- 1Laboratório de Glicobiologia, Instituto de Biofísica Carlos Chagas Filho, Universidade Federal do Rio de Janeiro, Rio de Janeiro, Brazil
- 2Laboratório de Imunomodulação, Instituto de Biofísica Carlos Chagas Filho, Universidade Federal do Rio de Janeiro, Rio de Janeiro, Brazil
- 3Laboratório de Pesquisa em Tuberculose, Faculdade de Medicina, Universidade Federal do Rio de Janeiro, Rio de Janeiro, Brazil
- 4Laboratório de Imunoparasitologia, Fundação Oswaldo Cruz, Rio de Janeiro, Brazil
The last decades have produced a plethora of evidence on the role of glycans, from cell adhesion to signaling pathways. Much of that information pertains to their role on the immune system and their importance on the surface of many human pathogens. A clear example of this is the flagellated protozoan Trypanosoma cruzi, which displays on its surface a great variety of glycoconjugates, including O-glycosylated mucin-like glycoproteins, as well as multiple glycan-binding proteins belonging to the trans-sialidase (TS) family. Among the latter, different and concurrently expressed molecules may present or not TS activity, and are accordingly known as active (aTS) and inactive (iTS) members. Over the last thirty years, it has been well described that T. cruzi is unable to synthesize sialic acid (SIA) on its own, making use of aTS to steal the host's SIA. Although iTS did not show enzymatic activity, it retains a substrate specificity similar to aTS (α-2,3 SIA-containing glycotopes), displaying lectinic properties. It is accepted that aTS members act as virulence factors in mammals coursing the acute phase of the T. cruzi infection. However, recent findings have demonstrated that iTS may also play a pathogenic role during T. cruzi infection, since it modulates events related to adhesion and invasion of the parasite into the host cells. Since both aTS and iTS proteins share structural substrate specificity, it might be plausible to speculate that iTS proteins are able to assuage and/or attenuate biological phenomena depending on the catalytic activity displayed by aTS members. Since SIA-containing glycotopes modulate the host immune system, it should not come as any surprise that changes in the sialylation of parasite's mucin-like molecules, as well as host cell glycoconjugates might disrupt critical physiological events, such as the building of effective immune responses. This review aims to discuss the importance of mucin-like glycoproteins and both aTS and iTS for T. cruzi biology, as well as to present a snapshot of how disturbances in both parasite and host cell sialoglycophenotypes may facilitate the persistence of T. cruzi in the infected mammalian host.
A Snapshot of the Nature of Trypanosoma cruzi Surface Coat
Trypanosoma cruzi presents a complex life cycle spanning two hosts, the hematophagous triatomine, and susceptible mammals (1). Throughout evolution, T. cruzi developed the capacity to adapt to hostile environments in both kinds of hosts. An important feature that was certainly decisive for the parasite adaptation to different hosts, as well as different niches within each host, was its ability to remodel its own surface coat (2, 3). It is well established that the cell surface of T. cruzi is composed by a wide variety of glycosylphosphatidylinositol (GPI)-anchored glycoconjugates expressed on a developmental stage-specific manner[(4–7).
Regarding the cell coat of the T. cruzi forms found in mammals, several studies revealed that it is mainly composed by both glycoinositolphospholipids (GIPLs) and heavily O-glycosylated mucin-like molecules (8, 9).
In addition, proteins belonging to trans-sialidase (TS) family (10–14); trypomastigote small surface antigen (TSSA) (15–17) and members of a multigenic family identified during the sequencing of the T. cruzi CL Brener genome, named mucin-associated surface proteins (MASPs) are found to a lesser extent (18–22).
Sialic Acid-Containing Glycans Modulate the Establishment of T. cruzi Infection in Mammals' Cells
Over the last twenty years, it has been known that simple, as well as complex carbohydrates (glycans) may play major structural, physical and metabolic roles in biological systems (23). Such functions include self/non-self-discrimination, ensuring correct protein folding, cell-to-cell signaling, cell adhesion and even differentiation, among others (24–27). The immune system, akin to the legions protecting the Roman Empire, is poised to defend the body against pathogens and transformed cells alike. One of the most important carbohydrates when it comes to the immune system is sialic acid (SIA) (28–30). More specifically the N-acetyl neuraminic acid (Neu5Ac). Immune responses deflagrated against T. cruzi are of particular interest, since the parasite is incapable of synthesizing SIA (31, 32). That would put T. cruzi squarely in the crosshairs of their mammal hosts' immune systems, since they somewhat rely on SIA to identify pathogens (3, 33, 34). The use of TS provides an elegant mechanism through which T. cruzi poaches SIA molecules from the hosts' cells and covers its own surface molecules, effectively creating a molecular ghillie suit to hide from mammalian phagocytes, posing a difficulty for the generation of an effective immune response (35–37). In addition to the enzymatically active members (aTS), which are able to modify the glycophenotype of both parasite and host cells (3, 13, 38, 39), TS also presents an inactive form (iTS), due to the naturally occurring Tyr342 → His substitution, which completely abolishes TS enzymatic activity (40). Despite the lack of catalytic function, it still plays an important role in T. cruzi-host cell interaction due to its lectinic activity (41–45) (Figure 1). Both extracellular (axenic) amastigote and trypomastigote forms of T. cruzi are infective to mammal cells (46–48). Regarding the trypomastigote forms, both iTS and aTS are GPI-anchored surface proteins (49). Recent findings revealed that sialylated mucins are present in lipid-raft-domains far away from TS molecules are found. By using unnatural sugar approach as chemical reporters, the authors demonstrated that the sialylation event is orchestrated by micro-vesicle-associated aTS instead of a membrane-anchored or fully soluble enzyme (34).
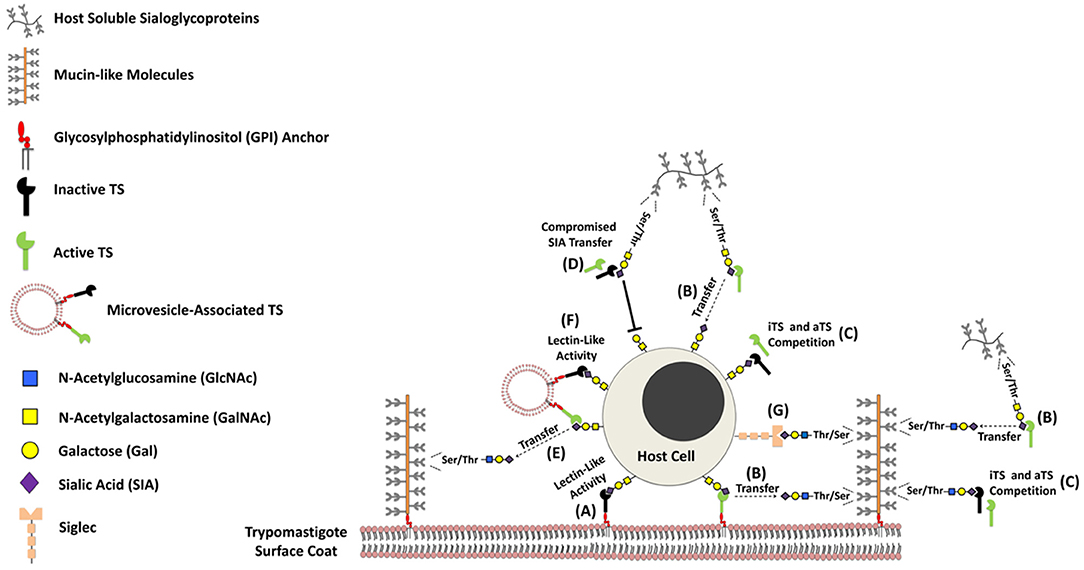
Figure 1. Schematic model showing the presence of trans-sialidases and mucin-like molecules on the parasite cell surface. The biological properties of both GPI-anchored proteins (trans-sialidases [TS] and mucin-like molecules) have been extensively studied over the last years, and their immunobiological functions have been gradually disclosed. Trypanosoma cruzi expresses on its surface both inactive (iTS) and active (aTS) TS proteins, that present similar substrate specificity (α-2,3 SIA). While iTS displays lectinic-like activity (A), aTS shows the ability to modulate the sialoglycophenotype of both parasite and host cell glycans (B). Since both TS proteins compete by α-2,3 sialo-containing glycans (C), it may attenuate and or abrogate the process of SIA transfer mediated by aTS (D). Consequently, it might be able to compromise biological phenomena depend on the catalytic activity displayed by enzymatically active members. In addition, both TS may be found associated to microvisicles, displaying the same properties mediated by both fully soluble enzyme (E, F). The sialylation of glycoproteins found in the parasite cell surface besides to promote protection against soluble factors of the host immune system, may also provide ligand for SIA-binding proteins expressed by host cells, such as Siglecs (G). Since this phenomenon compromises the effective function of immune cells, it may represent an interesting mechanism to guarantee the perpetuation of the parasite in their infected host.
The importance of SIA-containing glycans on T. cruzi-host cell interplay was suggested over twenty-five years ago, when the authors demonstrated that the parasite's ability to penetrate into SIA-deficient cells was reduced when compared with wild-type cell lines (50). After this finding, many groups began investigating the events triggered by TS in vitro and in murine models (3, 37, 51–53).
trans-Sialidases as Key Regulators of the Immune Evasion
Studies have shown that T. cruzi can recapitulate transient thymic aplasia in infected mice. It occurs in an early moment of the infection and aTS was proven responsible for the induction of apoptosis, since recombinant aTS alone can induce the alterations. In other studies, neutralizing anti-TS antibodies and the use of inhibitors prevented these effects (54). Also, an earlier study showed that recombinant iTS was incapable of eliciting these abnormalities (55). A study from Risso and colleagues demonstrated that the level of thymic damage was dependent on the parasite strain. More lethal strains (TcVI: RA, Q501, Cvd, and TcII: Br) present markedly higher levels of TS than their non-lethal counterparts (K-98, Ac and Hc - TcI) (56, 57). A different study showed that aTS does not appear to provoke thymocyte apoptosis directly. Instead, such effect seems to be centered on the thymic nurse cell complex, a region of the thymus cortex that contains mainly double-positive thymocytes, the most affected by TS (58). It is interesting to point out the studies that showed the pro-apoptotic effect was due to the alteration of the sialylation profile of target cells. By using lactitol, a competitive inhibitor that compromises the transfer of the sialyl residue to endogenous acceptors, but not the hydrolase activity of the enzyme, disallowed ex vivo and in vivo apoptosis caused by aTS (54). Years later, Lepletier and colleagues proposed that the apoptosis provoked by TS activity might also be capable of provoking an imbalance in the hypothalamus-pituitary-adrenal axis of T. cruzi-infected mice, leading to increased release of glucocorticoids, notorious immunossuppressants (59).
Early studies in the 90's already provided evidence of how aTS modulates the host immune system. Chuenkova and Pereira demonstrated that sensitizing mice with TS from conditioned supernatants, as well as recombinant aTS lead to higher parasitemia levels, and increased mortality rates. They also proposed that since animals with severe combined immunodeficiency, which lack functional T and B lymphocytes, were not affected. The logical conclusion was that TS was somehow affecting essential effector components of the adaptive immune system (60).
T lymphocytes must be activated to build up an effective response against invading organisms (61). This process involves loss of SIA residues in α-2,3 bonds from O-linked oligosaccharides, exposing free β-1,3 galactose (Gal) residues (62, 63). Such residues can be detected by the use of Peanut agglutinin lectin (PNA), which binds to terminal nonreducing Galβ1,3-GalNAc containing-sequences (64). That said T. cruzi's flagship enzyme unique ability to transfer SIA residues springs to mind as the perfect candidate to interfere with this process. Our group demonstrated this by showing that in a TS-free infection, i.e., Plasmodium berghei-infected mice, activated CD8+ T cells exhibited a great number of terminal β-Gal residues, while in the presence of aTS, such residues were re-sialylated (37) (Figure 1). While further investigation is necessary, it is safe to say that such an effect would be a great help to the parasite, as dampening the cellular response, would help ensure the protozoa's survival within the host. Further evidence of that statement is found in the work of Pereira-Chioccola et al. (65). The authors describe how anti-alpha-Gal antibodies, purified from chronic Chagas disease patients, strongly bind to α-Gal terminals in mucins, causing severe structural perturbations that lead to parasite lysis, while sialylation by TS activity diminishes the damage. The authors proposed that the negative charge provided by SIA helps stabilizing the T. cruzi surface coat by electrostatic repulsion (65).
Although it has been known for more than twenty years that both iTS and aTS have almost identical structures and compete for the same substrate (40, 42, 44), little is known about the biological effects triggered by iTS during T. cruzi infection.
In an interesting report, Pascuale et al. (45) demonstrated that the expression of iTS gene in iTS-null parasites was able to improve T. cruzi invasion into Vero cells and increased their in vivo virulence as shown by histopathologic findings in skeletal muscle and heart tissue of T. cruzi-infected mice (45). Although the molecular mechanisms have not been elucidated, the authors claim that iTS might play a different or complementary pathogenic role to aTS (45). Recently, our group demonstrated that mice treated with an elevated (non-physiological) concentration of recombinant iTS showed a compromise of T cells homing to the cardiac tissue during T. cruzi-infection (44). Since iTS is capable of recognizing SIA-containing glycans, which are carried by many glycoproteins involved in leukocyte extravasation through activated venular walls (66–68) it would be plausible to speculate that iTS, through its lectinic property, may bind to sialylated peripheral homing receptors, impairing the homing of inflammatory cells to the target tissues. The poor development of genetic tools to directly dissect the biological roles displayed by either iTS or aTS, leads researchers towards alternative approaches for this technical deadlock. The use of both recombinant T. cruzi-iTS and aTS, separately or together, may provide a good way for studying the effects triggered by both TS proteins (44). Over the last fifteen years, studies demonstrated that when administered separately, both iTS and aTS elicit similar biological effects (42, 69, 70). However, until recently, there was no published data showing their combined effects. Immunological studies carried out by our group revealed that in T. cruzi-infected mice, the intravenous administration of high concentrations of recombinant aTS was able to modulate the expression of inflammatory signals by splenic T cells (44). Nevertheless, when both recombinant iTS and aTS were injected in equivalent amounts, such phenomena were significantly compromised (44). Additional studies are necessary to confirm our previous findings, however, it is plausible to speculate that when present in a soluble form and/or associated to microvesicles (34), iTS may compete with aTS by the same SIA-containing glycotopes and attenuate/abrogate biological events depending of the addition and/or removal of SIA residues.
Another question that needs addressing is the degree to which iTS is able to attenuate or abrogate biological events induced by aTS. In 2010, Freire-de-Lima and colleagues demonstrated that CD8+ T cells from T. cruzi-infected mice treated with a high concentration of recombinant iTS, became positive for PNA. These results reinforce the idea that iTS competes with aTS for SIA-containing glycotopes, then compromising an expected re-sialylation phenomenon that naturally happens during T. cruzi infection (37).
Trypanosoma cruzi Mucins
Trypanosoma cruzi mucins are the parasite's most abundant surface glycoproteins. First described by Alves and Colli in epimastigotes, these highly glycosylated GPI-anchored mucin-like proteins were named A, B, and C glycoproteins (71). These proteins display a great deal of heterogeneity, with the genes responsible for encoding them being divided into two major families (3, 9, 72–74). The T. cruzi small mucin gene (TcSMUG) family encodes proteins that are expressed in the insect stages of the parasite's life, being essential to the infectivity on the insect host (75), while the TcMUC family, comprising from five to seven hundred genes, encodes the proteins expressed in the mammalian host. These proteins contain well-conserved N- and C-terminal regions, corresponding to ER and GPI anchor signals, respectively (72, 74, 76). This family can be further divided into three groups: (i) TcMUC I possesses a central domain with tandem repeats, with consensus sequences for O-glycosylation sites and it is more expressed in amastigotes (72, 73, 77); TcMUC II, found in trypomastigotes, displays a smaller number of repeats but is rich in serine and threonine residues (9, 72–74). Finally, TcMUCIII refers solely to the expression of a small surface protein, TSSA, or trypomastigote small surface antigen, being expressed only on cell-derived trypomastigotes (15). These mucin-like molecules contain a great number of O-linked oligosaccharides that are the main acceptors of SIA in the parasite's surface (Figure 1) (78–81). Unlike the classical vertebrate mucins, these oligosaccharides are linked to the protein core through α-GlcNAc residues, instead of α-GalNAc (82). Regardless, they contain a great number of free terminal β-Gal residues, which serve as ideal SIA acceptors (7, 78–81) (Figure 1). The O-linked oligosaccharides composition and size vary depending both the parasite strain (9, 78–80, 83–85) and its sialylation might promote immunosuppressive properties (please, see below).
The GPI-mucins expressed by T. cruzi, also known as sialoglycoproteins, are mucin-like molecules that are highly glycosylated and present a conserved GPI-anchor linked to the parasite cell surface (9, 80–87). All mucin GPI-anchors are constituted by a similar glycan core (Manα1-2Manα1-2Manα1-6Manα1-4GlcN) (9, 80, 85, 87). Except for the cell-derived trypomastigotes, where a branch of Gal residues can modify the GPI anchor (9, 84). The GPI-mucin lipid anchor differs according to the parasite's stage (80, 81, 85). In non-infective insect-derived epimastigotes, they are composed of saturated fatty acids; in metacyclic trypomastigotes, they are mainly inositol-phosphoceramides, and in the cell-derived trypomastigotes, they are composed wholly of alkylacyl-phosphatidylinositol (PI) structures, frequently insaturated (C18:1 or C18:2) (84, 85).
There is abundant data showing that following the early stages of T. cruzi infection, the patterns of resistance or susceptibility may be determined before adaptive immunity elements have a chance to respond, with components of the innate immune response playing crucial roles for parasite control (88). T. cruzi makes use of an expanded array of molecular strategies to invade an extensive range of host cells, as well as to avoid the host's immune defense. In the infection site, T. cruzi triggers the production of chemokines and pro-inflammatory cytokines, such as interleukin-12 (IL-12) and tumor necrosis factor-a (TNF-α), and the highly reactive oxygen and nitrogen species produced by cells of the Mϕ lineage (84, 85, 89–91). Over the last fifteen years, it has been described that GPI anchors expressed in the surface of T. cruzi are determinant in this process (85, 92, 93). In 2006, Bafica and colleagues demonstrated that the activation of innate immune response by T. cruzi-derived DNA and GPI anchors from trypomastigote mucins (tGPI-mucins anchors) forms, was able to promote the production of proinflammatory signals (84, 94). The authors revealed that the parasite's DNA stimulates cytokine production by Mϕ in a Toll-Like Receptor-9 (TLR9) dependent mechanism, and synergizes with parasite-derived tGPI-mucins, a TLR2 agonist, in the induction of IL-12 and TNF-α (94). More recently, it has been demonstrated that both living T. cruzi trypomastigote forms, as well as tGPI-mucins are able to induce high levels of IL-12 by human monocytes. Additionally, it has been proven that such effect depends on CD40-CD40L interaction and IFN-γ (95). In that work the authors claim that the polarized T1-type cytokine profile observed in T. cruzi-infected individuals might be a long-term effect of IL-12 production induced by lifelong exposure to T. cruzi tGPI-mucins (95).
It is well accepted that a great array of GPI-mucin genes is responsible for the variability of parasite cell surface (2). In 2004, an interesting work carried out by Buscaglia and collaborators demonstrated that the vast majority of the tGPI-mucin molecules found on the surface of the cell-derived trypomastigotes belong to the TcMUC II group. In this study, for the first time, the authors presented high evidence that multiple products of TcMUC II are concurrently expressed, suggesting that such molecules might represent a sophisticated strategy for the parasite to dampen the host immune response (9).
In 2002, Argibay and co-authors transfected higher eukaryotic cells (Vero cells) with TCMuc-e2 gene, which encodes for a mucin that is expressed in the blood-circulating stage of the parasite. The authors demonstrated that when transfected cells were exposed to human lymphocytes, an event of T cell anergy was observed. In this study, it was also demonstrated that the effect could be reversed by the addition of exogenous IL-2 (35). A different study discussed the effect of the interaction between the T. cruzi AgC10, a mucin-like molecule expressed by metacyclic trypomastigotes, as well as on amastigotes (96) and L-selectin in T cell surface. In an event independent of IFN-γ and nitric oxide, it was capable of inhibiting T cell proliferation and IL-2 secretion, as well as impairing IL-2 mRNA expression in response to mitogens. In fact, most genes whose expression is controlled by NFAT (Nuclear Factor of Activated T-cells) were affected and the overexpression of NFAT refuted the effects mediated by the parasite's glycoprotein (97).
The carbohydrate chains of mucin molecules are usually long extended structures (98). Over the last ten years has been demonstrated that the O-linked oligosaccharides composition of T. cruzi mucin-like molecules might exert direct effect on the host immune system. Since epimastigote forms are easier to be cultured in vitro, most of the studies investigating the biological roles triggered by T. cruzi O-linked glycans have been performed with non-infective forms for mammal cells. In 2013, Nunes and colleagues showed that a purified preparation of sialylated T. cruzi glycoproteins is capable of inhibiting clonal expansion as well as cytokine production by CD4+ lymphocytes. This happens through cell cycle arrest in the G1 phase and cannot be reversed by administration of exogenous IL-2, effectively rendering the cells anergic when stimulated through the T cell receptor (TCR) (99). The authors suggested that the starting point of this effect would be the interaction between the sialylated parasite mucins and Siglecs expressed on the T cell surface (Figure 1). An earlier study might substantiate this claim. Erdhmann and co-workers showed that the highly virulent T. cruzi Tulahuén strain was able to modulate the functionality of dendritic cells, through the interaction of its sialylated mucins with Siglec-E. The authors also confirmed that the desialylation of the parasite's surface molecules prevents such event (100).
Possible Therapeutic Targets
The mucin-like proteins present in the surface of T. cruzi bear a distinct characteristic when compared to mucins or any other O-glycosylated protein on the surface of human proteins: the presence of galactofuranose (Galf ) residues (79). The flavoenzyme UDP-galactopyranose mutase (UMG) is not found in humans, but is essential to the composition of bacterial and fungal cell walls, as well as an important virulence factor for protozoa (6, 101, 102). A study in the late 80's even managed to show that anti-galactofuranose antibodies lead to a 70% inhibition of cell invasion (103). It should not come as a surprise that some groups treat UMG as an ideal therapeutic target, since the enzyme is not present in humans, and are working towards the development of UMG inhibitors (104–106). One study shows promise in halting the growth of some Mycobacterium species (107). It is important to note that this strategy suffers from a fundamental problem in the fact that so far Galf residues have not been found in the mucins expressed in the mammalian host stages'. The presence of Galf residues in metacyclics has been demonstrated (81).
trans-Sialidases also comes off as a potential drug target for the treatment or prevention of Chagas disease, and as such, many groups have been pursuing different strategies focused on TS as a target for either therapeutic or prophylactic methods. Good examples of this are recombinant proteins and DNA vaccines (108–111). Despite early reports showing that immunization with TS inhibits Th1 immune response (70), it was recently demonstrated that such a response can be elicited by the clever use of adjuvants (112). The same group has also shown that using the same model, aTS elicits stronger humoral and cellular responses than other T. cruzi antigens (113). Over the last decade, works from many research groups have demonstrated that vaccines candidates based on TS proteins are capable of protecting T. cruzi-infected mice (111, 114–118). Groundbreaking studies carried out by Rodrigues and Tarleton groups (119–122) have demonstrated that immunodominant CD8+ T cell immune responses directed to epitopes expressed by members of the TS family contribute to control T. cruzi infection, suggesting that non-antibody mediated cellular immune responses to the antigens expressed in the mammalian forms of T. cruzi might be used for the purpose of vaccination. In 2015, Pereira and collaborators started the development of both prophylactic and therapeutic vaccine protocols. The vaccines take advantage of the immunostimulation provided by a replication-defective human Type 5 recombinant adenoviruses (rAd) vector carrying sequences of amastigote surface protein-2 (rAdASP2), and TS (rAdTS). This strategy, rather offers a rational approach for re-programming the host immunity, achieving a more protective profile, leading to interruption of damage and even tissue recovery, particularly when it comes to chronic Chagas heart disease (123).
Another important focus field concerning T. cruzi TS is the search for effective inhibitors. A di-sialylated N-lactoside compound was shown to promote a 70% inhibition of TS activity through a competition mechanism (124). Sulfasalazine, a first line sulfa drug for rheumatoid arthritis, is also a moderate TS inhibitor. Although it does not lead to a great inhibition of the enzyme activity and it is not particularly toxic to the parasite strains tested by Lara-Ramirez's group, it is a good starting point for the development of new drugs, especially because sulfasalazine has been in use since the early 50s (125).
Several other researches have reported results on promising drugs, from competitive to non-competitive inhibitors, acting through reversible or irreversible mechanisms, some of those reaching up to 50% inhibition in the millimolar range (126–130).
An earlier work from our group has shown that 2-difluoromethyl-4-nitrophenyl-3,5-dideoxy-D-glycero-α-D-galacto-2-nonulopyranosid acid (NeuNAcFNP) is able to irreversibly inhibit TS in a time and dose-dependant manner. More importantly, it is able to produce a 90% inhibition of the infection of LLC-MK2 cells by T. cruzi Y strain trypomastigotes (131). Although it provides a unique form of inhibition and a chance for less major adverse effects, especially since TS bears no semblance with any human enzyme (132).
Conclusion
In this review, we focused on the role of T. cruzi glycoconjugates and associated proteins in mediating the relationship between parasite and the human immune system. Throughout the years, several discoveries illustrated how TS, Tc-mucins and SIA are fundamental for the parasite to not only survive, but also thrive in an inhospitable environment like the human body. Mounds of evidence sustain the idea that TS is an important virulence factor, especially during the acute phase of the disease and is pivotal in aiding the parasite in bypassing the immune system. Authors also agree on the fact that mucins are major players in the balance between immune response and parasite survival, especially since it is the primary SIA acceptor in the protozoan membrane.
It is our belief that a better understanding of how T. cruzi is able to sabotage the human immune response will provide us with more effective tools to prevent and combat infections. Moreover, the parasite's unique system of handling SIA is almost certainly pivotal, since it involves a one-of-a-kind enzyme and an equally unique group of mucin-like proteins.
Author Contributions
LF, KdC, VC, CF-d-L, AM, LM-P, JP, and LF-d-L participated in the writing of the paper.
Funding
The work was supported by Conselho Nacional de Desenvolvimento Científico e Tecnológico (CNPq); Fundação de Amparo à Pesquisa do Estado do Rio de Janeiro (FAPERJ), and Coordenação de Aperfeiçoamento de Pessoal de Nível Superior (CAPES).
Conflict of Interest Statement
The authors declare that the research was conducted in the absence of any commercial or financial relationships that could be construed as a potential conflict of interest.
References
1. De Souza W. Basic cell biology of Trypanosoma cruzi. Curr Pharm Des. (2002) 8:269–85. doi: 10.2174/1381612023396276
2. Buscaglia CA, Campo VA, Frasch AC, Di Noia JM. Trypanosoma cruzi surface mucins: host-dependent coat diversity. Nat Rev Microbiol. (2006) 4:229–36. doi: 10.1038/nrmicro1351
3. Mucci J, Lantos AB, Buscaglia CA, Leguizamon MS, Campetella O. The Trypanosoma cruzi surface, a nanoscale patchwork quilt. Trends Parasitol. (2017) 33:102–12. doi: 10.1016/j.pt.2016.10.004
4. McConville MJ, Ferguson MA. The structure, biosynthesis and function of glycosylated phosphatidylinositols in the parasitic protozoa and higher eukaryotes. Biochem J. (1993) 294 (Pt. 2):305–24. doi: 10.1042/bj2940305
5. Previato JO, Wait R, Jones C, DosReis GA, Todeschini AR, Heise N, et al. Glycoinositolphospholipid from Trypanosoma cruzi: structure, biosynthesis and immunobiology. Adv Parasitol. (2004) 56:1–41. doi: 10.1016/S0065-308X(03)56001-8
6. de Lederkremer RM, Agusti R. Glycobiology of Trypanosoma cruzi. Adv Carbohydr Chem Biochem. (2009) 62:311–66. doi: 10.1016/S0065-2318(09)00007-9
7. Mendonca-Previato L, Penha L, Garcez TC, Jones C, Previato JO. Addition of alpha-O-GlcNAc to threonine residues define the post-translational modification of mucin-like molecules in Trypanosoma cruzi. Glycoconj J. (2013) 30:659–66. doi: 10.1007/s10719-013-9469-7
8. Almeida IC, Ferguson MA, Schenkman S, Travassos LR. Lytic anti-alpha-galactosyl antibodies from patients with chronic Chagas' disease recognize novel O-linked oligosaccharides on mucin-like glycosyl-phosphatidylinositol-anchored glycoproteins of Trypanosoma cruzi. Biochem J. (1994) 304 (Pt. 3):793–802. doi: 10.1042/bj3040793
9. Buscaglia CA, Campo VA, Di Noia JM, Torrecilhas AC, De Marchi CR, Ferguson MA, et al. The surface coat of the mammal-dwelling infective trypomastigote stage of Trypanosoma cruzi is formed by highly diverse immunogenic mucins. J Biol Chem. (2004) 279:15860–9. doi: 10.1074/jbc.M314051200
10. Colli W. Trans-sialidase: a unique enzyme activity discovered in the protozoan Trypanosoma cruzi. FASEB J. (1993) 7:1257–64. doi: 10.1096/fasebj.7.13.8405811
11. Cross GA, Takle GB. The surface trans-sialidase family of Trypanosoma cruzi. Annu Rev Microbiol. (1993) 47:385–411. doi: 10.1146/annurev.mi.47.100193.002125
12. Frasch AC. Functional diversity in the trans-sialidase and mucin families in Trypanosoma cruzi. Parasitol Today (2000) 16:282–6. doi: 10.1016/S0169-4758(00)01698-7
13. Dc-Rubin SS, Schenkman S. T rypanosoma cruzi trans-sialidase as a multifunctional enzyme in Chagas' disease. Cell Microbiol. (2012) 14:1522–30. doi: 10.1111/j.1462-5822.2012.01831.x
14. Freire-de-Lima L, Fonseca LM, Oeltmann T, Mendonca-Previato L, Previato JO. The trans-sialidase, the major Trypanosoma cruzi virulence factor: three decades of studies. Glycobiology (2015) 25:1142–9. doi: 10.1093/glycob/cwv057
15. Di Noia JM, Buscaglia CA, De Marchi CR, Almeida IC, Frasch AC. A Trypanosoma cruzi small surface molecule provides the first immunological evidence that Chagas' disease is due to a single parasite lineage. J Exp Med. (2002) 195:401–13. doi: 10.1084/jem.20011433
16. Canepa GE, Degese MS, Budu A, Garcia CR, Buscaglia CA. Involvement of TSSA (trypomastigote small surface antigen) in Trypanosoma cruzi invasion of mammalian cells. Biochem J. (2012) 444:211–8. doi: 10.1042/BJ20120074
17. Camara MLM, Canepa GE, Lantos AB, Balouz V, Yu H, Chen X, et al. The Trypomastigote Small Surface Antigen (TSSA) regulates Trypanosoma cruzi infectivity and differentiation. PLoS Negl Trop Dis. (2017) 11:e0005856. doi: 10.1371/journal.pntd.0005856
18. El-Sayed NM, Myler PJ, Blandin G, Berriman M, Crabtree J, Aggarwal G, et al. Comparative genomics of trypanosomatid parasitic protozoa. Science (2005) 309:404–9. doi: 10.1126/science.1112181
19. Bartholomeu DC, Cerqueira GC, Leao AC, daRocha WD, Pais FS, Macedo C, et al. Genomic organization and expression profile of the mucin-associated surface protein (masp) family of the human pathogen Trypanosoma cruzi. Nucleic Acids Res. (2009) 37:3407–17. doi: 10.1093/nar/gkp172
20. De Pablos LM, Gonzalez GG, Solano Parada J, Seco Hidalgo V, Diaz Lozano IM, Gomez Samblas MM, et al. Differential expression and characterization of a member of the mucin-associated surface protein family secreted by Trypanosoma cruzi. Infect Immun. (2011) 79:3993–4001. doi: 10.1128/IAI.05329-11
21. De Pablos LM, Osuna A. Multigene families in Trypanosoma cruzi and their role in infectivity. Infect Immun. (2012) 80:2258–64. doi: 10.1128/IAI.06225-11
22. Alves MJ, Kawahara R, Viner R, Colli W, Mattos EC, Thaysen-Andersen M, et al. Comprehensive glycoprofiling of the epimastigote and trypomastigote stages of Trypanosoma cruzi. J Proteomics (2017) 151:182–92. doi: 10.1016/j.jprot.2016.05.034
23. Varki A. Biological roles of oligosaccharides: all of the theories are correct. Glycobiology (1993) 3:97–130. doi: 10.1093/glycob/3.2.97
24. Macauley MS, Crocker PR, Paulson JC. Siglec-mediated regulation of immune cell function in disease. Nat Rev Immunol. (2014) 14:653–66. doi: 10.1038/nri3737
26. Vasta GR, Amzel LM, Bianchet MA, Cammarata M, Feng C, Saito K. F-type lectins: a highly diversified family of fucose-binding proteins with a unique sequence motif and structural fold, involved in self/non-self-recognition. Front Immunol. (2017) 8:1648. doi: 10.3389/fimmu.2017.01648
27. Wilson KM, Jagger AM, Walker M, Seinkmane E, Fox JM, Kroger R, et al. Glycans modify mesenchymal stem cell differentiation to impact on the function of resulting osteoblasts. J Cell Sci. (2018) 131:jcs209452. doi: 10.1242/jcs.209452
28. Kelm S, Schauer R, Crocker PR. The Sialoadhesins–a family of sialic acid-dependent cellular recognition molecules within the immunoglobulin superfamily. Glycoconj J. (1996) 13:913–26. doi: 10.1007/BF01053186
29. Varki A, Gagneux P. Multifarious roles of sialic acids in immunity. Ann N Y Acad Sci. (2012) 1253:16–36. doi: 10.1111/j.1749-6632.2012.06517.x
30. Fraschilla I, Pillai S. Viewing siglecs through the lens of tumor immunology. Immunol Rev. (2017) 276:178–91. doi: 10.1111/imr.12526
31. Schauer R, Reuter G, Muhlpfordt H, Andrade AF, Pereira ME. The occurrence of N-acetyl- and N-glycoloylneuraminic acid in Trypanosoma cruzi. Hoppe Seylers Z Physiol Chem. (1983) 364:1053–7. doi: 10.1515/bchm2.1983.364.2.1053
32. Previato JO, Andrade AF, Pessolani MC, Mendonca-Previato L. Incorporation of sialic acid into Trypanosoma cruzi macromolecules. A proposal for a new metabolic route. Mol Biochem Parasitol. (1985) 16:85–96. doi: 10.1016/0166-6851(85)90051-9
33. Freire-de-Lima L, da Fonseca LM, da Silva VA, da Costa KM, Morrot A, Freire-de-Lima CG, et al. Modulation of cell sialoglycophenotype: a stylish mechanism adopted by Trypanosoma cruzi to ensure its persistence in the infected host. Front Microbiol. (2016) 7:698. doi: 10.3389/fmicb.2016.00698
34. Lantos AB, Carlevaro G, Araoz B, Ruiz Diaz P, Camara Mde L, Buscaglia CA, et al. Sialic acid glycobiology unveils Trypanosoma cruzi trypomastigote membrane physiology. PLoS Pathog. (2016) 12:e1005559. doi: 10.1371/journal.ppat.1005559
35. Argibay PF, Di Noia JM, Hidalgo A, Mocetti E, Barbich M, Lorenti AS, et al. Trypanosoma cruzi surface mucin TcMuc-e2 expressed on higher eukaryotic cells induces human T cell anergy, which is reversible. Glycobiology (2002) 12:25–32. doi: 10.1093/glycob/12.1.25
36. Gao W, Wortis HH, Pereira MA. The Trypanosoma cruzi trans-sialidase is a T cell-independent B cell mitogen and an inducer of non-specific Ig secretion. Int Immunol. (2002) 14:299–308. doi: 10.1093/intimm/14.3.299
37. Freire-de-Lima L, Alisson-Silva F, Carvalho ST, Takiya CM, Rodrigues MM, DosReis GA, et al. Trypanosoma cruzi subverts host cell sialylation and may compromise antigen-specific CD8+ T cell responses. J Biol Chem. (2010) 285:13388–96. doi: 10.1074/jbc.M109.096305
38. Tribulatti MV, Mucci J, Van Rooijen N, Leguizamon MS, Campetella O. The trans-sialidase from Trypanosoma cruzi induces thrombocytopenia during acute Chagas' disease by reducing the platelet sialic acid contents. Infect Immun. (2005) 73:201–7. doi: 10.1128/IAI.73.1.201-207.2005
39. Freire-de-Lima L, Oliveira IA, Neves JL, Penha LL, Alisson-Silva F, Dias WB, et al. Sialic acid: a sweet swing between mammalian host and Trypanosoma cruzi. Front Immunol. (2012) 3:356. doi: 10.3389/fimmu.2012.00356
40. Cremona ML, Sanchez DO, Frasch AC, Campetella O. A single tyrosine differentiates active and inactive Trypanosoma cruzi trans-sialidases. Gene (1995) 160:123–8. doi: 10.1016/0378-1119(95)00175-6
41. Cremona ML, Campetella O, Sanchez DO, Frasch AC. Enzymically inactive members of the trans-sialidase family from Trypanosoma cruzi display beta-galactose binding activity. Glycobiology (1999) 9:581–7. doi: 10.1093/glycob/9.6.581
42. Todeschini AR, Girard MF, Wieruszeski JM, Nunes MP, DosReis GA, Mendonca-Previato L, et al. Trans-Sialidase from Trypanosoma cruzi binds host T-lymphocytes in a lectin manner. J Biol Chem. (2002) 277:45962–8. doi: 10.1074/jbc.M203185200
43. Todeschini AR, Dias WB, Girard MF, Wieruszeski JM, Mendonca-Previato L, Previato JO. Enzymatically inactive trans-sialidase from Trypanosoma cruzi binds sialyl and beta-galactopyranosyl residues in a sequential ordered mechanism. J Biol Chem. (2004) 279:5323–8. doi: 10.1074/jbc.M310663200
44. Freire-de-Lima L, Gentile LB, da Fonseca LM, da Costa KM, Santos Lemos J, Jacques LR, et al. Role of inactive and active Trypanosoma cruzi trans-sialidases on T cell homing and secretion of inflammatory cytokines. Front Microbiol. (2017) 8:1307. doi: 10.3389/fmicb.2017.01307
45. Pascuale CA, Burgos JM, Postan M, Lantos AB, Bertelli A, Campetella O, et al. Inactive trans- n iTS-null Trypanosoma cruzi generates virulent Trypomastigotes. Front Cell Infect Microbiol. (2017) 7:430. doi: 10.3389/fcimb.2017.00430
46. Mortara RA. Trypanosoma cruzi: amastigotes and trypomastigotes interact with different structures on the surface of HeLa cells. Exp Parasitol. (1991) 73:1–14. doi: 10.1016/0014-4894(91)90002-E
47. Mortara RA, Andreoli WK, Taniwaki NN, Fernandes AB, Silva CV, Fernandes MC, et al. Mammalian cell invasion and intracellular trafficking by Trypanosoma cruzi infective forms. An Acad Bras Cienc. (2005) 77:77–94. doi: 10.1590/S0001-37652005000100006
48. de Souza W, de Carvalho TM, Barrias ES. Review on Trypanosoma cruzi: host cell interaction. Int J Cell Biol. (2010) 2010:295394. doi: 10.1155/2010/295394
49. Agusti R, Couto AS, Campetella OE, Frasch AC, de Lederkremer RM. The trans-sialidase of Trypanosoma cruzi is anchored by two different lipids. Glycobiology (1997) 7:731–5. doi: 10.1093/glycob/7.6.731
50. Schenkman RP, Vandekerckhove F, Schenkman S. Mammalian cell sialic acid enhances invasion by Trypanosoma cruzi. Infect Immun. (1993) 61:898–902.
51. Schenkman S, Eichinger D, Pereira ME, Nussenzweig V. Structural and functional properties of Trypanosoma trans-sialidase. Annu Rev Microbiol. (1994) 48:499–523. doi: 10.1146/annurev.mi.48.100194.002435
52. Woronowicz A, De Vusser K, Laroy W, Contreras R, Meakin SO, Ross GM, et al. Trypanosome trans-sialidase targets TrkA tyrosine kinase receptor and induces receptor internalization and activation. Glycobiology (2004) 14:987–98. doi: 10.1093/glycob/cwh123
53. Chuenkova MV, Pereiraperrin M. Trypanosoma cruzi-derived neurotrophic factor: role in neural repair and neuroprotection. J Neuroparasitol. (2010) 1:55–60. doi: 10.4303/jnp/N100507
54. Mucci J, Risso MG, Leguizamon MS, Frasch AC, Campetella O. The trans-sialidase from Trypanosoma cruzi triggers apoptosis by target cell sialylation. Cell Microbiol. (2006) 8:1086–95. doi: 10.1111/j.1462-5822.2006.00689.x
55. Leguizamon MS, Mocetti E, Garcia Rivello H, Argibay P, Campetella O. Trans-sialidase from Trypanosoma cruzi induces apoptosis in cells from the immune system in vivo. J Infect Dis. (1999) 180:1398–402. doi: 10.1086/315001
56. Risso MG, Garbarino GB, Mocetti E, Campetella O, Gonzalez Cappa SM, Buscaglia CA, et al. Differential expression of a virulence factor, the trans-sialidase, by the main Trypanosoma cruzi phylogenetic lineages. J Infect Dis. (2004) 189:2250–9. doi: 10.1086/420831
57. Burgos JM, Risso MG, Breniere SF, Barnabe C, Campetella O, Leguizamon MS. Differential distribution of genes encoding the virulence factor trans-sialidase along Trypanosoma cruzi Discrete typing units. PLoS ONE (2013) 8:e58967. doi: 10.1371/journal.pone.0058967
58. Mucci J, Hidalgo A, Mocetti E, Argibay PF, Leguizamon MS, Campetella O. Thymocyte depletion in Trypanosoma cruzi infection is mediated by trans-sialidase-induced apoptosis on nurse cells complex. Proc Natl Acad Sci USA. (2002) 99:3896–901. doi: 10.1073/pnas.052496399
59. Lepletier A, de Frias Carvalho V, Morrot A, Savino W. Thymic atrophy in acute experimental Chagas disease is associated with an imbalance of stress hormones. Ann NY Acad Sci. (2012) 1262:45–50. doi: 10.1111/j.1749-6632.2012.06601.x
60. Chuenkova M, Pereira ME. Trypanosoma cruzi trans-sialidase: enhancement of virulence in a murine model of Chagas' disease. J Exp Med. (1995) 181:1693–703. doi: 10.1084/jem.181.5.1693
61. Pennock ND, White JT, Cross EW, Cheney EE, Tamburini BA, Kedl RM. T cell responses: naive to memory and everything in between. Adv Physiol Educ. (2013) 37:273–83. doi: 10.1152/advan.00066.2013
62. Galvan M, Murali-Krishna K, Ming LL, Baum L, Ahmed R. Alterations in cell surface carbohydrates on T cells from virally infected mice can distinguish effector/memory CD8+ T cells from naive cells. J Immunol. (1998) 161:641–8.
63. Priatel JJ, Chui D, Hiraoka N, Simmons CJ, Richardson KB, Page DM, et al. The ST3Gal-I sialyltransferase controls CD8+ T lymphocyte homeostasis by modulating O-glycan biosynthesis. Immunity (2000) 12:273–83. doi: 10.1016/S1074-7613(00)80180-6
64. Wu W, Harley PH, Punt JA, Sharrow SO, Kearse KP. Identification of CD8 as a peanut agglutinin (PNA) receptor molecule on immature thymocytes. J Exp Med. (1996) 184:759–64. doi: 10.1084/jem.184.2.759
65. Pereira-Chioccola VL, Acosta-Serrano A, Correia de Almeida I, Ferguson MA, Souto-Padron T, Rodrigues MM, et al. Mucin-like molecules form a negatively charged coat that protects Trypanosoma cruzi trypomastigotes from killing by human anti-alpha-galactosyl antibodies. J Cell Sci. (2000) 113 (Pt. 7):1299–307.
66. McEvoy LM, Sun H, Frelinger JG, Butcher EC. Anti-CD43 inhibition of T cell homing. J Exp Med. (1997) 185:1493–8. doi: 10.1084/jem.185.8.1493
67. Frommhold D, Ludwig A, Bixel MG, Zarbock A, Babushkina I, Weissinger M, et al. Sialyltransferase ST3Gal-IV controls CXCR2-mediated firm leukocyte arrest during inflammation. J Exp Med. (2008) 205:1435–46. doi: 10.1084/jem.20070846
68. Sperandio M, Gleissner CA, Ley K. Glycosylation in immune cell trafficking. Immunol Rev. (2009) 230:97–113. doi: 10.1111/j.1600-065X.2009.00795.x
69. Todeschini AR, Nunes MP, Pires RS, Lopes MF, Previato JO, Mendonca-Previato L, et al. Costimulation of host T lymphocytes by a trypanosomal trans-sialidase: involvement of CD43 signaling. J Immunol. (2002) 168:5192–8. doi: 10.4049/jimmunol.168.10.5192
70. Ruiz Diaz P, Mucci J, Meira MA, Bogliotti Y, Musikant D, Leguizamon MS, et al. Trypanosoma cruzi trans-sialidase prevents elicitation of Th1 cell response via interleukin 10 and downregulates Th1 effector cells. Infect Immun. (2015) 83:2099–108. doi: 10.1128/IAI.00031-15
71. Alves MJ, Colli W. Glycoproteins from Trypanosoma cruzi: partial purification by gel chromatography. FEBS Lett. (1975) 52:188–90. doi: 10.1016/0014-5793(75)80803-9
72. Di Noia JM, Sanchez DO, Frasch AC. The protozoan Trypanosoma cruzi has a family of genes resembling the mucin genes of mammalian cells. J Biol Chem. (1995) 270:24146–9. doi: 10.1074/jbc.270.41.24146
73. Di Noia JM, Pollevick GD, Xavier MT, Previato JO, Mendoca-Previato L, Sanchez DO, et al. High diversity in mucin genes and mucin molecules in Trypanosoma cruzi. J Biol Chem. (1996) 271:32078–83. doi: 10.1074/jbc.271.50.32078
74. Pollevick GD, Di Noia JM, Salto ML, Lima C, Leguizamon MS, de Lederkremer RM, et al. Trypanosoma cruzi surface mucins with exposed variant epitopes. J Biol Chem. (2000) 275:27671–80. doi: 10.1074/jbc.M000253200
75. Gonzalez MS, Souza MS, Garcia ES, Nogueira NF, Mello CB, Canepa GE, et al. Trypanosoma cruzi TcSMUG L-surface mucins promote development and infectivity in the triatomine vector Rhodnius prolixus. PLoS Negl Trop Dis. (2013) 7:e2552. doi: 10.1371/journal.pntd.0002552
76. Freitas-Junior LH, Briones MR, Schenkman S. Two distinct groups of mucin-like genes are differentially expressed in the developmental stages of Trypanosoma cruzi. Mol Biochem Parasitol. (1998) 93:101–14. doi: 10.1016/S0166-6851(98)00025-5
77. Campo VA, Buscaglia CA, Di Noia JM, Frasch AC. Immunocharacterization of the mucin-type proteins from the intracellular stage of Trypanosoma cruzi. Microbes Infect. (2006) 8:401–9. doi: 10.1016/j.micinf.2005.07.008
78. Almeida IC, Ferguson MA, Schenkman S, Travassos LR. GPI-anchored glycoconjugates from Trypanosoma cruzi trypomastigotes are recognized by lytic anti-alpha-galactosyl antibodies isolated from patients with chronic Chagas' disease. Braz J Med Biol Res. (1994) 27:443–7.
79. Previato JO, Jones C, Goncalves LP, Wait R, Travassos LR, Mendonca-Previato L. O-glycosidically linked N-acetylglucosamine-bound oligosaccharides from glycoproteins of Trypanosoma cruzi. Biochem J. (1994) 301 (Pt. 1):151–9.
80. Previato JO, Jones C, Xavier MT, Wait R, Travassos LR, Parodi AJ, et al. Structural characterization of the major glycosylphosphatidylinositol membrane-anchored glycoprotein from epimastigote forms of Trypanosoma cruzi Y-strain. J Biol Chem. (1995) 270:7241–50. doi: 10.1074/jbc.270.13.7241
81. Serrano AA, Schenkman S, Yoshida N, Mehlert A, Richardson JM, Ferguson MA. The lipid structure of the glycosylphosphatidylinositol-anchored mucin-like sialic acid acceptors of Trypanosoma cruzi changes during parasite differentiation from epimastigotes to infective metacyclic trypomastigote forms. J Biol Chem. (1995) 270:27244–53. doi: 10.1074/jbc.270.45.27244
82. Previato JO, Sola-Penna M, Agrellos OA, Jones C, Oeltmann T, Travassos LR, et al. Biosynthesis of O-N-acetylglucosamine-linked glycans in Trypanosoma cruzi. Characterization of the novel uridine diphospho-N-acetylglucosamine:polypeptide N-acetylglucosaminyltransferase-catalyzing formation of N-acetylglucosamine alpha1–>O-threonine. J Biol Chem. (1998) 273:14982–8.
83. Schenkman S, Ferguson MA, Heise N, de Almeida ML, Mortara RA, Yoshida N. Mucin-like glycoproteins linked to the membrane by glycosylphosphatidylinositol anchor are the major acceptors of sialic acid in a reaction catalyzed by trans-sialidase in metacyclic forms of Trypanosoma cruzi. Mol Biochem Parasitol. (1993) 59:293–303. doi: 10.1016/0166-6851(93)90227-O
84. Almeida IC, Camargo MM, Procopio DO, Silva LS, Mehlert A, Travassos LR, et al. Highly purified glycosylphosphatidylinositols from Trypanosoma cruzi are potent proinflammatory agents. EMBO J. (2000) 19:1476–85. doi: 10.1093/emboj/19.7.1476
85. Almeida IC, Gazzinelli RT. Proinflammatory activity of glycosylphosphatidylinositol anchors derived from Trypanosoma cruzi: structural and functional analyses. J Leukoc Biol. (2001) 70:467–77. doi: 10.1189/jlb.70.4.467
86. Nakayasu ES, Yashunsky DV, Nohara LL, Torrecilhas AC, Nikolaev AV, Almeida IC. GPIomics: global analysis of glycosylphosphatidylinositol-anchored molecules of Trypanosoma cruzi. Mol Syst Biol. (2009) 5:261. doi: 10.1038/msb.2009.13
87. Soares RP, Torrecilhas AC, Assis RR, Rocha MN, Moura e Castro FA, Freitas GF, et al. Intraspecies variation in Trypanosoma cruzi GPI-mucins: biological activities and differential expression of alpha-galactosyl residues. Am J Trop Med Hyg. (2012) 87:87–96. doi: 10.4269/ajtmh.2012.12-0015
88. Tarleton RL. Immune system recognition of Trypanosoma cruzi. Curr Opin Immunol. (2007) 19:430–4. doi: 10.1016/j.coi.2007.06.003
89. Camargo MM, Almeida IC, Pereira ME, Ferguson MA, Travassos LR, Gazzinelli RT. Glycosylphosphatidylinositol-anchored mucin-like glycoproteins isolated from Trypanosoma cruzi trypomastigotes initiate the synthesis of proinflammatory cytokines by macrophages. J Immunol. (1997) 158:5890–901.
90. Camargo MM, Andrade AC, Almeida IC, Travassos LR, Gazzinelli RT. Glycoconjugates isolated from Trypanosoma cruzi but not from Leishmania species membranes trigger nitric oxide synthesis as well as microbicidal activity in IFN-gamma-primed macrophages. J Immunol. (1997) 159:6131–9.
91. Campos MA, Almeida IC, Takeuchi O, Akira S, Valente EP, Procopio DO, et al. Activation of Toll-like receptor-2 by glycosylphosphatidylinositol anchors from a protozoan parasite. J Immunol. (2001) 167:416–23. doi: 10.4049/jimmunol.167.1.416
92. Ropert C, Gazzinelli RT. Signaling of immune system cells by glycosylphosphatidylinositol (GPI) anchor and related structures derived from parasitic protozoa. Curr Opin Microbiol. (2000) 3:395–403. doi: 10.1016/S1369-5274(00)00111-9
93. Ropert C, Ferreira LR, Campos MA, Procopio DO, Travassos LR, Ferguson MA, et al. Macrophage signaling by glycosylphosphatidylinositol-anchored mucin-like glycoproteins derived from Trypanosoma cruzi trypomastigotes. Microbes Infect. (2002) 4:1015–25. doi: 10.1016/S1286-4579(02)01609-X
94. Bafica A, Santiago HC, Goldszmid R, Ropert C, Gazzinelli RT, Sher A. Cutting edge: TLR9 and TLR2 signaling together account for MyD88-dependent control of parasitemia in Trypanosoma cruzi infection. J Immunol. (2006) 177:3515–9. doi: 10.4049/jimmunol.177.6.3515
95. Abel LC, Ferreira LR, Cunha Navarro I, Baron MA, Kalil J, Gazzinelli RT, et al. Induction of IL-12 production in human peripheral monocytes by Trypanosoma cruzi Is mediated by glycosylphosphatidylinositol-anchored mucin-like glycoproteins and potentiated by IFN- gamma and CD40-CD40L interactions. Mediators Inflamm. (2014) 2014:345659. doi: 10.1155/2014/345659
96. de Diego J, Punzon C, Duarte M, Fresno M. Alteration of macrophage function by a Trypanosoma cruzi membrane mucin. J Immunol. (1997) 159:4983–9.
97. Alcaide P, Fresno M. The Trypanosoma cruzi membrane mucin AgC10 inhibits T cell activation and IL-2 transcription through L-selectin. Int Immunol. (2004) 16:1365–75. doi: 10.1093/intimm/dxh138
98. Martinez-Saez N, Peregrina JM, Corzana F. Principles of mucin structure: implications for the rational design of cancer vaccines derived from MUC1-glycopeptides. Chem Soc Rev. (2017) 46:7154–75. doi: 10.1039/C6CS00858E
99. Nunes MP, Fortes B, Silva-Filho JL, Terra-Granado E, Santos L, Conde L, et al. Inhibitory effects of Trypanosoma cruzi sialoglycoproteins on CD4+ T cells are associated with increased susceptibility to infection. PLoS ONE (2013) 8:e77568. doi: 10.1371/journal.pone.0077568
100. Erdmann H, Steeg C, Koch-Nolte F, Fleischer B, Jacobs T. Sialylated ligands on pathogenic Trypanosoma cruzi interact with Siglec-E (sialic acid-binding Ig-like lectin-E). Cell Microbiol. (2009) 11:1600–11. doi: 10.1111/j.1462-5822.2009.01350.x
101. Beverley SM, Owens KL, Showalter M, Griffith CL, Doering TL, Jones VC, et al. Eukaryotic UDP-galactopyranose mutase (GLF gene) in microbial and metazoal pathogens. Eukaryot Cell (2005) 4:1147–54. doi: 10.1128/EC.4.6.1147-1154.2005
102. Tefsen B, Ram AF, van Die I, Routier FH. Galactofuranose in eukaryotes: aspects of biosynthesis and functional impact. Glycobiology (2012) 22:456–69. doi: 10.1093/glycob/cwr144
103. De Arruda MV, Colli W, Zingales B. Terminal beta-D-galactofuranosyl epitopes recognized by antibodies that inhibit Trypanosoma cruzi internalization into mammalian cells. Eur J Biochem. (1989) 182:413–21. doi: 10.1111/j.1432-1033.1989.tb14847.x
104. Boechi L, de Oliveira CA, Da Fonseca I, Kizjakina K, Sobrado P, Tanner JJ, et al. Substrate-dependent dynamics of UDP-galactopyranose mutase: Implications for drug design. Protein Sci. (2013) 22:1490–501. doi: 10.1002/pro.2332
105. El Bkassiny S, N'Go I, Sevrain CM, Tikad A, Vincent SP. Synthesis of a novel UDP-carbasugar as UDP-galactopyranose mutase inhibitor. Org Lett. (2014) 16:2462–5. doi: 10.1021/ol500848q
106. Shi Y, Arda A, Pinto BM. Combined molecular dynamics, STD-NMR, and CORCEMA protocol yields structural model for a UDP-galactopyranose mutase-inhibitor complex. Bioorg Med Chem Lett. (2015) 25:1284–7. doi: 10.1016/j.bmcl.2015.01.044
107. Dykhuizen EC, May JF, Tongpenyai A, Kiessling LL. Inhibitors of UDP-galactopyranose mutase thwart mycobacterial growth. J Am Chem Soc. (2008) 130:6706–7. doi: 10.1021/ja8018687
108. Vasconcelos JR, Boscardin SB, Hiyane MI, Kinoshita SS, Fujimura AE, Rodrigues MM. A DNA-priming protein-boosting regimen significantly improves type 1 immune response but not protective immunity to Trypanosoma cruzi infection in a highly susceptible mouse strain. Immunol Cell Biol. (2003) 81:121–9. doi: 10.1046/j.0818-9641.2002.01136.x
109. Arce-Fonseca M, Ramos-Ligonio A, Lopez-Monteon A, Salgado-Jimenez B, Talamas-Rohana P, Rosales-Encina JL. A DNA vaccine encoding for TcSSP4 induces protection against acute and chronic infection in experimental Chagas disease. Int J Biol Sci. (2011) 7:1230–8. doi: 10.7150/ijbs.7.1230
110. Salgado-Jimenez B, Arce-Fonseca M, Baylon-Pacheco L, Talamas-Rohana P, Rosales-Encina JL. Differential immune response in mice immunized with the A, R or C domain from TcSP protein of Trypanosoma cruzi or with the coding DNAs. Parasite Immunol. (2013) 35:32–41. doi: 10.1111/pim.12017
111. Bontempi IA, Vicco MH, Cabrera G, Villar SR, Gonzalez FB, Roggero EA, et al. Efficacy of a trans-sialidase-ISCOMATRIX subunit vaccine candidate to protect against experimental Chagas disease. Vaccine (2015) 33:1274–83. doi: 10.1016/j.vaccine.2015.01.044
112. Prochetto E, Roldan C, Bontempi IA, Bertona D, Peverengo L, Vicco MH, et al. Trans-sialidase-based vaccine candidate protects against Trypanosoma cruzi infection, not only inducing an effector immune response but also affecting cells with regulatory/suppressor phenotype. Oncotarget (2017) 8:58003–20.doi: 10.18632/oncotarget.18217
113. Bontempi I, Fleitas P, Poato A, Vicco M, Rodeles L, Prochetto E, et al. Trans-sialidase overcomes many antigens to be used as a vaccine candidate against Trypanosoma cruzi. Immunotherapy (2017) 9:555–65. doi: 10.2217/imt-2017-0009
114. Costa F, Pereira-Chioccola VL, Ribeirao M, Schenkman S, Rodrigues MM. Trans-sialidase delivered as a naked DNA vaccine elicits an immunological response similar to a Trypanosoma cruzi infection. Braz J Med Biol Res. (1999) 32:235–9. doi: 10.1590/S0100-879X1999000200013
115. Hoft DF, Eickhoff CS, Giddings OK, Vasconcelos JR, Rodrigues MM. Trans-sialidase recombinant protein mixed with CpG motif-containing oligodeoxynucleotide induces protective mucosal and systemic Trypanosoma cruzi immunity involving CD8+ CTL and B cell-mediated cross-priming. J Immunol. (2007) 179:6889–900. doi: 10.4049/jimmunol.179.10.6889
116. Fontanella GH, De Vusser K, Laroy W, Daurelio L, Nocito AL, Revelli S, et al. Immunization with an engineered mutant trans-sialidase highly protects mice from experimental Trypanosoma cruzi infection: a vaccine candidate. Vaccine (2008) 26:2322–34. doi: 10.1016/j.vaccine.2008.02.060
117. Rodrigues MM, de Alencar BC, Claser C, Tzelepis F, Silveira EL, Haolla FA, et al. Swimming against the current: genetic vaccination against Trypanosoma cruzi infection in mice. Mem Inst Oswaldo Cruz. (2009) 104 (Suppl. 1):281–7. doi: 10.1590/S0074-02762009000900037
118. Arce-Fonseca M, Ballinas-Verdugo MA, Zenteno ER, Suarez-Flores D, Carrillo-Sanchez SC, Alejandre-Aguilar R, et al. Specific humoral and cellular immunity induced by Trypanosoma cruzi DNA immunization in a canine model. Vet Res. (2013) 44:15. doi: 10.1186/1297-9716-44-15
119. Martin DL, Weatherly DB, Laucella SA, Cabinian MA, Crim MT, Sullivan S, et al. CD8+ T-Cell responses to Trypanosoma cruzi are highly focused on strain-variant trans-sialidase epitopes. PLoS Pathog. (2006) 2:e77. doi: 10.1371/journal.ppat.0020077
120. Tzelepis F, de Alencar BC, Penido ML, Claser C, Machado AV, Bruna-Romero O, et al. Infection with Trypanosoma cruzi restricts the repertoire of parasite-specific CD8+ T cells leading to immunodominance. J Immunol. (2008) 180:1737–48. doi: 10.4049/jimmunol.180.3.1737
121. Rosenberg CS, Martin DL, Tarleton RL. CD8+ T cells specific for immunodominant trans-sialidase epitopes contribute to control of Trypanosoma cruzi infection but are not required for resistance. J Immunol. (2010) 185:560–8. doi: 10.4049/jimmunol.1000432
122. Dominguez MR, Silveira EL, de Vasconcelos JR, de Alencar BC, Machado AV, Bruna-Romero O, et al. Subdominant/cryptic CD8 T cell epitopes contribute to resistance against experimental infection with a human protozoan parasite. PLoS ONE (2011) 6:e22011. doi: 10.1371/journal.pone.0022011
123. Pereira IR, Vilar-Pereira G, Marques V, da Silva AA, Caetano B, Moreira OC, et al. A human type 5 adenovirus-based Trypanosoma cruzi therapeutic vaccine re-programs immune response and reverses chronic cardiomyopathy. PLoS Pathog. (2015) 11:e1004594. doi: 10.1371/journal.ppat.1004594
124. Cano ME, Agusti R, Cagnoni AJ, Tesoriero MF, Kovensky J, Uhrig ML, et al. Synthesis of divalent ligands of beta-thio- and beta-N-galactopyranosides and related lactosides and their evaluation as substrates and inhibitors of Trypanosoma cruzi trans-sialidase. Beilstein J Org Chem. (2014) 10:3073–86. doi: 10.3762/bjoc.10.324
125. Lara-Ramirez EE, Lopez-Cedillo JC, Nogueda-Torres B, Kashif M, Garcia-Perez C, Bocanegra-Garcia V, et al. An in vitro and in vivo evaluation of new potential trans-sialidase inhibitors of Trypanosoma cruzi predicted by a computational drug repositioning method. Eur J Med Chem. (2017) 132:249–61. doi: 10.1016/j.ejmech.2017.03.063
126. Harrison JA, Kartha KP, Turnbull WB, Scheuerl SL, Naismith JH, Schenkman S, et al. Hydrolase and sialyltransferase activities of Trypanosoma cruzi trans-sialidase towards NeuAc-alpha-2,3-gal-Gal-beta-O-PNP. Bioorg Med Chem Lett. (2001) 11:141–4. doi: 10.1016/S0960-894X(00)00611-9
127. Tiralongo E, Schrader S, Lange H, Lemke H, Tiralongo J, Schauer R. Two trans-sialidase forms with different sialic acid transfer and sialidase activities from Trypanosoma congolense. J Biol Chem. (2003) 278:23301–10. doi: 10.1074/jbc.M212909200
128. Paris G, Ratier L, Amaya MF, Nguyen T, Alzari PM, Frasch AC. A sialidase mutant displaying trans-sialidase activity. J Mol Biol. (2005) 345:923–34. doi: 10.1016/j.jmb.2004.09.031
129. Neres J, Bonnet P, Edwards PN, Kotian PL, Buschiazzo A, Alzari PM, et al. Benzoic acid and pyridine derivatives as inhibitors of Trypanosoma cruzi trans-sialidase. Bioorg Med Chem. (2007) 15:2106–19. doi: 10.1016/j.bmc.2006.12.024
130. Carvalho I, Andrade P, Campo VL, Guedes PM, Sesti-Costa R, Silva JS, et al. 'Click chemistry' synthesis of a library of 1,2,3-triazole-substituted galactose derivatives and their evaluation against Trypanosoma cruzi and its cell surface trans-sialidase. Bioorg Med Chem. (2010) 18:2412–27. doi: 10.1016/j.bmc.2010.02.053
131. Carvalho ST, Sola-Penna M, Oliveira IA, Pita S, Goncalves AS, Neves BC, et al. A new class of mechanism-based inhibitors for Trypanosoma cruzi trans-sialidase and their influence on parasite virulence. Glycobiology (2010) 20:1034–45. doi: 10.1093/glycob/cwq065
Keywords: Trypanosoma cruzi, trans-sialidase, mucin-like molecule, sialic acid, glycan-binding protein, infectious disease, T-cell response
Citation: Fonseca LM, da Costa KM, Chaves VS, Freire-de-Lima CG, Morrot A, Mendonça-Previato L, Previato JO and Freire-de-Lima L (2019) Theft and Reception of Host Cell's Sialic Acid: Dynamics of Trypanosoma Cruzi Trans-sialidases and Mucin-Like Molecules on Chagas' Disease Immunomodulation. Front. Immunol. 10:164. doi: 10.3389/fimmu.2019.00164
Received: 02 November 2018; Accepted: 18 January 2019;
Published: 06 February 2019.
Edited by:
Monica M. Burdick, Ohio University, United StatesReviewed by:
Carlos A. Buscaglia, Consejo Nacional de Investigaciones Científicas y Técnicas (CONICET), ArgentinaEden Ramalho Ferreira, Federal University of São Paulo, Brazil
Igor C. Almeida, The University of Texas at El Paso, United States
Copyright © 2019 Fonseca, da Costa, Chaves, Freire-de-Lima, Morrot, Mendonça-Previato, Previato and Freire-de-Lima. This is an open-access article distributed under the terms of the Creative Commons Attribution License (CC BY). The use, distribution or reproduction in other forums is permitted, provided the original author(s) and the copyright owner(s) are credited and that the original publication in this journal is cited, in accordance with accepted academic practice. No use, distribution or reproduction is permitted which does not comply with these terms.
*Correspondence: Leonardo Marques da Fonseca, bGZvbnNlY2FAYmlvZi51ZnJqLmJy
Lucia Mendonça-Previato, bHVjaWFtcEBiaW9mLnVmcmouYnI=