflict of interest.
Acknowledgments
We thank for Judit Baunoch and Dr. Anna Sarai (Hungarian Academy of Sciences), Dorottya Csernus-Horvath, Nora Fekete, Katalin Karaszi, Prof. Ilona Kovalszky, Dr. Tibor Krenacs, Eva Matraine Balogh, and Zsofia Zsibai (Semmelweis University), Jolan Csapai, Laszlo Daru, Hajnalka Nyiro, and Erzsebet Szilagyi (Maternity Private Clinic) for their assistance and Maureen McGerty (Wayne State University) for her critical reading of the manuscript.
Supplementary Material
The Supplementary Material for this article can be found online at: https://www.frontiersin.org/articles/10.3389/fimmu.2019.01240/full#supplementary-material
References
1. Szekeres-Bartho J, Wegmann TG. A progesterone-dependent immunomodulatory protein alters the Th1/Th2 balance. J Reprod Immunol. (1996) 31:81–95. doi: 10.1016/0165-0378(96)00964-3
2. Moffett-King A. Natural killer cells and pregnancy. Nat Rev Immunol. (2002) 2:656–63. doi: 10.1038/nri886
3. Abrahams VM, Kim YM, Straszewski SL, Romero R, Mor G. Macrophages and apoptotic cell clearance during pregnancy. Am J Reprod Immunol. (2004) 51:275–82. doi: 10.1111/j.1600-0897.2004.00156.x
4. Mor G, Romero R, Aldo PB, Abrahams VM. Is the trophoblast an immune regulator? The role of Toll-like receptors during pregnancy. Crit Rev Immunol. (2005) 25:375–88. doi: 10.1615/CritRevImmunol.v25.i5.30
5. Richani K, Soto E, Romero R, Espinoza J, Chaiworapongsa T, Nien JK, et al. Normal pregnancy is characterized by systemic activation of the complement system. J Matern Fetal Neonatal Med. (2005) 17:239–45. doi: 10.1080/14767050500072722
6. Aluvihare VR, Betz AG. The role of regulatory T cells in alloantigen tolerance. Immunol Rev. (2006) 212:330–43. doi: 10.1111/j.0105-2896.2006.00408.x
7. Blois SM, Kammerer U, Alba Soto C, Tometten MC, Shaikly V, Barrientos G, et al. Dendritic cells: key to fetal tolerance? Biol Reprod. (2007) 77:590–8. doi: 10.1095/biolreprod.107.060632
8. Holzgreve W, Hahn S, Zhong XY, Lapaire O, Hosli I, Tercanli S, et al. Genetic communication between fetus and mother: short- and long-term consequences. Am J Obstet Gynecol. (2007) 196:372–81. doi: 10.1016/j.ajog.2006.12.013
9. Saito S, Shiozaki A, Sasaki Y, Nakashima A, Shima T, Ito M. Regulatory T cells and regulatory natural killer (NK) cells play important roles in feto-maternal tolerance. Semin Immunopathol. (2007) 29:115–22. doi: 10.1007/s00281-007-0067-2
10. Zenclussen AC, Schumacher A, Zenclussen ML, Wafula P, Volk HD. Immunology of pregnancy: cellular mechanisms allowing fetal survival within the maternal uterus. Expert Rev Mol Med. (2007) 9:1–14. doi: 10.1017/S1462399407000294
11. Kalkunte S, Chichester CO, Gotsch F, Sentman CL, Romero R, Sharma S. Evolution of non-cytotoxic uterine natural killer cells. Am J Reprod Immunol. (2008) 59:425–32. doi: 10.1111/j.1600-0897.2008.00595.x
12. Mor G. Inflammation and pregnancy: the role of toll-like receptors in trophoblast-immune interaction. Ann N Y Acad Sci. (2008) 1127:121–8. doi: 10.1196/annals.1434.006
13. Robertson SA, Guerin LR, Moldenhauer LM, Hayball JD. Activating T regulatory cells for tolerance in early pregnancy - the contribution of seminal fluid. J Reprod Immunol. (2009) 83:109–16. doi: 10.1016/j.jri.2009.08.003
14. Erlebacher A. Immune surveillance of the maternal/fetal interface: controversies and implications. Trends Endocrinol Metab. (2010) 21:428–34. doi: 10.1016/j.tem.2010.02.003
15. Robertson SA. Immune regulation of conception and embryo implantation-all about quality control? J Reprod Immunol. (2010) 85:51–7. doi: 10.1016/j.jri.2010.01.008
16. Munoz-Suano A, Hamilton AB, Betz AG. Gimme shelter: the immune system during pregnancy. Immunol Rev. (2011) 241:20–38. doi: 10.1111/j.1600-065X.2011.01002.x
17. Arck PC, Hecher K. Fetomaternal immune cross-talk and its consequences for maternal and offspring's health. Nat Med. (2013) 19:548–56. doi: 10.1038/nm.3160
18. Erlebacher A. Mechanisms of T cell tolerance towards the allogeneic fetus. Nat Rev Immunol. (2013) 13:23–33. doi: 10.1038/nri3361
19. Gomez-Lopez N, Vega-Sanchez R, Castillo-Castrejon M, Romero R, Cubeiro-Arreola K, Vadillo-Ortega F. Evidence for a role for the adaptive immune response in human term parturition. Am J Reprod Immunol. (2013) 69:212–30. doi: 10.1111/aji.12074
20. Hahn S, Giaglis S, Chowdhury CS, Hosli I, Hasler P. Modulation of neutrophil NETosis: interplay between infectious agents and underlying host physiology. Semin Immunopathol. (2013) 35:439–53. doi: 10.1007/s00281-013-0380-x
21. Hemberger M. Immune balance at the foeto-maternal interface as the fulcrum of reproductive success. J Reprod Immunol. (2013) 97:36–42. doi: 10.1016/j.jri.2012.10.006
22. Shynlova O, Lee YH, Srikhajon K, Lye SJ. Physiologic uterine inflammation and labor onset: integration of endocrine and mechanical signals. Reprod Sci. (2013) 20:154–67. doi: 10.1177/1933719112446084
23. Tilburgs T, Strominger JL. CD8+ effector T cells at the fetal-maternal interface, balancing fetal tolerance and antiviral immunity. Am J Reprod Immunol. (2013) 69:395–407. doi: 10.1111/aji.12094
24. Gomez-Lopez N, StLouis D, Lehr MA, Sanchez-Rodriguez EN, Arenas-Hernandez M. Immune cells in term and preterm labor. Cell Mol Immunol. (2014) 11:571–81. doi: 10.1038/cmi.2014.46
25. Kwan M, Hazan A, Zhang J, Jones RL, Harris LK, Whittle W, et al. Dynamic changes in maternal decidual leukocyte populations from first to second trimester gestation. Placenta. (2014) 35:1027–34. doi: 10.1016/j.placenta.2014.09.018
26. Lima PD, Zhang J, Dunk C, Lye SJ, Croy BA. Leukocyte driven-decidual angiogenesis in early pregnancy. Cell Mol Immunol. (2014) 11:522–37. doi: 10.1038/cmi.2014.63
27. Lash GE. Molecular cross-talk at the feto-maternal interface. Cold Spring Harb Perspect Med. (2015) 5:a023010. doi: 10.1101/cshperspect.a023010
28. Mor G, Kwon JY. Trophoblast-microbiome interaction: a new paradigm on immune regulation. Am J Obstet Gynecol. (2015) 213:S131–7. doi: 10.1016/j.ajog.2015.06.039
29. Schumacher A, Zenclussen AC. The paternal contribution to fetal tolerance. Adv Exp Med Biol. (2015) 868:211–25. doi: 10.1007/978-3-319-18881-2_10
30. Giaglis S, Stoikou M, Sur Chowdhury C, Schaefer G, Grimolizzi F, Rossi SW, et al. Multimodal regulation of NET formation in pregnancy: progesterone antagonizes the pro-NETotic effect of estrogen and G-CSF. Front Immunol. (2016) 7:565. doi: 10.3389/fimmu.2016.00565
31. El-Azzamy H, Balogh A, Romero R, Xu Y, LaJeunesse C, Plazyo O, et al. Characteristic changes in decidual gene expression signature in spontaneous term parturition. J Pathol Transl Med. (2017) 51:264–83. doi: 10.4132/jptm.2016.12.20
32. Kinder JM, Stelzer IA, Arck PC, Way SS. Immunological implications of pregnancy-induced microchimerism. Nat Rev Immunol. (2017) 17:483–94. doi: 10.1038/nri.2017.38
33. Liu S, Diao L, Huang C, Li Y, Zeng Y, Kwak-Kim JYH. The role of decidual immune cells on human pregnancy. J Reprod Immunol. (2017) 124:44–53. doi: 10.1016/j.jri.2017.10.045
34. Shah NM, Herasimtschuk AA, Boasso A, Benlahrech A, Fuchs D, Imami N, et al. Changes in T cell and dendritic cell phenotype from mid to late pregnancy are indicative of a shift from immune tolerance to immune activation. Front Immunol. (2017) 8:1138. doi: 10.3389/fimmu.2017.01138
35. Zhang J, Dunk C, Croy AB, Lye SJ. To serve and to protect: the role of decidual innate immune cells on human pregnancy. Cell Tissue Res. (2016) 363:249–65. doi: 10.1007/s00441-015-2315-4
36. Cheng SB, Davis S, Sharma S. Maternal-fetal cross talk through cell-free fetal DNA, telomere shortening, microchimerism, and inflammation. Am J Reprod Immunol. (2018) 79:e12851. doi: 10.1111/aji.12851
37. Schumacher A, Sharkey DJ, Robertson SA, Zenclussen AC. Immune cells at the fetomaternal interface: how the microenvironment modulates immune cells to foster fetal development. J Immunol. (2018) 201:325–34. doi: 10.4049/jimmunol.1800058
38. Szekeres-Bartho J, Sucurovic S, Mulac-Jericevic B. The role of extracellular vesicles and PIBF in embryo-maternal immune-interactions. Front Immunol. (2018) 9:2890. doi: 10.3389/fimmu.2018.02890
39. Vacca P, Vitale C, Munari E, Cassatella MA, Mingari MC, Moretta L. Human innate lymphoid cells: their functional and cellular interactions in decidua. Front Immunol. (2018) 9:1897. doi: 10.3389/fimmu.2018.01897
40. Ander SE, Diamond MS, Coyne CB. Immune responses at the maternal-fetal interface. Sci Immunol. (2019) 4:eaat6114. doi: 10.1126/sciimmunol.aat6114
41. Yang X, Zhang C, Chen G, Sun C, Li J. Antibodies: the major participants in maternal-fetal interaction. J Obstet Gynaecol Res. (2019) 45:39–46. doi: 10.1111/jog.13839
42. Mor G, Cardenas I, Abrahams V, Guller S. Inflammation and pregnancy: the role of the immune system at the implantation site. Ann N Y Acad Sci. (2011) 1221:80–7. doi: 10.1111/j.1749-6632.2010.05938.x
43. Racicot K, Kwon JY, Aldo P, Silasi M, Mor G. Understanding the complexity of the immune system during pregnancy. Am J Reprod Immunol. (2014) 72:107–16. doi: 10.1111/aji.12289
44. Aghaeepour N, Ganio EA, McIlwain D, Tsai AS, Tingle M, Van Gassen S, et al. An immune clock of human pregnancy. Sci Immunol. (2017) 2:eaan2946. doi: 10.1126/sciimmunol.aan2946
45. Chavan AR, Griffith OW, Wagner GP. The inflammation paradox in the evolution of mammalian pregnancy: turning a foe into a friend. Curr Opin Genet Dev. (2017) 47:24–32. doi: 10.1016/j.gde.2017.08.004
46. Griffith OW, Chavan AR, Protopapas S, Maziarz J, Romero R, Wagner GP. Embryo implantation evolved from an ancestral inflammatory attachment reaction. Proc Natl Acad Sci USA. (2017) 114:E6566–75. doi: 10.1073/pnas.1701129114
47. Uckan D, Steele A, Cherry, Wang BY, Chamizo W, Koutsonikolis A, et al. Trophoblasts express Fas ligand: a proposed mechanism for immune privilege in placenta and maternal invasion. Mol Hum Reprod. (1997) 3:655–62. doi: 10.1093/molehr/3.8.655
48. Hunt JS, Petroff MG, McIntire RH, Ober C. HLA-G and immune tolerance in pregnancy. FASEB J. (2005) 19:681–93. doi: 10.1096/fj.04-2078rev
49. Mincheva-Nilsson L, Nagaeva O, Chen T, Stendahl U, Antsiferova J, Mogren I, et al. Placenta-derived soluble MHC class I chain-related molecules down-regulate NKG2D receptor on peripheral blood mononuclear cells during human pregnancy: a possible novel immune escape mechanism for fetal survival. J Immunol. (2006) 176:3585–92. doi: 10.4049/jimmunol.176.6.3585
50. Trowsdale J, Betz AG. Mother's little helpers: mechanisms of maternal-fetal tolerance. Nat Immunol. (2006) 7:241–6. doi: 10.1038/ni1317
51. Rusterholz C, Hahn S, Holzgreve W. Role of placentally produced inflammatory and regulatory cytokines in pregnancy and the etiology of preeclampsia. Semin Immunopathol. (2007) 29:151–62. doi: 10.1007/s00281-007-0071-6
52. Than NG, Romero R, Erez O, Weckle A, Tarca AL, Hotra J, et al. Emergence of hormonal and redox regulation of galectin-1 in placental mammals: implication in maternal-fetal immune tolerance. Proc Natl Acad Sci USA. (2008) 105:15819–24. doi: 10.1073/pnas.0807606105
53. Than NG, Romero R, Goodman M, Weckle A, Xing J, Dong Z, et al. A primate subfamily of galectins expressed at the maternal-fetal interface that promote immune cell death. Proc Natl Acad Sci USA. (2009) 106:9731–6. doi: 10.1073/pnas.0903568106
54. Hunt JS, Pace JL, Gill RM. Immunoregulatory molecules in human placentas: potential for diverse roles in pregnancy. Int J Dev Biol. (2010) 54:457–67. doi: 10.1387/ijdb.082831jh
55. Petroff MG, Perchellet A. B7 family molecules as regulators of the maternal immune system in pregnancy. Am J Reprod Immunol. (2010) 63:506–19. doi: 10.1111/j.1600-0897.2010.00841.x
56. Than NG, Romero R, Kim CJ, McGowen MR, Papp Z, Wildman DE. Galectins: guardians of eutherian pregnancy at the maternal-fetal interface. Trends Endocrinol Metab. (2012) 23:23–31. doi: 10.1016/j.tem.2011.09.003
57. Schumacher A, Costa SD, Zenclussen AC. Endocrine factors modulating immune responses in pregnancy. Front Immunol. (2014) 5:196. doi: 10.3389/fimmu.2014.00196
58. Than NG, Balogh A, Romero R, Karpati E, Erez O, Szilagyi A, et al. Placental protein 13 (PP13) - a placental immunoregulatory galectin protecting pregnancy. Front Immunol. (2014) 5:348. doi: 10.3389/fimmu.2014.00348
59. Cheng SB, Sharma S. Interleukin-10: a pleiotropic regulator in pregnancy. Am J Reprod Immunol. (2015) 73:487–500. doi: 10.1111/aji.12329
60. Kinder JM, Jiang TT, Ertelt JM, Xin L, Strong BS, Shaaban AF, et al. Tolerance to noninherited maternal antigens, reproductive microchimerism and regulatory T cell memory: 60 years after 'Evidence for actively acquired tolerance to Rh antigens'. Chimerism. (2015) 6:8–20. doi: 10.1080/19381956.2015.1107253
61. Than NG, Romero R, Balogh A, Karpati E, Mastrolia SA, Staretz-Chacham O, et al. Galectins: double-edged swords in the cross-roads of pregnancy complications and female reproductive tract inflammation and neoplasia. J Pathol Transl Med. (2015) 49:181–208. doi: 10.4132/jptm.2015.02.25
62. Zhang YH, Tian M, Tang MX, Liu ZZ, Liao AH. Recent insight into the role of the PD-1/PD-L1 pathway in feto-maternal tolerance and pregnancy. Am J Reprod Immunol. (2015) 74:201–8. doi: 10.1111/aji.12365
63. Ferreira LMR, Meissner TB, Tilburgs T, Strominger JL. HLA-G: at the interface of maternal-fetal tolerance. Trends Immunol. (2017) 38:272–86. doi: 10.1016/j.it.2017.01.009
64. Le Bouteiller P, Bensussan A. Up-and-down immunity of pregnancy in humans. F1000Res. (2017) 6:1216. doi: 10.12688/f1000research.11690.1
65. Schumacher A. Human chorionic gonadotropin as a pivotal endocrine immune regulator initiating and preserving fetal tolerance. Int J Mol Sci. (2017) 18:E2166. doi: 10.3390/ijms18102166
66. Chang RQ, Li DJ, Li MQ. The role of indoleamine-2,3-dioxygenase in normal and pathological pregnancies. Am J Reprod Immunol. (2018) 79:e12786. doi: 10.1111/aji.12786
67. Robertson SA, Care AS, Moldenhauer LM. Regulatory T cells in embryo implantation and the immune response to pregnancy. J Clin Invest. (2018) 128:4224–35. doi: 10.1172/JCI122182
68. Raghupathy R, Al-Mutawa E, Al-Azemi M, Makhseed M, Azizieh F, Szekeres-Bartho J. Progesterone-induced blocking factor (PIBF) modulates cytokine production by lymphocytes from women with recurrent miscarriage or preterm delivery. J Reprod Immunol. (2009) 80:91–9. doi: 10.1016/j.jri.2009.01.004
69. Prins JR, Gomez-Lopez N, Robertson SA. Interleukin-6 in pregnancy and gestational disorders. J Reprod Immunol. (2012) 95:1–14. doi: 10.1016/j.jri.2012.05.004
70. Whitten AE, Romero R, Korzeniewski SJ, Tarca AL, Schwartz AG, Yeo L, et al. Evidence of an imbalance of angiogenic/antiangiogenic factors in massive perivillous fibrin deposition (maternal floor infarction): a placental lesion associated with recurrent miscarriage and fetal death. Am J Obstet Gynecol. (2013) 208:310 e1–310 e11. doi: 10.1016/j.ajog.2013.01.017
71. Shemesh A, Tirosh D, Sheiner E, Tirosh NB, Brusilovsky M, Segev R, et al. First trimester pregnancy loss and the expression of alternatively spliced NKp30 isoforms in maternal blood and placental tissue. Front Immunol. (2015) 6:189. doi: 10.3389/fimmu.2015.00189
72. Romero R, Mazor M, Brandt F, Sepulveda W, Avila C, Cotton DB, et al. Interleukin-1 alpha and interleukin-1 beta in preterm and term human parturition. Am J Reprod Immunol. (1992) 27:117–23. doi: 10.1111/j.1600-0897.1992.tb00737.x
73. Romero R, Mazor M, Sepulveda W, Avila C, Copeland D, Williams J. Tumor necrosis factor in preterm and term labor. Am J Obstet Gynecol. (1992) 166:1576–87. doi: 10.1016/0002-9378(92)91636-O
74. Cherouny PH, Pankuch GA, Romero R, Botti JJ, Kuhn DC, Demers LM, et al. Neutrophil attractant/activating peptide-1/interleukin-8: association with histologic chorioamnionitis, preterm delivery, and bioactive amniotic fluid leukoattractants. Am J Obstet Gynecol. (1993) 169:1299–303. doi: 10.1016/0002-9378(93)90297-V
75. Gotsch F, Romero R, Kusanovic JP, Erez O, Espinoza J, Kim CJ, et al. The anti-inflammatory limb of the immune response in preterm labor, intra-amniotic infection/inflammation, and spontaneous parturition at term: a role for interleukin-10. J Matern Fetal Neonatal Med. (2008) 21:529–47. doi: 10.1080/14767050802127349
76. Gomez-Lopez N, Laresgoiti-Servitje E, Olson DM, Estrada-Gutierrez G, Vadillo-Ortega F. The role of chemokines in term and premature rupture of the fetal membranes: a review. Biol Reprod. (2010) 82:809–14. doi: 10.1095/biolreprod.109.080432
77. Romero R, Dey SK, Fisher SJ. Preterm labor: one syndrome, many causes. Science. (2014) 345:760–5. doi: 10.1126/science.1251816
78. Romero R, Grivel JC, Tarca AL, Chaemsaithong P, Xu Z, Fitzgerald W, et al. Evidence of perturbations of the cytokine network in preterm labor. Am J Obstet Gynecol. (2015) 213:836 e831–836 e818. doi: 10.1016/j.ajog.2015.07.037
79. Vora B, Wang A, Kosti I, Huang H, Paranjpe I, Woodruff TJ, et al. Meta-analysis of maternal and fetal transcriptomic data elucidates the role of adaptive and innate immunity in preterm birth. Front Immunol. (2018) 9:993. doi: 10.3389/fimmu.2018.00993
80. Xu Y, Romero R, Miller D, Silva P, Panaitescu B, Theis KR, et al. Innate lymphoid cells at the human maternal-fetal interface in spontaneous preterm labor. Am J Reprod Immunol. (2018) 79:e12820. doi: 10.1111/aji.12820
81. Than NG, Abdul Rahman O, Magenheim R, Nagy B, Fule T, Hargitai B, et al. Placental protein 13 (galectin-13) has decreased placental expression but increased shedding and maternal serum concentrations in patients presenting with preterm pre-eclampsia and HELLP syndrome. Virchows Arch. (2008) 453:387–400. doi: 10.1007/s00428-008-0658-x
82. Than NG, Erez O, Wildman DE, Tarca AL, Edwin SS, Abbas A, et al. Severe preeclampsia is characterized by increased placental expression of galectin-1. J Matern Fetal Neonatal Med. (2008) 21:429–42. doi: 10.1080/14767050802041961
83. Than NG, Romero R, Meiri H, Erez O, Xu Y, Tarquini F, et al. PP13, maternal ABO blood groups and the risk assessment of pregnancy complications. PLoS ONE. (2011) 6:e21564. doi: 10.1371/journal.pone.0021564
84. Hsu P, Nanan RK. Innate and adaptive immune interactions at the fetal-maternal interface in healthy human pregnancy and pre-eclampsia. Front Immunol. (2014) 5:125. doi: 10.3389/fimmu.2014.00125
85. Stoikou M, Grimolizzi F, Giaglis S, Schafer G, van Breda SV, Hoesli IM, et al. Gestational diabetes mellitus Is associated with altered neutrophil activity. Front Immunol. (2017) 8:702. doi: 10.3389/fimmu.2017.00702
86. Geldenhuys J, Rossouw TM, Lombaard HA, Ehlers MM, Kock MM. Disruption in the regulation of immune responses in the placental subtype of preeclampsia. Front Immunol. (2018) 9:1659. doi: 10.3389/fimmu.2018.01659
87. Tsuda S, Zhang X, Hamana H, Shima T, Ushijima A, Tsuda K, et al. Clonally expanded decidual effector regulatory T cells increase in late gestation of normal pregnancy, but not in preeclampsia, in humans. Front Immunol. (2018) 9:1934. doi: 10.3389/fimmu.2018.01934
88. Dam TK, Brewer CF. Lectins as pattern recognition molecules: the effects of epitope density in innate immunity. Glycobiology. (2010) 20:270–9. doi: 10.1093/glycob/cwp186
89. Vasta GR, Ahmed H, Nita-Lazar M, Banerjee A, Pasek M, Shridhar S, et al. Galectins as self/non-self recognition receptors in innate and adaptive immunity: an unresolved paradox. Front Immunol. (2012) 3:199. doi: 10.3389/fimmu.2012.00199
90. Schnaar RL. Glycobiology simplified: diverse roles of glycan recognition in inflammation. J Leukoc Biol. (2016) 99:825–38. doi: 10.1189/jlb.3RI0116-021R
91. Gitt MA, Barondes SH. Evidence that a human soluble beta-galactoside-binding lectin is encoded by a family of genes. Proc Natl Acad Sci USA. (1986) 83:7603–7. doi: 10.1073/pnas.83.20.7603
92. Cooper DN. Galectinomics: finding themes in complexity. Biochim Biophys Acta. (2002) 1572:209–31. doi: 10.1016/S0304-4165(02)00310-0
93. Rabinovich GA, Toscano MA. Turning 'sweet' on immunity: galectin-glycan interactions in immune tolerance and inflammation. Nat Rev Immunol. (2009) 9:338–52. doi: 10.1038/nri2536
94. Blois SM, Barrientos G. Galectin signature in normal pregnancy and preeclampsia. J Reprod Immunol. (2014) 101–2:127–34. doi: 10.1016/j.jri.2013.05.005
95. Arthur CM, Baruffi MD, Cummings RD, Stowell SR. Evolving mechanistic insights into galectin functions. Methods Mol Biol. (2015) 1207:1–35. doi: 10.1007/978-1-4939-1396-1_1
96. Terness P, Kallikourdis M, Betz AG, Rabinovich GA, Saito S, Clark DA. Tolerance signaling molecules and pregnancy: IDO, galectins, and the renaissance of regulatory T cells. Am J Reprod Immunol. (2007) 58:238–54. doi: 10.1111/j.1600-0897.2007.00510.x
97. Kopcow HD, Rosetti F, Leung Y, Allan DS, Kutok JL, Strominger JL. T cell apoptosis at the maternal-fetal interface in early human pregnancy, involvement of galectin-1. Proc Natl Acad Sci USA. (2008) 105:18472–7. doi: 10.1073/pnas.0809233105
98. Molvarec A, Blois SM, Stenczer B, Toldi G, Tirado-Gonzalez I, Ito M, et al. Peripheral blood galectin-1-expressing T and natural killer cells in normal pregnancy and preeclampsia. Clin Immunol. (2011) 139:48–56. doi: 10.1016/j.clim.2010.12.018
99. Ramhorst RE, Giribaldi L, Fraccaroli L, Toscano MA, Stupirski JC, Romero MD, et al. Galectin-1 confers immune privilege to human trophoblast: implications in recurrent fetal loss. Glycobiology. (2012) 22:1374–86. doi: 10.1093/glycob/cws104
100. Meggyes M, Miko E, Polgar B, Bogar B, Farkas B, Illes Z, et al. Peripheral blood TIM-3 positive NK and CD8+ T cells throughout pregnancy: TIM-3/galectin-9 interaction and its possible role during pregnancy. PLoS ONE. (2014) 9:e92371. doi: 10.1371/journal.pone.0092371
101. Hu XH, Tang MX, Mor G, Liao AH. Tim-3: expression on immune cells and roles at the maternal-fetal interface. J Reprod Immunol. (2016) 118:92–9. doi: 10.1016/j.jri.2016.10.113
102. Sun J, Yang M, Ban Y, Gao W, Song B, Wang Y, et al. Tim-3 is upregulated in NK cells during early pregnancy and inhibits NK cytotoxicity toward trophoblast in galectin-9 dependent pathway. PLoS ONE. (2016) 11:e0147186. doi: 10.1371/journal.pone.0147186
103. Maquoi E, van den Brule FA, Castronovo V, Foidart JM. Changes in the distribution pattern of galectin-1 and galectin-3 in human placenta correlates with the differentiation pathways of trophoblasts. Placenta. (1997) 18:433–9. doi: 10.1016/S0143-4004(97)80044-6
104. Van den Brule FA, Fernandez PL, Buicu C, Liu FT, Jackers P, Lambotte R, et al. Differential expression of galectin-1 and galectin-3 during first trimester human embryogenesis. Dev Dyn. (1997) 209:399–405. doi: 10.1002/(SICI)1097-0177(199708)209:4<399::AID-AJA7>3.0.CO;2-D
105. Vicovac L, Jankovic M, Cuperlovic M. Galectin-1 and−3 in cells of the first trimester placental bed. Hum Reprod. (1998) 13:730–5. doi: 10.1093/humrep/13.3.730
106. Than NG, Pick E, Bellyei S, Szigeti A, Burger O, Berente Z, et al. Functional analyses of placental protein 13/galectin-13. Eur J Biochem. (2004) 271:1065–78. doi: 10.1111/j.1432-1033.2004.04004.x
107. Blois SM, Ilarregui JM, Tometten M, Garcia M, Orsal AS, Cordo-Russo R, et al. A pivotal role for galectin-1 in fetomaternal tolerance. Nat Med. (2007) 13:1450–7. doi: 10.1038/nm1680
108. Jeschke U, Mayr D, Schiessl B, Mylonas I, Schulze S, Kuhn C, et al. Expression of galectin-1,−3 (gal-1, gal-3) and the Thomsen-Friedenreich (TF) antigen in normal, IUGR, preeclamptic and HELLP placentas. Placenta. (2007) 28:1165–73. doi: 10.1016/j.placenta.2007.06.006
109. Miko E, Meggyes M, Doba K, Barakonyi A, Szereday L. Immune checkpoint molecules in reproductive immunology. Front Immunol. (2019) 10:846. doi: 10.3389/fimmu.2019.00846
110. Than NG, Romero R, Xu Y, Erez O, Xu Z, Bhatti G, et al. Evolutionary origins of the placental expression of chromosome 19 cluster galectins and their complex dysregulation in preeclampsia. Placenta. (2014) 35:855–65. doi: 10.1016/j.placenta.2014.07.015
111. Bohn H, Kraus W, Winckler W. Purification and characterization of two new soluble placental tissue proteins (PP13 and PP17). Oncodev Biol Med. (1983) 4:343–50.
112. Yang QS, Ying K, Yuan HL, Chen JZ, Meng XF, Wang Z, et al. Cloning and expression of a novel human galectin cDNA, predominantly expressed in placenta(1). Biochim Biophys Acta. (2002) 1574:407–11. doi: 10.1016/S0167-4781(01)00319-0
113. Balogh A, Pozsgay J, Matko J, Dong Z, Kim CJ, Varkonyi T, et al. Placental protein 13 (PP13/galectin-13) undergoes lipid raft-associated subcellular redistribution in the syncytiotrophoblast in preterm preeclampsia and HELLP syndrome. Am J Obstet Gynecol. (2011) 205:156 e151–114. doi: 10.1016/j.ajog.2011.03.023
114. Kliman HJ, Sammar M, Grimpel YI, Lynch SK, Milano KM, Pick E, et al. Placental protein 13 and decidual zones of necrosis: an immunologic diversion that may be linked to preeclampsia. Reprod Sci. (2012) 19:16–30. doi: 10.1177/1933719111424445
115. Chafetz I, Kuhnreich I, Sammar M, Tal Y, Gibor Y, Meiri H, et al. First-trimester placental protein 13 screening for preeclampsia and intrauterine growth restriction. Am J Obstet Gynecol. (2007) 197:35 e31–7. doi: 10.1016/j.ajog.2007.02.025
116. Romero R, Kusanovic JP, Than NG, Erez O, Gotsch F, Espinoza J, et al. First-trimester maternal serum PP13 in the risk assessment for preeclampsia. Am J Obstet Gynecol. (2008) 199:122 e121–122 e111. doi: 10.1016/j.ajog.2008.01.013
117. Grill S, Rusterholz C, Zanetti-Dallenbach R, Tercanli S, Holzgreve W, Hahn S, et al. Potential markers of preeclampsia–a review. Reprod Biol Endocrinol. (2009) 7:70. doi: 10.1186/1477-7827-7-70
118. Wortelboer EJ, Koster MP, Cuckle HS, Stoutenbeek PH, Schielen PC, Visser GH. First-trimester placental protein 13 and placental growth factor: markers for identification of women destined to develop early-onset pre-eclampsia. BJOG. (2010) 117:1384–9. doi: 10.1111/j.1471-0528.2010.02690.x
119. Huppertz B, Kawaguchi R. First trimester serum markers to predict preeclampsia. Wien Med Wochenschr. (2012) 162:191–5. doi: 10.1007/s10354-012-0072-5
120. Allen RE, Rogozinska E, Cleverly K, Aquilina J, Thangaratinam S. Abnormal blood biomarkers in early pregnancy are associated with preeclampsia: a meta-analysis. Eur J Obstet Gynecol Reprod Biol. (2014) 182:194–201. doi: 10.1016/j.ejogrb.2014.09.027
121. Meiri H, Sammar M, Herzog A, Grimpel YI, Fihaman G, Cohen A, et al. Prediction of preeclampsia by placental protein 13 and background risk factors and its prevention by aspirin. J Perinat Med. (2014) 42:591–601. doi: 10.1515/jpm-2013-0298
122. Madar-Shapiro L, Karady I, Trahtenherts A, Syngelaki A, Akolekar R, Poon L, et al. Predicting the risk to develop preeclampsia in the first trimester combining promoter variant−98A/C of LGALS13 (Placental Protein 13), black ethnicity, previous preeclampsia, obesity, and maternal age. Fetal Diagn Ther. (2018) 43:250–65. doi: 10.1159/000477933
123. Chaiworapongsa T, Gervasi MT, Refuerzo J, Espinoza J, Yoshimatsu J, Berman S, et al. Maternal lymphocyte subpopulations (CD45RA+ and CD45RO+) in preeclampsia. Am J Obstet Gynecol. (2002) 187:889–93. doi: 10.1067/mob.2002.127309
124. Saito S, Shiozaki A, Nakashima A, Sakai M, Sasaki Y. The role of the immune system in preeclampsia. Mol Aspects Med. (2007) 28:192–209. doi: 10.1016/j.mam.2007.02.006
125. Zhou Y, Bianco K, Huang L, Nien JK, McMaster M, Romero R, et al. Comparative analysis of maternal-fetal interface in preeclampsia and preterm labor. Cell Tissue Res. (2007) 329:559–69. doi: 10.1007/s00441-007-0428-0
126. Laresgoiti-Servitje E. A leading role for the immune system in the pathophysiology of preeclampsia. J Leukoc Biol. (2013) 94:247–57. doi: 10.1189/jlb.1112603
127. Djurisic S, Hviid TV. HLA Class Ib molecules and immune cells in pregnancy and preeclampsia. Front Immunol. (2014) 5:652. doi: 10.3389/fimmu.2014.00652
128. Perez-Sepulveda A, Torres MJ, Khoury M, Illanes SE. Innate immune system and preeclampsia. Front Immunol. (2014) 5:244. doi: 10.3389/fimmu.2014.00244
129. Than NG, Romero R, Tarca AL, Kekesi KA, Xu Y, Xu Z, et al. Integrated systems biology approach identifies novel maternal and placental pathways of preeclampsia. Front Immunol. (2018) 9:1661. doi: 10.3389/fimmu.2018.01661
130. Burton GJ, Jauniaux E. Placental oxidative stress: from miscarriage to preeclampsia. J Soc Gynecol Investig. (2004) 11:342–52. doi: 10.1016/j.jsgi.2004.03.003
131. Gupta AK, Hasler P, Holzgreve W, Hahn S. Neutrophil NETs: a novel contributor to preeclampsia-associated placental hypoxia? Semin Immunopathol. (2007) 29:163–7. doi: 10.1007/s00281-007-0073-4
132. Gupta AK, Joshi MB, Philippova M, Erne P, Hasler P, Hahn S, et al. Activated endothelial cells induce neutrophil extracellular traps and are susceptible to NETosis-mediated cell death. FEBS Lett. (2010) 584:3193–7. doi: 10.1016/j.febslet.2010.06.006
133. Lynch AM, Salmon JE. Dysregulated complement activation as a common pathway of injury in preeclampsia and other pregnancy complications. Placenta. (2010) 31:561–7. doi: 10.1016/j.placenta.2010.03.010
134. Calleja-Agius J, Muttukrishna S, Pizzey AR, Jauniaux E. Pro- and antiinflammatory cytokines in threatened miscarriages. Am J Obstet Gynecol. (2011) 205:83 e88–16. doi: 10.1016/j.ajog.2011.02.051
135. Hahn S, Giaglis S, Hoesli I, Hasler P. Neutrophil NETs in reproduction: from infertility to preeclampsia and the possibility of fetal loss. Front Immunol. (2012) 3:362. doi: 10.3389/fimmu.2012.00362
136. Hargitai B, Marton T, Cox PM. Best practice no 178. Examination of the human placenta. J Clin Pathol. (2004) 57:785–92. doi: 10.1136/jcp.2003.014217
137. Committee on Practice Bulletins-Gynecology. The American College of Obstetricians and Gynecologists Practice Bulletin no. 150. Early pregnancy loss. Obstet Gynecol. (2015) 125:1258–67. doi: 10.1097/01.AOG.0000465191.27155.25
138. Redline RW. Placental pathology: a systematic approach with clinical correlations. Placenta. (2008) 29(Suppl. A):S86–91. doi: 10.1016/j.placenta.2007.09.003
139. Khong TY, Mooney EE, Ariel I, Balmus NC, Boyd TK, Brundler MA, et al. Sampling and definitions of placental lesions: Amsterdam Placental Workshop Group Consensus Statement. Arch Pathol Lab Med. (2016) 140:698–713. doi: 10.5858/arpa.2015-0225-CC
140. Varkonyi T, Nagy B, Fule T, Tarca AL, Karaszi K, Schonleber J, et al. Microarray profiling reveals that placental transcriptomes of early-onset HELLP syndrome and preeclampsia are similar. Placenta. (2011) 32(Suppl.):S21–29. doi: 10.1016/j.placenta.2010.04.014
141. Szabo S, Xu Y, Romero R, Fule T, Karaszi K, Bhatti G, et al. Changes of placental syndecan-1 expression in preeclampsia and HELLP syndrome. Virchows Arch. (2013) 463:445–58. doi: 10.1007/s00428-013-1426-0
142. Szabo S, Mody M, Romero R, Xu Y, Karaszi K, Mihalik N, et al. Activation of villous trophoblastic p38 and ERK1/2 signaling pathways in preterm preeclampsia and HELLP syndrome. Pathol Oncol Res. (2015) 21:659–68. doi: 10.1007/s12253-014-9872-9
143. Karaszi K, Szabo S, Juhasz K, Kiraly P, Kocsis-Deak B, Hargitai B, et al. Increased placental expression of placental protein 5 (PP5) / tissue factor pathway inhibitor-2 (TFPI-2) in women with preeclampsia and HELLP syndrome: relevance to impaired trophoblast invasion? Placenta. (2019) 76:30–9. doi: 10.1016/j.placenta.2019.01.011
144. Kapust RB, Tozser J, Fox JD, Anderson DE, Cherry S, Copeland TD, et al. Tobacco etch virus protease: mechanism of autolysis and rational design of stable mutants with wild-type catalytic proficiency. Protein Eng. (2001) 14:993–1000. doi: 10.1093/protein/14.12.993
145. Dam TK, Gabius HJ, Andre S, Kaltner H, Lensch M, Brewer CF. Galectins bind to the multivalent glycoprotein asialofetuin with enhanced affinities and a gradient of decreasing binding constants. Biochemistry. (2005) 44:12564–71. doi: 10.1021/bi051144z
146. Bocker S, Elling L. Binding characteristics of galectin-3 fusion proteins. Glycobiology. (2017) 27:457–68. doi: 10.1093/glycob/cwx007
147. Zhu C, Anderson AC, Schubart A, Xiong H, Imitola J, Khoury SJ, et al. The Tim-3 ligand galectin-9 negatively regulates T helper type 1 immunity. Nat Immunol. (2005) 6:1245–52. doi: 10.1038/ni1271
148. Stillman BN, Hsu DK, Pang M, Brewer CF, Johnson P, Liu FT, et al. Galectin-3 and galectin-1 bind distinct cell surface glycoprotein receptors to induce T cell death. J Immunol. (2006) 176:778–89. doi: 10.4049/jimmunol.176.2.778
149. Motran CC, Molinder KM, Liu SD, Poirier F, Miceli MC. Galectin-1 functions as a Th2 cytokine that selectively induces Th1 apoptosis and promotes Th2 function. Eur J Immunol. (2008) 38:3015–27. doi: 10.1002/eji.200838295
150. Zarnani AH. Recurrent pregnancy loss through the lens of immunology. J Reprod Infertil. (2015) 16:59–60.
151. Varla-Leftherioti M, Immunology in reproduction. In: Carp, HJA, Carp H, editors. Recurrent Pregnancy Loss Causes, Controversies and Treatment, 2nd ed. Boca Raton: CRC Press (2015). p 233–48.
152. Liu AX, Jin F, Zhang WW, Zhou TH, Zhou CY, Yao WM, et al. Proteomic analysis on the alteration of protein expression in the placental villous tissue of early pregnancy loss. Biol Reprod. (2006) 75:414–20. doi: 10.1095/biolreprod.105.049379
153. Unverdorben L, Haufe T, Santoso L, Hofmann S, Jeschke U, Hutter S. Prototype and chimera-type galectins in placentas with spontaneous and recurrent miscarriages. Int J Mol Sci. (2016) 17:644. doi: 10.3390/ijms17050644
154. Sun FR, Chen CQ, Yu M, Wang SC, Li DJ, Du MR. Galectin-9 promotes human trophoblast cell invasion through matrix metalloproteinase-2 and p38 signaling pathway. Reprod Dev Med. (2018) 2:1–7. doi: 10.4103/2096-2924.232880
155. Tirado-Gonzalez I, Freitag N, Barrientos G, Shaikly V, Nagaeva O, Strand M, et al. Galectin-1 influences trophoblast immune evasion and emerges as a predictive factor for the outcome of pregnancy. Mol Hum Reprod. (2013) 19:43–53. doi: 10.1093/molehr/gas043
156. Cao A, Alluqmani N, Buhari FHM, Wasim L, Smith LK, Quaile AT, et al. Galectin-9 binds IgM-BCR to regulate B cell signaling. Nat Commun. (2018) 9:3288. doi: 10.1038/s41467-018-05771-8
157. Giovannone N, Liang J, Antonopoulos A, Geddes Sweeney J, King SL, Pochebit SM, et al. Galectin-9 suppresses B cell receptor signaling and is regulated by I-branching of N-glycans. Nat Commun. (2018) 9:3287. doi: 10.1038/s41467-018-05770-9
158. He M, Jiang M, Zhou Y, Li F, Yang M, Fan Y, et al. Impaired Gal-9 dysregulates the PBMC-induced Th1/Th2 imbalance in abortion-prone matings. J Immunol Res. (2018) 2018:9517842. doi: 10.1155/2018/9517842
159. Lajko A, Meggyes M, Fulop BD, Gede N, Reglodi D, Szereday L. Comparative analysis of decidual and peripheral immune cells and immune-checkpoint molecules during pregnancy in wild-type and PACAP-deficient mice. Am J Reprod Immunol. (2018) 80:e13035. doi: 10.1111/aji.13035
160. Olivares EG, Munoz R, Tejerizo G, Montes MJ, Gomez-Molina F, Abadia-Molina AC. Decidual lymphocytes of human spontaneous abortions induce apoptosis but not necrosis in JEG-3 extravillous trophoblast cells. Biol Reprod. (2002) 67:1211–7. doi: 10.1095/biolreprod67.4.1211
161. Sacks G, Sargent I, Redman C. An innate view of human pregnancy. Immunol Today. (1999) 20:114–8. doi: 10.1016/S0167-5699(98)01393-0
162. Saito S, Nakashima A, Shima T, Ito M. Th1/Th2/Th17 and regulatory T-cell paradigm in pregnancy. Am J Reprod Immunol. (2010) 63:601–10. doi: 10.1111/j.1600-0897.2010.00852.x
163. Figueiredo AS, Schumacher A. The T helper type 17/regulatory T cell paradigm in pregnancy. Immunology. (2016) 148:13–21. doi: 10.1111/imm.12595
164. Lhuillier C, Barjon C, Niki T, Gelin A, Praz F, Morales O, et al. Impact of exogenous galectin-9 on human T cells: contribution of the T cell receptor complex to antigen-independent activation but not to apoptosis induction. J Biol Chem. (2015) 290:16797–811. doi: 10.1074/jbc.M115.661272
165. Perillo NL, Pace KE, Seilhamer JJ, Baum LG. Apoptosis of T cells mediated by galectin-1. Nature. (1995) 378:736–9. doi: 10.1038/378736a0
166. Toscano MA, Bianco GA, Ilarregui JM, Croci DO, Correale J, Hernandez JD, et al. Differential glycosylation of TH1, TH2 and TH-17 effector cells selectively regulates susceptibility to cell death. Nat Immunol. (2007) 8:825–34. doi: 10.1038/ni1482
167. Alderson MR, Tough TW, Davis-Smith T, Braddy S, Falk B, Schooley KA, et al. Fas ligand mediates activation-induced cell death in human T lymphocytes. J Exp Med. (1995) 181:71–7. doi: 10.1084/jem.181.1.71
168. Richter GH, Mollweide A, Hanewinkel K, Zobywalski C, Burdach S. CD25 blockade protects T cells from activation-induced cell death (AICD) via maintenance of TOSO expression. Scand J Immunol. (2009) 70:206–15. doi: 10.1111/j.1365-3083.2009.02281.x
169. Zhan Y, Carrington EM, Zhang Y, Heinzel S, Lew AM. Life and death of activated T cells: how are they different from naive T cells? Front Immunol. (2017) 8:1809. doi: 10.3389/fimmu.2017.01809
170. Batista A, Millan J, Mittelbrunn M, Sanchez-Madrid F, Alonso MA. Recruitment of transferrin receptor to immunological synapse in response to TCR engagement. J Immunol. (2004) 172:6709–14. doi: 10.4049/jimmunol.172.11.6709
171. Trowbridge IS, Omary MB. Human cell surface glycoprotein related to cell proliferation is the receptor for transferrin. Proc Natl Acad Sci USA. (1981) 78:3039–43. doi: 10.1073/pnas.78.5.3039
172. Taetle R, Castagnola J, Mendelsohn J. Mechanisms of growth inhibition by anti-transferrin receptor monoclonal antibodies. Cancer Res. (1986) 46:1759–63.
173. van der Leij J, van den Berg A, Blokzijl T, Harms G, van Goor H, Zwiers P, et al. Dimeric galectin-1 induces IL-10 production in T-lymphocytes: an important tool in the regulation of the immune response. J Pathol. (2004) 204:511–8. doi: 10.1002/path.1671
174. Li A, Dubey S, Varney ML, Dave BJ, Singh RK. IL-8 directly enhanced endothelial cell survival, proliferation, and matrix metalloproteinases production and regulated angiogenesis. J Immunol. (2003) 170:3369–76. doi: 10.4049/jimmunol.170.6.3369
175. Amsalem H, Kwan M, Hazan A, Zhang J, Jones RL, Whittle W, et al. Identification of a novel neutrophil population: proangiogenic granulocytes in second-trimester human decidua. J Immunol. (2014) 193:3070–9. doi: 10.4049/jimmunol.1303117
176. Lash GE, Ernerudh J. Decidual cytokines and pregnancy complications: focus on spontaneous miscarriage. J Reprod Immunol. (2015) 108:83–9. doi: 10.1016/j.jri.2015.02.003
177. Santoni A, Zingoni A, Cerboni C, Gismondi A. Natural killer (NK) cells from killers to regulators: distinct features between peripheral blood and decidual NK cells. Am J Reprod Immunol. (2007) 58:280–8. doi: 10.1111/j.1600-0897.2007.00513.x
178. Bruno A, Pagani A, Pulze L, Albini A, Dallaglio K, Noonan DM, et al. Orchestration of angiogenesis by immune cells. Front Oncol. (2014) 4:131. doi: 10.3389/fonc.2014.00131
179. Gamliel M, Goldman-Wohl D, Isaacson B, Gur C, Stein N, Yamin R, et al. Trained memory of human uterine NK cells enhances their function in subsequent pregnancies. Immunity. (2018) 48:951–62 e955. doi: 10.1016/j.immuni.2018.03.030
180. Blois SM, Conrad ML, Freitag N, Barrientos G. Galectins in angiogenesis: consequences for gestation. J Reprod Immunol. (2015) 108:33–41. doi: 10.1016/j.jri.2014.12.001
181. Gizurarson S, Huppertz B, Osol G, Skarphedinsson JO, Mandala M, Meiri H. Effects of placental protein 13 on the cardiovascular system in gravid and non-gravid rodents. Fetal Diagn Ther. (2013) 33:257–64. doi: 10.1159/000345964
182. Gizurarson S, Sigurdardottir ER, Meiri H, Huppertz B, Sammar M, Sharabi-Nov A, et al. Placental protein 13 administration to pregnant rats lowers blood pressure and augments fetal growth and venous remodeling. Fetal Diagn Ther. (2016) 39:56–63. doi: 10.1159/000381914
183. Drobnjak T, Gizurarson S, Gokina NI, Meiri H, Mandala M, Huppertz B, et al. Placental protein 13 (PP13)-induced vasodilation of resistance arteries from pregnant and nonpregnant rats occurs via endothelial-signaling pathways. Hypertens Pregnancy. (2017) 36:186–95. doi: 10.1080/10641955.2017.1295052
184. Drobnjak T, Jonsdottir AM, Helgadottir H, Runolfsdottir MS, Meiri H, Sammar M, et al. Placental protein 13 (PP13) stimulates rat uterine vessels after slow subcutaneous administration. Int J Womens Health. (2019) 11:213–22. doi: 10.2147/IJWH.S188303
185. Levine RJ, Maynard SE, Qian C, Lim KH, England LJ, Yu KF, et al. Circulating angiogenic factors and the risk of preeclampsia. N Engl J Med. (2004) 350:672–83. doi: 10.1056/NEJMoa031884
186. Gotsch F, Romero R, Friel L, Kusanovic JP, Espinoza J, Erez O, et al. CXCL10/IP-10: a missing link between inflammation and anti-angiogenesis in preeclampsia? J Matern Fetal Neonatal Med. (2007) 20:777–92. doi: 10.1080/14767050701483298
187. Erez O, Romero R, Espinoza J, Fu W, Todem D, Kusanovic JP, et al. The change in concentrations of angiogenic and anti-angiogenic factors in maternal plasma between the first and second trimesters in risk assessment for the subsequent development of preeclampsia and small-for-gestational age. J Matern Fetal Neonatal Med. (2008) 21:279–87. doi: 10.1080/14767050802034545
188. Gotsch F, Romero R, Kusanovic JP, Chaiworapongsa T, Dombrowski M, Erez O, et al. Preeclampsia and small-for-gestational age are associated with decreased concentrations of a factor involved in angiogenesis: soluble Tie-2. J Matern Fetal Neonatal Med. (2008) 21:389–402. doi: 10.1080/14767050802046069
189. Maynard S, Epstein FH, Karumanchi SA. Preeclampsia and angiogenic imbalance. Annu Rev Med. (2008) 59:61–78. doi: 10.1146/annurev.med.59.110106.214058
190. Romero R, Nien JK, Espinoza J, Todem D, Fu W, Chung H, et al. A longitudinal study of angiogenic (placental growth factor) and anti-angiogenic (soluble endoglin and soluble vascular endothelial growth factor receptor-1) factors in normal pregnancy and patients destined to develop preeclampsia and deliver a small for gestational age neonate. J Matern Fetal Neonatal Med. (2008) 21:9–23. doi: 10.1080/14767050701830480
191. Chaiworapongsa T, Romero R, Korzeniewski SJ, Kusanovic JP, Soto E, Lam J, et al. Maternal plasma concentrations of angiogenic/antiangiogenic factors in the third trimester of pregnancy to identify the patient at risk for stillbirth at or near term and severe late preeclampsia. Am J Obstet Gynecol. (2013) 208:287 e281–287 e215. doi: 10.1016/j.ajog.2013.01.016
192. Giaglis S, Stoikou M, Grimolizzi F, Subramanian BY, van Breda SV, Hoesli I, et al. Neutrophil migration into the placenta: Good, bad or deadly? Cell Adh Migr. (2016) 10:208–25. doi: 10.1080/19336918.2016.1148866
193. Tomimatsu T, Mimura K, Endo M, Kumasawa K, Kimura T. Pathophysiology of preeclampsia: an angiogenic imbalance and long-lasting systemic vascular dysfunction. Hypertens Res. (2017) 40:305–10. doi: 10.1038/hr.2016.152
194. Sahay AS, Jadhav AT, Sundrani DP, Wagh GN, Mehendale SS, Chavan-Gautam P, et al. VEGF and VEGFR1 levels in different regions of the normal and preeclampsia placentae. Mol Cell Biochem. (2018) 438:141–52. doi: 10.1007/s11010-017-3121-y
195. D'Haene N, Sauvage S, Maris C, Adanja I, Le Mercier M, Decaestecker C, et al. VEGFR1 and VEGFR2 involvement in extracellular galectin-1- and galectin-3-induced angiogenesis. PLoS ONE. (2013) 8:e67029. doi: 10.1371/journal.pone.0067029
196. Freitag N, Tirado-Gonzalez I, Barrientos G, Herse F, Thijssen VL, Weedon-Fekjaer SM, et al. Interfering with Gal-1-mediated angiogenesis contributes to the pathogenesis of preeclampsia. Proc Natl Acad Sci USA. (2013) 110:11451–6. doi: 10.1073/pnas.1303707110
197. Crocker PR. Mammalian Carbohydrate Recognition Systems. Berlin; New York, NY: Springer (2001). doi: 10.1007/978-3-540-46410-5
Keywords: angiogenesis, glycomics, immune privilege, PP13, trophoblast differentiation, trophoblast invasion
Citation: Balogh A, Toth E, Romero R, Parej K, Csala D, Szenasi NL, Hajdu I, Juhasz K, Kovacs AF, Meiri H, Hupuczi P, Tarca AL, Hassan SS, Erez O, Zavodszky P, Matko J, Papp Z, Rossi SW, Hahn S, Pallinger E and Than NG (2019) Placental Galectins Are Key Players in Regulating the Maternal Adaptive Immune Response. Front. Immunol. 10:1240. doi: 10.3389/fimmu.2019.01240
Received: 11 February 2019; Accepted: 16 May 2019;
Published: 19 June 2019.
Edited by:
Anne Fletcher, Monash University, AustraliaReviewed by:
Stephen Lye, Lunenfeld-Tanenbaum Research Institute, CanadaSandra Maria Blois, Charité Medical University of Berlin, Germany
Copyright © 2019 Balogh, Toth, Romero, Parej, Csala, Szenasi, Hajdu, Juhasz, Kovacs, Meiri, Hupuczi, Tarca, Hassan, Erez, Zavodszky, Matko, Papp, Rossi, Hahn, Pallinger and Than. This is an open-access article distributed under the terms of the Creative Commons Attribution License (CC BY). The use, distribution or reproduction in other forums is permitted, provided the original author(s) and the copyright owner(s) are credited and that the original publication in this journal is cited, in accordance with accepted academic practice. No use, distribution or reproduction is permitted which does not comply with these terms.
*Correspondence: Nandor Gabor Than, dGhhbi5nYWJvciYjeDAwMDQwO3R0ay5tdGEuaHU=