- 1Research Division for Biotechnology, Korea Atomic Energy Research Institute, Jeongeup, South Korea
- 2Department of Internal Medicine, Korea University College of Medicine, Seoul, South Korea
- 3Department of Radiation Science and Technology, University of Science and Technology, Daejeon, South Korea
- 4DRI and BK21 Plus Program, Department of Oral Microbiology and Immunology, School of Dentistry, Seoul National University, Seoul, South Korea
- 5State Key Laboratory of Veterinary Etiological Biology, National Foot and Mouth Disease Reference Laboratory, Lanzhou Veterinary Research Institute, Chinese Academy of Agricultural Sciences, Lanzhou, China
- 6Department of Microbiology, Ewha Womans University College of Medicine, Seoul, South Korea
Streptococcus pneumoniae is the most common respiratory bacterial pathogen among cases of community-acquired infection in young children, older adults, and individuals with underlying medical conditions. Although capsular polysaccharide-based pneumococcal vaccines have contributed to significant decrease in invasive pneumococcal infections, these vaccines have some limitations, including limited serotype coverage, lack of effective mucosal antibody responses, and high costs. In this study, we investigated the safety and immunogenicity of a live, whole-cell pneumococcal vaccine constructed by deleting the gene for prolipoprotein diacylglyceryl transferase (lgt) from the encapsulated pneumococcal strain TIGR4 (TIGR4Δlgt) for protection against heterologous pneumococcal strains. Pneumococcal strain TIGR4 was successfully attenuated by deletion of lgt, resulting in the loss of inflammatory activity and virulence. TIGR4Δlgt colonized the nasopharynx long enough to induce strong mucosal IgA and IgG2b-dominant systemic antibody responses that were cross-reactive to heterologous pneumococcal serotypes. Finally, intranasal immunization with TIGR4Δlgt provided serotype-independent protection against pneumococcal challenge in mice. Taken together, our results suggest that TIGR4Δlgt is an avirulent and attractive broad-spectrum pneumococcal vaccine candidate. More broadly, we assert that modulation of such “master” metabolic genes represents an emerging strategy for developing more effective vaccines against numerous infectious agents.
Introduction
Streptococcus pneumoniae (Sp, also known as pneumococcus) is the most common bacterial pathogen causing human diseases such as otitis media, pneumonia, and life-threatening invasive pneumococcal diseases, including meningitis and sepsis. It is responsible for an estimated 900,000 cases of pneumonia, 40,000 cases of invasive pneumococcal disease, and 4,000 associated deaths annually in the U.S. alone (1). Sp asymptomatically colonizes the nasopharynx of ~60% of the population and disseminates to cause invasive disease under immunocompromised conditions and co-infection with respiratory viral infections (2). Thus, immunization against pneumococcus is strongly recommended for populations at risk, including young children, the elderly, and patients with underlying medical conditions (3). A mucosal protective immune response against pneumococcus may be the most effective strategy for preventing opportunistic and invasive infections by eliminating early pneumococcal colonization of the nasopharynx (4, 5).
Currently, two types of licensed pneumococcal vaccines are available, both of which are based on the generation of antibodies against pneumococcal capsular polysaccharides (6). A 23-valent pneumococcal PS vaccine (PPV23), composed of 23 different capsular polysaccharide from commonly encountered serotypes, is widely recommended for older adults but not for young children due to its low immunogenicity in infants (7). A protein-conjugated capsular polysaccharide vaccine (PCV) was initially developed to provide protection against the seven most prevalent serotypes causing invasive pneumococcal disease in young children. This was later expanded to include 10 or 13 of the most invasive pneumococcal serotypes and is highly immunogenic in both adults and infants (8). A dramatic decrease in the burden of invasive pneumococcal diseases has been reported in many countries in which PCVs are in widespread use (9, 10). However, both vaccines are complex and costly to manufacture, making them inaccessible for low-income populations. Additionally, these serotype-based vaccines have significant limitations in serotype coverage, resulting in serotypes being increasingly replaced by non-vaccine serotypes or the emergence of new serotypes and non-typeable pneumococcal strains that cause invasive pneumococcal disease (11–13). Although the overall incidence of invasive pneumococcal disease has declined by 47% as a result of the introduction of PCVs, the prevalence of non-vaccine serotypes (21, 23B, 33F, and 35F) has significantly increased among asymptomatic carriers and invasive pneumococcal diseases in countries in which a PCV is used nationwide (3, 14). In addition, both PPVs and PCVs have recently been reported to produce diminished mucosal immune responses, especially in the production of immunoglobulin A (IgA), a predominant Ig isotype at the respiratory surface commonly colonized by pneumococcus (15). Due to these limitations, inactivated and live whole-cell-based vaccines have been extensively studied as cost-effective and broad-spectrum vaccine candidates at the clinical and pre-clinical levels (16–19). Also, a low cost of production and an ease of storage and handling of whole cell vaccines compared to sophisticated alternative vaccines, such as PCV and PPV, would facilitate the widespread use of them in the developing and undeveloped countries, where the vaccination for pneumococcus is greatly limited. Likewise, the development of inactivated whole-cell-based vaccines for pneumococcus has been ongoing in both clinical and preclinical trials since 1911, and recent meta-analyses have affirmed their efficacy (18, 20).
As another approach for developing whole-cell-based vaccines, live attenuated vaccines are advantageous, as they enhance humoral and mucosal immune responses following a single-dose vaccination, in contrast to inactivated whole-cell-based vaccines (4, 21). However, the main obstacle to the development of live attenuated vaccines is achieving satisfactory attenuation without compromising immunogenicity. To date, most live attenuated pneumococcal vaccine strains have been constructed in a capsule-negative background and carry additional mutations in important virulence genes, such as pspA, ply, and lytA (21, 22). However, these live attenuated vaccines may be suboptimally immunogenic due to the deletion of several virulence genes that also induce protective immune responses. To overcome such drawbacks, central regulatory or component “master” genes have been used as alternative targets for live attenuated vaccine development, since their deletion may downregulate, but not necessarily silence the expression of many virulence and metabolic genes (19, 23). For example, a pneumococcal mutant strain deficient in the gene ftsY, which encodes a central component of the signal recognition particle (SRP) pathway responsible for delivering membrane and secretory proteins, was highly protective against multiple serotypes in mouse models of pneumococcal otitis media and invasive pneumococcal disease by bolstering IgG2 serum responses (21).
Lipoprotein diacylglyceryl transferase (Lgt) is a highly conserved, commitment-step enzyme that catalyzes diacylglyceryl attachment to an N-terminal cysteine residue in a consensus peptide sequence known as a pre-prolipoprotein on the membrane (24, 25). It is essential for growth in most Gram-negative bacteria but not in Streptococcus spp. or Staphylococcus spp. (26). Lipoproteins are membrane-anchoring proteins, which locates beneath peptidoglycan layer in Gram-positive bacteria, with a variety of physiological and pathogenic functions in bacteria, including roles in cell division, conjugation, nutrient acquisition, adhesion, invasion, and immune evasion. In addition, the lipoprotein lipid moiety significantly contributes to the activation of Toll-like receptor 2 (TLR2)-mediated host innate immune responses (27–29). Following the diacylation of pre-prolipoproteins by Lgt, the N-terminal signal peptide is cleaved off by lipoprotein signal peptidase II (Lsp), resulting in a mature lipoprotein (25, 30, 31). Deletion of lgt in Gram-positive bacteria can strongly attenuate virulence, resulting in improper lipoprotein anchoring in the cytoplasmic membrane (32–36). A previous study also showed that a pneumococcal mutant strain deficient in lgt exhibited reduced TLR2-mediated inflammatory responses in vitro and diminished invasiveness in vivo (37). Since lgt-mutant pneumococcal strains exhibit greatly diminished virulence while still expressing many surface antigens, we hypothesized that a novel intranasal vaccine based on an lgt-mutant strain would be a promising and effective pneumococcal live attenuated vaccines candidate.
In this study, we aimed to develop an encapsulated pneumococcal TIGR4 strain deficient in lgt (TIGR4Δlgt) and evaluate its efficacy in protecting against infection from heterologous pneumococcal strains in mice.
Materials and Methods
Reagents
All antibodies, purified proteins, antibiotics, and other reagents used in this study were purchased from Sigma-Aldrich (St. Louis, MO, USA). The mouse TNF-α enzyme-linked immunosorbent assay (ELISA) kit was purchased from eBioscience (San Diego, CA, USA). An anti-PsaA monoclonal antibody was kindly provided by Prof. Nahm (University of Alabama at Birmingham, AL, USA).
Bacterial Strains and Generation of Supernatants and Crude Extracts
The bacteria and plasmids used in this study are listed in Supplement Table 1. Streptococcus pneumoniae (Sp) strain TIGR4 was obtained from the American Type Culture Collection (ATCC; Manassas, VA, USA). Sp serotypes 2 (D39), 3 (wu2), 6B, 9V, 19F, and 23F were kindly provided by Prof. Nahm (University of Alabama at Birmingham). Pneumococcal strains were grown in Todd-Hewitt broth (THB; Difco, Franklin Lakes, NJ, USA) supplemented with 0.5% yeast extract (Difco). At mid-log phase [optical density at 600 nm (OD600) of 0.3–0.4], bacteria were pelleted by centrifugation, and culture supernatants were filtered through a 0.22-μm membrane filter (Merck Millipore, Billerica, MA, USA). Bacterial pellets were resuspended in phosphate-buffered saline (PBS; Lonza, Basel, Switzerland).
Western Blotting Analysis
Bacterial culture supernatants were loaded and separated on Bis-Tris Bolt sodium dodecyl sulfate polyacrylamide gels (Invitrogen, Carlsbad, CA, USA), followed by transfer to nitrocellulose membranes (Bio-Rad, Hercules, CA, USA). The membranes were blocked with 5% dry skim milk (Bio-Rad) in PBS/0.05% Tween-20 (PBS-T) and then incubated with anti-PsaA monoclonal antibody (Xir-126) or anti-phosphocholine monoclonal antibody (TEPC-15, Sigma-Aldrich). The membrane was then washed with PBS-T and incubated with a horseradish peroxidase-conjugated rabbit anti-mouse immunoglobulin (Southern Biotech, Birmingham, AL, USA). Membrane-bound peroxidase was detected with 3,3′,5,5′-tetramethylbenzidine substrate solution (Thermo-Fisher Scientific, Waltham, MA, USA).
Construction of lgt-Deficient TIGR Strain
Primers used in this study are listed in Supplement Table 2. A gene replacement cassette was constructed by cloning the chromosomal regions flanking lgt and inserting them upstream and downstream of the kan gene in pK326, as described previously (38). A 1,023-bp upstream segment and a 930-bp downstream segment were amplified using paired primers (LGT-UpF/LGT-UpR and LGT-DnF/LGT-DnR, respectively). Amplified fragments were then cloned sequentially into the pK326 plasmid, resulting in the pKO-lgt plasmid, which was then introduced into TIGR4 by natural transformation, as previously described (39). In brief, a culture of TIGR4 was diluted 100-fold in fresh transformation THB supplemented with 2% bovine serum albumin (BSA; Sigma-Aldrich), 0.1% CaCl2 (Sigma-Aldrich), and 200 ng/ml competence-stimulating peptide. After incubation at 37°C for 14 min, cells were mixed with ~5 μg of pKO-lgt, followed by an additional 2 h of incubation at 37°C. Transformed cells were then plated onto blood agar plates containing 300 μg/ml kanamycin (Sigma-Aldrich) for selection of the TIGR4Δlgt strain.
Inactivation of lgt-Deficient TIGR Strain
Harvested TIGR4Δlgt (1 × 1010 CFU/ml) were irradiated using a 60Co-gamma irradiator (point source AECL, IR-79, MDS Nordion International Co., Ottawa, ON, Canada) at the Advanced Radiation Technology Institute of Korea Atomic Energy Research Institute (Jeoneup, Korea) with absorbed dose of 5 kGy for 1 h at room temperature.
Mammalian Cells and Culture Conditions
Mouse RAW264.7 cells (TIB-71) were obtained from ATCC. RAW264.7 cells were cultured in Dulbecco's modified Eagle's medium (Cellgro Mediatech, Herndon, VA, USA) supplemented with 10% heat-inactivated fetal bovine serum (HyClone, Logan, UT, USA), 100 units/ml penicillin, and 100 μg/ml streptomycin at 37°C in a humidified incubator with 5% CO2.
Measurement of Nitrite and TNF-α
Thioglycollate-elicited peritoneal cells were collected from wild-type (WT), ΔTLR2, and ΔTLR4 male C57BL/6 mice at 6 weeks of age provided kindly from Prof. Suzanne Michalek (University of Alabama at Birminghum). Mouse primary peritoneal cells or RAW264.7 cells were dispensed into 96-well plates (SPL, Suwon, Korea) at a density of 2 × 105 cells/ml and stimulated with TIGR4 or TIGR4Δlgt for 12 h. For nitrite measurement, 100 μl of culture supernatant was mixed with an equal volume of Griess reagent (1% sulfanilamide, 0.1% naphthylethylenediamine dihydrochloride, and 2% phosphoric acid) and incubated at RT for 5 min. The optical density was then measured at a wavelength of 540 nm using a Victor X3 light plate reader (Perkin-Elmer, Waltham, MA, USA). NaNO2 solution was used to generate the standard curve. The amount of TNF-α in the cell culture supernatant was determined with a commercially available sandwich-type ELISA (eBioscience) according to the manufacturer's protocol.
Purification of Lipoteichoic Acid (LTA)
Pneumococcal LTA was prepared using organic solvent extraction, octyl-Sepharose, and ion-exchange chromatography, as previously described (40). Levels of endotoxin contamination were determined using the QCL-1000 quantitative chromogenic Limulus Amoebocyte Lysate (LAL) assay (Bio-Whittaker, Walkersville, MD, USA) according to the manufacturer's instructions.
Microarray Analysis
To examine the effect of the loss of lipoprotein biosynthesis on global gene expression in Sp, microarray analysis was performed to compare transcript levels between WT TIGR4 and TIGR4Δlgt, as described previously (41). Total bacterial RNA was isolated from cells grown statically to mid-log phase (OD600 = ~0.35) using an SV total RNA isolation kit (Promega, Madison, WI, USA) according to the manufacturer's instructions. A total of 75 μg RNA with 3 μg random hexamer dissolved in 29.5 μl nuclease free-water was denatured at 65°C for 10 min and then placed on ice. After adding 6 μl of 0.1 M dithiothreitol, 12 μl first-strand buffer, 1.5 μl dNTP mix (25 mM dATP, 25 mM dGTP, 25 mM dCTP, 10 mM dTTP), 4 μl Superscript II® reverse transcriptase (Invitrogen), 2 μl RNasin (Promega), and 4 μl of either Cy3- or Cy5-conjugated dTTP (Amersham Biosciences, Buckinghamshire, UK), the labeling mixture (60 μl) was incubated at 42°C for 2 h and supplemented with 2 μl Superscript II at the end of the first hour. Each probe was then denatured with 10 μl of 1 M NaOH and neutralized with 10 μl of 1 M HCl, followed by purification with a PCR purification kit (Qiagen, Venlo, Netherlands). It was then concentrated with a speed vacuum drier. Each probe separately labeled with Cy3 or Cy5 was resuspended in 20 μl distilled water. Whole-genome TIGR4 oligonucleotide microarray chips were obtained from the J. Craig Venter Institute (Rockville, MD, USA). The array consisted of 70-mer oligonucleotide probes, representing 2060 TIGR4 open-reading frames (ORFs) and 457 ORFs from strains G54 and R6. Each oligonucleotide probe sequence was spotted at least five times on the array. All microarray experiments were performed by eBiogen (Seoul, Korea). In brief, equal volumes of labeled probes (20 μl each) from the TIGR4 and TIGR4Δlgt strains were mixed with 40 μl of 2 × hybridization solution consisting of 50% formamide, 10 × saline-sodium citrate, and 0.2% SDS and denatured by boiling for 5 min. Probes were simultaneously hybridized to a chip overnight at 42°C in a hybridization chamber (Corning, Corning, NY, USA) submerged in water. Scans were performed with a Scan Array 5,000 laser scanner using ScanArray 2.1 software (Packard BioChip Technologies, Billerica, MA, USA). Signal intensity was quantified using QuantArray 3.0 software (Packard BioChip Technologies). The statistical significance of differential expression relative to that in the control was assessed using a paired t-test and Bayesian analysis, as Bayesian estimates of within-treatment variation tend to reduce the rate of false positives (42). The threshold for a significant change in gene expression was set at P < 0.001. Of the genes passing this threshold, only those with a Bayesian confidence value (B-value) above zero were regarded as being significantly affected by Δlgt mutation. B-values were computed using Cyber-T (cybert.microarray.ics.uci.edu).
Quantitative Real-Time PCR (qRT-PCR) Analysis
Total RNAs were isolated from log-phase cultures of WT TIGR4 and TIGR4Δlgt using an RNeasy® mini kit (Qiagen) according to the manufacturer's instructions. For real-time PCR analysis, cDNA was synthesized from 1 μg total purified RNA using a Primescript 1st strand cDNA synthesis Kit (Takara Bio Inc., Kyoto, Japan) following the manufacturer's instructions. Primers for various genes were designed using Primer Express v2.0 software and are listed in Supplement Table 2. qRT-PCR amplification was performed with SYBR Premix Ex Taq (Takara Bio Inc.) on a Bio-Rad CFX Real-Time System. PCR reactions were performed with one cycle of 95°C for 5 min; 40 cycles of 95°C for 15 s, 60°C for 30 s, and 72°C for 30 s; and one cycle of 72°C for 10 min. Relative quantification of gene expression was determined by the comparative threshold (ΔΔCt) method. The 16S rRNA gene (rrsH) was used as a control to normalize the expression levels of target genes.
Animal Experiments
All animal experiments conducted in this study were approved by the Committee on the Use and Care of Animals at the Korea Atomic Energy Research Institute (KAERI) and performed according to accepted veterinary standards. Six-week-old male C57BL/6 mice were purchased from Orient Bio Inc. and were intranasally (i.n.) vaccinated twice at 14-day intervals with live or killed TIGR4Δlgt. At 10 days after the second vaccination, blood was collected, and antibodies specific to pneumococcus were measured by ELISA. To examine the protective effect of TIGR4Δlgt vaccination, mice were i.n. infected with pneumococcal strains TIGR4, wu2, or D39 at 10 days after the second vaccination. Mouse survival was monitored for 10 days after infection. Blood, nasal washes, and lungs were collected at the time of death or at the end of the experiment for bacterial counting. Tissues were homogenized in 1.5 ml PBS and passed through a 40-μm mesh strainer, and bacterial numbers were then counted.
Measurement of Mouse Immunoglobulin Levels
Mouse blood samples were taken every 2 weeks. Serum was isolated via centrifugation and stored at −80°C until use. Pneumococcal strains were cultured in THY (THB with 2% yeast extract) and harvested at mid-log phase. The absorbance of the pneumococcal pellet was adjusted to an OD600 of 0.1 by dilution with PBS. Then, 96-well immunoplates (SPL) were coated with 100 μl pneumococcal suspension and incubated overnight at 4°C to allow adherence of bacterial cells. The plates were then washed five times with PBS-T, followed by blocking with 1% BSA in PBS for 1 h at RT. After blocking, diluted serum was added to each well and incubated at RT for 1 h, and unbound antibodies were removed by washing with PBS-T. Appropriate dilutions of goat anti-mouse Ig-HRP (Sigma-Aldrich), goat anti-mouse IgG-HRP (Southern-Biotech), or goat anti-mouse IgM-HRP (Southern-Biotech) were added to wells and incubated for 30 min at RT. After washing the plates five times with PBS-T, 100 μl TMB substrate reagent (BD Biosciences, Franklin Lakes, NJ, USA) was added. When colors developed, 50 μl of 2 N H2SO4 was added, and the absorbance was measured at 450 nm using a Victor X3 light plate reader (Perkin-Elmer).
Hematoxylin and Eosin (H&E) Staining
Following i.n. inoculation of WT TIGR4 or TIGR4Δlgt, mouse lungs were harvested at 24 h after inoculation. The left lung of each mouse was fixed with 4% paraformaldehyde prior to paraffin embedding to preserve the pulmonary architecture. Paraffin-embedded tissues were then cut into 4-μm-thick sections and stained with H&E to observe histopathological changes. Tissue images were captured with a light microscope (Eclipse Ci-L; Nikon Corp., Tokyo, Japan) and processed using i-Solution Lite software (iMTechnology, Vancouver, BC, Canada).
Data Analysis
Data are expressed as mean ± standard deviation (SD). Data in bar graphs and bacterial numbers between groups were compared by unpaired t-tests. Mouse survival was analyzed by Kaplan–Meier analysis. The significance of differences was assessed by log-rank test using GraphPad Prism (GraphPad Software, La Jolla, CA, USA). Differences with P-values < 0.05 were considered statistically significant.
Results
Biological Characteristics of lgt-Deficient Pneumococcus
To investigate its potential clinical use as a pneumococcal live attenuated vaccine, an lgt-deficient pneumococcal strain was constructed from the pneumococcal strain TIGR4 (TIGR4Δlgt). Its biological properties were then examined. First, to verify whether lipoprotein biosynthesis was abrogated in TIGR4Δlgt, the localization of PsaA, a major lipoprotein, was examined in cell lysates and culture supernatants (Figure 1A). As expected, the expression of PsaA was detected in the cell lysate of wild-type (WT) TIGR4, while PsaA accumulated in the culture supernatant of TIGR4Δlgt, indicating its improper anchoring in the membrane. Of note, TIGR4Δlgt grew well in nutrient-rich medium, similar to its parent strain (data not shown).
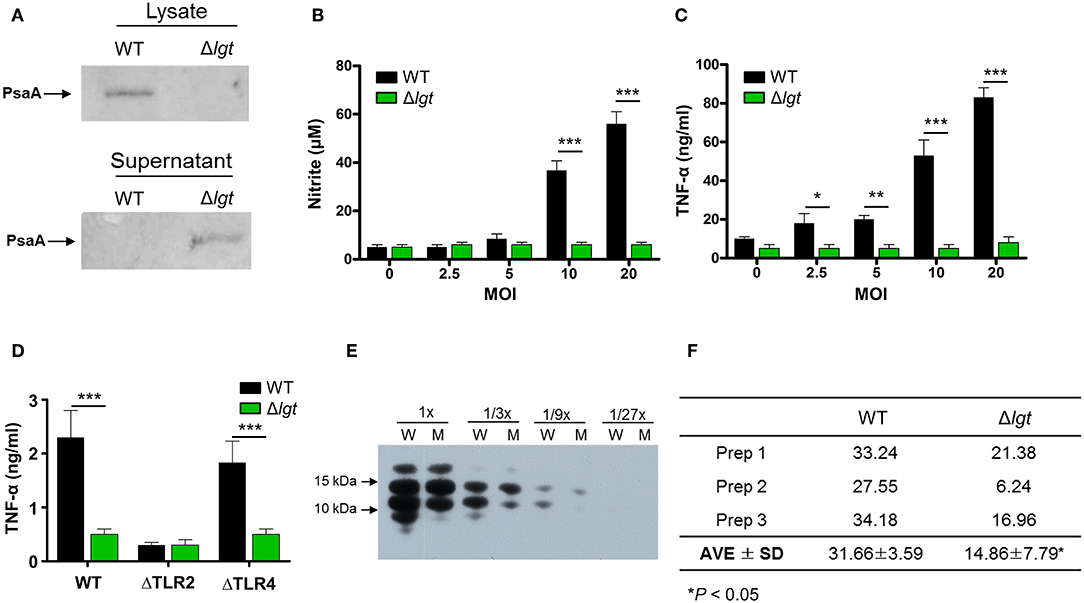
Figure 1. Biochemical and Immunological Characteristics of TIGR4Δlgt. (A) Expression of PsaA protein in cell lysates and culture supernatants from wild-type (WT) and lgt-deficient (Δlgt) TIGR4 strains. (B,C) Nitric oxide (B) and TNF-α (C) production in culture supernatants of RAW264.7 cells treated with WT or Δlgt strain for 12 h. (D) TNF-α production in the culture supernatants of peritoneal cells from wild-type (WT), TLR2-deficient (ΔTLR2), and TLR4-deficient (ΔTLR4) mice infected with WT or Δlgt strain for 12 h. Data in (B,C), and (D) represent mean values ± S.D. of triplicate results. *P < 0.05, **P < 0.01, and ***P < 0.001 compared to the WT treatment group. (E) LTA expression levels in 3-fold serial dilutions of culture supernatants from WT (W) or Δlgt (M) strains. (F) Quantification of LTA isolated from the pellets of WT and Δlgt strains. *P < 0.05 compared to the WT group.
Since pneumococcus can produce over 32 families of lipoproteins and more than 88 lipoprotein-related proteins (43), it was possible that the lgt-deficient mutant would exhibit impairment of cellular functions associated with transporting and synthesizing membrane components. To determine whether the pneumococcal transcriptome was altered by lgt deletion, gene expression microarray analysis was performed. In TIGR4Δlgt, 164 genes were upregulated, and 161 genes were downregulated compared to their expression levels in the TIGR4 parent strain (P < 0.01). Among these, the mRNA expression levels of previously identified vaccine targets, such as choline binding proteins, lipoproteins, and LPxTG motif cell wall-anchoring proteins were further analyzed (Tables 1, 2). This revealed that, of 18 selected vaccine targets, expression levels of potD, vgcL, clpP, and htrK were downregulated but not significantly so in TIGR4Δlgt, while the expression of the other genes was similar to or significantly higher than that in TIGR4.
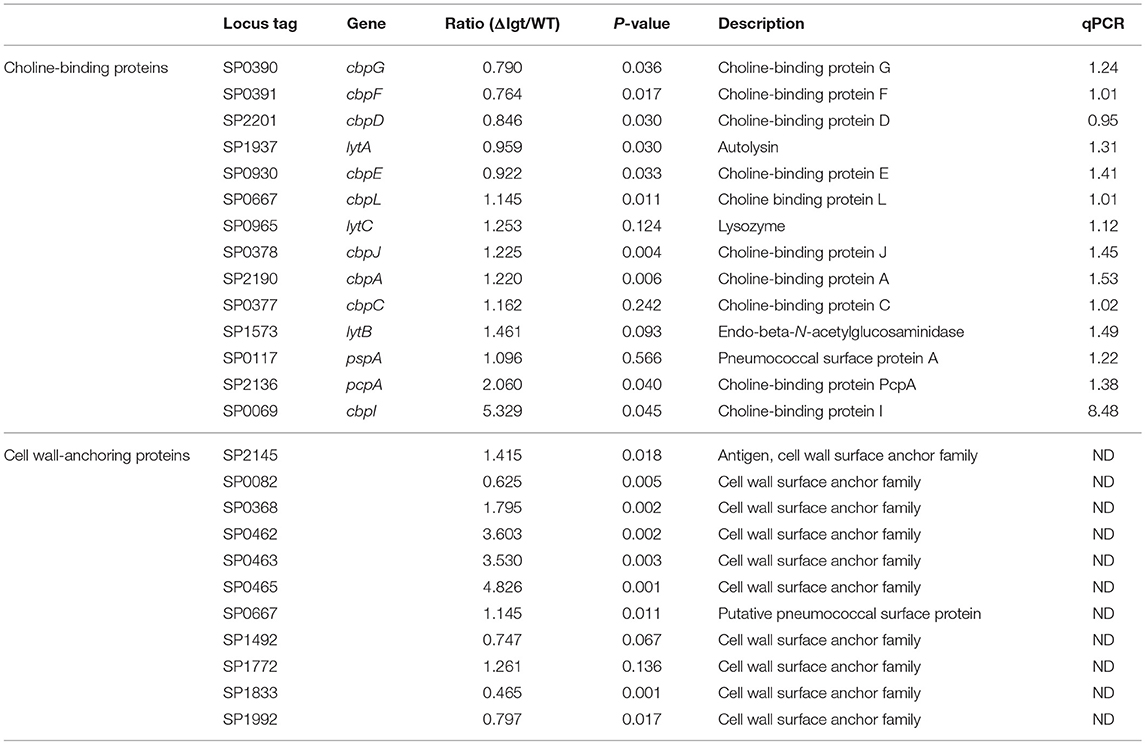
Table 2. Expression of choline-binding protein members and cell wall-anchoring protein genes in wild-type TIGR4 and TIGR4Δlgt pneumococci.
Lipoproteins are known to be potential inflammatory stimulants via the activation of TLR2. Various Gram-positive bacteria deficient in lgt have shown significant loss or complete abolition of inflammation-stimulating activity (29, 37). To determine the inflammation-stimulating activity of TIGR4Δlgt, mouse RAW264.7 cells were infected with either TIGR4 or TIGR4Δlgt for 12 h, and the production of nitrite and TNF-α was then examined (Figures 1B,C). TIGR4 treatment significantly upregulated levels of nitrite and TNF-α expression in a dose-dependent manner. By contrast, nitrite, and TNF-α production was not induced in cells infected with TIGR4Δlgt, indicating that TIGR4Δlgt failed to produce mature functional lipoproteins known to be responsible for stimulating inflammation. To examine whether the reduction in the inflammation-stimulating activity of TIGR4Δlgt was due to the loss of TLR2-stimulating activity, mouse peritoneal macrophages isolated from wild-type (WT), TLR2-deficient (ΔTLR2), or TLR4-deficient (ΔTLR4) mice were treated with TIGR4 or TIGR4Δlgt, and the level of TNF-α production was measured (Figure 1D). As expected, TIGR4 treatment induced TNF-α production in cells from WT and ΔTLR4 mice but not in cells from ΔTLR2 mice. However, TIGR4Δlgt treatment produced no or significantly lower levels of TNF-α in cells from all three groups, suggesting that pneumococcus-induced expression of functional TLR2 agonists is dependent on Lgt and that TIGR4Δlgt is likely defective in the production of TLR2 ligands.
Previous studies have suggested that LTA is another dominant TLR2 coactivator in Gram-positive bacteria (58, 59). Similar to lgt deletion, mutant bacteria lacking LTA elicit significantly reduced inflammatory activities in a variety of immune cells (60). Since pneumococci also express LTA, we questioned whether the lack of lgt in TIGR4 might affect the expression of LTA, thus affecting its ability to activate TLR2-mediated inflammation. Consequently, expression levels of LTA in the culture supernatants and cell walls of TIGR4 and TIGR4Δlgt were measured. As shown in Figure 1E, the expression level of LTA in the culture supernatant of TIGR4Δlgt was noticeably lower than that in the culture supernatant of TIGR4 based on Western blotting analysis using anti-phosphocholine antibody. LTA was further isolated from three different batches of pneumococcal cell wall via butanol extraction (Figure 1F). The average amount of LTA produced by TIGR4Δlgt was about 53% less than that produced by its parent strain. These results indicate that deletion of lgt in pneumococci may impair host inflammatory responses owing to the synthesis of non-functional lipoproteins and the downregulation of LTA biosynthesis.
Durable Colonization and Attenuated Pathogenicity of TIGR4Δlgt
To maximize immune responses, live attenuated vaccines should be able to effectively and durably colonize the mucosa without being invasive (19). To examine the role of lgt in colonization, mice were i.n. inoculated with either TIGR4 or TIGR4Δlgt [107 colony forming units (CFU)], and the number of bacteria colonizing the nasopharynx was measured in nasal washes at 24, 48, and 72 h post-infection (hpi) (Figures 2A–C). A significant reduction in the number of colonized bacteria was observed in TIGR4Δlgt-inoculated mice compared to that in TIGR4-inoculated mice. However, TIGR4Δlgt effectively colonized the nasopharynx for more than 3 days. No viable TIGR4Δlgt was detected at 14 days post-infection (dpi) (data not shown).
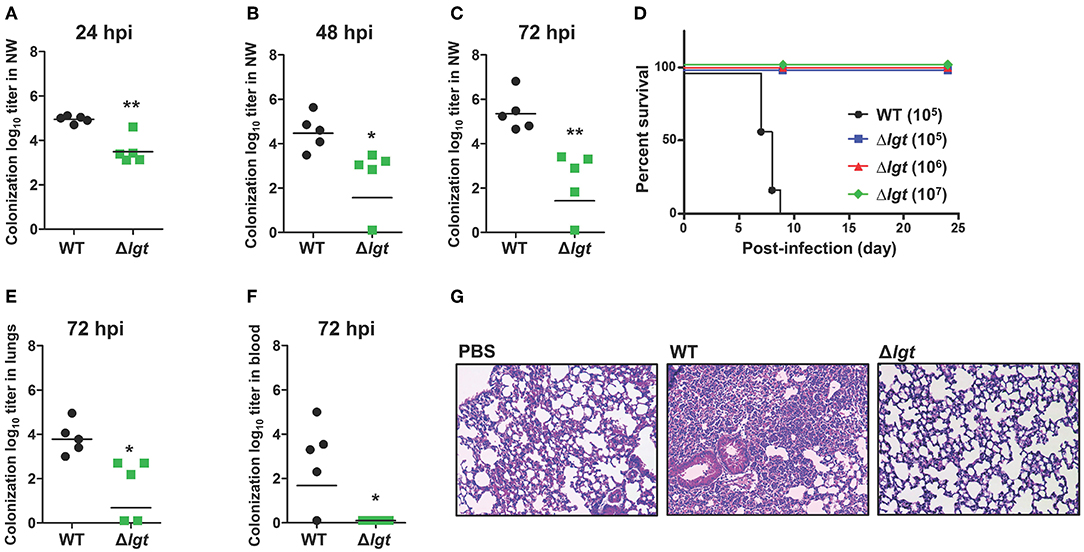
Figure 2. Durable Colonization of TIGR4Δlgt in the Nasopharynx. (A–C) Pneumococcal colonization of the nasopharynx in mice (n = 5 per group) inoculated intranasally (i.n.) with either wild-type (WT) or Δlgt TIGR4 (107 CFU) at 24 h (A), 48 h (B), and 72 h (C) after inoculation. (D) Survival of mice (n = 10 per group) inoculated i.n. with either WT (105 CFU) or Δlgt TIGR4 (105, 106, or 107 CFU). (E,F) Pneumococcal numbers in serially diluted lung tissue homogenates (E) and blood (F) from mice (n = 5 per group) inoculated i.n. with either WT or Δlgt TIGR4 (107 CFU) at 3 days after infection. (G) Hematoxylin & eosin (H&E) staining of lungs from mice inoculated with WT or Δlgt TIGR4 (107 CFU). *P < 0.05 and **P < 0.01 compared with the WT-infected mouse group.
To determine the invasiveness of TIGR4Δlgt, mice were i.n. inoculated with TIGR4 (105 CFU) or TIGR4Δlgt (105, 106, or 107 CFU), and mouse survival was recorded for 24 days. As shown in Figure 2D, all mice infected with TIGR4Δlgt survived for more than 24 days, even at infection levels of 107 CFU. However, all mice inoculated with 105 CFU of TIGR4 died by 9 dpi. No significant weight loss or noticeable disease symptoms were observed in mice inoculated with TIGR4Δlgt, suggesting a lack of systemic toxicity (data not shown). Next, the dissemination of bacteria into the lungs and blood was measured at 3 dpi with TIGR4 or TIGR4Δlgt (107 CFU). The bacterial load in the lungs was significantly reduced in TIGR4Δlgt-inoculated mice compared to that in TIGR4-inoculated mice (Figure 2E). No dissemination of TIGR4Δlgt into the bloodstream was observed for up to 14 days (Figure 2F and data not shown). Consistent with findings from survival and microbiological analysis, lungs from mice infected with TIGR4 showed marked pathological changes in inflammation, including enhanced neutrophil infiltration, loss of alveolar architecture, and red blood cell leakage (Figure 2G). However, although a modest number of TIGR4Δlgt was disseminated into the lungs, no significant pathological change was found in the lungs of TIGR4Δlgt-infected mice (Figure 2G). These data suggest that TIGR4 was successfully attenuated by deleting lgt but that TIGR4Δlgt can still colonize the nasopharynx.
Protective Humoral Immune Responses Induced by TIGR4Δlgt Vaccination
We next evaluated the immunogenicity of live TIGR4Δlgt (live Δlgt) and compared it with that of inactivated TIGR4Δlgt (killed Δlgt). Mice were i.n. immunized with either live or killed Δlgt twice at 14-day intervals (days 0 and 14). Pneumococcal-specific immunoglobulin levels in the serum and bronchoalveolar lavage fluid (BALF) were then measured at 10 days after the second immunization. As shown in Figures 3A,B, both live and killed Δlgt vaccines induced significantly higher serum levels of pneumococcal-specific IgM (Sp-specific IgM) and Sp-specific IgG than inoculation with PBS (control group). Moreover, Sp-specific IgM levels in the sera of live Δlgt-immunized mice were significantly higher than those in the sera of mice immunized with killed Δlgt. However, no differences in Sp-specific IgG levels were found between the live and killed Δlgt immunization groups. In addition, significant induction of Sp-specific IgA was found in BALF from both the live and killed Δlgt-immunized groups (Figure 3C), indicating that both live and killed Δlgt vaccines effectively induced mucosal immune responses.
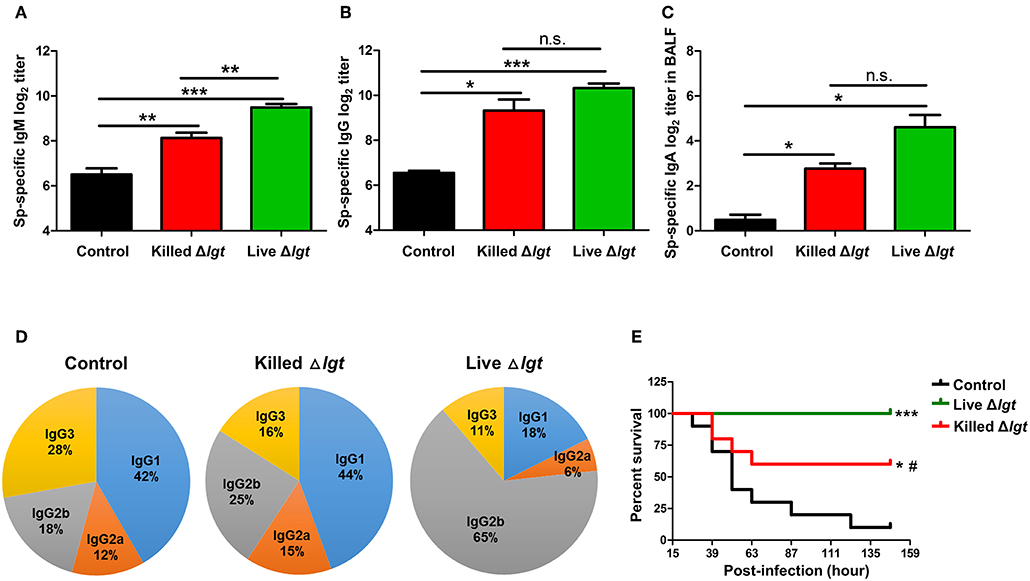
Figure 3. Humoral and Mucosal Protective Immune Responses Induced by Live Δlgt Vaccination. (A–D) Levels of Sp-specific serum IgM (A), serum IgG (B), and BALF IgA (C) and ratios of serum IgG subclasses (D) determined 10 days after second immunization in mice (n = 5 per group) i.n. immunized twice with killed Δlgt or live Δlgt at 14-day intervals. Data in (A–C) indicate mean values ± S.D. n.s.; not significant, *P < 0.05, **P < 0.01, and ***P < 0.001 compared to PBS-inoculated controls. (E) Survival of mice (n = 5 per group) i.n. immunized twice with killed Δlgt or live Δlgt at 14-day intervals, followed by intraperitoneal (i.p.) challenge with 107 CFU of wild-type TIGR4. *P < 0.05 and ***P < 0.001 compared to control groups immunized with PBS. #P < 0.05 compared to the live Δlgt-vaccinated group.
Distinct IgG subclasses have different efficacies in terms of their functions, such as serum bactericidal activity and opsonophagocytosis. Therefore, IgG subclass distributions may contribute to the protective effects of vaccination. Despite the fact that similar levels of serum IgG were elicited by live and killed Δlgt, IgG subclass analysis showed markedly different IgG subclass patterns between the two immunization groups (Figure 3D). Specifically, IgG1 (44%) was predominant, followed by IgG2b (25%), IgG3 (16%), and IgG2a (15%), in the sera of mice immunized with killed Δlgt, whereas a strong shift to an IgG2b (65%) antibody response was observed in mice immunized with live Δlgt.
Next, we determined the immunological efficacy of the live vs. killed Δlgt vaccines by measuring their protective effects against detrimental challenge with TIGR4. Mice immunized with either live or killed Δlgt were i.n. challenged with WT TIGR4 (107 CFU), and mouse survival was recorded 10 days after the second immunization. As shown in Figure 3E, 90% of control mice (PBS-immunized) died by 5 dpi, whereas 60% of mice immunized with killed Δlgt and 100% of mice immunized with live Δlgt survived for more than 6 dpi. Taken together, these findings demonstrate that live Δlgt vaccination induces high levels of IgM and IgG2-prone humoral immune responses, providing protection against detrimental challenge with its parent strain, TIGR4, in mice.
Serotype-Independent Protection Conferred by TIGR4Δlgt Vaccination
To overcome the major limitations of currently available pneumococcal vaccines, vaccines should ideally be designed to provide serotype-independent, broad-spectrum protection against heterologous pneumococcal serotypes. Therefore, we assessed the cross-reactivity of the antibodies induced by TIGR4Δlgt to different pneumococcal serotypes. As shown in Figures 4A–F, the IgM and IgG antibodies induced by TIGR4Δlgt showed capsular serotype-independent reactivities to heterologous pneumococcal serotypes (STs), including ST2 (D39), ST3 (wu2), ST6B, ST9V, ST19F, and ST23F. In addition, we investigated production of the antibodies against conserved pneumococcal surface antigens such as LytA, an autolysin, and pneumococcal surface protein PspA, a component of transport system. TIGR4Δlgt immunization produced antibodies against LytA and PspA as well as antibodies against purified cell wall PS (Figures 4G,H).
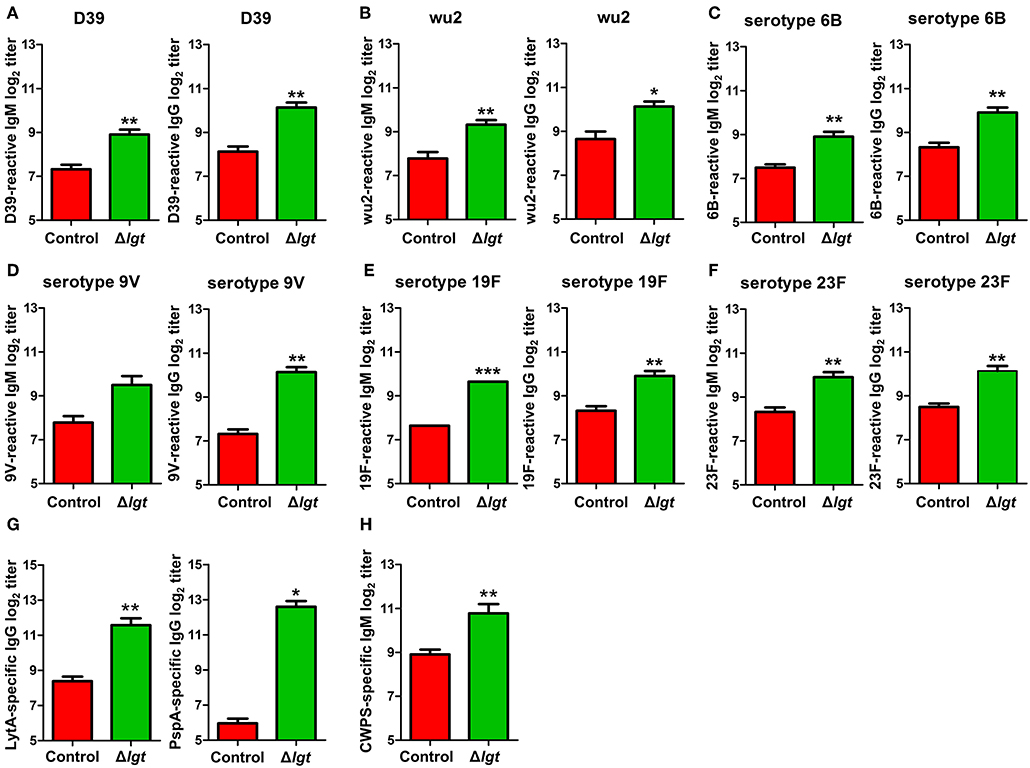
Figure 4. Cross-reactive antibody production following immunization with TIGR4Δlgt. Serum levels of IgM and IgG reactive to serotypes 2 (D39; A), 3 (wu2; B), 6B (C), 9V (D), 19F (E), and 23F (F) and serum levels of LytA-, PspA-, and cell wall PS-specific IgG (G,H) were determined at 10 days after the second immunization in mice (n = 5 per group) i.n. immunized twice with Δlgt (106 CFU) at 14-day intervals. *P < 0.05, **P < 0.01 and ***P < 0.001 compared with control groups immunized with PBS.
Next, we investigated whether TIGR4Δlgt vaccination provided cross-protection against different serotypes. Mice were i.n. immunized with TIGR4Δlgt twice at 14-day intervals (day 0 and day 14) and challenged with a lethal dose of wu2 (Figure 5A) or D39 (Figure 5B) at 10 days after the second immunization. All mice challenged with wu2 without immunization (control) died by 7 dpi (0% survival). However, immunization with TIGR4Δlgt resulted in complete protection against wu2 challenge (100% survival) (Figure 5A). Similarly, D39 challenge resulted in 100% mortality for the unimmunized group by 7 dpi, while 75% of mice immunized with TIGR4Δlgt survived for more than 10 dpi, indicating serotype-independent protection offered by TIGR4Δlgt vaccination (Figure 5B). Bacterial loads in the nasopharynx, lungs, and blood of mice were also significantly reduced by TIGR4Δlgt vaccination following detrimental challenge with either wu2 (Figures 5C–E) or D39 (Figures 5F–H).
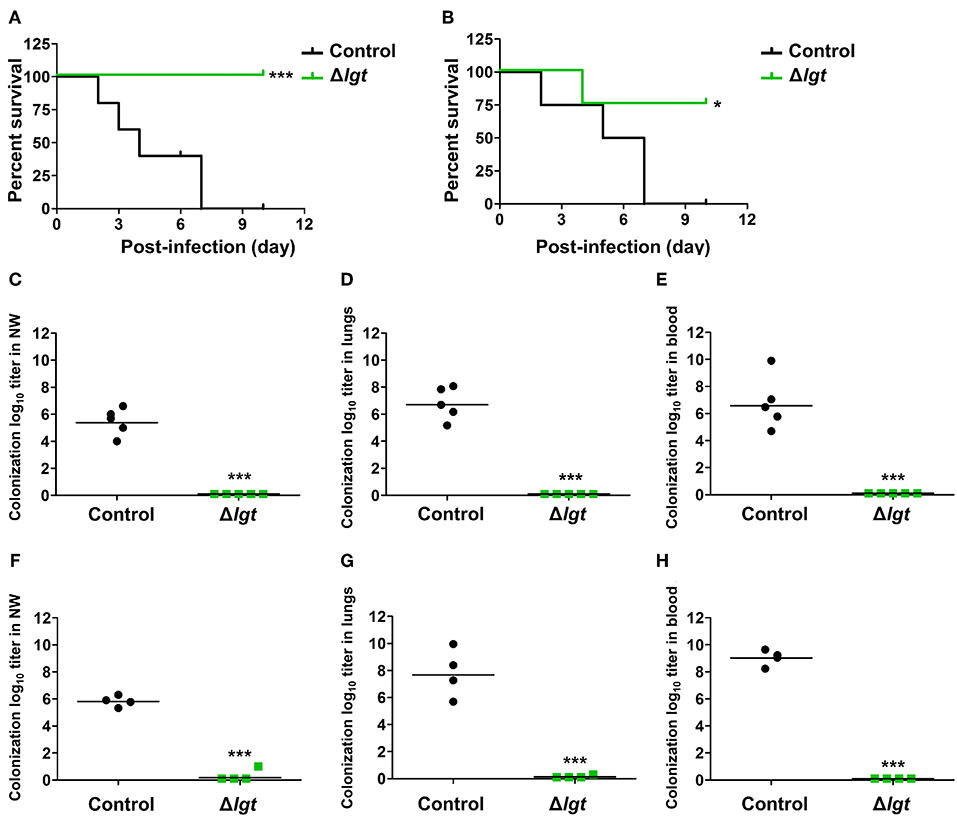
Figure 5. Serotype-independent protection conferred by TIGR4Δlgt vaccination. (A,B) Survival of mice (n = 5) i.n. immunized with Δlgt (106 CFU) twice at 14-day intervals, followed by i.n. challenge with 108 CFU of wu2 (A) or D39 (B) strains. (C–H) Pneumococcal numbers counted within serially diluted nasal washes (C,F), lung tissue homogenates (D,G), and blood (E,H) from Δlgt (106 CFU)-immunized mice challenged with wu2 (C–E) or D39 (F–H). *P < 0.05 and ***P < 0.001 compared to the control group immunized with PBS.
Discussion
After adding PCVs to the immunization schedule for the pediatric population, the incidence of invasive pneumococcal diseases in both vaccinated children and unvaccinated individuals of all ages has been significantly reduced (9, 10). However, non-vaccine serotypes and new serotypes have emerged as major causes of pneumococcal diseases (11, 12, 61). To overcome these limitations, the development of innovative vaccines including protein-based vaccines, inactivated whole-cell-based vaccines, and live attenuated vaccines capable of covering all or most pneumococcal serotypes is actively being pursued (16, 19, 20, 62). Among these vaccines, live attenuated vaccines offer several advantages as cross-protective, broad-spectrum pneumococcal vaccines, such as the effective induction of both memory and cell-mediated immune responses against common and important pneumococcal antigens (21). Despite these benefits, a degree of unpredictability raises safety and stability concerns regarding the widespread adaptation of live attenuated vaccines (63). For example, Bacillus Calmette-Guerin (BCG) vaccination for tuberculosis can result in severe local inflammation or disseminated infection (64). In the current study, we developed a live attenuated vaccine for pneumococcal infections by deleting the lgt gene in the encapsulated pneumococcal strain TIGR4 (TIGR4Δlgt), resulting in significant attenuation of invasiveness and inflammation-activating abilities. However, TIGR4Δlgt was able to colonize the mouse nasopharynx long enough to elicit mucosal antibody responses and IgG2b-dominant, Th1-biased systemic immune responses that were cross-reactive to heterologous pneumococcal serotypes. Moreover, the live TIGR4Δlgt vaccine provided better protection than the killed whole-cell-based vaccine against subsequent detrimental challenge with the parental WT (TIGR4) strain.
Lipoproteins comprise the largest group of bacterial surface proteins and contribute significantly to bacterial adaptation to environmental changes, uptake of nutrients, and adherence to host membranes during infection (24). The importance of bacterial lipoproteins in TLR2-mediated immune recognition and pro-inflammatory responses has been extensively investigated using lgt-deficient pathogens (27, 65, 66). Both the present and previous studies showed that lgt-deficient pneumococci cannot activate TLR2-dependent cellular responses in macrophages. Although TLR2 responses are required for stimulating the innate and adaptive immune responses (e.g., Th1, Th2, Th17, Treg) of the host (67–69), our data showed that live Δlgt vaccination induces IgG2-dominant humoral responses, which are prone to Th1-biased responses in mice. Unlike in human, in which IgG1 is more effective in opsonization and FcγR-mediated pneumococcal phagocytosis, IgG2 functions as a highly effective opsonin and more significantly involved in opsonophagocytosis and serum bactericidal activities than IgG1 in mouse (70, 71). IgG2, both IgG2a and 2b, is a surrogate marker for Th1 response and highly activate complements and opsonophagocytosis than IgG1 (70, 72). Such a significant change in IgG subclass pattern may contribute to the enhanced protection induced by live Δlgt vaccination. In addition, a significantly higher level of IgM, which is a major Ig class responsible for complement activation, and IgM-mediated complement activation may also contribute to the enhanced protective effects observed in live Δlgt vaccination. Previous studies have also indicated that staphylococcal and pneumococcal Δlgt strains elicit significant upregulation of proinflammatory cytokines (IL-8, IL6, and TNF-α) in human leukocytes, though it is lower than that of WT, which may be mediated by pathogen-associated molecular patterns (PAMPs) other than lipoproteins, including peptidoglycan, pneumolysin, and LTA (40, 58, 73). Consequently, we hypothesized that live TIGR4Δlgt may still have the ability to stimulate adaptive immune responses via lipoprotein-TLR2-independent mechanisms. In addition, IFN-γ production is known to regulate pneumococcal infections and T cell-mediated immunological memory responses (74, 75). Similar to the results of a previous study (37), our observations showed that live TIGR4Δlgt vaccination elicited significantly higher levels of IFN-γ production by splenocytes than killed TIGR4Δlgt vaccination (data not shown), suggesting that the superior protection against pneumococcus conferred by live TIGR4Δlgt vaccination may be due to the IgG2b/IgG2a-predominant Th1 response via the IFN-γ pathway. However, further insight into how lipoprotein-deficient pneumococci modulate Th1-mediated host defenses is warranted.
Pneumococcal colonization leads to naturally acquired mucosal events that induce both Th17 cell-mediated and humoral adaptive immune responses (76, 77). Thus, prolonged colonization of the nasopharynx may be another critical factor in the development of effective pneumococcal live attenuated vaccines. Many attenuated pneumococcal strains lacking major virulence genes, such as lytA, pspA, and pspC, have failed to establish successful nasopharyngeal colonization (78, 79). However, while the TIGR4Δlgt developed in this study showed significantly reduced colonization of the mouse nasopharynx compared to that of its parent strain, it still colonized long enough to induce mucosal and systemic humoral antibody responses against various lethal pneumococcal strains. Despite such prolonged colonization, TIGR4Δlgt did not result in significant inflammation or dissemination into the lungs or bloodstream, even at a multiplicity of infection >1,000 times the median lethal dose of the parental strain. Such localized colonization indicates the highly successful attenuation of invasiveness, thus ensuring the great potential of TIGR4Δlgt as a vaccine candidate.
Since airway is a major route of pneumococcal infection, alveolar macrophages play a role on defense against pneumococcus. Although the level of IgA, which is a major Ig class in the airway, was not significantly different in BALF between killed and live TIGR4Δlgt vaccination, live TIGR4Δlgt immunization more effectively removed pneumococcus from the alveolar airway. These findings may suggest that alveolar macrophage-mediated phagocytosis may play roles on enhanced protection observed in live TIGR4Δlgt immunization. Although TIGR4Δlgt failed to produce mature lipoproteins functioning as adhesins, our microarray data indicated that most cell surface-anchoring proteins (e.g., choline-binding proteins) were expressed at levels similar to that in the parental TIGR4 strain, likely facilitating sufficient colonization. Thus, prolonged colonization of TIGR4Δlgt may elicit cross-reactive, pneumococcal-specific IgG and IgM production and, therefore, more efficient Th1-biased antibody responses, providing improved protective immunity against multiple serotypes.
In conclusion, this study demonstrated that the deletion of a single pneumococcal “master” gene, lgt, results in the creation of a live attenuated vaccine with attenuated virulence and invasive ability. Furthermore, this study showed that TIGR4Δlgt has the potential to induce broad-spectrum, serotype-independent, protective immunity against life-threatening pneumococcal infections. Since conventional serotype-based vaccines have limitations, including a lack of protection against non-vaccine serotypes, the need for multiple doses and adjuvants, and high cost, the findings of this study may be useful in the development of inexpensive, but highly efficacious, vaccines against pneumococcal infections. In a broader sense, the modulation of major metabolic regulators represents an emerging strategy in the design of immunologic agents.
Ethics Statement
All animal experiments conducted in this study were approved by the Committee on the Use and Care of Animals at the Korea Atomic Energy Research Institute (KAERI) and performed according to accepted veterinary standards.
Author Contributions
JL and HS conceived the study. A-YJ, KA, and HS designed the experiments. A-YJ, KA, YZ, HJ-J, JZ, and HS performed the experiments. A-YJ, KA, and HS analyzed and/or interpreted the data and contributed to discussion of the results, followed by writing and reviewing the manuscript. SH, HG, SL, JS, JL, and HS provided critical comments and contributed to discussion of the results, followed by writing and reviewing the manuscript.
Funding
This work was supported by the Nuclear R&D Program of the Ministry of Science and ICT (SL), National Research Foundation of Korea under Grants NRF-2017M2A2A6A02020925 and 2018K2A2A06023828 to HS and 2015R1D1A1A01059338 to JL, and the Ministry of Food and Drug Administration under Grant 18172MFDS253 to JS.
Conflict of Interest Statement
The authors declare that the research was conducted in the absence of any commercial or financial relationships that could be construed as a potential conflict of interest.
Supplementary Material
The Supplementary Material for this article can be found online at: https://www.frontiersin.org/articles/10.3389/fimmu.2019.01212/full#supplementary-material
References
1. Morrill HJ, Caffrey AR, Noh E, LaPlante KL. Epidemiology of pneumococcal disease in a national cohort of older adults. Infect Dis Ther. (2014) 3:19–33. doi: 10.1007/s40121-014-0025-y
2. Henriques-Normark B, Tuomanen EI. The pneumococcus: epidemiology, microbiology, and pathogenesis. Cold Spring Harb Perspect Med. (2013) 3:a010215. doi: 10.1101/cshperspect.a010215
3. Savulescu C, Krizova P, Lepoutre A, Mereckiene J, Vestrheim DF, Ciruela P, et al. Effect of high-valency pneumococcal conjugate vaccines on invasive pneumococcal disease in children in SpIDnet countries: an observational multicentre study. Lancet Respir Med. (2017) 5:648–56. doi: 10.1016/S2213-2600(17)30110-8
4. Xu X, Wang H, Liu Y, Wang Y, Zeng L, Wu K, et al. Mucosal immunization with the live attenuated vaccine SPY1 induces humoral and Th2-Th17-regulatory T cell cellular immunity and protects against pneumococcal infection. Infect Immun. (2015) 83:90–100. doi: 10.1128/IAI.02334-14
5. Trzcinski K, Thompson CM, Srivastava A, Basset A, Malley R, Lipsitch M. Protection against nasopharyngeal colonization by Streptococcus pneumoniae is mediated by antigen-specific CD4+ T cells. Infect Immun. (2008) 76:2678–84. doi: 10.1128/IAI.00141-08
6. Wuorimaa T, Kayhty H. Current state of pneumococcal vaccines. Scand J Immunol. (2002) 56:111–29. doi: 10.1046/j.1365-3083.2002.01124.x
7. Vila-Corcoles A, Ochoa-Gondar O, Hospital I, Ansa X, Vilanova A, Rodriguez T, et al. Protective effects of the 23-valent pneumococcal polysaccharide vaccine in the elderly population: the EVAN-65 study. Clin Infect Dis. (2006) 43:860–8. doi: 10.1086/507340
8. Esposito S, Principi N. Impacts of the 13-valent pneumococcal conjugate vaccine in children. J Immunol Res. (2015) 2015:591580. doi: 10.1155/2015/591580
9. Corcoran M, Vickers I, Mereckiene J, Murchan S, Cotter S, Fitzgerald M, et al. The epidemiology of invasive pneumococcal disease in older adults in the post-PCV era. Has there been a herd effect? Epidemiol Infect. (2017) 145:2390–9. doi: 10.1017/S0950268817001194
10. Conklin L, Loo JD, Kirk J, Fleming-Dutra KE, Deloria Knoll M, Park DE, et al. Systematic review of the effect of pneumococcal conjugate vaccine dosing schedules on vaccine-type invasive pneumococcal disease among young children. Pediatr Infect Dis J. (2014) 33 (Suppl. 2):S109–18. doi: 10.1097/INF.0000000000000078
11. Hausdorff WP, Van Dyke MK, Van Effelterre T. Serotype replacement after pneumococcal vaccination. Lancet. (2012) 379:1387–8; author reply 8–9. doi: 10.1016/S0140-6736(12)60589-3
12. Spratt BG, Greenwood BM. Prevention of pneumococcal disease by vaccination: does serotype replacement matter? Lancet. (2000) 356:1210–1. doi: 10.1016/S0140-6736(00)02779-3
13. Gladstone RA, Devine V, Jones J, Cleary D, Jefferies JM, Bentley SD, et al. Pre-vaccine serotype composition within a lineage signposts its serotype replacement - a carriage study over 7 years following pneumococcal conjugate vaccine use in the UK. Microb Genom. (2017) 3:e000119. doi: 10.1099/mgen.0.000119
14. Gladstone RA, Jefferies JM, Tocheva AS, Beard KR, Garley D, Chong WW, et al. Five winters of pneumococcal serotype replacement in UK carriage following PCV introduction. Vaccine. (2015) 33:2015–21. doi: 10.1016/j.vaccine.2015.03.012
15. Lynch JM, Briles DE, Metzger DW. Increased protection against pneumococcal disease by mucosal administration of conjugate vaccine plus interleukin-12. Infect Immun. (2003) 71:4780–8. doi: 10.1128/IAI.71.8.4780-4788.2003
16. Moffitt KL, Malley R, Lu YJ. Identification of protective pneumococcal T(H)17 antigens from the soluble fraction of a killed whole cell vaccine. PLoS ONE. (2012) 7:e43445. doi: 10.1371/journal.pone.0043445
17. Moffitt KL, Yadav P, Weinberger DM, Anderson PW, Malley R. Broad antibody and T cell reactivity induced by a pneumococcal whole-cell vaccine. Vaccine. (2012) 30:4316–22. doi: 10.1016/j.vaccine.2012.01.034
18. Chien YW, Klugman KP, Morens DM. Efficacy of whole-cell killed bacterial vaccines in preventing pneumonia and death during the 1918 influenza pandemic. J Infect Dis. (2010) 202:1639–48. doi: 10.1086/657144
19. Rosch JW, Iverson AR, Humann J, Mann B, Gao G, Vogel P, et al. A live-attenuated pneumococcal vaccine elicits CD4+ T-cell dependent class switching and provides serotype independent protection against acute otitis media. EMBO Mol Med. (2014) 6:141–54. doi: 10.1002/emmm.201202150
20. Moffitt KL, Malley R. Next generation pneumococcal vaccines. Curr Opin Immunol. (2011) 23:407–13. doi: 10.1016/j.coi.2011.04.002
21. Rosch JW. Promises and pitfalls of live attenuated pneumococcal vaccines. Hum Vaccin Immunother. (2014) 10:3000–3. doi: 10.4161/21645515.2014.970496
22. Roche AM, King SJ, Weiser JN. Live attenuated Streptococcus pneumoniae strains induce serotype-independent mucosal and systemic protection in mice. Infect Immun. (2007) 75:2469–75. doi: 10.1128/IAI.01972-06
23. Kim EH, Choi SY, Kwon MK, Tran TD, Park SS, Lee KJ, et al. Streptococcus pneumoniae pep27 mutant as a live vaccine for serotype-independent protection in mice. Vaccine. (2012) 30:2008–19. doi: 10.1016/j.vaccine.2011.11.073
24. Kovacs-Simon A, Titball RW, Michell SL. Lipoproteins of bacterial pathogens. Infect Immun. (2011) 79:548–61. doi: 10.1128/IAI.00682-10
25. Kohler S, Voss F, Gomez Mejia A, Brown JS, Hammerschmidt S. Pneumococcal lipoproteins involved in bacterial fitness, virulence, and immune evasion. FEBS Lett. (2016) 590:3820–39. doi: 10.1002/1873-3468.12352
26. Torti SV, Park JT. Lipoprotein of gram-negative bacteria is essential for growth and division. Nature. (1976) 263:323–6. doi: 10.1038/263323a0
27. Snapper CM, Rosas FR, Jin L, Wortham C, Kehry MR, Mond JJ. Bacterial lipoproteins may substitute for cytokines in the humoral immune response to T cell-independent type II antigens. J Immunol. (1995) 155:5582–9.
28. Hashimoto M, Tawaratsumida K, Kariya H, Kiyohara A, Suda Y, Krikae F, et al. Not lipoteichoic acid but lipoproteins appear to be the dominant immunobiologically active compounds in Staphylococcus aureus. J Immunol. (2006) 177:3162–9. doi: 10.4049/jimmunol.177.5.3162
29. Kretschmer D, Hanzelmann D, Peschel A. Lipoprotein immunoproteomics question the potential of Staphylococcus aureus TLR2 agonists as vaccine antigens. Proteomics. (2016) 16:2603–4. doi: 10.1002/pmic.201600351
30. Mao G, Zhao Y, Kang X, Li Z, Zhang Y, Wang X, et al. Crystal structure of E. coli lipoprotein diacylglyceryl transferase. Nat Commun. (2016) 7:10198. doi: 10.1038/ncomms10198
31. Zuckert WR. Secretion of bacterial lipoproteins: through the cytoplasmic membrane, the periplasm and beyond. Biochim Biophys Acta. (2014) 1843:1509–16. doi: 10.1016/j.bbamcr.2014.04.022
32. Baumgartner M, Karst U, Gerstel B, Loessner M, Wehland J, Jansch L. Inactivation of Lgt allows systematic characterization of lipoproteins from Listeria monocytogenes. J Bacteriol. (2007) 189:313–24. doi: 10.1128/JB.00976-06
33. Arimoto T, Igarashi T. Role of prolipoprotein diacylglyceryl transferase (Lgt) and lipoprotein-specific signal peptidase II (LspA) in localization and physiological function of lipoprotein MsmE in Streptococcus mutans. Oral Microbiol Immunol. (2008) 23:515–9. doi: 10.1111/j.1399-302X.2008.00455.x
34. Bray BA, Sutcliffe IC, Harrington DJ. Impact of lgt mutation on lipoprotein biosynthesis and in vitro phenotypes of Streptococcus agalactiae. Microbiology. (2009) 155 (Pt 5):1451–8. doi: 10.1099/mic.0.025213-0
35. Wichgers Schreur PJ, Rebel JM, Smits MA, van Putten JP, Smith HE. Lgt processing is an essential step in Streptococcus suis lipoprotein mediated innate immune activation. PLoS ONE. (2011) 6:e22299. doi: 10.1371/journal.pone.0022299
36. Pailler J, Aucher W, Pires M, Buddelmeijer N. Phosphatidylglycerol::prolipoprotein diacylglyceryl transferase (Lgt) of Escherichia coli has seven transmembrane segments, and its essential residues are embedded in the membrane. J Bacteriol. (2012) 194:2142–51. doi: 10.1128/JB.06641-11
37. Tomlinson G, Chimalapati S, Pollard T, Lapp T, Cohen J, Camberlein E, et al. TLR-mediated inflammatory responses to Streptococcus pneumoniae are highly dependent on surface expression of bacterial lipoproteins. J Immunol. (2014) 193:3736–45. doi: 10.4049/jimmunol.1401413
38. Seo HS, Xiong YQ, Mitchell J, Seepersaud R, Bayer AS, Sullam PM. Bacteriophage lysin mediates the binding of Streptococcus mitis to human platelets through interaction with fibrinogen. PLoS Pathog. (2010) 6:e1001047. doi: 10.1371/journal.ppat.1001047
39. Bricker AL, Camilli A. Transformation of a type 4 encapsulated strain of Streptococcus pneumoniae. FEMS Microbiol Lett. (1999) 172:131–5. doi: 10.1111/j.1574-6968.1999.tb13460.x
40. Seo HS, Cartee RT, Pritchard DG, Nahm MH. A new model of pneumococcal lipoteichoic acid structure resolves biochemical, biosynthetic, and serologic inconsistencies of the current model. J Bacteriol. (2008) 190:2379–87. doi: 10.1128/JB.01795-07
41. Brown LR, Gunnell SM, Cassella AN, Keller LE, Scherkenbach LA, Mann B, et al. AdcAII of Streptococcus pneumoniae affects pneumococcal invasiveness. PLoS ONE. (2016) 11:e0146785. doi: 10.1371/journal.pone.0146785
42. Lonnstedt I, Britton T. Hierarchical Bayes models for cDNA microarray gene expression. Biostatistics. (2005) 6:279–91. doi: 10.1093/biostatistics/kxi009
43. Bartual SG, Alcorlo M, Martinez-Caballero S, Molina R, Hermoso JA. Three-dimensional structures of lipoproteins from Streptococcus pneumoniae and Staphylococcus aureus. Int J Med Microbiol. (2018) 308:692–704. doi: 10.1016/j.ijmm.2017.10.003
44. Ware D, Jiang Y, Lin W, Swiatlo E. Involvement of potD in Streptococcus pneumoniae polyamine transport and pathogenesis. Infect Immun. (2006) 74:352–61. doi: 10.1128/IAI.74.1.352-361.2006
45. Lebon A, Verkaik NJ, Labout JA, de Vogel CP, Hooijkaas H, Verbrugh HA, et al. Natural antibodies against several pneumococcal virulence proteins in children during the pre-pneumococcal-vaccine era: the generation R study. Infect Immun. (2011) 79:1680–7. doi: 10.1128/IAI.01379-10
46. Mahdi LK, Wang H, Van der Hoek MB, Paton JC, Ogunniyi AD. Identification of a novel pneumococcal vaccine antigen preferentially expressed during meningitis in mice. J Clin Invest. (2012) 122:2208–20. doi: 10.1172/JCI45850
47. Cao J, Chen D, Xu W, Chen T, Xu S, Luo J, et al. Enhanced protection against pneumococcal infection elicited by immunization with the combination of PspA, PspC, and ClpP. Vaccine. (2007) 25:4996–5005. doi: 10.1016/j.vaccine.2007.04.069
48. Obert C, Sublett J, Kaushal D, Hinojosa E, Barton T, Tuomanen EI, et al. Identification of a candidate Streptococcus pneumoniae core genome and regions of diversity correlated with invasive pneumococcal disease. Infect Immun. (2006) 74:4766–77. doi: 10.1128/IAI.00316-06
49. Ling E, Feldman G, Portnoi M, Dagan R, Overweg K, Mulholland F, et al. Glycolytic enzymes associated with the cell surface of Streptococcus pneumoniae are antigenic in humans and elicit protective immune responses in the mouse. Clin Exp Immunol. (2004) 138:290–8. doi: 10.1111/j.1365-2249.2004.02628.x
50. Pracht D, Elm C, Gerber J, Bergmann S, Rohde M, Seiler M, et al. PavA of Streptococcus pneumoniae modulates adherence, invasion, and meningeal inflammation. Infect Immun. (2005) 73:2680–9. doi: 10.1128/IAI.73.5.2680-2689.2005
51. Cole JN, Henningham A, Gillen CM, Ramachandran V, Walker MJ. Human pathogenic streptococcal proteomics and vaccine development. Proteomics Clin Appl. (2008) 2:387–410. doi: 10.1002/prca.200780048
52. Bergmann S, Rohde M, Hammerschmidt S. Glyceraldehyde-3-phosphate dehydrogenase of Streptococcus pneumoniae is a surface-displayed plasminogen-binding protein. Infect Immun. (2004) 72:2416–9. doi: 10.1128/IAI.72.4.2416-2419.2004
53. Jedrzejas MJ. Pneumococcal virulence factors: structure and function. Microbiol Mol Biol Rev. (2001) 65:187–207. doi: 10.1128/MMBR.65.2.187-207.2001
54. Li Y, Hill A, Beitelshees M, Shao S, Lovell JF, Davidson BA, et al. Directed vaccination against pneumococcal disease. Proc Natl Acad Sci USA. (2016) 113:6898–903. doi: 10.1073/pnas.1603007113
55. Pichichero ME, Kaur R, Casey JR, Xu Q, Almudevar A, Ochs M. Antibody response to Streptococcus pneumoniae proteins PhtD, LytB, PcpA, PhtE and Ply after nasopharyngeal colonization and acute otitis media in children. Hum Vaccin Immunother. (2012) 8:799–805. doi: 10.4161/hv.19820
56. Zhang Y, Masi AW, Barniak V, Mountzouros K, Hostetter MK, Green BA. Recombinant PhpA protein, a unique histidine motif-containing protein from Streptococcus pneumoniae, protects mice against intranasal pneumococcal challenge. Infect Immun. (2001) 69:3827–36. doi: 10.1128/IAI.69.6.3827-3836.2001
57. Janapatla RP, Chen CL, Hsu MH, Liao WT, Chiu CH. Immunization with pneumococcal neuraminidases NanA, NanB, and NanC to generate neutralizing antibodies and to increase survival in mice. J Med Microbiol. (2018) 67:709–723. doi: 10.1099/jmm.0.000724
58. Seo HS, Michalek SM, Nahm MH. Lipoteichoic acid is important in innate immune responses to gram-positive bacteria. Infect Immun. (2008) 76:206–13. doi: 10.1128/IAI.01140-07
59. Grundling A, Schneewind O. Synthesis of glycerol phosphate lipoteichoic acid in Staphylococcus aureus. Proc Natl Acad Sci USA. (2007) 104:8478–83. doi: 10.1073/pnas.0701821104
60. Baik JE, Ryu YH, Han JY, Im J, Kum KY, Yun CH, et al. Lipoteichoic acid partially contributes to the inflammatory responses to Enterococcus faecalis. J Endod. (2008) 34:975–82. doi: 10.1016/j.joen.2008.05.005
61. Geno KA, Gilbert GL, Song JY, Skovsted IC, Klugman KP, Jones C, et al. Pneumococcal capsules and their types: past, present, and future. Clin Microbiol Rev. (2015) 28:871–99. doi: 10.1128/CMR.00024-15
62. Akeda Y, Ikuta K. Next generation pneumococcal vaccine. Nihon Naika Gakkai Zasshi. (2015) 104:2351–6. doi: 10.2169/naika.104.2351
63. Minor PD. Live attenuated vaccines: historical successes and current challenges. Virology. (2015) 479–480:379–92. doi: 10.1016/j.virol.2015.03.032
64. Abu-Arja RF, Gonzalez BE, Jacobs MR, Cabral L, Egler R, Auletta J, et al. Disseminated bacillus calmette-guerin (BCG) infection following allogeneic hematopoietic stem cell transplant in a patient with bare lymphocyte syndrome type II. Transpl Infect Dis. (2014) 16:830–7. doi: 10.1111/tid.12263
65. Hashimoto M, Tawaratsumida K, Kariya H, Aoyama K, Tamura T, Suda Y. Lipoprotein is a predominant Toll-like receptor 2 ligand in Staphylococcus aureus cell wall components. Int Immunol. (2005) 18:355–62. doi: 10.1093/intimm/dxh374
66. Takeuchi O, Sato S, Horiuchi T, Hoshino K, Takeda K, Dong Z, et al. Cutting edge: role of Toll-like receptor 1 in mediating immune response to microbial lipoproteins. J Immunol. (2002) 169:10–4. doi: 10.4049/jimmunol.169.1.10
67. Barra NG, Gillgrass A, Ashkar AA. Effective control of viral infections by the adaptive immune system requires assistance from innate immunity. Expert Rev Vaccines. (2010) 9:1143–7. doi: 10.1586/erv.10.119
68. Iwasaki A, Medzhitov R. Control of adaptive immunity by the innate immune system. Nat Immunol. (2015) 16:343–53. doi: 10.1038/ni.3123
69. Wang J, Roderiquez G, Norcross MA. Control of adaptive immune responses by Staphylococcus aureus through IL-10, PD-L1, and TLR2. Sci Rep. (2012) 2:606. doi: 10.1038/srep00606
70. Michaelsen TE, Kolberg J, Aase A, Herstad TK, Hoiby EA. The four mouse IgG isotypes differ extensively in bactericidal and opsonophagocytic activity when reacting with the P1.16 epitope on the outer membrane PorA protein of Neisseria meningitidis. Scand J Immunol. (2004) 59:34–9. doi: 10.1111/j.0300-9475.2004.01362.x
71. Nimmerjahn F, Bruhns P, Horiuchi K, Ravetch JV. FcgammaRIV: a novel FcR with distinct IgG subclass specificity. Immunity. (2005) 23:41–51. doi: 10.1016/j.immuni.2005.05.010
72. Collins AM. IgG subclass co-expression brings harmony to the quartet model of murine IgG function. Immunol Cell Biol. (2016) 94:949–54. doi: 10.1038/icb.2016.65
73. Koppe U, Suttorp N, Opitz B. Recognition of Streptococcus pneumoniae by the innate immune system. Cell Microbiol. (2012) 14:460–6. doi: 10.1111/j.1462-5822.2011.01746.x
74. Rijneveld AW, Lauw FN, Schultz MJ, Florquin S, Te Velde AA, Speelman P, et al. The role of interferon-gamma in murine pneumococcal pneumonia. J Infect Dis. (2002) 185:91–7. doi: 10.1086/338122
75. Mureithi MW, Finn A, Ota MO, Zhang Q, Davenport V, Mitchell TJ, et al. T cell memory responseto pneumococcal protein antigens in an area of high pneumococcal carriage and disease. J Infect Dis. (2009) 200:783–93. doi: 10.1086/605023
76. Cohen JM, Khandavilli S, Camberlein E, Hyams C, Baxendale HE, Brown JS. Protective contributions against invasive Streptococcus pneumoniae pneumonia of antibody and Th17-cell responses to nasopharyngeal colonisation. PLoS ONE. (2011) 6:e25558. doi: 10.1371/journal.pone.0025558
77. Ferreira DM, Neill DR, Bangert M, Gritzfeld JF, Green N, Wright AK, et al. Controlled human infection and rechallenge with Streptococcus pneumoniae reveals the protective efficacy of carriage in healthy adults. Am J Respir Crit Care Med. (2013) 187:855–64. doi: 10.1164/rccm.201212-2277OC
78. Magee AD, Yother J. Requirement for capsule in colonization by Streptococcus pneumoniae. Infect Immun. (2001) 69:3755–61. doi: 10.1128/IAI.69.6.3755-3761.2001
Keywords: Streptococcus pneumoniae, Lgt, lipoprotein, live attenuated vaccine, mucosal immunity
Citation: Jang A-Y, Ahn KB, Zhi Y, Ji H-J, Zhang J, Han SH, Guo H, Lim S, Song JY, Lim JH and Seo HS (2019) Serotype-Independent Protection Against Invasive Pneumococcal Infections Conferred by Live Vaccine With lgt Deletion. Front. Immunol. 10:1212. doi: 10.3389/fimmu.2019.01212
Received: 14 January 2019; Accepted: 13 May 2019;
Published: 29 May 2019.
Edited by:
Rajko Reljic, St. George's, University of London, United KingdomReviewed by:
Pietro Speziale, University of Pavia, ItalySimon Michael Cutting, Royal Holloway, University of London, United Kingdom
Copyright © 2019 Jang, Ahn, Zhi, Ji, Zhang, Han, Guo, Lim, Song, Lim and Seo. This is an open-access article distributed under the terms of the Creative Commons Attribution License (CC BY). The use, distribution or reproduction in other forums is permitted, provided the original author(s) and the copyright owner(s) are credited and that the original publication in this journal is cited, in accordance with accepted academic practice. No use, distribution or reproduction is permitted which does not comply with these terms.
*Correspondence: Jae Hyang Lim, amxpbTE5QGV3aGEuYWMua3I=; Ho Seong Seo, aG9zZW9uZ3Nlb0BrYWVyaS5yZS5rcg==
†These authors have contributed equally to this work