- Paul Klein Center for Immune Intervention, Institute for Molecular Medicine, University Medical Center of the Johannes Gutenberg-University, Mainz, Germany
Dendritic cells (DC) fulfill an essential sentinel function within the immune system, acting at the interface of innate and adaptive immunity. The DC family, both in mouse and man, shows high functional heterogeneity in order to orchestrate immune responses toward the immense variety of pathogens and other immunological threats. In this review, we focus on the Langerin+CD8+ DC subpopulation in the spleen. Langerin+CD8+ DC exhibit a high ability to take up apoptotic/dying cells, and therefore they are essential to prime and shape CD8+ T cell responses. Next to the induction of immunity toward blood-borne pathogens, i.e., viruses, these DC are important for the regulation of tolerance toward cell-associated self-antigens. The ontogeny and differentiation pathways of CD8+CD103+ DC should be further explored to better understand the immunological role of these cells as a prerequisite of their therapeutic application.
Introduction
Dendritic cells (DC) link pathogen sensing and activation of innate immunity to the initiation of (primary) adaptive immune responses. For the latter, DC function as professional antigen (Ag)-presenting phagocytes that orchestrate the priming and polarization of naïve T cells. Importantly, next to stimulating protective immunity following infection, cancer or vaccination, DC are also crucial for the maintenance of immunological (self-) tolerance.
In the steady state, the murine DC family encompasses several cell populations that are very heterogeneous in development, phenotype and differ in their immune-regulatory functions. This variety among DC that have evolved at distinct immunological sites, allows immune responses to be specifically tailored to a given pathogenic threat (1, 2). In general, DC can be categorized into two classes (Figure 1A). The first class consists of the natural type I interferon-producing plasmacytoid DC (pDC: CD11cintCD45RA+Ly6C+ cells). These pDC are poor in Ag-presentation but play a crucial role as first-line defense against viral infections and are involved in anti-tumor responses as well (3). The second class of DC comprises conventional (classical) DC (cDC), which are characterized by the expression of high levels of CD11c and MHC class II (MHCII). These cDC can be further separated into functionally specialized cDC1 and cDC2 populations, initially according to their phenotype, and later through their molecular signatures, ontogeny and unique transcription factor dependency (4) (Table 1). These cDC1 and cDC2 populations are defined across different organs, and display distinct responses to pathogen- and danger-associated signals and, subsequently, specialized capacities to interact with T cells (5, 6). Understanding DC biology becomes even more complex as both the cDC1 and cDC2 populations can be further divided based on their localization and migratory abilities into (i) peripheral tissue (migratory) DC and (ii) lymphoid organ-resident DC subpopulations. Whereas, resident DC do not leave the lymph nodes (LN), spleen or thymus, migratory DC are the prototypic DC described by the Langerhans paradigm (7, 8). These migratory DC strategically line the barrier organs toward the external environment (e.g., skin and mucosa), and sample the tissues for invading pathogens (including commensals) and incoming immunogenic particles. Upon Ag encounter, together with pro-inflammatory stimuli, these DC move from the tissues into the T cell areas of local LN where they initiate protective T cell responses (9).
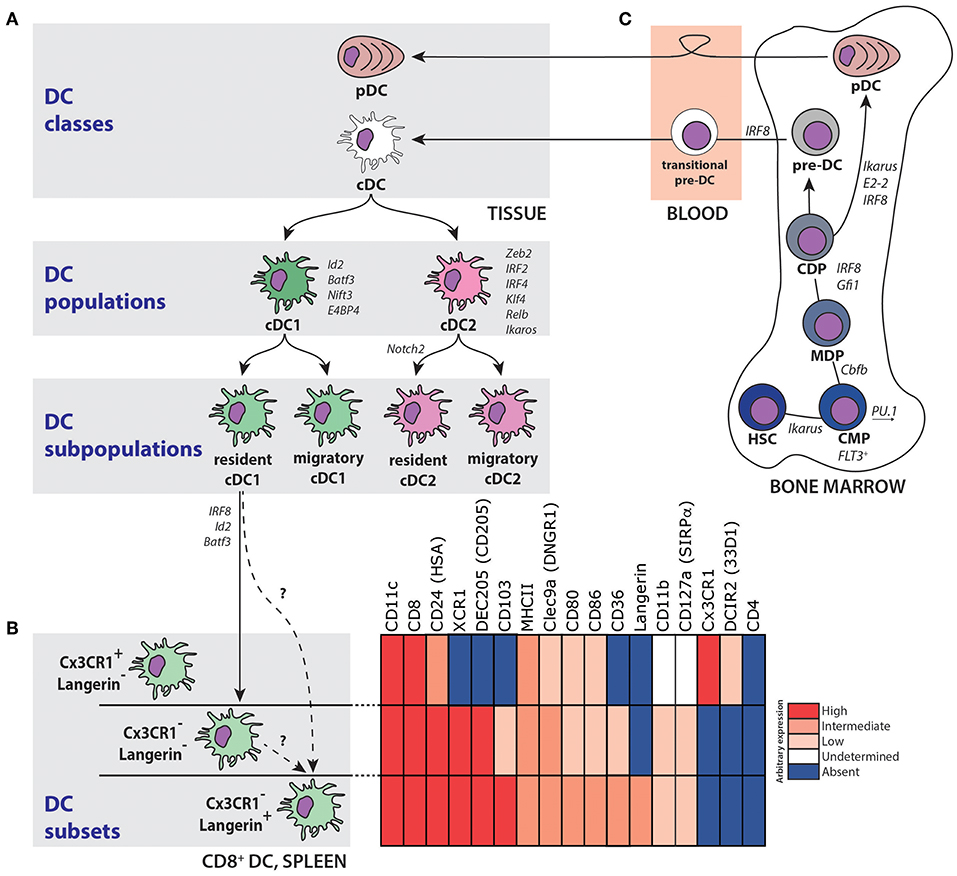
Figure 1. Development and division of the DC network. (A) The DC family can be divided into two distinct classes; plasmacytoid DC (pDC) and conventional DC (cDC). Subsequently, these cDC can be further subdivided into a cDC1 and a cDC2 population, of which both a lymphoid organ-resident and migratory tissue-specific subpopulations exist. A selection of transcription and other factors important for cDC1 and cDC2 differentiation and homeostasis is indicated in italics (B) Resident CD8+ DC in the spleen consist of, at least, three subsets with both phenotypical and functional specializations. Expression of selected markers on these subsets is pointed out with the indicated color-code. (C) Multipotent progenitors in the BM give rise to DC via a hierarchical series of dichotomous cell fate decisions. Selected transcription factors and other mediators important for DC development are indicated in italics. HSC, hematopoietic stem cell; CMP, common myeloid progenitor; MDP, macrophage/DC precursor; CDP, common DC precursor; pre-DC, precursor DC.
In this review, we will first recapitulate cDC heterogeneity in the spleen, and then zoom in on one particular splenic DC subset, namely, Langerin+CD8+ cDC1. In particular, we will summarize recent highlights in the biology of this DC subset, discuss its functional specialization in mice, touch upon the human equivalents and finally conclude by discussing potential concepts to harness these Langerin+CD8+ cDC1 to develop improved therapeutic and / or vaccination strategies.
Heterogeneity of Splenic cDC Subsets
The spleen is the largest secondary lymphoid organ of the body and is functionally linked to the systemic blood circulation (Figure 2A). Histologically, the spleen consists of red pulp (RP) and white pulp (WP). The RP is a loose venous sinusoidal meshwork involved in blood filtration, while the WP contains T cell-rich periarteriolar lymphoid sheaths (PALS) and discrete B cell follicles. Thereby, the WP resembles the lymphoid structures found in LN and is thus essential for the induction of adaptive immune responses. A specialized environment called the marginal zone (MZ) is uniquely situated at the transition site between the scavenging RP and the lymphoid WP. As the arterial bloodstream opens into the marginal sinuses, most of the blood entering the spleen passes the MZ (Figure 2A). The splenic MZ, therefore, is together with the RP involved in the filtration of the blood and constitutes the prime site for the detection of blood-borne Ag (10).
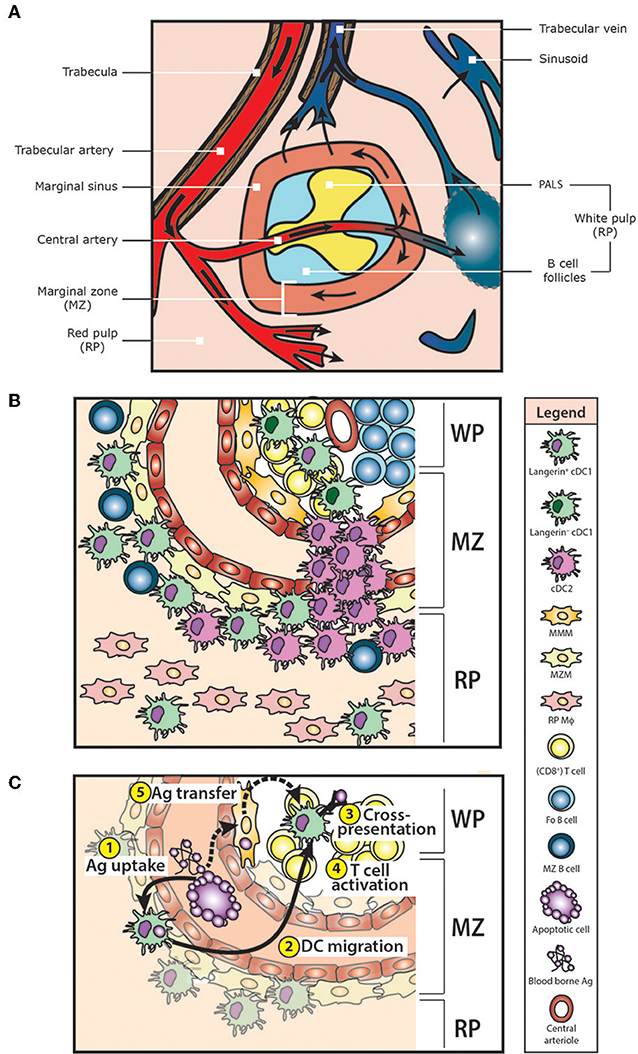
Figure 2. Structure and cellular composition of the murine spleen. (A) The spleen consists of red pulp (RP) and white pulp (WP). Blood enters the spleen via the splenic artery, which is subsequently branching into the trabecular arteries and central arteries. Finally, small arterioles and capillaries end up in the RP. The RP is a venous sinusoidal system containing connective tissue, sinuses and venules. Here, blood can leave the open ends of splenic RP capillaries, allowing free percolation into the RP and subsequent re-collected into the sinuses for venous drainage. In mice, the WP is composed of B cell follicles and T cell areas (the periarterial lymphatic sheaths, PALS) surrounding a central arteriole. The marginal zone (MZ) separates the WP from the RP. As marginal sinuses are opening in the MZ, most of the arterial blood that enters the spleen is running through the MZ. Furthermore, re-circulating lymphocytes can leave the blood in the MZ. (B) At least 2 types of macrophages are present in the MZ. Marginal metallophilic macrophages (MMM) are located as a tight network in the inner part of the MZ near the WP. Marginal zone macrophages (MZM) can be found in the outer MZ facing the RP. Scattered between these MZM are marginal zone B cells (MZ B cells) and Langerin+CD8+ cDC1, whereas cDC2 are mainly located in so called bridging channels, which are interruptions in the MZ sinus and macrophage rims. Some Langerin+CD8+ cDC1 are also present in the RP and WP T cell areas. In the RP, red pulp macrophages (RP Mϕ) can be identified. (C) Langerin+CD8+ cDC1 are involved in the direct uptake, processing and cross-presentation of blood-borne antigens (Ag). Upon Ag encounter ①, Langerin+CD8+ cDC1 migrate out of the MZ into the WP T cell areas ② to prime Ag-specific T cell responses ③. Depending on the type of Ag, this results in CD8+ T cell activation, or in CD8+ T cell tolerance ④. Moreover, Langerin+CD8+ cDC1 are able to acquire Ag from other cells (e.g., potentially from MMM), via a process called Ag-transfer ⑤.
Ag larger than 75 kDa are trapped and cleared by a large number of specialized MZ-resident phagocytic cells, including marginal zone macrophages (MZM), marginal metallophilic macrophages (MMM) and marginal zone B cells (MZB), thereby initiating immune responses against systemic pathogens (10–13) (Figure 2B). Moreover, the MZ is of vital importance for the clearance of apoptotic cells and the subsequent induction of self-tolerance, which can be abrogated by the depletion of macrophages (Mϕ) in the MZ (14, 15).
The splenic DC compartment only consists of resident DC as the spleen is not connected to the afferent lymphatic system by which migratory DC traffic from the peripheral tissues to LN. Historically, splenic cDC were defined based on the reciprocal expression of CD4 and CD11b or the CD8αα homodimer into at least three distinct DC subsets: (i) a CD8αα-expressing CD8+CD11b− cDC1 subset, and a CD11b+ cDC2 subpopulation that can be further divided into (ii) CD4+CD8− DC and (iii) CD4−CD8− double-negative DC subsets. To date, unsupervised phenotypic analysis, for example using (single cell) RNA sequencing and high-dimensional flow cytometry or mass cytometry, has added a large number of additional subpopulation-specific markers, confirming the existence of heterogeneity (DC subsets) within both cDC1 and cDC2 subpopulations (16). All of these phenotypically distinct cDC subsets may exert specialized roles in, respectively, promoting and suppressing different facets of immunity (Table 1).
Splenic cDC1
Analysis by flow cytometry indicated that the majority of splenic CD8+ cDC1 co-express the C-type lectin receptors DEC205 (CD205) and Langerin (CD207) (Figure 1B). Initially, staining spleen sections for DEC205 localized CD8+ cDC1 in the PALS only (11, 15, 17–20), resulting in the dogma that CD8+ cDC1 were restricted to the WP (17, 19, 21–23). In contrast, Langerin was predominantly detected in the MZ and only in limited amounts in the RP and the PALS by histology (24–28). This discrepancy in (co-) localization of Langerin and DEC205 between methods may be due to DEC205 levels too low to be detected by histology, resulting in variable DEC205 expression on slides. Therefore, it is now generally accepted that in the steady state CD8+ cDC1 are mainly located in the MZ and RP, and that they are not limited to the WP (28–30) (Figure 2B).
CD8+ cDC1 are characterized by a high ability to cross-present cell-associated and soluble Ag (31–36), and predominantly induce TH1-type helper T cell responses (36–38), as well as regulatory T cells (TREG) via TGFβ (Figure 2C). Moreover, CD8+ cDC1 can activate and polarize invariant natural killer T (iNKT) cells via CD1d presentation of glycolipid Ag (39).
Although multiple reports revealed considerable heterogeneity within this subpopulation, functional features (e.g., cross-presentation) are, nevertheless, mainly attributed to the cDC1 subpopulation as a whole. However, differential expression of DEC205 and CX3CR1, for example, is believed to divide the CD8+ DC subpopulation into subsets that have distinct functions in pathogen-recognition and immune-modulation (40, 41) (Figure 1B). Although the origin of CX3CR1+CD8+ DC is not clear yet, these cells seem to lack many functional hallmarks of classical CD8+ cDC1, including cross-presentation and IL-12 secretion in response to microbial challenge. In addition, CX3CR1-expressing DC rearranged immunoglobulin genes and are thought to rather resemble pDC and to be closely related to CD8− DC (41), and therefore might not be considered as cDC1. Another chemokine receptor highly expressed on splenic CD8+ cDC1 is XCR1 (42), which potentially allows close interaction with activated T cells and NK cells. Surprisingly however, Diphtheria-toxin (DT) treatment of XCR1-DTR knock-in mice did not result in complete depletion, indicating that splenic CD8+ cDC1 include a distinct population that is not eliminated due to heterogeneous XCR1 expression (43). Also in the absence of functional Notch2 signaling the number of CD8+ DC is diminished, suggesting that at least a subset of splenic CD8+ cDC1 also depend on Notch2 (44).
Taken together, these observations indicate that several distinct resident CD8+ cDC1 subsets are present in the spleen, but that the potential functional heterogeneity within this cDC1 subpopulation is currently underappreciated, and that several cDC1-specific functions might turn out to be rather CD8+ subset-restricted characteristics.
Splenic cDC2
CD11b+CD8− cDC2 are the most abundant cDC in the lymphoid organs. In contrast to CD8+ cDC1, this cDC2 subpopulation is known to be heterogeneous, but less well defined in function. In general, CD8− cDC2 (also characterized by the specific expression of the C-type lectin receptor DCIR2) are preferentially involved in MHCII-restricted Ag presentation and TH2 priming (31, 45), although they also have the ability to cross-present exogenous Ag under certain circumstances (34, 46, 47).
The heterogeneous cDC2 population can be further subdivided according to the differential expression of CD4 and the endothelial cell-selective adhesion molecule (ESAM), although this does not result in clearly defined homogenous populations (48), which makes it difficult to determine individual immune-modulatory capacities. Due to their similarities in phenotype and gene expression profiles, both CD4+CD8− cDC2 (which largely co-express ESAM) and CD4−CD8− double-negative cDC2 are often collectively referred to as CD8− cDC2 (19, 49–52), however, according to recent studies these two subsets appear different (44, 53). For example, ESAMlow cDC2 exhibit a more myeloid signature with Csf-1R, Csf3R, CCR2 and Lysozyme expression, suggesting that they are related to monocytes rather than to cDC. As migratory ESAM−CD11b+ tissue cDC2 can arise from both bone marrow (BM)-DC progenitors and monocytes, it is still under debate whether these splenic ESAMlow cDC originate from circulating monocytes or not (44, 54). Most likely they arise from early progenitors such as Macrophage and Dendritic Cell Precursors (MDP) without the contribution of the Common Dendritic Cell Precursor (CDP) (44, 55).
CD8− cDC2 reside in the MZ and bridging channels of the spleen (18, 31, 56), which are interruptions in the MZ where the PALS is in contact with the RP allowing T cell entry into the WP (57, 58) (Figure 2B). The development of CD8− cDC2 (and more specifically, of the ESAM+ CD8− cDC2 subset) depends on Notch2 (59). Furthermore, the G protein-coupled receptor EBI2 determines the specific MZ positioning, thereby allowing signaling and crosstalk with MZ B cells and other cells via LTßR and SIRPα, which is essential for the homeostasis of CD8− cDC2 (18, 56, 60). In addition, Runx3 is required for the specification and homeostasis of CD8− cDC2, as ablation of Runx3 expression resulted in a substantial decrease of CD8− cDC2 numbers in the spleen (55).
Splenic Langerin+CD8+ cDC1
Expression of the endocytic receptor Langerin is a classical hallmark of Langerhans cells (LC) in the epidermis and skin-draining lymph nodes (61, 62). However, Langerin expression is not restricted to LC as also other skin DC subsets (i.e. dermal CD103+ DC) express Langerin and are functionally distinct (63–66). In addition, Langerin+ DC can be found as interdigitating cells in the T cell zones of LN, as well as in the gut and the lung (25, 62, 67–70). Among splenic cDC, Langerin expression is mainly found on CD8+ cDC1, though its expression is lower than on LC and primarily intracellular in location (71). Although percentages vary depending on the genetic background of the experimental mice, the Langerin+CD8+ DC subset constitutes the majority of CD8+ cDC1 in the spleen and DT-mediated ablation in Lang-DTREGFP mice can reach about 70% of the splenic CD8+ cDC1 subpopulation (24, 72–75).
Langerin+CD8+ cDC1 are primarily localized in the MZ, just internal to the F4/80+ RP Mϕ, interspersed with MZM and forming a ring around the CD169+ MMM (Figure 2B). In addition, a minor fraction of Langerin+CD8+ cDC1 can be found in the RP and PALS (74). Compared to their Langerin− counterparts, Langerin+CD8+ cDC1 share a common morphology with a similar expression profile of the classical splenic cDC1 markers like CD8αα, CD24, CD36, DEC205, Clec9a, ICAM, and XCR1 (Figure 1B and Table 1). In addition, Langerin+CD8+ cDC1 co-express high levels of the integrin CD103. In steady state, splenic cDC display a rather immature phenotype with low levels of MHCII and co-stimulatory molecules (76). In contrast to Langerin− cDC1, the baseline expression of the activation markers CD80 and CD86 are slightly higher on the Langerin+CD8+ cDC1 subset (24, 72, 75), but whether this reflects a functionally more mature state remains unknown. However, steady state levels of serum IL-12 were significantly decreased in mice depleted of Langerin+CD8+ cDC1, indicating that these DC are responsible for basal IL-12 production (77). At least, Langerin−CD8+ cDC1 are not unresponsive to inflammation as the characteristics of upregulated activation markers during Toll-like receptor (TLR) or glycolipid antigen α-galactosylceramide (α-Gal-Cer) stimulation are similar to Langerin+CD8+ cDC1 (75). Conclusively, Langerin marks a proportion of CD8+ cDC1 in the splenic MZ. Based on their specific localization and phenotypic characteristics, it is suggestive that Langerin+CD8+ cDC1 are important regulators of immune responses toward blood-borne Ag in the steady state and during inflammation.
Ontogeny and Molecular Regulation of Langerin+CD8+ cDC1
To date, conclusive data are lacking to define Langerin+CD8+ and Langerin−CD8+ DC as distinct steady state cDC1 subsets, because alternatively, Langerin-expression could merely reflect different developmental stages within the CD8+ cDC1 subset (Figure 1B). CD8+ cDC1 express much lower levels of Langerin as compared to LC and they lack the LC-specific intracellular organelles known as Birbeck granules. In addition, the spleen does not drain the skin. Therefore, Langerin+CD8+ cDC1 in the spleen are unrelated to LC and are continuously replaced by blood-borne precursors of a non-LC origin (65, 67).
In the tissue, cDC have, in general, a relatively finite half-life of about 4–6 days, with CD8+ cDC1 in the spleen having even higher turnover rates (36). Indeed, DT-mediated depletion of a significant proportion of splenic CD8+ cDC1 in Lang-DTREGFP knock-in mice was evident for a period of 2–3 days after which Langerin+CD8+ cDC1 repopulated the spleen and reached homeostatic levels again by day 7 (72, 73, 78). This differs somewhat from Langerin+CD8− dermal cDC1, which follow a similar kinetics after DT-mediated depletion, but fail to reach full reconstitution (79). Langerin+CD8+ cDC1 exhibit decreased survival as compared to Langerin−CD8+ cDC1 upon in vitro activation or upon in vivo cell transfer. For example, treatment of mice with TLR-ligands or the innate invariant NKT (iNKT) cell ligand α-Gal-Cer also resulted in a fast decline of splenic Langerin+CD8+ cDC1 numbers, which peaked after 15–24 h. However, Langerin−CD8+ cDC1 numbers remained unchanged, suggesting that activation does not convert Langerin+ into Langerin− cDC1 but that Langerin+CD8+ cDC1 are rather sensitive to activation-induced cell death (24, 75, 80). Although the exact mechanisms remain elusive, in this setup TNFα may be one factor inducing cell death in Langerin+CD8+ cDC1 (80).
cDC are of myeloid origin and develop from hematopoietic stem cells (HSC) in the BM, but the exact developmental pathways of different cDC lineages remain controversial and difficult to elucidate (1, 5, 50, 54, 81–86) (Figure 1). It is generally accepted that all cDC precursors share a common differentiation pathway depending on the transcription factors PU.1 and Zbtb46 until they become committed common DC precursors (CDP) (87, 88). Subsequently, the developmental pathway of pDC and cDC diverges as CPD have the ability to differentiate into either cells of the pDC lineage or cDC precursors (pre-DC). Pre-DC, that are dependent on FLT3-L (89, 90), migrate into peripheral tissues to further mature into either cDC1 or cDC2 driven by specific transcription factors and cytokine combinations (1, 91, 92). Further differentiation of cDC1 is strictly guided by the hierarchical expression of Irf8, Id2, and Batf3, as targeted deletion of these transcription factors in mice leads to severe developmental defects in cDC1 causing a marked decrease of splenic CD8+ DC numbers (34, 93–95).
So far, no Langerin+CD8+ cDC1-specific transcriptional program has been identified. Moreover, both Langerin+CD8+ and Langerin−CD8+ cDC1 express similar levels of the cDC1-associated transcription factors Irf8, Id2, Nfil3, and Batf3 (75), indicating that the two subsets share a similar ontogeny and thus do not arise from distinct developmental pathways. Indeed, the complete lack of CD8+ cDC1 in Irf8-deficient mice suggests that Irf8 is critically involved in Langerin+CD8+ cDC1 development (96–99). Interestingly, infection with intracellular bacteria (e.g., Mycobaterium tuberculosis, Listeria and Toxoplasma) could functionally restore the CX3CR1−Langerin−CD8+ cDC1 compartment in Batf3 KO mice, while these mice still lack the majority of splenic Langerin+CD8+ cDC1 (100, 101). Purified Langerin−CD8+ cDC1 started to express Langerin upon transfer into naïve mice, with up to 60–70% of cells stably expressing the Langerin receptor 40 h post transfer (75). Moreover, gain of Langerin expression by Langerin−CD8+ cDC1 has been associated with their differentiation into a more mature cDC population with increased capacity to phagocytose dead cells, secrete IL-12 and cross-prime CD8+ T cells (75). The cytokine granulocyte-macrophage CSF (GM-CSF) enhances the differentiation of cross-presenting splenic CD103+ cDC1 during bacterial infection (102), but whether GM-SCF signaling is also able to induce Langerin expression on these cDC1 is not clear yet. Conclusively, these data suggest that Langerin−CX3CR1−CD8+ DC might be precursors of the (functionally mature) Langerin+CD8+ subset, and that the differentiation into mature Langerin+CD8+ DC depends on Batf3 and yet undefined conditions (75, 94).
Cross-Presentation of Cell-Associated AG by Langerin+CD8+ DC
CD8+ cDC1 not only cross-present Ag under inflammatory settings, as cross-presentation by these cDC1 under steady state conditions is important for efficient induction of tolerance to self-Ag. Moreover, antigen-presenting cells (APC) in the splenic MZ exhibit a high phagocytic capacity for dying / apoptotic cells, suggesting that the MZ is essential for the initiation of immune self-tolerance (14). Indeed, experiments using intravenously injected apoptotic cells revealed that these cells initially accumulated in the MZ where they were preferentially phagocytosed by CD8+ cDC1 rather than by CD8− cDC2. Notably, not all CD8+ cDC1 had the ability to cross-present, as only about half of the CD8+ cDC1 phagocytosed apoptotic cells, independent of the number of injected apoptotic cells (24). Phagocytic active CD8+ cDC1 specifically expressed Langerin and CD103, whereas Langerin− cDC1 did not acquire apoptotic cells (24, 75). Phagocytosis of apoptotic cells by Langerin+CD8+ cDC1 induced their migration from the MZ to the WP (24). This is in line with previous studies demonstrating redistribution of CD8+XCR1+ cDC1 from the MZ to the center of the T cell zones, where CD8+ T cells concentrate upon challenge with LPS (21, 28). Here, phagocytosis of apoptotic cells by Langerin+CD8+ DC resulted in efficient cross-presentation of their cell-associated Ag, while no significant CD4+ T cell priming was detected (24, 72).
The observed differences in uptake and cross-presentation between Langerin+CD8+ and Langerin−CD8+ cDC1 could result from a general inability of Langerin−CD8+ cDC1 to phagocytose apoptotic cells due to a lack of the respective uptake receptors for apoptotic cells. For example, antibody (Ab)-mediated Ag targeting to the recognition receptors DEC205 and Clec9a resulted in efficient cross-presentation and CTL priming (31, 103). Because Langerin+CD8+ cDC1 highly express DEC205 and Clec9a, as well as increased levels of the supposed dead-cell receptor CD36 (24), this cDC1 subset may represent a functionally distinct population with specific phagocytic capacities for apoptotic cells as compared to Langerin−CD8+ cDC1. Indeed, Langerin+CD8+ cDC1 displayed an intrinsic activity for the uptake of cell-associated Ag, while Langerin+CD8+ and Langerin−CD8+ cDC1 did not differ in their capacity to phagocytose bacteria, beads or soluble Ag (24). Nevertheless, CD8+ T cell activation in response to soluble Ag was much stronger in Langerin+CD8+ cDC1 compared to Langerin−CD8+ cDC1. This suggests that, although both cDC1 subsets acquired comparable amounts of Ag, Langerin+CD8+ and Langerin−CD8+ cDC1 subsets exhibit inherent differences in their Ag-processing machinery. To examine this in more detail, mice were challenged with exogenous cytochrome c (cyt c). As this pro-apoptotic molecule induces apoptosis when diverted into the cytoplasm, cyt c specifically depleted cells that possess cytosolic export mechanisms required for cross-presentation (104). Indeed, cyt c treatment selectively and dose-dependently ablated Langerin+CD8+ cDC1 but not Langerin−CD8+ DC. Moreover, depletion of splenic Langerin+CD8+ cDC1 in Lang-DTR mice also abrogated CD8+ T cell responses (72, 77). These data would identify Langerin+CD8+ cDC1 as the main professional cross-presenting subset within the CD8+ cDC1 subpopulation (24, 41, 72, 104).
Presentation of cell-associated Ag by Langerin+CD8+ cDC1 is critical for the maintenance of self-tolerance. Depletion of Langerin+CD8+ cDC1 prior to injection of MOG-expressing apoptotic cells and subsequent MOG/CFA immunization resulted in Ag-specific CD8+ T cell hypo-responsiveness and impaired EAE-progression (24). Whether this tolerance depends on the induction of regulatory T cells (TREG) by Langerin+CD8+ cDC1 remains unknown. However, DEC205 and Langerin Ag-targeting experiments revealed that, in contrast to Langerin+ migratory skin cDC, Langerin+CD8+ cDC1 in the spleen were inefficient in generating TREG in vivo (105). Splenic Langerin+CD8+ cDC1 can also acquire, process and cross-present lymphoma-derived Ag, both in vitro and in vivo (106). In this setting, Langerin+CD8+ cDC1 exhibit a tolerogenic function, indicated by decreased antitumor immunity, resulting from impaired naïve CD8+ T cells priming, possibly due to the lack of DC maturation and enhanced expression of the T cell suppressive ligand PD-L2 (106). In response to phagocytosis of dead cells, Langerin+CD8+ cDC1 strongly upregulate the expression of CD80 and the TNF superfamily ligand 4-1BBL (24), both T cell co-stimulatory molecules. Therefore, in combination with the appropriate stimulation such as TLR or licensing by bystander iNK T cells, Langerin-targeted Ag could stimulate immunity and thus Ag-specific CTL responses (72, 103). In line, Langerin+CD8+ cDC1 specifically enhanced protective immune responses when pre-activated CD8+ T cells were transferred as antitumor treatment strategy (106).
Notably, the spleen is, next to the liver and BM, important for the clearance of aged red blood cells (RBC), where mainly Mϕ in the peripheral RP actively remove these senescent RBC (erythrophagocytosis) (107). However, damaged RBC that undergo ‘programmed cell death’-like apoptosis (eryptosis) are primarily taken up by splenic MZM and Langerin+ cDC (108).
In summary, the Langerin+CD8+ cDC1 subset is predominantly involved in clearance and cross-presentation of circulating apoptotic cells, but not, or only minimally, in MHCII presentation and subsequent CD4+ T cell priming in responses to the same Ag. Again, this does not answer whether the ability to cross-present depends on a certain maturation stage characterized by the expression of Langerin, or whether Langerin+CD8+ and Langerin−CD8+ cDC1 are two functionally discrete cDC1 subsets, and this should be examined.
Function of Langerin+CD8+ DC During Systemic Infection
Langerin-expression identifies the cross-presenting cDC1 subset in the spleen. So far, it is not clear whether Langerin is merely a phenotypic marker for this specific CD8+ cDC1 subset, or whether the receptor actually exhibits functional properties. In LC, Langerin is associated with the formation of Birbeck granules. However, these structures are absent in Langerin+CD8+ cDC1, and their formation cannot be induced by stimulation with anti-Langerin monoclonal Ab (67). In general, C-type lectin receptors like Langerin function as innate pattern recognition receptors. Langerin itself recognizes cell-surface carbohydrate structures on pathogens (e.g., mannose, fucose and n-acetylglucosamine). This recognition normally results in the internalization and subsequent presentation of pathogen-associated Ag on MHC molecules (109, 110). DEC205 is found in MHCII-rich late endosomes and lysosomes (111), and Ab-mediated Ag targeting to DEC205 in the absence of adjuvant resulted in CD4+ T cell priming and TREG expansion (112). However, direct comparison of Ab-mediated Ag targeting to Langerin and DEC205 indicate that, although targeting Langerin and DEC205 both resulted in comparable TH1 responses as determined by CD4+ T cell IFNγ production, DEC205 targeting resulted predominantly in CD8+ T cell proliferation (31, 103). In contrast, langerin-targeting resulted in efficient priming of both CD4+ and CD8+ T cells which was persistent for at least 14 days (113), indicating that Langerin on Langerin+CD8+ cDC1 may be involved in the delivery of Ag for presentation on both MHCI and MHCII molecules (103). As DEC205 and Langerin are co-expressed by CD8+ cDC1 subset, these data might suggest that both lectin receptors feed into different Ag-processing and presentation pathways. Since Langerin deficiency did not impair Ag-presentation of soluble Ag by LC (114), the expression of Langerin on Langerin+CD8+ cDC1 per se may not be a prerequisite for cross-presentation.
CD8+ cDC1 are specialized cross-presenting cells and the most potent producers of IL-12 under several inflammatory settings, such as CD40 stimulation (29, 36, 37, 51, 115, 116). IL-12 is a pro-inflammatory cytokine involved in NK cell responses and the differentiation of TH1 T cells (117). Langerin+CD8+ cDC1 produce high levels of IL-12 upon systemic stimulation, whereas Langerin−CD8+ cDC1 are poor IL-12 producers (77, 118). However, the requirement of IL-12 production by CD8+ cDC1 seems to depend on the type and timing of infection. While during the first hours of infection, Langerin−CD8+ cDC1 were essential for early and transient IL-12 production, later on and at least until 3 weeks post-infection, Langerin+CD8+ cDC1 were the dominant source of IL-12 (77). This study also identified that the depletion of Langerin+CD8+ cDC1 resulted in diminished protective immune responses against intravenous Mycobacterium bovis infection. Langerin+CD8+ cDC1-depleted mice displayed increased bacterial loads, due to decreased IL-12 production in combination with delayed and diminished CD8+ T cell responses (77). Interestingly, although CD8+ T cell responses recovered over time, the bacterial load continued to increase and could not be controlled. This indicates that early immune priming effects by Langerin+CD8+ cDC1 are essential for the fate of the immune response (77), which was also found for the negative regulatory role of LC during cutaneous Leishmania major infection (119).
Upon activation iNKT cells rapidly produce proinflammatory cytokines. Due to their immunoregulatory function, iNKT cells are implicated to play a role in infectious diseases, autoimmune diseases and cancer. iNKT cells can be activated by cDC and in turn activate cDC to produce IL-12. Although Langerin+CD8+ cDC1 are not required for the initial activation of iNKT cells (80), conditioning of Langerin+CD8+ cDC1 by these iNKT cells in combination with TLR stimulation synergistically enhanced cytokine secretion and sustained T cell priming capacities of Langerin+CD8+ cDC1 (120).
Although the crucial role of the spleen and its CD8+ cDC1 compartment for bacterial and viral clearance and for providing protective immunity is known, evidence about the specific contribution of Langerin+CD8+ cDC1 in these models is so far limited. Therefore, further studies will be needed to pursue the implication of Langerin+CD8+ cDC1-specific functions during systemic infections.
Langerin+CD8+ DC and Macrophage Interactions
In general, CD8+ cDC1 obtain Ag directly from their surrounding environment. The specific localization of Langerin+CD8+ cDC1 within the MZ strongly suggests that these cells are involved in efficient sampling of the blood (Figure 2C). However, these DC poorly phagocytose blood-borne Ag as compared to the various Mϕ subsets in the MZ (74). Using polystyrene particles or bacteria, <10% of the Langerin+CD8+ cDC1 were able to phagocytose these Ag as compared to the majority of MZ Mϕ (24, 113). Thus, the bulk of particles from the blood is cleared by Mϕ and not DC, even though the cells are in close proximity. Notably, phagocytosis assays primarily assess the level of particle scavenging or clearance, a feature of Mϕ, but they do not provide information regarding the efficiency of downstream steps, such as Ag-processing and Ag-presentation by DC to T cells.
Another, although a less well appreciated mechanism of Ag acquisition is the transfer of Ag between APC (121, 122). This functional interaction would allow cDC to initiate protective T cell responses even if Ag availability and accessibility is limited. For example, Langerin+CD8+ cDC1 are able to cross-present Ag from injected Ag-loaded allogeneic BM-DC and mount CD8+ T cell responses without affecting CD4+ T cell responses (118). Depletion of the Langerin+CD8+ DC population in the Lang-DTREGFP mice abrogated this indirect Ag-presentation and thus subsequent CD8+ T cell priming. These data indicate that Langerin+CD8+ cDC1 were able to acquire Ag indirectly from other APC populations via Ag-transfer in the presence of a potent adjuvant. Notably, this Ag transfer is not limited to protein Ag, as the glycolipid α-Gal-Cer could also be acquired and presented by endogenous Langerin+CD8+ cDC1 (118).
Moreover, Ag initially acquired by CD169+ MMM, either after monoclonal Ab-mediated Ag targeting or during adenoviral infection, could specifically be presented by CD8+ DC, suggesting transfer from MMM to CD8+ cDC1 (123–125). Unfortunately, Langerin-expression on this cross-presenting CD8+ cDC1 has not been studied, but as this Ag-transfer absolutely requires a Batf3-dependent, Clec9a+ cDC1 (125), it is very suggestive that the Langerin+CD8+ cDC1 subset is the prime candidate to govern this process due to its Clec9a expression, Batf3-dependency and localization in the MZ (Figure 2C).
Langerin+CD8+ cDC1: Implications for Immunotherapy
The ultimate goal of every vaccination strategy to treat chronic infections and cancer is the induction of durable and protective T cell responses. For this purpose, proteins are very useful, were it not that they are poorly immunogenic. Notably, the immunogenicity of proteins can be immensely enhanced via targeting to cDC. This Ag-targeting, in combination with appropriate DC maturation signals like αCD40, PolyIC or conditioning by activated NKT cell strongly boost Ag-specific T cell responses. Therefore, identification and functional characterization of cDC (subsets) to reinforce vaccination efficiency is of great interest. Currently, many protein-targeting strategies utilize DEC205 and Clec9a receptors, but this will result in targeting of additional cell types as their expression is not cDC restricted (103). In contrast, murine Langerin expression is confined to the CD8+ cDC1 subpopulation. Furthermore, the Langerin+CD8+ cDC1 subset appears to be specialized in prolonged Ag cross-presentation with sustained T cell priming capacities and IL-12 production, making it a prime candidate for improved DC targeting and vaccination strategies (120).
However, do human equivalents of murine Langerin+CD8+ cDC1 actually exist? And if so, what will be their relevance and how much of the murine knowledge is translatable to the human system? At first glance, similar to mice, the human DC network can be divided into multiple phenotypically and functionally distinct cDC1 and cDC2 resident and migratory DC subpopulations (16, 126, 127). However, direct comparison of the murine and human cDC subsets remains challenging (85). First of all, several differences in DC ontogeny between mice and men exist. For example, Irf8-deficiency in human resulted in a lack of both cDC1 and cDC2 subsets (128). Secondly, many of the markers used to phenotypically discriminate between the different murine DC subsets cannot be used in humans. Yet, it is now widely accepted that the expression of CD141 (BDCA3), Clec9a and XCR1 marks human cDC1, while human cDC2 are identified by CD1c (BDCA1) expression (4, 126, 129–133) (Table 1). Indeed, the CD141+ cDC exhibit several phenotypical (16, 134–137), transcriptional (138, 139) and functional characteristics (140–142) corresponding to murine CD8+ cDC1. On the other hand, no expression of Langerin could be detected on these human CD141+ cDC1. Moreover, the division of labor between human cDC1 and cDC2 might be less strict as compared to mice. Generally, human CD141+ cDC1 and CD1c+ cDC2 are specialized in MHCI and MHCII presentation, respectively. However, depending on the type of Ag they encounter the both human resident cDC subpopulations can do both (143–146). Furthermore, human cDC1 produce IL-12, but in contrast to the mouse, also human cDC2 produce IL-12 at similar or even higher levels. These observations essentially suggest that human cDC2 are involved in orchestrating TH1 immune responses. In line, a population of human CD1a+ cDC, closely related to CD1c+ cDC2, expresses low levels of Langerin (142, 147–149). Accordingly, a fraction of Langerin+ cDC in the mouse lacks the expression of various markers that are associated with cross-presenting cDC1 (e.g., CD103), suggesting that some cDC2 are included in the Langerin+ cDC fraction as well (150). Therefore, the question whether human CD141+ cDC1 are functional equivalents of the murine Langerin+CD8+ cDC1 remains open, leaving the possibility that these counterparts may be found within the human CD1c+ cDC2 subpopulation.
Another factor potentially determining the functional specialization of the murine Langerin+CD8+ cDC1 subset might be their unique micro-anatomical niche within the spleen (Figure 2). Like the murine spleen, human spleen consists of RP and WP with similar functions, except that its micro-architecture differs in several ways. Importantly, humans lack marginal sinuses, and therefore the well-defined MZ found in rodents is as such absent in human spleen. Instead, humans possess a distinct histological compartment consisting of an inner and outer MZ surrounded by the perifollicular zone (10, 151, 152). Although this region might functionally represent the murine MZ, it is characterized by a different blood flow and different cellular composition (153, 154). To prevent confusion, this region may therefore better be described as the superficial zone (153). DEC205+ cDC are abundantly localized in this superficial zone (155), indicating that these cells, equivalent to mice, are involved in initiating (adaptive) immune responses toward blood-borne Ag. This incomplete picture also illustrates that still many open questions remain, which should be the subject of further research into human cDC subpopulations in order to harness these cells for immunotherapy.
Conclusions and Future Perspectives
Many (if not all) immune functions attributed to the splenic cDC1 subpopulation appear to be exerted by the Langerin+ subset. However, several developmental and functional insights regarding the Langerin+CD8+ cDC1 subset and, in particular, the identification of its human counterpart remain to be clarified. On one hand, Langerin expression could reflect a more mature state of CD8+ cDC1, enabling them to perform their specific immune regulatory functions. On the other hand, certain factors expressed by cell types unique to the MZ (including MZM, MMM, MZ B cells, and sinus lining cells) may facilitate the specific properties of, exclusively, the Langerin+CD8+ cDC1 subset. Therefore, the elucidation of the relationship between Langerin+CD8+ cDC1 and the MZ, including the determination of factors supporting the unique properties of Langerin+CD8+ cDC1, may be of particular interest. The combination of high-dimensional techniques and unbiased analysis has already revealed distinct differentiation stages and/or subpopulations of human cDC1 and cDC2 (16, 126), and might allow the identification of human equivalents of the murine Langerin+CD8+ cDC1. These human cDC could then potentially be exploited for future therapies of e.g., chronic inflammatory diseases or cancer.
Author Contributions
All authors listed have made a substantial, direct and intellectual contribution to writing this review article, and approved it for publication.
Conflict of Interest Statement
The authors declare that the research was conducted in the absence of any commercial or financial relationships that could be construed as a potential conflict of interest.
Acknowledgments
The authors would like to thank the former and present members of their laboratories for discussion and their contributions to the work discussed in this review. We also thank Marlene Backer for comments on the manuscript. Currently, the work in our laboratory is supported by grants from the German Research Foundation (DFG) to RAB (BA 5939/2-1) and BEC (CL 419/2-1, CL 419/4-1 and CRC 1292). BEC and RAB are members of the Research Center for Immunotherapy (FZI) Mainz.
References
1. Shortman K, Naik SH. Steady-state and inflammatory dendritic-cell development. Nat Rev Immunol. (2007) 7:19–30. doi: 10.1038/nri1996
2. Macri C, Pang ES, Patton T, O'Keeffe M. Dendritic cell subsets. Semin Cell Dev Biol. (2017). 84:11–21. doi: 10.1016/j.semcdb.2017.12.009
3. Alculumbre S, Raieli S, Hoffmann C, Chelbi R, Danlos FX, Soumelis V. Plasmacytoid pre-dendritic cells (pDC): from molecular pathways to function and disease association. Semin Cell Dev Biol. (2018) 86:24–35. doi: 10.1016/j.semcdb.2018.02.014
4. Guilliams M, Ginhoux F, Jakubzick C, Naik SH, Onai N, Schraml BU, et al. Dendritic cells, monocytes and macrophages: a unified nomenclature based on ontogeny. Nat Rev Immunol. (2014) 14:571–8. doi: 10.1038/nri3712
5. Sichien D, Lambrecht BN, Guilliams M, Scott CL. Development of conventional dendritic cells: from common bone marrow progenitors to multiple subsets in peripheral tissues. Mucosal Immunol. (2017) 10:831–44. doi: 10.1038/mi.2017.8
6. Schlitzer A, McGovern N, Ginhoux F. Dendritic cells and monocyte-derived cells: two complementary and integrated functional systems. Semin Cell Dev Biol. (2015) 41:9–22. doi: 10.1016/j.semcdb.2015.03.011
7. Wilson NS, Villadangos JA. Lymphoid organ dendritic cells: beyond the langerhans cells paradigm. Immunol Cell Biol. (2004) 82:91–8. doi: 10.1111/j.1440-1711.2004.01216.x
8. Clausen BE, Stoitzner P. Functional specialization of skin dendritic cell subsets in regulating T cell responses. Front Immunol. (2015) 6:534. doi: 10.3389/fimmu.2015.00534
9. Steinman RM, Idoyaga J. Features of the dendritic cell lineage. Immunol Rev. (2010) 234:5–17. doi: 10.1111/j.0105-2896.2009.00888.x
10. Mebius RE, Kraal G. Structure and function of the spleen. Nat Rev Immunol. (2005) 5:606–16. doi: 10.1038/nri1669
11. Kraal G, Mebius R. New insights into the cell biology of the marginal zone of the spleen. Int Rev Cytol. (2006) 250:175–215. doi: 10.1016/S0074-7696(06)50005-1
12. den Haan JM, Kraal G. Innate immune functions of macrophage subpopulations in the spleen. J Innate Immun. (2012) 4:437–45. doi: 10.1159/000335216
13. Kraal G. Cells in the marginal zone of the spleen. Int Rev Cytol. (1992) 132:31–74. doi: 10.1016/S0074-7696(08)62453-5
14. McGaha TL, Karlsson MC. Apoptotic cell responses in the splenic marginal zone: a paradigm for immunologic reactions to apoptotic antigens with implications for autoimmunity. Immunol Rev. (2016) 269:26–43. doi: 10.1111/imr.12382
15. Miyake Y, Asano K, Kaise H, Uemura M, Nakayama M, Tanaka M. Critical role of macrophages in the marginal zone in the suppression of immune responses to apoptotic cell-associated antigens. J Clin Invest. (2007) 117:2268–78. doi: 10.1172/JCI31990
16. Guilliams M, Dutertre CA, Scott CL, McGovern N, Sichien D, Chakarov S, et al. Unsupervised high-dimensional analysis aligns dendritic cells across tissues and species. Immunity. (2016) 45:669–84. doi: 10.1016/j.immuni.2016.08.015
17. Kraal G, Breel M, Janse M, Bruin G. Langerhans' cells, veiled cells, and interdigitating cells in the mouse recognized by a monoclonal antibody. J Exp Med. (1986) 163:981–97. doi: 10.1084/jem.163.4.981
18. Yi T, Cyster JG. EBI2-mediated bridging channel positioning supports splenic dendritic cell homeostasis and particulate antigen capture. Elife. (2013) 2:e00757. doi: 10.7554/eLife.00757
19. Naik SH. Demystifying the development of dendritic cell subtypes, a little. Immunol Cell Biol. (2008) 86:439–52. doi: 10.1038/icb.2008.28
20. Park SH, Cheong C, Idoyaga J, Kim JY, Choi JH, Do Y, et al. Generation and application of new rat monoclonal antibodies against synthetic FLAG and OLLAS tags for improved immunodetection. J Immunol Methods. (2008) 331:27–38. doi: 10.1016/j.jim.2007.10.012
21. De Smedt T, Pajak B, Muraille E, Lespagnard L, Heinen E, De Baetselier P, et al. Regulation of dendritic cell numbers and maturation by lipopolysaccharide in vivo. J Exp Med. (1996) 184:1413–24. doi: 10.1084/jem.184.4.1413
22. Lopez-Bravo M, Ardavin C. In vivo induction of immune responses to pathogens by conventional dendritic cells. Immunity. (2008) 29:343–51. doi: 10.1016/j.immuni.2008.08.008
23. Wu L, Liu YJ. Development of dendritic-cell lineages. Immunity. (2007) 26:741–50. doi: 10.1016/j.immuni.2007.06.006
24. Qiu CH, Miyake Y, Kaise H, Kitamura H, Ohara O, Tanaka M. Novel subset of CD8{alpha}+ dendritic cells localized in the marginal zone is responsible for tolerance to cell-associated antigens. J Immunol. (2009) 182:4127–36. doi: 10.4049/jimmunol.0803364
25. Valladeau J, Clair-Moninot V, Dezutter-Dambuyant C, Pin JJ, Kissenpfennig A, Mattei MG, et al. Identification of mouse langerin/CD207 in Langerhans cells and some dendritic cells of lymphoid tissues. J Immunol. (2002) 168:782–92. doi: 10.4049/jimmunol.168.2.782
26. Crozat K, Tamoutounour S, Vu Manh TP, Fossum E, Luche H, Ardouin L, et al. Cutting edge: expression of XCR1 defines mouse lymphoid-tissue resident and migratory dendritic cells of the CD8alpha+ type. J Immunol. (2011) 187:4411–5. doi: 10.4049/jimmunol.1101717
27. Alexandre YO, Ghilas S, Sanchez C, Le Bon A, Crozat K, Dalod M. XCR1+ dendritic cells promote memory CD8+ T cell recall upon secondary infections with Listeria monocytogenes or certain viruses. J Exp Med. (2016) 213:75–92. doi: 10.1084/jem.20142350
28. Calabro S, Liu D, Gallman A, Nascimento MS, Yu Z, Zhang TT, et al. Differential intrasplenic migration of dendritic cell subsets tailors adaptive immunity. Cell Rep. (2016) 16:2472–85. doi: 10.1016/j.celrep.2016.07.076
29. Neuenhahn M, Kerksiek KM, Nauerth M, Suhre MH, Schiemann M, Gebhardt FE, et al. CD8alpha+ dendritic cells are required for efficient entry of Listeria monocytogenes into the spleen. Immunity. (2006) 25:619–30. doi: 10.1016/j.immuni.2006.07.017
30. Chaussabel D, Pajak B, Vercruysse V, Bisseye C, Garze V, Habib M, et al. Alteration of migration and maturation of dendritic cells and T-cell depletion in the course of experimental trypanosoma cruzi infection. Lab Invest. (2003) 83:1373–82. doi: 10.1097/01.LAB.0000087587.93781.6F
31. Dudziak D, Kamphorst AO, Heidkamp GF, Buchholz VR, Trumpfheller C, Yamazaki S, et al. Differential antigen processing by dendritic cell subsets in vivo. Science. (2007) 315:107–11. doi: 10.1126/science.1136080
32. den Haan JM, Lehar SM, Bevan MJ. CD8(+) but not CD8(-) dendritic cells cross-prime cytotoxic T cells in vivo. J Exp Med. (2000) 192:1685–96. doi: 10.1084/jem.192.12.1685
33. Schnorrer P, Behrens GM, Wilson NS, Pooley JL, Smith CM, El-Sukkari D, et al. The dominant role of CD8+ dendritic cells in cross-presentation is not dictated by antigen capture. Proc Natl Acad Sci USA. (2006) 103:10729–34. doi: 10.1073/pnas.0601956103
34. Hildner K, Edelson BT, Purtha WE, Diamond M, Matsushita H, Kohyama M, et al. Batf3 deficiency reveals a critical role for CD8alpha+ dendritic cells in cytotoxic T cell immunity. Science. (2008) 322:1097–100. doi: 10.1126/science.1164206
35. Pooley JL, Heath WR, Shortman K. Cutting edge: intravenous soluble antigen is presented to CD4 T cells by CD8- dendritic cells, but cross-presented to CD8 T cells by CD8+ dendritic cells. J Immunol. (2001) 166:5327–30. doi: 10.4049/jimmunol.166.9.5327
36. Shortman K, Heath WR. The CD8+ dendritic cell subset. Immunol Rev. (2010) 234:18–31. doi: 10.1111/j.0105-2896.2009.00870.x
37. Maldonado-Lopez R, De Smedt T, Michel P, Godfroid J, Pajak B, Heirman C, et al. CD8alpha+ and CD8alpha- subclasses of dendritic cells direct the development of distinct T helper cells in vivo. J Exp Med. (1999) 189:587–92. doi: 10.1084/jem.189.3.587
38. Pulendran B, Smith JL, Caspary G, Brasel K, Pettit D, Maraskovsky E, et al. Distinct dendritic cell subsets differentially regulate the class of immune response in vivo. Proc Natl Acad Sci USA. (1999) 96:1036–41. doi: 10.1073/pnas.96.3.1036
39. Arora P, Baena A, Yu KO, Saini NK, Kharkwal SS, Goldberg MF, et al. A single subset of dendritic cells controls the cytokine bias of natural killer T cell responses to diverse glycolipid antigens. Immunity. (2014) 40:105–16. doi: 10.1016/j.immuni.2013.12.004
40. Heath WR, Belz GT, Behrens GM, Smith CM, Forehan SP, Parish IA, et al. Cross-presentation, dendritic cell subsets, and the generation of immunity to cellular antigens. Immunol Rev. (2004) 199:9–26. doi: 10.1111/j.0105-2896.2004.00142.x
41. Bar-On L, Birnberg T, Lewis KL, Edelson BT, Bruder D, Hildner K, et al. CX3CR1+ CD8alpha+ dendritic cells are a steady-state population related to plasmacytoid dendritic cells. Proc Natl Acad Sci USA. (2010) 107:14745–50. doi: 10.1073/pnas.1001562107
42. Dorner BG, Dorner MB, Zhou X, Opitz C, Mora A, Guttler S, et al. Selective expression of the chemokine receptor XCR1 on cross-presenting dendritic cells determines cooperation with CD8+ T cells. Immunity. (2009) 31:823–33. doi: 10.1016/j.immuni.2009.08.027
43. Yamazaki C, Sugiyama M, Ohta T, Hemmi H, Hamada E, Sasaki I, et al. Critical roles of a dendritic cell subset expressing a chemokine receptor, XCR1. J Immunol. (2013) 190:6071–82. doi: 10.4049/jimmunol.1202798
44. Lewis KL, Caton ML, Bogunovic M, Greter M, Grajkowska LT, Ng D, et al. Notch2 receptor signaling controls functional differentiation of dendritic cells in the spleen and intestine. Immunity. (2011) 35:780–91. doi: 10.1016/j.immuni.2011.08.013
45. Schulz O, Reis e Sousa C. Cross-presentation of cell-associated antigens by CD8alpha+ dendritic cells is attributable to their ability to internalize dead cells. Immunology. (2002) 107:183–9. doi: 10.1046/j.1365-2567.2002.01513.x
46. den Haan JM, Bevan MJ. Constitutive versus activation-dependent cross-presentation of immune complexes by CD8(+) and CD8(-) dendritic cells in vivo. J Exp Med. (2002) 196:817–27. doi: 10.1084/jem.20020295
47. Backer R, van Leeuwen F, Kraal G, den Haan JM. CD8- dendritic cells preferentially cross-present Saccharomyces cerevisiae antigens. Eur J Immunol. (2008) 38:370–80. doi: 10.1002/eji.200737647
48. Jaitin DA, Kenigsberg E, Keren-Shaul H, Elefant N, Paul F, Zaretsky I, et al. Massively parallel single-cell RNA-seq for marker-free decomposition of tissues into cell types. Science. (2014) 343:776–9. doi: 10.1126/science.1247651
49. Vremec D, Pooley J, Hochrein H, Wu L, Shortman K. CD4 and CD8 expression by dendritic cell subtypes in mouse thymus and spleen. J Immunol. (2000) 164:2978–86. doi: 10.4049/jimmunol.164.6.2978
50. Moore AJ, Anderson MK. Dendritic cell development: a choose-your-own-adventure story. Adv Hematol. (2013) 2013:949513. doi: 10.1155/2013/949513
51. McLellan AD, Kapp M, Eggert A, Linden C, Bommhardt U, Brocker EB, et al. Anatomic location and T-cell stimulatory functions of mouse dendritic cell subsets defined by CD4 and CD8 expression. Blood. (2002) 99:2084–93. doi: 10.1182/blood.V99.6.2084
52. Liu K, Nussenzweig MC. Origin and development of dendritic cells. Immunol Rev. (2010) 234:45–54. doi: 10.1111/j.0105-2896.2009.00879.x
53. Bialecki E, Macho Fernandez E, Ivanov S, Paget C, Fontaine J, Rodriguez F, et al. Spleen-resident CD4+ and CD4- CD8alpha- dendritic cell subsets differ in their ability to prime invariant natural killer T lymphocytes. PLoS ONE. (2011) 6:e26919. doi: 10.1371/journal.pone.0026919
54. Merad M, Sathe P, Helft J, Miller J, Mortha A. The dendritic cell lineage: ontogeny and function of dendritic cells and their subsets in the steady state and the inflamed setting. Annu Rev Immunol. (2013) 31:563–604. doi: 10.1146/annurev-immunol-020711-074950
55. Dicken J, Mildner A, Leshkowitz D, Touw IP, Hantisteanu S, Jung S, et al. Transcriptional reprogramming of CD11b+Esam(hi) dendritic cell identity and function by loss of Runx3. PLoS ONE. (2013) 8:e77490. doi: 10.1371/journal.pone.0077490
56. Gatto D, Wood K, Caminschi I, Murphy-Durland D, Schofield P, Christ D, et al. The chemotactic receptor EBI2 regulates the homeostasis, localization and immunological function of splenic dendritic cells. Nat Immunol. (2013) 14:446–53. doi: 10.1038/ni.2555
57. Mitchell J. Lymphocyte circulation in the spleen. marginal zone bridging channels and their possible role in cell traffic. Immunology. (1973) 24:93–107
58. Bajenoff M, Glaichenhaus N, Germain RN. Fibroblastic reticular cells guide T lymphocyte entry into and migration within the splenic T cell zone. J Immunol. (2008) 181:3947–54. doi: 10.4049/jimmunol.181.6.3947
59. Sekine C, Moriyama Y, Koyanagi A, Koyama N, Ogata H, Okumura K, et al. Differential regulation of splenic CD8- dendritic cells and marginal zone B cells by notch ligands. Int Immunol. (2009) 21:295–301. doi: 10.1093/intimm/dxn148
60. Kabashima K, Banks TA, Ansel KM, Lu TT, Ware CF, Cyster JG. Intrinsic lymphotoxin-beta receptor requirement for homeostasis of lymphoid tissue dendritic cells. Immunity. (2005) 22:439–50. doi: 10.1016/j.immuni.2005.02.007
61. Valladeau J, Ravel O, Dezutter-Dambuyant C, Moore K, Kleijmeer M, Liu Y, et al. Langerin, a novel C-type lectin specific to langerhans cells, is an endocytic receptor that induces the formation of birbeck granules. Immunity. (2000) 12:71–81. doi: 10.1016/S1074-7613(00)80160-0
62. Takahara K, Omatsu Y, Yashima Y, Maeda Y, Tanaka S, Iyoda T, et al. Identification and expression of mouse langerin (CD207) in dendritic cells. Int Immunol. (2002) 14:433–44. doi: 10.1093/intimm/14.5.433
63. Bursch LS, Wang L, Igyarto B, Kissenpfennig A, Malissen B, Kaplan DH, et al. Identification of a novel population of Langerin+ dendritic cells. J Exp Med. (2007) 204:3147–56. doi: 10.1084/jem.20071966
64. Ginhoux F, Collin MP, Bogunovic M, Abel M, Leboeuf M, Helft J, et al. Blood-derived dermal langerin+ dendritic cells survey the skin in the steady state. J Exp Med. (2007) 204:3133–46. doi: 10.1084/jem.20071733
65. Poulin LF, Henri S, de Bovis B, Devilard E, Kissenpfennig A, Malissen B. The dermis contains langerin+ dendritic cells that develop and function independently of epidermal langerhans cells. J Exp Med. (2007) 204:3119–31. doi: 10.1084/jem.20071724
66. Nagao K, Ginhoux F, Leitner WW, Motegi S, Bennett CL, Clausen BE, et al. Murine epidermal langerhans cells and langerin-expressing dermal dendritic cells are unrelated and exhibit distinct functions. Proc Natl Acad Sci USA. (2009) 106:3312–7. doi: 10.1073/pnas.0807126106
67. Douillard P, Stoitzner P, Tripp CH, Clair-Moninot V, Ait-Yahia S, McLellan AD, et al. Mouse lymphoid tissue contains distinct subsets of langerin/CD207 dendritic cells, only one of which represents epidermal-derived Langerhans cells. J Invest Dermatol. (2005) 125:983–94. doi: 10.1111/j.0022-202X.2005.23951.x
68. Flacher V, Douillard P, Ait-Yahia S, Stoitzner P, Clair-Moninot V, Romani N, et al. Expression of langerin/CD207 reveals dendritic cell heterogeneity between inbred mouse strains. Immunology. (2008) 123:339–47. doi: 10.1111/j.1365-2567.2007.02785.x
69. Sung SS, Fu SM, Rose CE Jr, Gaskin F, Ju ST, Beaty SR. A major lung CD103 (alphaE)-beta7 integrin-positive epithelial dendritic cell population expressing langerin and tight junction proteins. J Immunol. (2006) 176:2161–72. doi: 10.4049/jimmunol.176.9.5683
70. del Rio ML, Bernhardt G, Rodriguez-Barbosa JI, Forster R. Development and functional specialization of CD103+ dendritic cells. Immunol Rev. (2010) 234:268–81. doi: 10.1111/j.0105-2896.2009.00874.x
71. Cheong C, Idoyaga J, Do Y, Pack M, Park SH, Lee H, et al. Production of monoclonal antibodies that recognize the extracellular domain of mouse langerin/CD207. J Immunol Methods. (2007) 324:48–62. doi: 10.1016/j.jim.2007.05.001
72. Farrand KJ, Dickgreber N, Stoitzner P, Ronchese F, Petersen TR, Hermans IF. Langerin+ CD8alpha+ dendritic cells are critical for cross-priming and IL-12 production in response to systemic antigens. J Immunol. (2009) 183:7732–42. doi: 10.4049/jimmunol.0902707
73. Prendergast KA, Osmond TL, Ochiai S, Hermans IF, Kirman JR. Sustained in vivo depletion of splenic langerin(+) CD8alpha(+) dendritic cells is well-tolerated by lang-DTREGFP mice. J Immunol Methods. (2014) 406:104–9. doi: 10.1016/j.jim.2014.02.005
74. Idoyaga J, Suda N, Suda K, Park CG, Steinman RM. Antibody to Langerin/CD207 localizes large numbers of CD8alpha+ dendritic cells to the marginal zone of mouse spleen. Proc Natl Acad Sci USA. (2009) 106:1524–9. doi: 10.1073/pnas.0812247106
75. Petersen TR, Knight DA, Tang CW, Osmond TL, Hermans IF. Batf3-independent langerin- CX3CR1- CD8alpha+ splenic DCs represent a precursor for classical cross-presenting CD8alpha+ DCs. J Leukoc Biol. (2014) 96:1001–10. doi: 10.1189/jlb.1A0314-130R
76. Wilson NS, El-Sukkari D, Belz GT, Smith CM, Steptoe RJ, Heath WR, et al. Most lymphoid organ dendritic cell types are phenotypically and functionally immature. Blood. (2003) 102:2187–94. doi: 10.1182/blood-2003-02-0513
77. Prendergast KA, Daniels NJ, Petersen TR, Hermans IF, Kirman JR. Langerin(+) CD8alpha(+) dendritic cells drive early CD8(+) T cell activation and IL-12 production during systemic bacterial infection. Front Immunol. (2018) 9:953. doi: 10.3389/fimmu.2018.00953
78. Kissenpfennig A, Henri S, Dubois B, Laplace-Builhe C, Perrin P, Romani N, et al. Dynamics and function of langerhans cells in vivo: dermal dendritic cells colonize lymph node areas distinct from slower migrating langerhans cells. Immunity. (2005) 22:643–54. doi: 10.1016/j.immuni.2005.04.004
79. Noordegraaf M, Flacher V, Stoitzner P, Clausen BE. Functional redundancy of langerhans cells and langerin+ dermal dendritic cells in contact hypersensitivity. J Invest Dermatol. (2010) 130:2752–9. doi: 10.1038/jid.2010.223
80. Simkins HM, Hyde E, Farrand KJ, Ong ML, Degli-Esposti MA, Hermans IF, et al. Administration of alpha-galactosylceramide impairs the survival of dendritic cell subpopulations in vivo. J Leukoc Biol. (2011) 89:753–62. doi: 10.1189/jlb.0910480
81. Belz GT, Nutt SL. Transcriptional programming of the dendritic cell network. Nat Rev Immunol. (2012) 12:101–13. doi: 10.1038/nri3149
82. Liu K, Victora GD, Schwickert TA, Guermonprez P, Meredith MM, Yao K, et al. In vivo analysis of dendritic cell development and homeostasis. Science. (2009) 324:392–7. doi: 10.1126/science.1170540
83. Mildner A, Jung S. Development and function of dendritic cell subsets. Immunity. (2014) 40:642–56. doi: 10.1016/j.immuni.2014.04.016
84. Malissen B, Tamoutounour S, Henri S. The origins and functions of dendritic cells and macrophages in the skin. Nat Rev Immunol. (2014) 14:417–28. doi: 10.1038/nri3683
85. Schlitzer A, Ginhoux F. Organization of the mouse and human DC network. Curr Opin Immunol. (2014) 26:90–9. doi: 10.1016/j.coi.2013.11.002
86. Murphy TL, Grajales-Reyes GE, Wu X, Tussiwand R, Briseno CG, Iwata A, et al. Transcriptional control of dendritic cell development. Annu Rev Immunol. (2016) 34:93–119. doi: 10.1146/annurev-immunol-032713-120204
87. Meredith MM, Liu K, Darrasse-Jeze G, Kamphorst AO, Schreiber HA, Guermonprez P, et al. Expression of the zinc finger transcription factor zDC (Zbtb46, Btbd4) defines the classical dendritic cell lineage. J Exp Med. (2012) 209:1153–65. doi: 10.1084/jem.20112675
88. Satpathy AT, Kc W, Albring JC, Edelson BT, Kretzer NM, et al. Zbtb46 expression distinguishes classical dendritic cells and their committed progenitors from other immune lineages. J Exp Med. (2012) 209:1135–52. doi: 10.1084/jem.20120030
89. Naik SH, Sathe P, Park HY, Metcalf D, Proietto AI, Dakic A, et al. Development of plasmacytoid and conventional dendritic cell subtypes from single precursor cells derived in vitro and in vivo. Nat Immunol. (2007) 8:1217–26. doi: 10.1038/ni1522
90. Onai N, Obata-Onai A, Schmid MA, Ohteki T, Jarrossay D, Manz MG. Identification of clonogenic common Flt3+M-CSFR+ plasmacytoid and conventional dendritic cell progenitors in mouse bone marrow. Nat Immunol. (2007) 8:1207–16. doi: 10.1038/ni1518
91. Naik SH, Metcalf D, van Nieuwenhuijze A, Wicks I, Wu L, O'Keeffe M, et al. Intrasplenic steady-state dendritic cell precursors that are distinct from monocytes. Nat Immunol. (2006) 7:663–71. doi: 10.1038/ni1340
92. Diao J, Winter E, Cantin C, Chen W, Xu L, Kelvin D, et al. In situ replication of immediate dendritic cell (DC) precursors contributes to conventional DC homeostasis in lymphoid tissue. J Immunol. (2006) 176:7196–206. doi: 10.4049/jimmunol.176.12.7196
93. Seillet C, Jackson JT, Markey KA, Brady HJ, Hill GR, Macdonald KP, et al. CD8alpha+ DCs can be induced in the absence of transcription factors Id2, Nfil3, and Batf3. Blood. (2013) 121:1574–83. doi: 10.1182/blood-2012-07-445650
94. Edelson BT, Bradstreet TR, Kc W, Hildner K, Herzog JW, Sim J, et al. Batf3-dependent CD11b(low/-) peripheral dendritic cells are GM-CSF-independent and are not required for Th cell priming after subcutaneous immunization. PLoS ONE. (2011) 6:e25660. doi: 10.1371/journal.pone.0025660
95. Schiavoni G, Mattei F, Sestili P, Borghi P, Venditti M, Morse HC 3rd, et al. ICSBP is essential for the development of mouse type I interferon-producing cells and for the generation and activation of CD8alpha(+) dendritic cells. J Exp Med. (2002) 196:1415–25. doi: 10.1084/jem.20021263
96. Aliberti J, Schulz O, Pennington DJ, Tsujimura H, Reis e Sousa C, Ozato K, et al. Essential role for ICSBP in the in vivo development of murine CD8alpha + dendritic cells. Blood. (2003) 101:305–10. doi: 10.1182/blood-2002-04-1088
97. Tamura T, Tailor P, Yamaoka K, Kong HJ, Tsujimura H, O'Shea JJ, et al. IFN regulatory factor-4 and−8 govern dendritic cell subset development and their functional diversity. J Immunol. (2005) 174:2573–81. doi: 10.4049/jimmunol.174.5.2573
98. Tailor P, Tamura T, Morse HC 3rd, Ozato K. The BXH2 mutation in IRF8 differentially impairs dendritic cell subset development in the mouse. Blood. (2008) 111:1942–5. doi: 10.1182/blood-2007-07-100750
99. Sichien D, Scott CL, Martens L, Vanderkerken M, Van Gassen S, Plantinga M, et al. IRF8 transcription factor controls survival and function of terminally differentiated conventional and plasmacytoid dendritic cells, respectively. Immunity. (2016) 45:626–40. doi: 10.1016/j.immuni.2016.08.013
100. Tussiwand R, Lee WL, Murphy TL, Mashayekhi M, Kc W, Albring JC, et al. Compensatory dendritic cell development mediated by BATF-IRF interactions. Nature. (2012) 490:502–7. doi: 10.1038/nature11531
101. Mott KR, Maazi H, Allen SJ, Zandian M, Matundan H, Ghiasi YN, et al. Batf3 deficiency is not critical for the generation of CD8alpha(+) dendritic cells. Immunobiology. (2015) 220:518–24. doi: 10.1016/j.imbio.2014.10.019
102. Zhan Y, Carrington EM, van Nieuwenhuijze A, Bedoui S, Seah S, Xu Y, et al. GM-CSF increases cross-presentation and CD103 expression by mouse CD8(+) spleen dendritic cells. Eur J Immunol. (2011) 41:2585–95. doi: 10.1002/eji.201141540
103. Idoyaga J, Lubkin A, Fiorese C, Lahoud MH, Caminschi I, Huang Y, et al. Comparable T helper 1 (Th1) and CD8 T-cell immunity by targeting HIV gag p24 to CD8 dendritic cells within antibodies to langerin, DEC205, and Clec9A. Proc Natl Acad Sci USA. (2011) 108:2384–9. doi: 10.1073/pnas.1019547108
104. Lin ML, Zhan Y, Proietto AI, Prato S, Wu L, Heath WR, et al. Selective suicide of cross-presenting CD8+ dendritic cells by cytochrome c injection shows functional heterogeneity within this subset. Proc Natl Acad Sci USA. (2008) 105:3029–34. doi: 10.1073/pnas.0712394105
105. Idoyaga J, Fiorese C, Zbytnuik L, Lubkin A, Miller J, Malissen B, et al. Specialized role of migratory dendritic cells in peripheral tolerance induction. J Clin Invest. (2013) 123:844–54. doi: 10.1172/JCI65260
106. Gibbins JD, Ancelet LR, Osmond TL, Petersen TR, Hermans IF. Splenic dendritic cells involved in cross-tolerance of tumor antigens can play a stimulatory role in adoptive t-cell therapy. J Immunother. (2015) 38:321–9. doi: 10.1097/CJI.0000000000000096
107. Klei TR, Meinderts SM, van den Berg TK, van Bruggen R. From the cradle to the grave: the role of macrophages in erythropoiesis and erythrophagocytosis. Front Immunol. (2017) 8:73. doi: 10.3389/fimmu.2017.00073
108. Larsson A, Hult A, Nilsson A, Olsson M, Oldenborg PA. Red blood cells with elevated cytoplasmic Ca(2+) are primarily taken up by splenic marginal zone macrophages and CD207+ dendritic cells. Transfusion. (2016) 56:1834–44. doi: 10.1111/trf.13612
109. Brown GD, Willment JA, Whitehead L. C-type lectins in immunity and homeostasis. Nat Rev Immunol. (2018) 18:374–89. doi: 10.1038/s41577-018-0004-8
110. van der Vlist M, Geijtenbeek TB. Langerin functions as an antiviral receptor on langerhans cells. Immunol Cell Biol. (2010) 88:410–5. doi: 10.1038/icb.2010.32
111. Mahnke K, Guo M, Lee S, Sepulveda H, Swain SL, Nussenzweig M, et al. The dendritic cell receptor for endocytosis, DEC-205, can recycle and enhance antigen presentation via major histocompatibility complex class II-positive lysosomal compartments. J Cell Biol. (2000) 151:673–84. doi: 10.1083/jcb.151.3.673
112. Ring S, Maas M, Nettelbeck DM, Enk AH, Mahnke K. Targeting of autoantigens to DEC205(+) dendritic cells in vivo suppresses experimental allergic encephalomyelitis in mice. J Immunol. (2013) 191:2938–47. doi: 10.4049/jimmunol.1202592
113. Idoyaga J, Cheong C, Suda K, Suda N, Kim JY, Lee H, et al. Cutting edge: langerin/CD207 receptor on dendritic cells mediates efficient antigen presentation on MHC I and II products in vivo. J Immunol. (2008) 180:3647–50. doi: 10.4049/jimmunol.180.6.3647
114. Kissenpfennig A, Ait-Yahia S, Clair-Moninot V, Stossel H, Badell E, Bordat Y, et al. Disruption of the langerin/CD207 gene abolishes Birbeck granules without a marked loss of langerhans cell function. Mol Cell Biol. (2005) 25:88–99. doi: 10.1128/MCB.25.1.88-99.2005
115. Yrlid U, Wick MJ. Antigen presentation capacity and cytokine production by murine splenic dendritic cell subsets upon salmonella encounter. J Immunol. (2002) 169:108–16. doi: 10.4049/jimmunol.169.1.108
116. Reis e Sousa C, Hieny S, Scharton-Kersten T, Jankovic D, Charest H, Germain RN, et al. In vivo microbial stimulation induces rapid CD40 ligand-independent production of interleukin 12 by dendritic cells and their redistribution to T cell areas. J Exp Med. (1997) 186:1819–29. doi: 10.1084/jem.186.11.1819
117. Sun L, He C, Nair L, Yeung J, Egwuagu CE. Interleukin 12 (IL-12) family cytokines: role in immune pathogenesis and treatment of CNS autoimmune disease. Cytokine. (2015) 75:249–55. doi: 10.1016/j.cyto.2015.01.030
118. Petersen TR, Sika-Paotonu D, Knight DA, Simkins HM, Hermans IF. Exploiting the role of endogenous lymphoid-resident dendritic cells in the priming of NKT cells and CD8+ T cells to dendritic cell-based vaccines. PLoS ONE. (2011) 6:e17657. doi: 10.1371/journal.pone.0017657
119. Kautz-Neu K, Noordegraaf M, Dinges S, Bennett CL, John D, Clausen BE, et al. Langerhans cells are negative regulators of the anti-leishmania response. J Exp Med. (2011) 208:885–91. doi: 10.1084/jem.20102318
120. Osmond TL, Farrand KJ, Painter GF, Ruedl C, Petersen TR, Hermans IF. Activated NKT cells can condition different splenic dendritic cell subsets to respond more effectively to TLR engagement and enhance cross-priming. J Immunol. (2015) 195:821–31. doi: 10.4049/jimmunol.1401751
121. Allan RS, Waithman J, Bedoui S, Jones CM, Villadangos JA, Zhan Y, et al. Migratory dendritic cells transfer antigen to a lymph node-resident dendritic cell population for efficient CTL priming. Immunity. (2006) 25:153–62. doi: 10.1016/j.immuni.2006.04.017
122. Belz GT, Smith CM, Kleinert L, Reading P, Brooks A, Shortman K, et al. Distinct migrating and nonmigrating dendritic cell populations are involved in MHC class I-restricted antigen presentation after lung infection with virus. Proc Natl Acad Sci USA. (2004) 101:8670–5. doi: 10.1073/pnas.0402644101
123. Backer R, Schwandt T, Greuter M, Oosting M, Jungerkes F, Tuting T, et al. Effective collaboration between marginal metallophilic macrophages and CD8+ dendritic cells in the generation of cytotoxic T cells. Proc Natl Acad Sci USA. (2010) 107:216–21. doi: 10.1073/pnas.0909541107
124. Bernhard CA, Ried C, Kochanek S, Brocker T. CD169+ macrophages are sufficient for priming of CTLs with specificities left out by cross-priming dendritic cells. Proc Natl Acad Sci USA. (2015) 112:5461–6. doi: 10.1073/pnas.1423356112
125. van Dinther D, Veninga H, Iborra S, Borg EGF, Hoogterp L, Olesek K, et al. Functional CD169 on macrophages mediates interaction with dendritic cells for CD8(+) T cell cross-priming. Cell Rep. (2018) 22:1484–95. doi: 10.1016/j.celrep.2018.01.021
126. Villani AC, Satija R, Reynolds G, Sarkizova S, Shekhar K, Fletcher J, et al. Single-cell RNA-seq reveals new types of human blood dendritic cells, monocytes, and progenitors. Science. (2017) 356:eaah4573. doi: 10.1126/science.aah4573
127. See P, Dutertre CA, Chen J, Gunther P, McGovern N, Irac SE, et al. Mapping the human DC lineage through the integration of high-dimensional techniques. Science. (2017) 356:eaag3009. doi: 10.1126/science.aag3009
128. Hambleton S, Salem S, Bustamante J, Bigley V, Boisson-Dupuis S, Azevedo J, et al. IRF8 mutations and human dendritic-cell immunodeficiency. N Engl J Med. (2011) 365:127–38. doi: 10.1056/NEJMoa1100066
129. MacDonald KP, Munster DJ, Clark GJ, Dzionek A, Schmitz J, Hart DN. Characterization of human blood dendritic cell subsets. Blood. (2002) 100:4512–20. doi: 10.1182/blood-2001-11-0097
130. Dzionek A, Fuchs A, Schmidt P, Cremer S, Zysk M, Miltenyi S, et al. BDCA-2, BDCA-3, and BDCA-4: three markers for distinct subsets of dendritic cells in human peripheral blood. J Immunol. (2000) 165:6037–46. doi: 10.4049/jimmunol.165.11.6037
131. Summers KL, Hock BD, McKenzie JL, Hart DN. Phenotypic characterization of five dendritic cell subsets in human tonsils. Am J Pathol. (2001) 159:285–95. doi: 10.1016/S0002-9440(10)61694-X
132. McIlroy D, Troadec C, Grassi F, Samri A, Barrou B, Autran B, et al. Investigation of human spleen dendritic cell phenotype and distribution reveals evidence of in vivo activation in a subset of organ donors. Blood. (2001) 97:3470–7. doi: 10.1182/blood.V97.11.3470
133. Villadangos JA, Shortman K. Found in translation: the human equivalent of mouse CD8+ dendritic cells. J Exp Med. (2010) 207:1131–4. doi: 10.1084/jem.20100985
134. Caminschi I, Lahoud MH, Shortman K. Enhancing immune responses by targeting antigen to DC. Eur J Immunol. (2009) 39:931–8. doi: 10.1002/eji.200839035
135. Huysamen C, Willment JA, Dennehy KM, Brown GD. CLEC9A is a novel activation C-type lectin-like receptor expressed on BDCA3+ dendritic cells and a subset of monocytes. J Biol Chem. (2008) 283:16693–701. doi: 10.1074/jbc.M709923200
136. Sancho D, Mourao-Sa D, Joffre OP, Schulz O, Rogers NC, Pennington DJ, et al. Tumor therapy in mice via antigen targeting to a novel, DC-restricted C-type lectin. J Clin Invest. (2008) 118:2098–110. doi: 10.1172/JCI34584
137. Crozat K, Guiton R, Contreras V, Feuillet V, Dutertre CA, Ventre E, et al. The XC chemokine receptor 1 is a conserved selective marker of mammalian cells homologous to mouse CD8alpha+ dendritic cells. J Exp Med. (2010) 207:1283–92. doi: 10.1084/jem.20100223
138. Robbins SH, Walzer T, Dembele D, Thibault C, Defays A, Bessou G, et al. Novel insights into the relationships between dendritic cell subsets in human and mouse revealed by genome-wide expression profiling. Genome Biol. (2008) 9:R17. doi: 10.1186/gb-2008-9-1-r17
139. Crozat K, Guiton R, Guilliams M, Henri S, Baranek T, Schwartz-Cornil I, et al. Comparative genomics as a tool to reveal functional equivalences between human and mouse dendritic cell subsets. Immunol Rev. (2010) 234:177–98. doi: 10.1111/j.0105-2896.2009.00868.x
140. Bachem A, Guttler S, Hartung E, Ebstein F, Schaefer M, Tannert A, et al. Superior antigen cross-presentation and XCR1 expression define human CD11c+CD141+ cells as homologues of mouse CD8+ dendritic cells. J Exp Med. (2010) 207:1273–81. doi: 10.1084/jem.20100348
141. Jongbloed SL, Kassianos AJ, McDonald KJ, Clark GJ, Ju X, Angel CE, et al. Human CD141+ (BDCA-3)+ dendritic cells (DCs) represent a unique myeloid DC subset that cross-presents necrotic cell antigens. J Exp Med. (2010) 207:1247–60. doi: 10.1084/jem.20092140
142. Haniffa M, Shin A, Bigley V, McGovern N, Teo P, See P, et al. Human tissues contain CD141hi cross-presenting dendritic cells with functional homology to mouse CD103+ nonlymphoid dendritic cells. Immunity. (2012) 37:60–73. doi: 10.1016/j.immuni.2012.04.012
143. Macri C, Dumont C, Johnston AP, Mintern JD. Targeting dendritic cells: a promising strategy to improve vaccine effectiveness. Clin Transl Immunol. (2016) 5:e66. doi: 10.1038/cti.2016.6
144. Mittag D, Proietto AI, Loudovaris T, Mannering SI, Vremec D, Shortman K, et al. Human dendritic cell subsets from spleen and blood are similar in phenotype and function but modified by donor health status. J Immunol. (2011) 186:6207–17. doi: 10.4049/jimmunol.1002632
145. Segura E, Valladeau-Guilemond J, Donnadieu MH, Sastre-Garau X, Soumelis V, Amigorena S. Characterization of resident and migratory dendritic cells in human lymph nodes. J Exp Med. (2012) 209:653–60. doi: 10.1084/jem.20111457
146. Segura E, Durand M, Amigorena S. Similar antigen cross-presentation capacity and phagocytic functions in all freshly isolated human lymphoid organ-resident dendritic cells. J Exp Med. (2013) 210:1035–47. doi: 10.1084/jem.20121103
147. De Monte A, Olivieri CV, Vitale S, Bailleux S, Castillo L, Giordanengo V, et al. CD1c-related DCs that express CD207/langerin, but are distinguishable from langerhans cells, are consistently present in human tonsils. Front Immunol. (2016) 7:197. doi: 10.3389/fimmu.2016.00197
148. Bigley V, McGovern N, Milne P, Dickinson R, Pagan S, Cookson S, et al. Langerin-expressing dendritic cells in human tissues are related to CD1c+ dendritic cells and distinct from langerhans cells and CD141high XCR1+ dendritic cells. J Leukoc Biol. (2015) 97:627–34. doi: 10.1189/jlb.1HI0714-351R
149. Melum GR, Farkas L, Scheel C, Van Dieren B, Gran E, Liu YJ, et al. A thymic stromal lymphopoietin-responsive dendritic cell subset mediates allergic responses in the upper airway mucosa. J Allergy Clin Immunol. (2014) 134:613–21 e7. doi: 10.1016/j.jaci.2014.05.010
150. Henri S, Poulin LF, Tamoutounour S, Ardouin L, Guilliams M, de Bovis B, et al. CD207+ CD103+ dermal dendritic cells cross-present keratinocyte-derived antigens irrespective of the presence of langerhans cells. J Exp Med. (2010) 207:189–206. doi: 10.1084/jem.20091964
151. van Krieken JH, Te Velde J, Kleiverda K, Leenheers-Binnendijk L, van de Velde CJ. The human spleen; a histological study in splenectomy specimens embedded in methylmethacrylate. Histopathology. (1985) 9:571–85. doi: 10.1111/j.1365-2559.1985.tb02841.x
152. van Krieken JH, te Velde J. Immunohistology of the human spleen: an inventory of the localization of lymphocyte subpopulations. Histopathology. (1986) 10:285–94. doi: 10.1111/j.1365-2559.1986.tb02482.x
153. Steiniger BS. Human spleen microanatomy: why mice do not suffice. Immunology. (2015) 145:334–46. doi: 10.1111/imm.12469
154. Steiniger BS, Ulrich C, Berthold M, Guthe M, Lobachev O. Capillary networks and follicular marginal zones in human spleens. three-dimensional models based on immunostained serial sections. PLoS ONE. (2018) 13:e0191019. doi: 10.1371/journal.pone.0191019
Keywords: Conventional dendritic cells, cross-presentation, dendritic cell subsets, immunotherapy, macrophages, marginal zone, plasmacytoid dendritic cells, spleen
Citation: Backer RA, Diener N and Clausen BE (2019) Langerin+CD8+ Dendritic Cells in the Splenic Marginal Zone: Not So Marginal After All. Front. Immunol. 10:741. doi: 10.3389/fimmu.2019.00741
Received: 12 October 2018; Accepted: 19 March 2019;
Published: 12 April 2019.
Edited by:
Silvia Beatriz Boscardin, University of São Paulo, BrazilReviewed by:
Kristian Michael Hargadon, Hampden–Sydney College, United StatesJoke M. M. Den Haan, VU University Medical Center, Netherlands
Copyright © 2019 Backer, Diener and Clausen. This is an open-access article distributed under the terms of the Creative Commons Attribution License (CC BY). The use, distribution or reproduction in other forums is permitted, provided the original author(s) and the copyright owner(s) are credited and that the original publication in this journal is cited, in accordance with accepted academic practice. No use, distribution or reproduction is permitted which does not comply with these terms.
*Correspondence: Ronald A. Backer, ci5iYWNrZXJAdW5pLW1haW56LmRl
Björn E. Clausen, YmNsYXVzZW5AdW5pLW1haW56LmRl