- National Institute for Health Research Birmingham Liver Biomedical Research Unit and Centre for Liver and Gastrointestinal Research, Institute of Immunology and Immunotherapy, University of Birmingham, Birmingham, United Kingdom
Scavenger receptors are a highly diverse superfamily of proteins which are grouped by their inherent ability to bind and internalize a wide array of structurally diverse ligands which can be either endogenous or exogenous in nature. Consequently, scavenger receptors are known to play important roles in host homeostasis, with common endogenous ligands including apoptotic cells, and modified low density lipoproteins (LDLs); additionally, scavenger receptors are key regulators of inflammatory diseases, such as atherosclerosis. Also, as a consequence of their affinity for a wide range of microbial products, their role in innate immunity is also being increasingly studied. However, in this review, a secondary function of a number of endothelial-expressed scavenger receptors is discussed. There is increasing evidence that some endothelial-expressed scavenger receptors are able to directly bind leukocyte-expressed ligands and subsequently act as adhesion molecules in the trafficking of leukocytes in lymphatic and vascular tissues. Here, we cover the current literature on this alternative role for endothelial-expressed scavenger receptors and also speculate on their therapeutic potential.
Introduction
The first scavenger receptor was described in the late 1970s by Brown and Goldstein and was defined by its ability to bind and subsequently internalize low density lipoproteins (LDLs) (1, 2). However, the term “scavenger receptor” was not coined until a couple of years later in the early 1980s by Fogelman et al. who were studying the functionality of Brown and Goldstein's LDL receptor in monocytes and macrophages (3). Scavenger receptors are now a large superfamily of proteins which are highly diverse in structure and are sub-divided into a number of classes (class A-J), with each class sharing structural features; however, there is little or no sequence homology between the classes and the superfamily grouping is purely a consequence of shared functional properties (4). Functionally, scavenger receptors have an important role in both homeostatic and disease states, as they detect and remove, or scavenge, unsolicited self-antigens, which predominantly manifest as damage-associated molecular patterns (DAMPs), such as phosphatidylserine on apoptotic cells (5–7) and products of oxidative stress (e.g., oxidized (ox)LDLs) (8, 9), from general circulation. The removal of apoptotic host cells by scavenger receptors is particularly pertinent in the context of autoimmune diseases, such as systemic lupus erythematosus (SLE), which has been shown to spontaneously develop in some lines of scavenger receptor-deficient mice (7, 10), thus highlighting their role in homeostasis. Also, other clinical manifestations, for example severe renal glomerular fibrosis and premature mortality, have been shown to spontaneously develop in some multiple scavenger receptor-deficient mice as a result of impaired clearance of harmful factors, such as growth differentiation factor (GDF)-15, from the systemic blood supply (11). These severe phenotypes are somewhat surprising given that several scavenger receptors are able to bind a number of common ligands; therefore, one would assume there would be a certain amount of redundancy in their function and, in the absence of one scavenger receptor, the others would be up-regulated in a compensatory manner to maintain homeostasis. Nevertheless, this is clearly not the case for several members of the scavenger superfamily.
In a number of murine models of inflammatory diseases, the lack of certain scavenger receptors has been shown to be highly detrimental, thus implicating these receptors in the limitation of disease pathology. For example, in a murine model of Alzheimer's disease, reduction or deletion of scavenger receptor class B type I (SR-BI) resulted in increased severity of disease due to impaired clearance of amyloid-β by infiltrating macrophages (12). More recently, we have shown that a lack of the class H scavenger receptor, stabilin-1, in murine models of liver injury promotes fibrogenesis, due to impaired clearance of malondialdehyde (MDA) modified oxLDLs (MDA-LDLs) (13). Conversely, some scavenger receptors have been shown to actively contribute to disease pathology, with several implicated in the establishment, and progression of atherosclerosis due their role in the uptake and storage of LDLs in macrophages (14–17). Furthermore, scavenger receptors also play an important role in the host innate immune system (18–21), as the majority of scavenger receptors are differentially expressed in a number of professional innate immune cells, such as monocytes, macrophages and dendritic cells (22, 23), and are able to recognize a huge array of microbial antigens (24, 25). However, the paradigm is now being established that scavenger receptors require the presence of other pattern recognition receptors (PRRs), such as Toll-like receptors (TLRs), in order to elicit an immunological response (26–30).
In addition to their intrinsic scavenging capacity, a number of endothelial-expressed scavenger receptors also exhibit a secondary function in host immunity as they are able to directly interact with leukocytes and mediate their passage across a range of endothelia. This secondary function has led to the study of some scavenger receptors in lymphocyte migration in lymph nodes and in the extravasation of leukocytes during inflammation. In this review, we initially discuss the processes of leukocyte trafficking, subsequently explore the current knowledge of scavenger receptor involvement in these processes and speculate on future research and potential for this relatively understudied function of scavenger receptors.
Lymphocyte Trafficking in Lymph Nodes
The antigen-driven adaptive immune system requires regulated trafficking of T cells in order to orchestrate lymphocyte development, immune surveillance, rapid immunological responses, and memory (31). Consequently, lymphocytes are continually recirculating between the vascular and lymphatic systems and organ tissues. T cells which have not previously encountered antigens, termed naïve T cells, are programmed to undergo migratory cycles into and out of secondary lymphoid organs (SLOs), such as peripheral lymph nodes, tonsils, and Peyers patches, in search of cognate antigens (31). T cells enter lymph nodes (LNs) through afferent lymphatic vessels or high endothelial venules (HEVs) (32) and subsequently interact with antigen presenting cells, primarily dendritic cells (DCs), which present antigens encountered in inflamed tissues on their surface via major histocompatibility complex (MHC) proteins (33). Once T cells encounter cognate MHC/antigen, in concert with the relevant co-stimulatory or co-inhibitory molecules, they become activated, and undergo differentiation into antigen-specific effector or memory cells (33). The trafficking of T cells to and from lymph nodes is known to involve intimate interactions with lymphatic endothelial cells (LECs); however, the endothelial-expressed molecules involved in these processes are not well characterized (31). Nevertheless, the involvement of scavenger receptors has been suggested and is discussed throughout this review.
The Leukocyte Adhesion Cascade
During injury or infection, leukocytes in the blood are required to migrate from general circulation, across the vascular endothelium, and into the inflamed tissue, with the primary aim of eliminating the inflammatory trigger and/or contributing to tissue repair (34). In general, the migration of leukocytes from the blood into inflamed tissues occurs in post-capillary venules, with the exception of the liver, spleen and lungs (34). Leukocyte migration is achieved via a multi-step process known as the leukocyte adhesion cascade (35) (Figure 1), in which the leukocytes initially tether and roll on the luminal surface of the blood vessel and undergo arrest, followed by firm adhesion and, finally, migrate through the endothelial barrier into the tissue (36). This sequence of events is mediated by a large number of chemoattractant cytokines (chemokines) (37) and adhesion molecules (Figure 1) which determine the subset of leukocyte to be recruited to the site of inflammation and subsequently regulate their numbers (34). Additionally, crossing the vascular wall is not only a highly selective and regulatory step in leukocyte migration, but also acts to prime the tissue-infiltrating leukocytes (38) in order to deliver an efficient and effective immunological response.
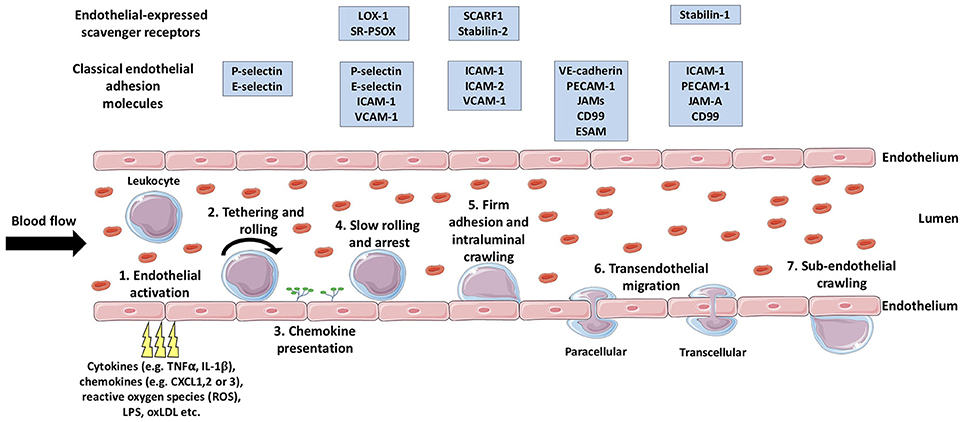
Figure 1. The multistep leukocyte adhesion cascade. Leukocytes are recruited from the bloodstream to inflamed tissues via sequential multi-step process known as the leukocyte adhesion cascade. Firstly, endothelial activation is triggered by a range of endogenous or exogenous stimuli from the inflamed tissue (1), which triggers the selectin-dependent tethering and rolling of leukocytes along the luminal surface of the vessel (2). Subsequently, chemokines are presented on the luminal surface of the endothelium (3) which activate leukocyte-expressed integrins allowing stronger bond formation with their endothelial-expressed ligands. The formation of these stronger leukocyte-endothelium bonds results in leukocyte arrest (4), following which, intraluminal crawling occurs (5). Next, the leukocyte will undergo transendothelial migration (6) either via the paracellular or the transcellular pathway. Once the leukocyte has crossed the endothelial layer, it may undertake in sub-endothelial crawling (7), prior to entering the target tissue proper. TNFα, tumor necrosis factor-α; IL-1β, interleukin-1β; LPS, lipopolysaccharide; oxLDL, oxidized low density lipoprotein; LOX-1, Lectin-like oxidized low-density lipoprotein receptor-1; SR-PSOX, scavenger receptor that binds phosphatidylserine and oxidized lipids; ICAM-1, intercellular adhesion molecule-1; VCAM-1, vascular cell adhesion molecule-1 ; SCARF1, scavenger receptor class F; member 1; VE-cadherin, vascular endothelial cadherin; PECAM-1, platelet endothelial cell adhesion molecule-1; JAMs, junctional adhesion molecules; ESAM, endothelial cell-specific adhesion molecule. (Stock images provided by Servier medical for use under the Creative Commons Attribution 3.0 Unported License).
Endothelial Activation, Initial Capture, and Rolling
Endothelial activation is the initial step which results in the expression of adhesion molecules and chemokines on the luminal membrane of endothelial cells involved in the initial capture of leukocytes from shear flow. Endothelial activation can be triggered by a wide range of stimuli and is classified as “type I” or “type II,” depending on the mediating signal molecule. Type I activation of endothelial cells is a protein-synthesis-independent process and is predominantly mediated via ligands of heterotrimeric G-protein-coupled receptors (GPCRs), such as histamine and thrombin (39). Type I activation results in the trafficking of pre-formed P-selectin to the cell membrane within minutes, thus allowing the rapid recruitment of neutrophils to vascular endothelia (40–43). Type I activation is a highly transient event and, in order to limit the extent of neutrophil extravasation, the GPCRs involved are presumed to undergo desensitization (44, 45) to their stimuli after 10–20 min to prevent further endothelial stimulation (39). Type II activation of endothelial cells is a much slower process known to be triggered by a much wider range of stimuli, including inflammatory cytokines [e.g., tumor necrosis factor (TNF)α, interferon (IFN)γ, and interleukin (IL)-1β (46)], microbial antigens [e.g., lipopolysaccharide (LPS) (47, 48)], and oxLDLs (49, 50). Type II activation results in morphological changes, via the reorganization of actin filaments (51) and de novo expression of leukocyte adhesion molecules, such as E-selectin, intracellular adhesion molecule (ICAM)-1 and vascular cell-adhesion molecule (VCAM)-1 (52–55), and chemokines (56, 57) on the luminal surface of the endothelial cells. Unlike, type I activation which is stringently regulated via receptor desensitization, type II activation is much more long-lived and can chronically persist until the inflammatory stimulus is removed and the regulatory anti-inflammatory feedback mechanisms are able to effectively counteract the proinflammatory exacerbation, commonly via regulation of the nuclear factor (NF)-κB pathway (58, 59).
Following endothelial activation, the initial capture of leukocytes from shear flow is mediated by selectins, a family of three Type I transmembrane Ca2+-dependent lectins which bind to glycoprotein ligands (60). The selectins are named according to the cell type in which they were originally described in (platelet (P)-selectin, leukocyte (L)-selectin and endothelial (E)-selectin) and consist of an extracellular N-terminal C-type lectin domain, an epidermal growth factor (EGF)-like domain, a series of short consensus repeats (SCRs), a transmembrane domain and a short C-terminal intracellular domain (61). As mentioned above, stores of pre-formed P-selectin are held within human endothelial cells (62) and are rapidly trafficked to the surface in the event of type I activation, but P-selectin is also differentially regulated in a range of chronic inflammatory diseases (63–66) and plays a major role in leukocyte recruitment during prolonged type II activation (67–70). L-selectin is expressed in the majority of circulating leukocytes and is one of the first leukocyte-expressed cell adhesion molecules to interact with the endothelial layer in the initial “tethering” event (71), whereas E-selectin is constitutively expressed in bone marrow endothelial cells (72), but is inducible in other endothelia (54). E-selectin is predominantly involved in the rolling and slow rolling steps of the adhesion cascade (73, 74). Rolling is the transient and reversible selectin-ligand interaction which involves the “catch-bond” phenomenon, where bonds are strengthened with increasing shear stress (75). Also, the rolling motion of leukocytes is able to generate new selectin-ligand bonds before old ones are broken via the “tether and sling” phenomenon utilized by neutrophils (76, 77) and differentiated T cell subsets (78). The rolling and slow rolling steps aim to initiate leukocyte-endothelial contact and, consequently, further activate the leukocyte, thus promoting the successive steps in the adhesion cascade.
Leukocyte Arrest and Crawling
The arrest of leukocytes rolling along the surface of the endothelium is triggered by chemokines which are expressed upon endothelial activation and are immobilized on the luminal surface via highly negatively-charged polysaccharides, such as glycosaminoglycans (GAGs) (79, 80). As a consequence of chemokine-induced “inside-out” signaling, heterodimeric adhesion receptors expressed on the surface of leukocytes, known as integrins, undergo conformational changes and become “activated” (81, 82). Once activated, integrins are able to form high affinity bonds with their endothelial-expressed ligands and their clustering in focal adhesion contacts allows for stronger leukocyte-endothelial bonds (83), thus resulting in leukocyte arrest [reviewed in detail by Ley et al. (35)].
Once firmly adhered to the endothelial layer, innate immune cells, such as monocytes, have been shown to patrol the vessel wall surface (84), scavenging microparticles, and supporting the recruitment of other cells, such as neutrophils (85). This intraluminal “crawling” behavior has also been observing in neutrophils and is thought to mediate their transmigration across the endothelial layer, as they search for sites of exit from the blood vessel (86–88). Additionally, a novel phenomenon in hepatic sinusoidal endothelial cells (HSEC) was recently described in which peripheral blood lymphocytes were shown to migrate horizontally from one endothelial cell to another (89). This intracellular crawling appeared to be HSEC-specific as it did not occur in more conventional vascular endothelial cells (HUVEC; human umbilical vein endothelial cells). It was subsequently speculated that this process could represent a liver-specific method of immune surveillance (89); however, studies of this phenomenon were all undertaken in vitro and it is yet to be confirmed in vivo. Interestingly, once leukocytes have traversed the endothelial barrier, they have also been shown to undergo sub-endothelial crawling (90–92) prior to their migration into the tissue proper.
Transendothelial Migration
The final step in the leukocyte adhesion cascade is the crossing of the endothelial barrier, which is known as transendothelial migration (93). Transendothelial migration of leukocytes is a highly regulated process as maintenance of barrier integrity is paramount and endothelial cells undergo significant cytoskeletal remodeling to facilitate the passage of leukocytes, whilst also preventing vascular leakage (94). There are two possible routes for leukocytes to transmigrate the endothelial barrier, the paracellular pathway, or the transcellular pathway [reviewed extensively by Ley et al. (35) and more recently by Vestweber (36)]. The paracellular route describes the passage of leukocytes between the cell-cell junctions of the endothelial layer and has inevitably been shown to be mediated via a number of key junctional proteins, such as platelet endothelial cell adhesion molecule (PECAM)-1 (also known as CD31) (95), CD99 (95, 96), and junctional adhesion molecules (JAMs) (97, 98). Also, vascular endothelial (VE)-cadherin has been shown to play an instrumental role in the inhibition of leukocyte extravasation and must be actively moved away from the site of leukocyte transmigration to allow the process to occur (99, 100). The vast majority (~80–95 %) of cells undergo transendothelial migration via the paracellular route; however, the remainder transmigrate through the transcellular pathway which involves leukocytes passing directly through the cell body of endothelial cells This process is highly coordinated and requires extensive remodeling of the endothelial cell's actin cytoskeleton to form an appropriately sized pore to accommodate the passage of the leukocyte, and in particular its nucleus (101). Unsurprisingly, the transcellular migration of leukocytes is stringently regulated by the endothelial cell to minimize vascular leakage (101). The molecules involved in transcellular are less well-studied than those for paracellular migration; nevertheless, to date, ICAM-1 (53, 94, 102, 103) has been identified as the major contributor, but other molecules, such as PECAM-1, JAM-A, and CD99 (104, 105) have also been shown to play a role in this process.
With the technological advancements in microscopy, our knowledge of the processes involved in leukocyte transmigration are ever-increasing (94, 101, 106, 107); nevertheless the molecular mechanisms which determine whether leukocytes transmigrate through the paracellular or transcellular pathways still remain a mystery. The possibility of scavenger receptors playing a role in these processes is a tangible prospect and should be investigated in future studies.
Scavenger Receptors in Leukocyte Trafficking
Given that a number contain similar structural domains to those found in the selectin family [e.g., C-type lectin domains or epidermal growth factor (EGF)-like domains], it is perhaps unsurprising that several endothelial-expressed scavenger receptors are also able to directly bind to leukocytes. Consequently, several scavenger receptors have been shown to play a role in leukocyte trafficking through lymph nodes and/or in their extravasation through a range of endothelia. Discussed below are the scavenger receptors identified to date which play a role in these processes.
SR-AI
Scavenger receptor (SR)-AI, also known as macrophage scavenger receptor (MSR)-1 or CD204, was the first scavenger receptor to be cloned (108), and hence is the first member of the Class A family and arguably the most studied scavenger receptor (109). SR-AI is a Type II membrane protein, with its structure consisting of a short N-terminal cytoplasmic tail, a transmembrane domain, a spacer region, an α-helical coiled-coil domain, a collagen-like domain, and a C-terminal scavenger receptor cysteine-rich (SRCR) domain (110). As is characteristic of most scavenger receptors, SR-AI has been shown to bind a highly diverse range of endogenous products including: an array of modified LDLs (111, 112); apoptotic cells (113); heat shock proteins (Hsp) (114); collagen (115); β-amyloid (116); apolipoproteins (117), and advanced glycation end products (AGE) (118). Additionally, SR-AI can also bind a range of exogenous ligands, such as bacterial lipopolysaccharide (LPS) (119), and lipoteichoic acid (LTA) (120), fungal β-glucan (121), and viral double stranded (ds)RNA (122–124). SR-AI is predominantly expressed in myeloid cells, such as monocytes and tissue-resident macrophages, but was also shown to be expressed in high endothelial cells of postcapillary venules (HEV) in peripheral lymph nodes a number of years ago (125). The adhesive ability of SR-AI has only recently been considered; however, this recent study has focused on lymphocyte binding to lymphatic endothelial cells (LEC) (126). In their investigation of SR-AI in LEC, Iftakhar-E-Khuda et al. utilized binding assays to primary murine lymphatic endothelial cells in vitro and antibody blockade on human and murine lymphatic tissue sections ex vivo to demonstrate its lymphocyte binding capacity in afferent lymphatics (126). However, they did not observe any differences in lymphocyte populations in the lymph nodes of wild type (WT) and SR-AI−/− mice, possibly suggesting a possible redundancy in SR-AI's lymphocyte binding activity in vivo, under homeostatic conditions. This discrepancy between the in vitro and in vivo data suggests that further investigation of SR-AI's adhesive properties is warranted and future studies could possibly explore lymph node trafficking of leukocytes in mice subjected to injury, such as LPS-induced toxemia. Additionally, given the SR-AI expression in HEVs and that inducible expression of SR-AI has been found in human arterial endothelial cells (127), it is not unreasonable for future investigations to explore SR-AI expression in a range of vascular endothelia from different tissues. If found in these vascular endothelial cells, basic static and flow-based adhesion assays, such as those utilized previously in our lab (128), could be employed to determine which step in the leukocyte adhesion cascade SR-AI potentially acts. Furthermore, a leukocyte-expressed ligand has yet to be explored and so future studies should also aim to identify the molecule(s) involved in SR-AI-mediated leukocyte binding to these endothelia.
LOX-1
Lectin-like oxidized low-density lipoprotein receptor-1 (LOX-1) is another Type II membrane protein which comprises of a short N-terminal cytoplasmic domain, a single transmembrane region and an extracellular domain containing a coiled-coil “neck” region and a C-type lectin-like domain (129) and was the first member of the Class E family to be described. As its name suggests, LOX-1 was initially identified as a receptor for oxLDLs in endothelial cells (129), but has since been shown to bind a number of other modified LDLs, such as carbamylated LDLs (130) and glycoxidised LDLs (131). Subsequently, LOX-1 has also been found to bind a more diverse range of ligands, including phosphotidylserine on apoptotic cells (132, 133), Gram positive and Gram negative bacteria (134), and C-reactive protein (CRP) (135). Nevertheless, LOX-1 is a “non-essential” protein, as LOX-1−/− mice do not exhibit any phenotypic traits. Also, under physiological conditions, LOX-1 is expressed in relatively low levels in vascular endothelial cells, but is inducible upon endothelial activation by ligand binding (136, 137), inflammatory cytokines (138, 139) or shear stress (140). The leukocyte adhesive ability of LOX-1 was first described in 2002, when Hayashida et al. demonstrated that transfected Chinese hamster ovarian (CHO) cells over-expressing LOX-1 augmented the adhesion of primary peripheral blood mononuclear cells (PBMCs), and monocytic cell line, THP-1, when compared to control transfected cells (141). Interestingly, this effect appeared to be monocytic cell-specific, as they did not observe any effects on the Jurkat leukaemic T cell line (141). Additionally, they demonstrated that the enhanced adhesion of THP-1 cells to the LOX-1-CHO could be reversed by antibody or oxLDL blockade and recapitulated this blockade on bovine aortic endothelial cells (BAEC) in vitro (141). Finally, they demonstrated that THP-1 cells flowed over LOX-1-CHO cells at increasing shear stress exhibited increased numbers of cells rolling and at lower rolling velocities than those flowed over WT-CHO cells (141), thus suggesting that LOX-1 acts as an adhesion molecule in the early stages of the leukocyte adhesion cascade.
Following this initial study, Li et al. then demonstrated that antibody blockade of LOX-1 in vivo, in a rat myocardial ischaemia-reperfusion model, was able to significantly reduce the number of infiltrating leukocytes to the myocardial tissues, which also resulted in a significant decrease in the myocardial infarct (142). However, their data suggested that the diminished leukocyte infiltration was due to an indirect effect of LOX-1 blockade, as they showed a reduction in the expression of adhesion molecules, such as ICAM-1, VCAM-1, and P-selectin (142). Nevertheless, in a seminal study, Honjo et al. demonstrated in a rat model of endotoxemia and endotoxin-induced uveitis, that antibody blockade of LOX-1 expression induced in retinal endothelial cells significantly reduced the number of rolling infiltrating leukocytes, which predominantly consisted of neutrophils, and also increased the velocity of rolling (143). This data is suggestive of a direct interaction with leukocytes in vivo and adds to in vitro studies which show that LOX-1 functions as adhesion molecule in the early stages of the leukocyte adhesion cascade. Also, more recently, Ding et al. demonstrated that LOX-1−/− mice fed a high cholesterol diet exhibit a lower level of macrophage accumulation in their aortas compared to WT mice (144); nevertheless, it is unclear whether this was due a lack of LOX-1-mediated recruitment by the aortic endothelial cells or a migratory defect in the LOX-1-deficient macrophages.
From the current data implicating it in the leukocyte adhesion cascade, it is clear that LOX-1 contributes to the rolling stage of the adhesion cascade in the recruitment of myeloid cells to a range of vascular endothelia. Nevertheless, despite a number of studies now demonstrating this both in vitro and in vivo, the leukocyte-expressed ligand(s) responsible for LOX-1 binding have not yet been identified. Additionally, initial studies have suggested that the adhesive properties of endothelial-expressed LOX-1 do not extend to cells of lymphoid lineage, this has only been tested utilizing a leukaemic T cell line and so further investigation with primary lymphocytes could in fact be warranted.
Mannose Receptor
The third member of the Class E scavenger receptor family to be described, the mannose receptor (MR) or CD206, is a Type I membrane glycoprotein which consists of a short intracellular domain, a transmembrane domain, and an extracellular region comprising of a eight C-type lectin-like domains, a fibronectin type II domain and an N-terminal cysteine-rich domain (145). As its name suggests, MR was originally discovered to bind mannose and other carbohydrate groups in a range of glycoproteins (146); nevertheless, given that its extracellular region comprises of several functionally distinct domains, MR has since been shown to bind a wide range of other endogenous ligands, including collagen (147, 148), CD45 (149), tumoural mucins (150), and neutrophil-derived myeloperoxidases (151). Additionally, MR can bind a range of bacterial- (152, 153), viral- (154–157), fungal- (158–161), and parasite-derived (21) antigens. The mannose receptor is predominantly expressed by macrophages (162, 163), but has also been described in a range of endothelial cells, such as hepatic sinusoidal endothelial cells (HSEC) (89, 164), dermal endothelial cells (165) and lymphatic endothelial cells (LEC) (166–168). The leukocyte adhesive properties of MR were first described by the Jalkanen group based at University of Turku, Finland in 2001, when they suggested that MR plays a role in lymphocyte exiting from lymph nodes as their data confirmed the MR-mediated adhesion of lymphocytes to LECs (167). These studies also demonstrated that L-selectin, was the lymphocyte-expressed ligand required for MR-mediated static adhesion of lymphocytes to LECs in vitro, which the authors believed to most accurately mimic physiological conditions within lymph nodes in vivo (167). Further studies by the same group demonstrated the binding of B lymphoblastoid cell lines to LEC and high endothelial venules (HEVs) both on tissues sections ex vivo and on isolated cells in vitro (166), further strengthening the evidence for the adhesive functionality of MR. Subsequently, these in vitro findings were corroborated with in vivo experiments by Marttila-Ichihara et al. who demonstrated that the adhesion of both normal lymphocytes and tumor cells to afferent lymphatic vessels was significantly reduced in MR-deficient mice, compared to WT (168). More recently, the Jalkanen group also showed that L-selectin-negative leukocytes trafficking to the lymph nodes utilize CD44 to bind to MR expressed on LECs and subsequently migrate to draining lymph nodes (169). The authors also suggest that therapeutic targeting of MR on LEC could selectively reduce leukocyte migration from the periphery into the draining lymph nodes thus potentially acting to dampen inappropriate inflammatory reactions (169). Expression in vascular endothelial cells, such as HSEC, suggests that MR could also potentially facilitate leukocyte binding in the adhesion cascade and future studies could investigate this.
SCARF1
Scavenger receptor class F, member 1 (SCARF1 or SR-F1), also known as scavenger receptor expressed by endothelial cells (SREC)-I, was first identified in cDNA libraries from human umbilical vein endothelial cells (HUVEC) (170). SCARF1 is a type I membrane protein which comprises of several extracellular EGF-like domains, a transcellular domain and, unusually for a scavenger receptor, a long serine- and proline-rich cytoplasmic tail (171). SCARF1 has been shown to bind modified low density lipoproteins (LDLs), specifically acLDLs (172), and acts as an endocytic receptor for a wide range of damage-associated products (173), including heat-shock proteins (Hsps) (174–176) and apoptotic host cells via phosphotidylserine-bound C1q protein (7). SCARF1 has been shown to play a key role in the prevention of autoimmunity, as SCARF1-deficient mice spontaneously develop systemic lupus erythematosus (SLE) due to the severely impaired clearance of apoptotic cells in the spleen (7). In addition to binding and internalizing a diverse range of endogenous proteins, SCARF1 also binds a wide array of viral (29, 177, 178), fungal (179), and bacterial (28, 30, 180, 181) antigens and SCARF1 expression in alveolar macrophages has been shown to play an important role in immunological responses to fungal lung infection (179). SCARF1 is also expressed in murine splenic endothelial cells (179) and liver sinusoidal endothelial cells (178) and our lab has corroborated this and recently described for the first time the expression on SCARF1 in primary human hepatic sinusoidal endothelial cells (HSEC) (182). Subsequently, utilizing a combination of flow-based adhesion assays with immobilized recombinant proteins, HSEC, and siRNA silencing in HSEC, we were able to robustly demonstrate that SCARF1 plays a role in the selective recruitment of CD4+ T cells to the sinusoidal endothelium under physiological shear stress (182). Additionally, we showed that SCARF1 facilitates this process via the formation of adhesive cups which were also rich in ICAM-1 and F-actin and proposed that SCARF1 acts in the firm adhesion step of the leukocyte adhesion cascade (182). However, we did not explore the possibility SCARF1's involvement in the transendothelial migration step and future investigations from our lab will explore this. SCARF1 is known to form moderate homophilic interactions (183); however, we ruled out the possibility of these interactions in this context, as CD4+ T cells do not express SCARF1 (182). Therefore, the lymphocyte-expressed ligand of SCARF1 is yet to be identified and screening experiments could be employed to determine this in future investigations.
SR-PSOX
Scavenger receptor that binds phosphatidylserine and oxidized lipids (SR-PSOX) is the only member belonging to the class G family of scavenger receptors to date (184) and is structurally unique within the scavenger receptor superfamily. SR-PSOX is a type I transmembrane glycoprotein with its N-terminal extracellular domain, consisting of a CXC chemokine motif and a mucin-like stalk, linked to a transmembrane domain and a short C-terminal intracellular domain (185). SR-PSOX also exists in a soluble form which is shed or enzymatically cleaved from the cell surface via a disintegrin and metalloproteinase (ADAM)-10 and ADAM-17 (186–189). SR-PSOX was first identified in the human monocytic cell line THP-1 and was shown to bind and internalize oxLDL and phosphatidylserine (190). Subsequently, SR-PSOX has also been shown to bind eryptotic erythrocytes (191, 192) and bacterial antigens (193, 194) and has been found to be expressed in a wide range of cell types, including macrophages (195), DCs (196), smooth muscle cells (197), and endothelial cells (189, 198, 199). Early cloning studies on a chemokine known as CXCL16 (200, 201) found it to be structurally identical to SR-PSOX and, as CXCL16 is a highly specific ligand for the chemokine receptor CXCR6, it was soon discovered that SR-PSOX was able to support the adhesion of a range of CXCR6+ leukocytes (202–205). Subsequent to these findings, it was suggested that SR-PSOX acts in the “arrest” stage of the adhesion cascade by triggering the conformational activation of β1 integrins on leukocytes (206).
Possibly the best studied role for SR-PSOX in the recruitment of leukocytes is in the context of hepatic inflammation (207), with its endothelial-expressed form known to interact with intrahepatic CXCR6+ immune cells, such as effector T cells (206, 208), natural killer (NK) cells (209, 210) and NKT cells (199). It has recently been shown that genetic deficiency of SR-PSOX in mice inhibits the extent of inflammation in a model of acetaminophen (APAP)-induced acute liver injury (211). In addition, pharmacological intervention with neutralizing antibodies raised against SR-PSOX has shown inflammation-reducing efficacy in preclinical murine models of sepsis-mediated (212, 213) and carbon tetrachloride (CCl4)-mediated (207) acute liver injury. Conversely, an elegant study by Ma et al. has recently shown that HSEC-expressed SR-PSOX plays a key role in the recruitment of anti-tumourigenic NKT cells to the liver in a number of murine models of primary and metastatic hepatic cancers (214). Thus, the therapeutic targeting of SR-PSOX to inhibit hepatic inflammation must be carefully considered with regards to context of the inflammatory injury being treated.
Stabilin-1
Stabilin-1 is a highly conserved type I transmembrane protein and was the first member of the Class H family of scavenger receptor to be described. It was originally described in 1991 as MS-1 antigen, when it was used as a histological marker for non-continuous splenic sinusoidal endothelial cells (215). Subsequently, three labs independently described the same molecule as FEEL-1, due to its structure containing fasciclin (FAS), epidermal growth factor (EGF)-like, laminin-type EGF-like, and link domains (171), stabilin-1 (216) and common lymphatic endothelial and vascular endothelial receptor (CLEVER)-1 (217); however, due to its official gene nomenclature, STAB1, stabilin-1 is increasingly utilized in the literature. An early indicator of stabilin-1's capacity as a scavenger receptor was its constitutive expression in the professional scavenging cells of the non-continuous sinusoidal endothelia in the spleen (215), lymph nodes (218, 219), and liver (220). Interestingly, stabilin-1 expression is also inducible in continuous endothelia, in response to angiogenic and proinflammatory stimuli (221). This inducible expression is thought to originate from the transient non-continuous state that vascular endothelial cells transition through during the rapid growth of blood vessels throughout the wound healing process, tumor vascularization, and chronic inflammatory skin conditions, such as psoriasis. As is a prerequisite of being classified as a “scavenger receptor,” stabilin-1 has been shown to bind a wide variety of ligands, such as: modified LDLs (13, 222); phosphotidylserine expressed on apoptotic cells (223–225); secreted protein acidic and rich in cysteine (SPARC) (226); placental lactogen (227) and microparticles from both Gram positive and Gram negative bacteria (228).
Additionally, a number of early studies showed stabilin-1 to be a multi-functional scavenger receptor with the ability to directly interact with leukocytes and effectively act as a leukocyte adhesion molecule. However, the ability of stabilin-1 to perform this particular function has historically been considered a contentious issue (229, 230), which is possibly confounded by the fact that the leukocyte-expressed ligand(s) for stabilin-1 still remains unidentified. Nevertheless, there is a growing body of evidence for this adhesive function and its first description was by the Jalkanen group (217), when they demonstrated that antibody blockade of stabilin-1 on high endothelial venules (HEVs) and lymphatic vessels, in both in vitro static adhesion assays and under flow conditions in vivo, significantly diminished the number of adherent lymphocytes, granulocytes, and monocytes (217). Around this time, the same group presented further evidence, showing the stabilin-1-mediated adhesion of B lymphoblastoid cell lines to lymphatic endothelial cells and HEVs in vitro (166). Subsequently, this group then demonstrated that stabilin-1 plays a key role in the transmigration of leukocytes through vascular and lymphatic endothelial cells in vitro (218) and later confirmed in vivo that it mediates the transendothelial migration of T cells and B cells across HEVs to the draining lymph nodes (219). Furthermore, they also showed that antibody blockade of stabilin-1 effectively inhibited peritonitis in mice by decreasing granulocyte recruitment by ~50%, whilst migration of monocytes and lymphocytes into the inflamed peritoneum was almost completely inhibited (219). More recently, the Jalkanen group have also shown that stabilin-1 plays a key role in the recruitment of immunosuppressive macrophages and T regulatory (Treg) lymphocytes in in vivo models of tumor growth and metastasis, with reduced numbers of both cell types demonstrated in the absence and therapeutic blockade of stabilin-1 (231).
In addition to this, and consistent with the Jalkanen group's data, our lab has implicated stabilin-1 in the transendothelial migration of both Tregs and B-cells through hepatic sinusoidal endothelial cells (HSECs) in vitro, under conditions which mimic the physiological flow and proinflammatory microenvironment of the hepatic sinusoids during liver injury (89, 220, 232). Interestingly, in the context of hepatic microvasculature, monocyte recruitment does not appear to be supported by stabilin-1, with antibody blockade on HSEC in vitro exhibiting no effect on neither monocyte adhesion nor transmigration, under physiological flow (unpublished data). Also, the leukocyte adhesion function of HSEC-expressed stabilin-1 appeared to be redundant in vivo, in murine models of liver injury, as no significant differences in Treg or B cell numbers were found between stabilin-1−/− mice and their wild type counterparts, in both carbon tetrachloride (CCl4)- and methionine and choline-deficient (MCD) diet-induced liver injury models (13). Nevertheless, given that Karikoski et al. showed significantly decreased numbers of Tregs were present in their murine tumor models when stabilin-1−/− mice were compared to WT controls (231), it can be speculated that stabilin-1's role in the recruitment of Tregs across HSEC will be potentially important in the context of hepatocellular carcinoma (HCC). Karikoski et al. also showed that stabilin-1−/− mice presented with smaller primary and metastatic tumors than WT mice (231) and these findings were corroborated with preliminary data in human HCC tissues ex vivo, which has shown that stabilin-1 expression is highly augmented in peritumoural endothelia and correlated with adverse histological features (233). This suggests that stabilin-1 potentially plays an adverse role in malignancy by potentiating the suppression of the host immune response to a neoplasm; consequently, a Phase I/II trial, TIETALC, (Tumor Immunity Enabling Technology Against Liver Cancer) is currently being designed at the University of Birmingham to test the efficacy of targeting stabilin-1 in HCC (234).
Stabilin-2
The second member of the Class H scavenger receptor family, stabilin-2, also known as FEEL2 or HARE (hyaluronan receptor for endocytosis), is very similar in structure to stabilin-1 with both exhibiting similar domain organization in their extracellular regions. Stabilin-2 was originally described as a clearance receptor for hyaluronan (216, 235, 236); however, it is now known to bind a wide range of structurally diverse ligands. For example, stabilin-2 has also been shown to bind to acLDLs (228), heparin (237), apoptotic (238, 239), and necrotic (240) cells and microparticles from both Gram positive and Gram negative bacteria (228). Like stabilin-1, stabilin-2 has also been shown to be expressed in HSEC (235, 241, 242) and can also mediate lymphocyte recruitment to the hepatic sinusoidal endothelium (241). Through a number of mutation experiments and antibody blockade studies in vitro, Jung et al. found that the fasciclin 1 (FAS1) domains of stabilin-2 were response for lymphocyte binding and identified αMβ2 integrin as the lymphocyte-expressed ligand (241). They also determined that stabilin-2 expression was not regulated in HSEC by proinflammatory stimuli previously shown to activate endothelia and subsequently suggested that stabilin-2 predominantly acts in the firm adhesion step of the leukocyte adhesion cascade as its forced down regulation via shRNA treatment did not affect lymphocyte rolling or transendothelial migration, but was still able to significantly reduce the number of adherent cells (241). Despite their identification of the lymphocyte-expressed ligand for stabilin-2, the study undertaken by Jung et al. remains the only exploration of stabilin-2's lymphocyte binding ability to date. Since monocytes (243) and neutrophils (244) also express αMβ2, it would be interesting to investigate whether or not stabilin-2 is also able to mediate the binding of these myeloid populations. Furthermore, the Jung study was restricted to stabilin-2-mediated lymphocyte binding in the context of HSEC (241); however, splice-variants have also been identified in non-continuous sinusoidal endothelia of other tissues, such as lymph nodes and the spleen (235, 245) and so future studies could also explore the potential role of stabilin-2 in leukocyte recruitment to these alternative tissues.
Future Work and Therapeutic Potential
Trafficking of leukocytes represents the fundamental basis of any type of immunological response and so targeting this process remains an attractive prospect in the suppression of a wide variety of inflammatory diseases. Whilst many of the key players in this process have been identified, we have summarized the gathering evidence that scavenger receptors can act as atypical adhesion receptors which contribute to leukocyte homing (Figure 1). In summarizing this literature, it is evident that further work is required to understand the exact mechanisms by which scavenger receptors contribute to leukocyte adhesion and migration.
Scavenger receptors can rapidly recycle from the cell membrane (246) and are also known to interact with other pattern recognition receptors (20); this therefore leads to the question of whether or not scavenger receptors contribute to leukocyte adhesion in a direct or indirect manner. In addition, given that scavenger receptors have important homeostatic functions in the remove of endogenous waste products from cell turnover, further experimental work is required to understand how the multifunctional properties of these receptors influence their in vivo contributions. It is currently unclear if there is a hierarchy in ligand recognition/affinity and how the leukocyte homing properties of scavenger receptors work alongside their homeostatic functionality. Whilst the experiments described in this review have confirmed a role for scavenger receptors in leukocyte homing, in several cases the identity of the ligand they bind on leukocytes have not been elucidated (Table 1), although imaging has demonstrated that some these receptors, such as stabilin-1 and SCARF1, directly interact with leukocytes on the endothelial surface (182, 220). The development of high resolution imaging will hopefully help answer some of these questions, focusing on the trafficking of scavenger receptors and their membrane dynamics during leukocyte recruitment as well as their interaction with other cell membrane molecules.
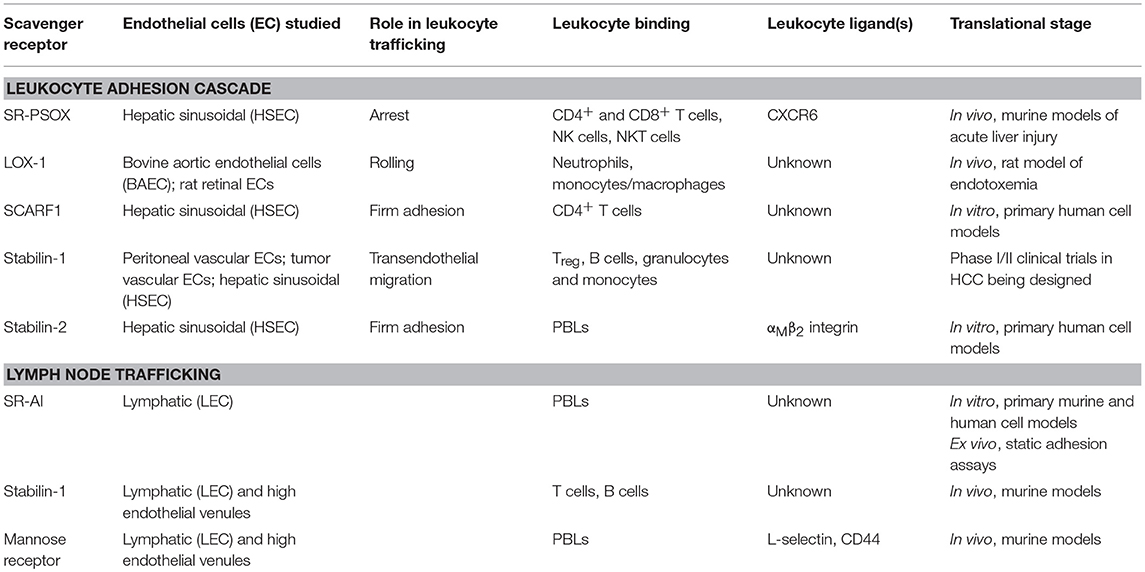
Table 1. Summary of endothelial-expressed scavenger receptor function, leukocyte/ligand binding, and translational stage of research.
Despite the need for further experimental work in this area, the potential of scavenger receptors as therapeutic targets in inflammatory disease should be explored. Due to their enrichment in specialized vascular beds, such as lymphatics and other sinusoidal endothelial vasculature, and the fact that leukocyte recruitment differs here from conventional vascular beds, scavenger receptors may predominantly influence recruitment in an organ-specific manner. They present a promising avenue for the translational development of clinical therapies to target inappropriate inflammatory reactions, such as autoimmunity, as well as hepatic inflammation and recruitment in the bone marrow niche. With regards to the potential targeting of scavenger receptors in the leukocyte adhesion cascade, liver-specific targeting may present more viable therapeutic targets than endothelia of other organs, given the increased expression of scavenger receptors in HSEC (247). Additionally, the low shear stress environment results in a largely selectin-free leukocyte adhesion cascade, thus allowing for a greater contribution by atypical adhesion molecules to leukocyte recruitment.
However, targeting the leukocyte adhesion cascade to treat inflammatory diseases could potentially be associated with significant side effects related to impaired immune surveillance and increased risk of invasion by pathogenic organisms. Nevertheless, detailed analysis of leukocyte recruitment of some scavenger receptors have shown that, rather than a pan-leukocyte effect, some of them influence the trafficking of specific subsets of leukocytes (Table 1). This suggests that these receptors may indeed be therapeutically effective in shaping the immune microenvironment by altering the balance of immune populations at the site of inflammation, whilst also minimizing potential side effects. Stabilin-1, for example, predominantly mediates the recruitment of regulatory T cells across liver endothelium suggesting that blocking its action would be more appropriate in the setting of malignancy to boost tumor-specific immune responses, whilst other scavenger receptors, such as LOX-1, appear to be more pro-inflammatory. Several preclinical experimental approaches have utilized monoclonal antibodies to block the action of this family of receptors in leukocyte recruitment; therefore, the development of humanized therapeutic antibodies appears to be a reasonable approach to target these receptors in the clinic. However, a caveat when using monoclonal antibodies is the probability of off-target effects, considering the differential expression of many scavenger receptors in a range of professional immune cells. Therefore, a more LEC- or HSEC-specific approach, e.g., adenoviral vector (AVV) delivery of siRNA, would perhaps be the most germane approach, as this would feasibly negate any potential off-target effects. Finally, the emerging evidence that scavenger receptors interact with other receptors and their multifunctional properties suggest that, as well as monotherapies, scavenger receptors could also be combined with other therapies, for example TLR-directed treatments, to alter leukocyte trafficking and boost the effectiveness of other therapies which target other arms of the immune response.
Conclusions
There is an increasing amount of evidence describing the role of endothelial-expressed scavenger receptors in leukocyte trafficking. In this capacity, a number of scavenger receptors are able to directly interact with leukocytes and mediate their passage across a range of endothelia. This secondary function is relatively understudied and further work could lead to novel immunological therapies which could effectively treat inflammatory conditions and contribute to combinatorial approaches to manage these conditions.
Disclaimer
This paper presents independent research supported by the Birmingham NIHR Liver Biomedical Research Unit based at the University Hospitals Birmingham NHS Foundation Trust and the University of Birmingham. The views expressed are those of the author and not necessarily those of the NHS, the NIHR or the Department of Health.
Author Contributions
DP conceived the review, wrote, and edited the manuscript. SS wrote the manuscript.
Funding
This work was funded by a Medical Research Council Project Grant (MR/R010013/1).
Conflict of Interest Statement
SS has received a research grant from Faron Pharmaceuticals to design a Phase I/II trial (TIETALC) of the drug “Clevergen” in patients with HCC. SS also reports consulting for Faron Pharmaceuticals.
The remaining author declares that the research was conducted in the absence of any commercial or financial relationships that could be construed as a potential conflict of interest.
References
1. Brown MS, Goldstein JL. Receptor-mediated endocytosis: insights from the lipoprotein receptor system. Proc Natl Acad Sci USA. (1979) 76:3330–7. doi: 10.1073/pnas.76.7.3330
2. Brown MS, Goldstein JL, Krieger M, Ho Y, Anderson R. Reversible accumulation of cholesteryl esters in macrophages incubated with acetylated lipoproteins. J Cell Biol. (1979) 82:597–613. doi: 10.1083/jcb.82.3.597
3. Fogelman A, Haberland M, Seager J, Hokom M, Edwards P. Factors regulating the activities of the low density lipoprotein receptor and the scavenger receptor on human monocyte-macrophages. J Lipid Res. (1981) 22:1131–41.
4. Canton J, Neculai D, Grinstein S. Scavenger receptors in homeostasis and immunity. Nat Rev Immunol. (2013) 13:621–34. doi: 10.1038/nri3515
5. Sambrano GR, Steinberg D. Recognition of oxidatively damaged and apoptotic cells by an oxidized low density lipoprotein receptor on mouse peritoneal macrophages: role of membrane phosphatidylserine. Proc Natl Acad Sci USA. (1995) 92:1396–400. doi: 10.1073/pnas.92.5.1396
6. Navazo MDP, Daviet L, Savill J, Ren Y, Leung LL, Mcgregor JL. Identification of a domain (155–183) on CD36 implicated in the phagocytosis of apoptotic neutrophils. J Biol Chem. (1996) 271:15381–5. doi: 10.1074/jbc.271.26.15381
7. Ramirez-Ortiz ZG, Pendergraft Iii WF, Prasad A, Byrne MH, Iram T, Blanchette CJ, et al. The scavenger receptor SCARF1 mediates the clearance of apoptotic cells and prevents autoimmunity. Nat Immunol. (2013) 14:917–26. doi: 10.1038/ni.2670
8. Rigotti A, Acton SL, Krieger M. The class B scavenger receptors SR-BI and CD36 are receptors for anionic phospholipids. J Biol Chem. (1995) 270:16221–4. doi: 10.1074/jbc.270.27.16221
9. Kunjathoor VV, Febbraio M, Podrez EA, Moore KJ, Andersson L, Koehn S, et al. Scavenger receptors class AI/II and CD36 are the principal receptors responsible for the uptake of modified low density lipoprotein leading to lipid loading in macrophages. J Biol Chem. (2002) 277:49982–8. doi: 10.1074/jbc.M209649200
10. Wermeling F, Chen Y, Pikkarainen T, Scheynius A, Winqvist O, Izui S, et al. Class A scavenger receptors regulate tolerance against apoptotic cells, and autoantibodies against these receptors are predictive of systemic lupus. J Exp Med. (2007) 204:2259–65. doi: 10.1084/jem.20070600
11. Schledzewski K, Géraud C, Arnold B, Wang S, Gröne H-J, Kempf T, et al. Deficiency of liver sinusoidal scavenger receptors stabilin-1 and-2 in mice causes glomerulofibrotic nephropathy via impaired hepatic clearance of noxious blood factors. J Clin Invest. (2011) 121:703–14. doi: 10.1172/JCI44740
12. Thanopoulou K, Fragkouli A, Stylianopoulou F, Georgopoulos S. Scavenger receptor class B type I (SR-BI) regulates perivascular macrophages and modifies amyloid pathology in an Alzheimer mouse model. Proc Natl Acad Sci USA. (2010) 107:20816–21. doi: 10.1073/pnas.1005888107
13. Rantakari P, Patten DA, Valtonen J, Karikoski M, Gerke H, Dawes H, et al. Stabilin-1 expression defines a subset of macrophages that mediate tissue homeostasis and prevent fibrosis in chronic liver injury. Proc Natl Acad Sci USA. (2016) 113:9298–303. doi: 10.1073/pnas.1604780113
14. Mehta JL, Sanada N, Hu CP, Chen J, Dandapat A, Sugawara F, et al. Deletion of LOX-1 reduces atherogenesis in LDLR knockout mice fed high cholesterol diet. Circ Res. (2007) 100:1634–42. doi: 10.1161/CIRCRESAHA.107.149724
15. Febbraio M, Podrez EA, Smith JD, Hajjar DP, Hazen SL, Hoff HF, et al. Targeted disruption of the class B scavenger receptor CD36 protects against atherosclerotic lesion development in mice. J Clin Invest. (2000) 105:1049–56. doi: 10.1172/JCI9259
16. Kuchibhotla S, Vanegas D, Kennedy DJ, Guy E, Nimako G, Morton RE, et al. Absence of CD36 protects against atherosclerosis in ApoE knock-out mice with no additional protection provided by absence of scavenger receptor AI/II. Cardiovasc Res. (2007) 78:185–96. doi: 10.1093/cvr/cvm093
17. Mäkinen PI, Lappalainen JP, Heinonen SE, Leppänen P, Lähteenvuo MT, Aarnio JV, et al. Silencing of either SR-A or CD36 reduces atherosclerosis in hyperlipidaemic mice and reveals reciprocal upregulation of these receptors. Cardiovasc Res. (2010) 88:530–8. doi: 10.1093/cvr/cvq235
18. Areschoug T, Gordon S. Scavenger receptors: role in innate immunity and microbial pathogenesis. Cell Microbiol. (2009) 11:1160–9. doi: 10.1111/j.1462-5822.2009.01326.x
19. Eddie Ip W, Takahashi K, Alan Ezekowitz R, Stuart LM. Mannose-binding lectin and innate immunity. Immunol Rev. (2009) 230:9–21. doi: 10.1111/j.1600-065X.2009.00789.x
20. Murshid A, Borges TJ, Lang BJ, Calderwood SK. The scavenger receptor SREC-I cooperates with toll-like receptors to trigger inflammatory innate immune responses. Front Immunol. (2016) 7:226. doi: 10.3389/fimmu.2016.00226
21. Van Die I, Cummings RD. The mannose receptor in regulation of helminth-mediated host immunity. Front Immunol. (2017) 8:1677. doi: 10.3389/fimmu.2017.01677
22. Kzhyshkowska J, Neyen C, Gordon S. Role of macrophage scavenger receptors in atherosclerosis. Immunobiology (2012) 217:492–502. doi: 10.1016/j.imbio.2012.02.015
23. Wang D, Sun B, Feng M, Feng H, Gong W, Liu Q, et al. Role of scavenger receptors in dendritic cell function. Hum Immunol. (2015) 76:442–6. doi: 10.1016/j.humimm.2015.03.012
24. Mukhopadhyay S, Gordon S. The role of scavenger receptors in pathogen recognition and innate immunity. Immunobiology (2004) 209:39–49. doi: 10.1016/j.imbio.2004.02.004
25. Plüddemann A, Mukhopadhyay S, Gordon S. The interaction of macrophage receptors with bacterial ligands. Expert Rev Mol Med. (2006) 8:1–25. doi: 10.1017/S1462399406000159
26. Amiel E, Alonso A, Uematsu S, Akira S, Poynter ME, Berwin B. Pivotal advance: toll-like receptor regulation of scavenger receptor-A-mediated phagocytosis. J Leukoc Biol. (2009) 85:595–605. doi: 10.1189/jlb.1008631
27. Mukhopadhyay S, Varin A, Chen Y, Liu B, Tryggvason K, Gordon S. SR-A/MARCO–mediated ligand delivery enhances intracellular TLR and NLR function, but ligand scavenging from cell surface limits TLR4 response to pathogens. Blood (2011) 117:1319–28. doi: 10.1182/blood-2010-03-276733
28. Jeannin P, Bottazzi B, Sironi M, Doni A, Rusnati M, Presta M, et al. Complexity and complementarity of outer membrane protein A recognition by cellular and humoral innate immunity receptors. Immunity (2005) 22:551–60. doi: 10.1016/j.immuni.2005.03.008
29. Murshid A, Gong J, Ahmad R, Borges TJ, Calderwood SK. Scavenger receptor SREC-I promotes double stranded RNA-mediated TLR3 activation in human monocytes. Immunobiology (2015) 220:823–32. doi: 10.1016/j.imbio.2014.12.011
30. Murshid A, Gong J, Prince T, Borges TJ, Calderwood SK. Scavenger receptor SREC-I mediated entry of TLR4 into lipid microdomains and triggered inflammatory cytokine release in RAW 264.7 cells upon LPS activation. PLoS ONE (2015) 10:e0122529. doi: 10.1371/journal.pone.0122529
31. Carman CV, Martinelli R. T lymphocyte–endothelial interactions: emerging understanding of trafficking and antigen-specific immunity. Front Immunol. (2015) 6:603. doi: 10.3389/fimmu.2015.00603
32. Girard J-P, Moussion C, Förster R. HEVs, lymphatics and homeostatic immune cell trafficking in lymph nodes. Nat Rev Immunol. (2012) 12:762. doi: 10.1038/nri3298
33. Guermonprez P, Valladeau J, Zitvogel L, Théry C, Amigorena S. Antigen presentation and T cell stimulation by dendritic cells. Annu Rev Immunol. (2002) 20:621–67. doi: 10.1146/annurev.immunol.20.100301.064828
34. Nourshargh S, Alon R. Leukocyte migration into inflamed tissues. Immunity (2014) 41:694–707. doi: 10.1016/j.immuni.2014.10.008
35. Ley K, Laudanna C, Cybulsky MI, Nourshargh S. Getting to the site of inflammation: the leukocyte adhesion cascade updated. Nat Rev Immunol. (2007) 7:678. doi: 10.1038/nri2156
36. Vestweber D. How leukocytes cross the vascular endothelium. Nat Rev Immunol. (2015) 15:692. doi: 10.1038/nri3908
37. Olson TS, Ley K. Chemokines and chemokine receptors in leukocyte trafficking. Am J Physiol Regul Integr Compar Physiol. (2002) 283:R7–R28. doi: 10.1152/ajpregu.00738.2001
38. Stark K, Eckart A, Haidari S, Tirniceriu A, Lorenz M, Von Brühl M-L, et al. Capillary and arteriolar pericytes attract innate leukocytes exiting through venules and'instruct'them with pattern-recognition and motility programs. Nat Immunol. (2013) 14:41. doi: 10.1038/ni.2477
39. Pober JS, Sessa WC. Evolving functions of endothelial cells in inflammation. Nat Rev Immunol. (2007) 7:803. doi: 10.1038/nri2171
40. Jones D, Abbassi O, Mcintire L, Mcever R, Smith CW. P-selectin mediates neutrophil rolling on histamine-stimulated endothelial cells. Biophys J. (1993) 65:1560–9. doi: 10.1016/S0006-3495(93)81195-0
41. Sugama Y, Tiruppathi C, Andersen T, Fenton J, Malik A. Thrombin-induced expression of endothelial P-selectin and intercellular adhesion molecule-1: a mechanism for stabilizing neutrophil adhesion. J Cell Biol. (1992) 119:935–44. doi: 10.1083/jcb.119.4.935
42. Geng J-G, Bevilacquat MP, Moore KL, Mclntyre TM, Prescott SM, Kim JM, et al. Rapid neutrophil adhesion to activated endothelium mediated by GMP-140. Nature (1990) 343:757. doi: 10.1038/343757a0
43. Lorant DE, Patel KD, Mcintyre TM, Mcever RP, Prescott SM, Zimmerman GA. Coexpression of GMP-140 and PAF by endothelium stimulated by histamine or thrombin: a juxtacrine system for adhesion and activation of neutrophils. J Cell Biol. (1991) 115:223–34. doi: 10.1083/jcb.115.1.223
44. Kelly E, Bailey CP, Henderson G. Agonist-selective mechanisms of GPCR desensitization. Br J Pharmacol. (2008) 153. doi: 10.1038/sj.bjp.0707604
45. Rajagopal S, Shenoy SK. GPCR desensitization: acute and prolonged phases Cell Signal . (2017) 41:9–16. doi: 10.1016/j.cellsig.2017.01.024
46. Pober JS, Gimbrone MA, Lapierre LA, Mendrick DL, Fiers W, Rothlein R, et al. Overlapping patterns of activation of human endothelial cells by interleukin 1, tumor necrosis factor, and immune interferon. J Immunol. (1986) 137:1893–6.
47. Pugin J, Schürer-Maly C, Leturcq D, Moriarty A, Ulevitch RJ, Tobias PS. Lipopolysaccharide activation of human endothelial and epithelial cells is mediated by lipopolysaccharide-binding protein and soluble CD14. Proc Natl Acad Sci USA. (1993) 90:2744–8. doi: 10.1073/pnas.90.7.2744
48. Zeuke S, Ulmer AJ, Kusumoto S, Katus HA, Heine H. TLR4-mediated inflammatory activation of human coronary artery endothelial cells by LPS. Cardiovasc Res. (2002) 56:126–34. doi: 10.1016/S0008-6363(02)00512-6
49. Amberger A, Maczek C, Jürgens G, Michaelis D, Schett G, Trieb K, et al. Co-expression of ICAM-1, VCAM-1, ELAM-1 and Hsp60 in human arterial and venous endothelial cells in response to cytokines and oxidized low-density lipoproteins. Cell Stress Chaperones (1997) 2:94.
50. Cominacini L, Garbin U, Pasini AF, Davoli A, Campagnola M, Contessi GB, et al. Antioxidants inhibit the expression of intercellular cell adhesion molecule-1 and vascular cell adhesion molecule-1 induced by oxidized LDL on human umbilical vein endothelial cells. Free Rad Biol Med. (1997) 22:117–27. doi: 10.1016/S0891-5849(96)00271-7
51. Campos SB, Ashworth SL, Wean S, Hosford M, Sandoval RM, Hallett MA, et al. Cytokine-induced F-actin reorganization in endothelial cells involves RhoA activation. Am J Physiol Renal Physiol. (2009) 296:F487–F495. doi: 10.1152/ajprenal.00112.2008
52. Leeuwenberg J, Smeets E, Neefjes J, Shaffer M, Cinek T, Jeunhomme T, et al. E-selectin and intercellular adhesion molecule-1 are released by activated human endothelial cells in vitro . Immunology (1992) 77:543.
53. Yang L, Froio RM, Sciuto TE, Dvorak AM, Alon R, Luscinskas FW. ICAM-1 regulates neutrophil adhesion and transcellular migration of TNF-α-activated vascular endothelium under flow. Blood (2005) 106:584–92. doi: 10.1182/blood-2004-12-4942
54. Fries J, Williams AJ, Atkins R, Newman W, Lipscomb M, Collins T. Expression of VCAM-1 and E-selectin in an in vivo model of endothelial activation. Am J Pathol. (1993) 143:725.
55. Pigott R, Dillon L, Hemingway I, Gearing A. Soluble forms of E-selectin, ICAM-1 and VCAM-1 are present in the supernatants of cytokine activated cultured endothelial cells. Biochem Biophys Res Commun. (1992) 187:584–9. doi: 10.1016/0006-291X(92)91234-H
56. Oo YH, Shetty S, Adams DH. The role of chemokines in the recruitment of lymphocytes to the liver. Digest Dis. (2010) 28:31–44. doi: 10.1159/000282062
57. Middleton J, Patterson AM, Gardner L, Schmutz C, Ashton BA. Leukocyte extravasation: chemokine transport and presentation by the endothelium. Blood (2002) 100:3853–60. doi: 10.1182/blood.V100.12.3853
58. Sugimoto MA, Sousa LP, Pinho V, Perretti M, Teixeira MM. Resolution of inflammation: what controls its onset? Front Immunol. (2016) 7:160. doi: 10.3389/fimmu.2016.00160
59. Winsauer G, De Martin R. Resolution of inflammation: intracellular feedback loops in the endothelium. Thromb Haemost. (2007) 98:364–9. doi: 10.1160/TH06-08-0473
60. Mcever RP. Selectins: initiators of leucocyte adhesion and signalling at the vascular wall. Cardiovasc Res. (2015) 107:331–9. doi: 10.1093/cvr/cvv154
61. Kansas GS. Selectins and their ligands: current concepts and controversies. Blood (1996) 88:3259–87.
62. Wagner D. The Weibel-Palade body: the storage granule for von Willebrand factor and P-selectin. Thromb Haemost. (1993) 70:105. doi: 10.1055/s-0038-1646169
63. Tenaglia AN, Buda AJ, Wilkins RG, Barron MK, Jeffords PR, Vo K, et al. Levels of expression of P-selectin, E-selectin, and intercellular adhesion molecule-1 in coronary atherectomy specimens from patients with stable and unstable angina pectoris. Am J Cardiol. (1997) 79:742–7. doi: 10.1016/S0002-9149(96)00861-2
64. Johnson-Tidey RR, Mcgregor JL, Taylor PR, Poston RN. Increase in the adhesion molecule P-selectin in endothelium overlying atherosclerotic plaques. Coexpression with intercellular adhesion molecule-1. Am J Pathol. (1994) 144:952–61.
65. Schürmann G, Bishop A, Facer P, Vecchio M, Lee J, Rampton D, et al. Increased expression of cell adhesion molecule P-selectin in active inflammatory bowel disease. Gut (1995) 36:411–8. doi: 10.1136/gut.36.3.411
66. González-Tajuelo R, Silván J, Pérez-Frías A, De La Fuente-Fernández M, Tejedor R, Espartero-Santos M, et al. P-Selectin preserves immune tolerance in mice and is reduced in human cutaneous lupus. Sci Rep. (2017) 7:41841. doi: 10.1038/srep41841
67. Norman K, Moore K, Mcever R, Ley K. Leukocyte rolling in vivo is mediated by P-selectin glycoprotein ligand-1. Blood (1995) 86:4417–21.
68. Ley K, Bullard DC, Arbonés ML, Bosse R, Vestweber D, Tedder TF, et al. Sequential contribution of L-and P-selectin to leukocyte rolling in vivo . J Exp Med. (1995) 181:669–75. doi: 10.1084/jem.181.2.669
69. Mayadas TN, Johnson RC, Rayburn H, Hynes RO, Wagner DD. Leukocyte rolling and extravasation are severely compromised in P selectin-deficient mice. Cell (1993) 74:541–54. doi: 10.1016/0092-8674(93)80055-J
70. Liu Z, Miner JJ, Yago T, Yao L, Lupu F, Xia L, et al. Differential regulation of human and murine P-selectin expression and function in vivo . J Exp Med. (2010) 20101545. doi: 10.1084/jem.20101545
71. Ivetic A. Signals regulating L-selectin-dependent leucocyte adhesion and transmigration. Int J Biochem Cell Biol. (2013) 45:550–5. doi: 10.1016/j.biocel.2012.12.023
72. Schweitzer KM, Dräger A, Van Der Valk P, Thijsen S, Zevenbergen A, Theijsmeijer AP, et al. Constitutive expression of E-selectin and vascular cell adhesion molecule-1 on endothelial cells of hematopoietic tissues. Am J Pathol. (1996) 148:165.
73. Kunkel EJ, Ley K. Distinct phenotype of E-selectin–deficient mice: E-selectin is required for slow leukocyte rolling in vivo . Circ Res. (1996) 79:1196–204. doi: 10.1161/01.RES.79.6.1196
74. Li Q, Wayman A, Lin J, Fang Y, Zhu C, Wu J. Flow-enhanced stability of rolling adhesion through E-selectin. Biophys J. (2016) 111:686–99. doi: 10.1016/j.bpj.2016.07.014
75. Marshall BT, Long M, Piper JW, Yago T, Mcever RP, Zhu C. Direct observation of catch bonds involving cell-adhesion molecules. Nature (2003) 423:190. doi: 10.1038/nature01605
76. Sundd P, Gutierrez E, Koltsova EK, Kuwano Y, Fukuda S, Pospieszalska MK, et al. ‘Slings' enable neutrophil rolling at high shear. Nature (2012) 488:399–403. doi: 10.1038/nature11248
77. Marki A, Buscher K, Mikulski Z, Pries A, Ley K. Rolling neutrophils form tethers and slings under physiologic conditions in vivo . J Leukoc Biol. (2018) 103:67–70. doi: 10.1189/jlb.1AB0617-230R
78. Abadier M, Pramod AB, Mcardle S, Marki A, Fan Z, Gutierrez E, et al. Effector and regulatory T cells roll at high shear stress by inducible tether and sling formation. Cell Rep. (2017) 21:3885–99. doi: 10.1016/j.celrep.2017.11.099
79. Hamel DJ, Proudfoot AE, Handel TM. Interactions of chemokines with glycosaminoglycans. Meth Enzymol. (2009) 461:71–102. doi: 10.1016/S0076-6879(09)05404-4
80. Johnson Z, Proudfoot A, Handel T. Interaction of chemokines and glycosaminoglycans: a new twist in the regulation of chemokine function with opportunities for therapeutic intervention. Cytokine Growth Factor Rev. (2005) 16:625–36. doi: 10.1016/j.cytogfr.2005.04.006
81. Alon R, Shulman Z. Chemokine triggered integrin activation and actin remodeling events guiding lymphocyte migration across vascular barriers. Exp Cell Res. (2011) 317:632–41. doi: 10.1016/j.yexcr.2010.12.007
82. Askari JA, Buckley PA, Mould AP, Humphries MJ. Linking integrin conformation to function. J Cell Sci. (2009) 122:165–70. doi: 10.1242/jcs.018556
83. Grabovsky V, Feigelson S, Chen C, Bleijs DA, Peled A, Cinamon G, et al. Subsecond induction of α4 integrin clustering by immobilized chemokines stimulates leukocyte tethering and rolling on endothelial vascular cell adhesion molecule 1 under flow conditions. J Exp Med. (2000) 192:495–506. doi: 10.1084/jem.192.4.495
84. Auffray C, Fogg D, Garfa M, Elain G, Join-Lambert O, Kayal S, et al. Monitoring of blood vessels and tissues by a population of monocytes with patrolling behavior. Science (2007) 317:666–70. doi: 10.1126/science.1142883
85. Carlin LM, Stamatiades EG, Auffray C, Hanna RN, Glover L, Vizcay-Barrena G, et al. Nr4a1-dependent Ly6C low monocytes monitor endothelial cells and orchestrate their disposal. Cell (2013) 153:362–75. doi: 10.1016/j.cell.2013.03.010
86. Sumagin R, Prizant H, Lomakina E, Waugh RE, Sarelius IH. LFA-1 and Mac-1 define characteristically different intralumenal crawling and emigration patterns for monocytes and neutrophils in situ . J Immunol . (2010) 201:1001638. doi: 10.4049/jimmunol.1001638
87. Halai K, Whiteford J, Ma B, Nourshargh S, Woodfin A. ICAM-2 facilitates luminal neutrophil-endothelial cell interactions in vivo . J Cell Sci . (2013) 127(Pt 3):620–9. doi: 10.1242/jcs.137463
88. Phillipson M, Heit B, Colarusso P, Liu L, Ballantyne CM, Kubes P. Intraluminal crawling of neutrophils to emigration sites: a molecularly distinct process from adhesion in the recruitment cascade. J Exp Med. (2006) 203:2569–75. doi: 10.1084/jem.20060925
89. Patten DA, Wilson GK, Bailey D, Shaw RK, Jalkanen S, Salmi M, et al. Human liver sinusoidal endothelial cells promote intracellular crawling of lymphocytes during recruitment: a new step in migration. Hepatology (2017) 65:294–309. doi: 10.1002/hep.28879
90. Lee J, Song KH, Kim T, Doh J. endothelial cell Focal adhesion regulates Transendothelial Migration and subendothelial crawling of T cells. Front Immunol. (2018) 9:48. doi: 10.3389/fimmu.2018.00048
91. Song KH, Lee J, Park H, Kim HM, Park J, Kwon KW, et al. Roles of endothelial A-type lamins in migration of T cells on and under endothelial layers. Sci Rep. (2016) 6:23412. doi: 10.1038/srep23412
92. Proebstl D, Voisin MB, Woodfin A, Whiteford J, D'acquisto F, Jones G E, et al. Pericytes support neutrophil subendothelial cell crawling and breaching of venular walls in vivo . J Exp Med. (2012) 209:1219–34. doi: 10.1084/jem.20111622
93. Muller WA. Transendothelial migration: unifying principles from the endothelial perspective. Immunol Rev. (2016) 273:61–75. doi: 10.1111/imr.12443
94. Heemskerk N, Schimmel L, Oort C, Van Rijssel J, Yin T, Ma B, et al. F-actin-rich contractile endothelial pores prevent vascular leakage during leukocyte diapedesis through local RhoA signalling. Nat Commun. (2016) 7:10493. doi: 10.1038/ncomms10493
95. Sullivan DP, Watson RL, Muller WA. 4D intravital microscopy uncovers critical strain differences for the roles of PECAM and CD99 in leukocyte diapedesis. Am J Physiol Heart Circul Physiol. (2016) 311:H621–32. doi: 10.1152/ajpheart.00289.2016
96. Schenkel AR, Mamdouh Z, Chen X, Liebman RM, Muller WA. CD99 plays a major role in the migration of monocytes through endothelial junctions. Nat Immunol. (2002) 3:143. doi: 10.1038/ni749
97. Nourshargh S, Krombach F, Dejana E. The role of JAM-A and PECAM-1 in modulating leukocyte infiltration in inflamed and ischemic tissues. J Leukoc Biol. (2006) 80:714–8. doi: 10.1189/jlb.1105645
98. Ostermann G, Weber KS, Zernecke A, Schröder A, Weber C. JAM-1 is a ligand of the β 2 integrin LFA-1 involved in transendothelial migration of leukocytes. Nat Immunol. (2002) 3:151. doi: 10.1038/ni755
99. Schulte D, Küppers V, Dartsch N, Broermann A, Li H, Zarbock A, et al. Stabilizing the VE-cadherin–catenin complex blocks leukocyte extravasation and vascular permeability. EMBO J. (2011) 30:4157–70. doi: 10.1038/emboj.2011.304
100. Shaw SK, Bamba PS, Perkins BN, Luscinskas FW. Real-time imaging of vascular endothelial-cadherin during leukocyte transmigration across endothelium. J Immunol. (2001) 167:2323–30. doi: 10.4049/jimmunol.167.4.2323
101. Alon R, Van Buul JD. Leukocyte breaching of endothelial barriers: the actin link. Trends Immunol. (2017) 38:606–15. doi: 10.1016/j.it.2017.05.002
102. Millán J, Hewlett L, Glyn M, Toomre D, Clark P, Ridley AJ. Lymphocyte transcellular migration occurs through recruitment of endothelial ICAM-1 to caveola-and F-actin-rich domains. Nat Cell Biol. (2006) 8:113. doi: 10.1038/ncb1356
103. Van Buul JD, Allingham MJ, Samson T, Meller J, Boulter E, García-Mata R, et al. RhoG regulates endothelial apical cup assembly downstream from ICAM1 engagement and is involved in leukocyte trans-endothelial migration. J Cell Biol. (2007) 178:1279–93. doi: 10.1083/jcb.200612053
104. Carman CV, Sage PT, Sciuto TE, Miguel A, Geha RS, Ochs HD, et al. Transcellular diapedesis is initiated by invasive podosomes. Immunity (2007) 26:784–97. doi: 10.1016/j.immuni.2007.04.015
105. Mamdouh Z, Mikhailov A, Muller WA. Transcellular migration of leukocytes is mediated by the endothelial lateral border recycling compartment. J Exp Med. (2009) 206:2795–808. doi: 10.1084/jem.20082745
106. Colom B, Bodkin JV, Beyrau M, Woodfin A, Ody C, Rourke C, et al. Leukotriene B4-neutrophil elastase axis drives neutrophil reverse transendothelial cell migration in vivo . Immunity (2015) 42:1075–86. doi: 10.1016/j.immuni.2015.05.010
107. Kroon J, Schaefer A, Van Rijssel J, Hoogenboezem M, Van Alphen F, Hordijk P, et al. Inflammation-sensitive myosin-X functionally supports leukocyte extravasation by Cdc42-mediated ICAM-1–rich endothelial filopodia formation. J Immunol. (2018) 200:1790–801. doi: 10.4049/jimmunol.1700702
108. Kodama T, Freeman M, Rohrer L, Zabrecky J, Matsudaira P, Krieger M. Type I macrophage scavenger receptor contains α-helical and collagen-like coiled coils. Nature (1990) 343:531. doi: 10.1038/343531a0
109. Kelley JL, Ozment TR, Li C, Schweitzer JB, Williams DL. Scavenger receptor-A (CD204): a two-edged sword in health and disease. Crit Rev Immunol. (2014) 34. doi: 10.1615/CritRevImmunol.2014010267
110. Matsumoto A, Naito M, Itakura H, Ikemoto S, Asaoka H, Hayakawa I, et al. Human macrophage scavenger receptors: primary structure, expression, and localization in atherosclerotic lesions. Proc Natl Acad Sci USA. (1990) 87:9133–7. doi: 10.1073/pnas.87.23.9133
111. Goldstein JL, Ho Y, Basu SK, Brown MS. Binding site on macrophages that mediates uptake and degradation of acetylated low density lipoprotein, producing massive cholesterol deposition. Proc Natl Acad Sci USA. (1979) 76:333–7. doi: 10.1073/pnas.76.1.333
112. Dejager S, Mietus-Synder M, Pitas RE. Oxidized low density lipoproteins bind to the scavenger receptor expressed by rabbit smooth muscle cells and macrophages. Arterioscler Thromb Vasc Biol. (1993) 13:371–8. doi: 10.1161/01.ATV.13.3.371
113. Todt JC, Hu B, Curtis JL. The scavenger receptor SR-AI/II (CD204) signals via the receptor tyrosine kinase Mertk during apoptotic cell uptake by murine macrophages. J Leukoc Biol. (2008) 84:510–8. doi: 10.1189/jlb.0307135
114. Berwin B, Hart JP, Rice S, Gass C, Pizzo SV, Post SR, et al. Scavenger receptor-A mediates gp96/GRP94 and calreticulin internalization by antigen-presenting cells. EMBO J. (2003) 22:6127–36. doi: 10.1093/emboj/cdg572
115. El Khoury J, Thomas CA, Loike JD, Hickman SE, Cao L, Silverstein SC. Macrophages adhere to glucose-modified basement membrane collagen IV via their scavenger receptors. J Biol Chem. (1994) 269:10197–200.
116. El Khoury J, Hickman SE, Thomas CA, Cao L, Silverstein SC, Loike JD. Scavenger receptor-mediated adhesion of microglia to β-amyloid fibrils. Nature (1996) 382:716. doi: 10.1038/382716a0
117. Neyen C, PlüDdemann A, Roversi P, Thomas B, Cai L, Van Der Westhuyzen DR, et al. Macrophage scavenger receptor A mediates adhesion to apolipoproteins AI and E. Biochemistry (2009) 48:11858–71. doi: 10.1021/bi9013769
118. Araki N, Higashi T, Mori T, Shibayama R, Kawabe Y, Kodama T, et al. Macrophage scavenger receptor mediates the endocytic uptake and degradation of advanced glycation end products of the Maillard reaction. FEBS J. (1995) 230:408–15. doi: 10.1111/j.1432-1033.1995.0408h.x
119. Hampton RY, Golenbock DT, Penman M, Krieger M, Raetz CR. Recognition and plasma clearance of endotoxin by scavenger receptors. Nature (1991) 352:342. doi: 10.1038/352342a0
120. Dunne DW, Resnick D, Greenberg J, Krieger M, Joiner KA. The type I macrophage scavenger receptor binds to gram-positive bacteria and recognizes lipoteichoic acid. Proc Natl Acad Sci USA. (1994) 91:1863–7. doi: 10.1073/pnas.91.5.1863
121. Rice PJ, Kelley JL, Kogan G, Ensley HE, Kalbfleisch JH, Browder IW, et al. Human monocyte scavenger receptors are pattern recognition receptors for (1 → 3)-β-D-glucans. J Leukoc Biol. (2002) 72:140–6. doi: 10.1189/jlb.72.1.140
122. Dewitte-Orr SJ, Collins SE, Bauer CM, Bowdish DM, Mossman KL. An accessory to the ‘Trinity': SR-As are essential pathogen sensors of extracellular dsRNA, mediating entry and leading to subsequent type I IFN responses. PLoS Pathog. (2010) 6:e1000829. doi: 10.1371/journal.ppat.1000829
123. Limmon GV, Arredouani M, Mccann KL, Minor RAC, Kobzik L, Imani F. Scavenger receptor class-A is a novel cell surface receptor for double-stranded RNA. FASEB J. (2008) 22:159–67. doi: 10.1096/fj.07-8348com
124. Yew K-H, Carsten B, Harrison C. Scavenger receptor A1 is required for sensing HCMV by endosomal TLR-3/-9 in monocytic THP-1 cells. Mol Immunol. (2010) 47:883–93. doi: 10.1016/j.molimm.2009.10.009
125. Geng YJ, Hansson G. High endothelial cells of postcapillary venules express the scavenger receptor in human peripheral lymph nodes. Scand J Immunol. (1995) 42:289–96. doi: 10.1111/j.1365-3083.1995.tb03658.x
126. Iftakhar-E-Khuda I, Fair-Mäkelä R, Kukkonen-Macchi A, Elima K, Karikoski M, Rantakari P, et al. Gene-expression profiling of different arms of lymphatic vasculature identifies candidates for manipulation of cell traffic. Proc Natl Acad Sci USA. (2016) 113:10643–8. doi: 10.1073/pnas.1602357113
127. Hashizume M, Mihara M. Blockade of IL-6 and TNF-α inhibited oxLDL-induced production of MCP-1 via scavenger receptor induction. Eur J Pharmacol. (2012) 689:249–54. doi: 10.1016/j.ejphar.2012.05.035
128. Shetty S, Weston CJ, Adams DH, Lalor PF. A flow adhesion assay to study leucocyte recruitment to human hepatic sinusoidal endothelium under conditions of shear stress. J Visual Exp. (2014) 85:51330. doi: 10.3791/51330
129. Sawamura T, Kume N, Aoyama T, Moriwaki H, Hoshikawa H, Aiba Y, et al. An endothelial receptor for oxidized low-density lipoprotein. Nature (1997) 386:73. doi: 10.1038/386073a0
130. Speer T, Owala FO, Holy EW, Zewinger S, Frenzel FL, Stähli BE, et al. Carbamylated low-density lipoprotein induces endothelial dysfunction. Eur Heart J. (2014) 35:3021–32. doi: 10.1093/eurheartj/ehu111
131. Shiu SW, Tan KC, Wong Y, Leng L, Bucala R. Glycoxidized LDL increases lectin-like oxidized low density lipoprotein receptor-1 in diabetes mellitus. Atherosclerosis (2009) 203:522–7. doi: 10.1016/j.atherosclerosis.2008.07.012
132. Oka K, Sawamura T, Kikuta K-I, Itokawa S, Kume N, Kita T, et al. Lectin-like oxidized low-density lipoprotein receptor 1 mediates phagocytosis of aged/apoptotic cells in endothelial cells. Proc Natl Acad Sci USA. (1998) 95:9535–40. doi: 10.1073/pnas.95.16.9535
133. Murphy JE, Tacon D, Tedbury PR, Hadden JM, Knowling S, Sawamura T, et al. LOX-1 scavenger receptor mediates calcium-dependent recognition of phosphatidylserine and apoptotic cells. Biochem J. (2006) 393:107–15. doi: 10.1042/BJ20051166
134. Shimaoka T, Kume N, Minami M, Hayashida K, Sawamura T, Kita T, et al. LOX-1 supports adhesion of Gram-positive and Gram-negative bacteria. J Immunol. (2001) 166:5108–14. doi: 10.4049/jimmunol.166.8.5108
135. Shih HH, Zhang S, Cao W, Hahn A, Wang J, Paulsen JE, et al. CRP is a novel ligand for the oxidized LDL receptor LOX-1. Am J Physiol Heart Circulat Physiol. (2009) 296:H1643–50. doi: 10.1152/ajpheart.00938.2008
136. Mehta J, Li D. Identification and autoregulation of receptor for OX-LDL in cultured human coronary artery endothelial cells. Biochem Biophys Res Commun. (1998) 248:511–4. doi: 10.1006/bbrc.1998.9004
137. Li L, Roumeliotis N, Sawamura T, Renier G. C-reactive protein enhances LOX-1 expression in human aortic endothelial cells: relevance of LOX-1 to C-reactive protein–induced endothelial dysfunction. Circ Res. (2004) 95:877–83. doi: 10.1161/01.RES.0000147309.54227.42
138. Kume N, Murase T, Moriwaki H, Aoyama T, Sawamura T, Masaki T, et al. Inducible expression of lectin-like oxidized LDL receptor-1 in vascular endothelial cells. Circ Res. (1998) 83:322–7. doi: 10.1161/01.RES.83.3.322
139. Minami M, Kume N, Kataoka H, Morimoto M, Hayashida K, Sawamura T, et al. Transforming growth factor-β1 increases the expression of lectin-like oxidized low-density lipoprotein receptor-1. Biochem Biophys Res Commun. (2000) 272:357–61. doi: 10.1006/bbrc.2000.2778
140. Murase T, Kume N, Korenaga R, Ando J, Sawamura T, Masaki T, et al. Fluid shear stress transcriptionally induces lectin-like oxidized LDL receptor-1 in vascular endothelial cells. Circ Res. (1998) 83:328–33. doi: 10.1161/01.RES.83.3.328
141. Hayashida K, Kume N, Minami M, Kita T. Lectin-like oxidized LDL receptor-1 (LOX-1) supports adhesion of mononuclear leukocytes and a monocyte-like cell line THP-1 cells under static and flow conditions. FEBS Lett. (2002) 511:133–8. doi: 10.1016/S0014-5793(01)03297-5
142. Li D, Williams V, Liu L, Chen H, Sawamura T, Antakli T, et al. LOX-1 inhibition in myocardial ischemia-reperfusion injury: modulation of MMP-1 and inflammation. Am J Physiol Heart Circul Physiol. (2002) 283:H1795–801. doi: 10.1152/ajpheart.00382.2002
143. Honjo M, Nakamura K, Yamashiro K, Kiryu J, Tanihara H, Mcevoy LM, et al. Lectin-like oxidized LDL receptor-1 is a cell-adhesion molecule involved in endotoxin-induced inflammation. Proc Natl Acad Sci USA. (2003) 100:1274–9. doi: 10.1073/pnas.0337528100
144. Ding Z, Mizeracki AM, Hu C, Mehta JL. LOX-1 deletion and macrophage trafficking in atherosclerosis. Biochem Biophys Res Commun. (2013) 440:210–4. doi: 10.1016/j.bbrc.2013.09.020
145. Taylor ME, Bezouska K, Drickamer K. Contribution to ligand binding by multiple carbohydrate-recognition domains in the macrophage mannose receptor. J Biol Chem. (1992) 267:1719–26.
146. Stahl PD, Rodman JS, Miller MJ, Schlesinger PH. Evidence for receptor-mediated binding of glycoproteins, glycoconjugates, and lysosomal glycosidases by alveolar macrophages. Proc Natl Acad Sci USA. (1978) 75:1399–403. doi: 10.1073/pnas.75.3.1399
147. Martinez-Pomares L, Wienke D, Stillion R, Mckenzie EJ, Arnold JN, Harris J, et al. Carbohydrate-independent recognition of collagens by the macrophage mannose receptor. Eur J Immunol. (2006) 36:1074–82. doi: 10.1002/eji.200535685
148. Malovic I, Sørensen KK, Elvevold KH, Nedredal GI, Paulsen S, Erofeev AV, et al. The mannose receptor on murine liver sinusoidal endothelial cells is the main denatured collagen clearance receptor. Hepatology (2007) 45:1454–61. doi: 10.1002/hep.21639
149. MartiNez-Pomares L, Crocker PR, DaSilva R, Holmes N, Colominas C, Rudd P, et al. Cell-specific glycoforms of sialoadhesin and CD45 are counter-receptors for the cysteine-rich domain of the mannose receptor. J Biol Chem. (1999) 274:35211–8. doi: 10.1074/jbc.274.49.35211
150. Allavena P, Chieppa M, Bianchi G, Solinas G, Fabbri M, Laskarin G, et al. Engagement of the mannose receptor by tumoral mucins activates an immune suppressive phenotype in human tumor-associated macrophages. Clin Dev Immunol. (2010) 2010:547179. doi: 10.1155/2010/547179
151. Shepherd VL, Hoidal JR. Clearance of neutrophil-derived myeloperoxidase by the macrophage mannose receptor. Am J Respir Cell Mol Biol. (1990) 2:335–40. doi: 10.1165/ajrcmb/2.4.335
152. Kang PB, Azad AK, Torrelles JB, Kaufman TM, Beharka A, Tibesar E, et al. The human macrophage mannose receptor directs Mycobacterium tuberculosis lipoarabinomannan-mediated phagosome biogenesis. J Exp Med. (2005) 202:987–99. doi: 10.1084/jem.20051239
153. Schulert GS, Allen LAH. Differential infection of mononuclear phagocytes by Francisella tularensis: role of the macrophage mannose receptor. J Leukoc Biol. (2006) 80:563–71. doi: 10.1189/jlb.0306219
154. Miller JLM, Dewet BJ, Martinez-Pomares L, Radcliffe CM, Dwek RA, Rudd PM, et al. (2008). The mannose receptor mediates dengue virus infection of macrophages. PLoS Pathog. 4:e17. doi: 10.1371/annotation/98b92fca-fa6e-4bf3-9b39-13b66b640476
155. Reading PC, Miller JL, Anders EM. Involvement of the mannose receptor in infection of macrophages by influenza virus. J Virol. (2000) 74:5190–7. doi: 10.1128/JVI.74.11.5190-5197.2000
156. Upham JP, Pickett D, Irimura T, Anders EM, Reading PC. Macrophage receptors for influenza A virus: role of the macrophage galactose-type lectin and mannose receptor in viral entry. J Virol. (2010) 84:3730–7. doi: 10.1128/JVI.02148-09
157. Nguyen DG, Hildreth JE. Involvement of macrophage mannose receptor in the binding and transmission of HIV by macrophages. Eur J Immunol. (2003) 33:483–93. doi: 10.1002/immu.200310024
158. Dan JM, Kelly RM, Lee CK, Levitz SM. Role of the mannose receptor in a murine model of Cryptococcus neoformans infection. Infect Immun. (2008) 76:2362–7. doi: 10.1128/IAI.00095-08
159. Mansour MK, Schlesinger LS, Levitz SM. Optimal T cell responses to Cryptococcus neoforman s mannoprotein are dependent on recognition of conjugated carbohydrates by mannose receptors. J Immunol. (2002) 168:2872–9. doi: 10.4049/jimmunol.168.6.2872
160. Yamamoto Y, Klein TW, Friedman H. Involvement of mannose receptor in cytokine interleukin-1beta (IL-1beta), IL-6, and granulocyte-macrophage colony-stimulating factor responses, but not in chemokine macrophage inflammatory protein 1beta (MIP-1beta), MIP-2, and KC responses, caused by attachment of Candida albicans to macrophages. Infect Immun. (1997) 65:1077–82.
161. Van De Veerdonk FL, Marijnissen RJ, Kullberg BJ, Koenen HJ, Cheng S-C, Joosten I, et al. The macrophage mannose receptor induces IL-17 in response to Candida albicans. Cell Host Microbe (2009) 5:329–40. doi: 10.1016/j.chom.2009.02.006
162. Linehan SA, Martínez-Pomares L, Stahl PD, Gordon S. Mannose receptor and its putative ligands in normal murine lymphoid and nonlymphoid organs: in situ expression of mannose receptor by selected macrophages, endothelial cells, perivascular microglia, and mesangial cells, but not dendritic cells. J Exp Med. (1999) 189:1961–72. doi: 10.1084/jem.189.12.1961
163. Stein M, Keshav S, Harris N, Gordon S. Interleukin 4 potently enhances murine macrophage mannose receptor activity: a marker of alternative immunologic macrophage activation. J Exp Med. (1992) 176:287–92. doi: 10.1084/jem.176.1.287
164. Magnusson S, Berg T. Extremely rapid endocytosis mediated by the mannose receptor of sinusoidal endothelial rat liver cells. Biochem J. (1989) 257:651. doi: 10.1042/bj2570651
165. Gröger M, Holnthoner W, Maurer D, Lechleitner S, Wolff K, Mayr BB, et al. Dermal microvascular endothelial cells express the 180-kDa macrophage mannose receptor in situ and in vitro . J Immunol. (2000) 165:5428–34. doi: 10.4049/jimmunol.165.10.5428
166. Irjala H, Alanen K, Grénman R, Heikkilä P, Joensuu H, Jalkanen S. Mannose receptor (MR) and common lymphatic endothelial and vascular endothelial receptor (CLEVER)-1 direct the binding of cancer cells to the lymph vessel endothelium. Cancer Res. (2003) 63:4671–6.
167. Irjala H, Johansson E-L, Grenman R, Alanen K, Salmi M, Jalkanen S. Mannose receptor is a novel ligand for L-selectin and mediates lymphocyte binding to lymphatic endothelium. J Exp Med. (2001) 194:1033–42. doi: 10.1084/jem.194.8.1033
168. Marttila-Ichihara F, Turja R, Miiluniemi M, Karikoski M, Maksimow M, Niemelä J, et al. Macrophage mannose receptor on lymphatics controls cell trafficking. Blood (2008) 112:64–72. doi: 10.1182/blood-2007-10-118984
169. Salmi M, Karikoski M, Elima K, Rantakari P, Jalkanen S. CD44 binds to macrophage mannose receptor on lymphatic endothelium and supports lymphocyte migration via afferent lymphatics. Circ Res Circresaha (2013) 112.300476. doi: 10.1161/CIRCRESAHA.111.300476
170. Adachi H, Tsujimoto M, Arai H, Inoue K. Expression cloning of a novel scavenger receptor from human endothelial cells. J Biol Chem. (1997) 272:31217–20. doi: 10.1074/jbc.272.50.31217
171. Adachi H, Tsujimoto M. Characterization of the human gene encoding the scavenger receptor expressed by endothelial cell and its regulation by a novel transcription factor, endothelial zinc finger protein-2. J Biol Chem. (2002) 277:24014–21. doi: 10.1074/jbc.M201854200
172. Tamura Y, Osuga JI, Adachi H, Tozawa RI, Takanezawa Y, Ohashi K, et al. Scavenger receptor expressed by endothelial cells I (SREC-I) mediates the uptake of acetylated low density lipoproteins by macrophages stimulated with lipopolysaccharide. J Biol Chem. (2004) 279:30938–44. doi: 10.1074/jbc.M313088200
173. Patten DA. SCARF1: a multifaceted, yet largely understudied, scavenger receptor. Inflamm Res. (2018) 67:627–32. doi: 10.1007/s00011-018-1154-7
174. Murshid A, Gong J, Calderwood SK. Hsp90–peptide complexes stimulate antigen presentation through the class II pathway after binding scavenger receptor SREC-I. Immunobiology (2014) 219:924–31. doi: 10.1016/j.imbio.2014.08.001
175. Facciponte JG, Wang XY, Subjeck JR. Hsp110 and Grp170, members of the Hsp70 superfamily, bind to scavenger receptor-A and scavenger receptor expressed by endothelial cells-I. Eur J Immunol. (2007) 37:2268–79. doi: 10.1002/eji.200737127
176. Gong J, Zhu B, Murshid A, Adachi H, Song B, Lee A, et al. T cell activation by heat shock protein 70 vaccine requires TLR signaling and scavenger receptor expressed by endothelial cells-1. J Immunol. (2009) 183:3092–8. doi: 10.4049/jimmunol.0901235
177. Beauvillain C, Meloni F, Sirard J-C, Blanchard S, Jarry U, Scotet M, et al. The scavenger receptors SRA-1 and SREC-I cooperate with TLR2 in the recognition of the hepatitis C virus non-structural protein 3 by dendritic cells. J Hepatol. (2010) 52:644–51. doi: 10.1016/j.jhep.2009.11.031
178. Piccolo P, Vetrini F, Mithbaokar P, Grove NC, Bertin T, Palmer D, et al. SR-A and SREC-I are Kupffer and endothelial cell receptors for helper-dependent adenoviral vectors. Mol Ther. (2013) 21:767–74. doi: 10.1038/mt.2012.287
179. Means TK, Mylonakis E, Tampakakis E, Colvin RA, Seung E, Puckett L, et al. Evolutionarily conserved recognition and innate immunity to fungal pathogens by the scavenger receptors SCARF1 and CD36. J Exp Med. (2009) 206:637–53. doi: 10.1084/jem.20082109
180. Rechner C, Kühlewein C, Müller A, Schild H, Rudel T. Host glycoprotein Gp96 and scavenger receptor SREC interact with PorB of disseminating Neisseria gonorrhoeae in an epithelial invasion pathway. Cell Host Microbe (2007) 2:393–403. doi: 10.1016/j.chom.2007.11.002
181. Baur S, Rautenberg M, Faulstich M, Grau T, Severin Y, Unger C, et al. A nasal epithelial receptor for Staphylococcus aureus WTA governs adhesion to epithelial cells and modulates nasal colonization. PLoS Pathog. (2014) 10:e1004089. doi: 10.1371/journal.ppat.1004089
182. Patten DA, Kamarajah SK, Rose JM, Tickle J, Shepherd EL, Adams DH, et al. SCARF-1 promotes adhesion of CD4+ T cells to human hepatic sinusoidal endothelium under conditions of shear stress. Sci Rep. (2017) 7:17600. doi: 10.1038/s41598-017-17928-4
183. Ishii J, Adachi H, Aoki J, Koizumi H, Tomita S, Suzuki T, et al. SREC-II, a new member of the scavenger receptor type F family, trans-interacts with SREC-I through its extracellular domain. J Biol Chem. (2002) 277:39696–702. doi: 10.1074/jbc.M206140200
184. Prabhudas MR, Baldwin CL, Bollyky PL, Bowdish DM, Drickamer K, Febbraio M, et al. A consensus definitive classification of scavenger receptors and their roles in health and disease. J Immunol. (2017) 198:3775–89. doi: 10.4049/jimmunol.1700373
185. Zani IA, Stephen SL, Mughal NA, Russell D, Homer-Vanniasinkam S, Wheatcroft SB, et al. Scavenger receptor structure and function in health and disease. Cells (2015) 4:178–201. doi: 10.3390/cells4020178
186. Ludwig A, Hundhausen C, Lambert MH, Broadway N, Andrews RC, Bickett DM, et al. Metalloproteinase inhibitors for the disintegrin-like metalloproteinases ADAM10 and ADAM17 that differentially block constitutive and phorbol ester-inducible shedding of cell surface molecules. Comb Chem High Throughput Screen. (2005) 8:161–71. doi: 10.2174/1386207053258488
187. Hundhausen C, Schulte A, Schulz B, Andrzejewski MG, Schwarz N, Von Hundelshausen P, et al. Regulated shedding of transmembrane chemokines by the disintegrin and metalloproteinase 10 facilitates detachment of adherent leukocytes. J Immunol. (2007) 178:8064–72. doi: 10.4049/jimmunol.178.12.8064
188. Gough PJ, Garton KJ, Wille PT, Rychlewski M, Dempsey PJ, Raines EW. A disintegrin and metalloproteinase 10-mediated cleavage and shedding regulates the cell surface expression of CXC chemokine ligand 16. J Immunol. (2004) 172:3678–85. doi: 10.4049/jimmunol.172.6.3678
189. Abel S, Hundhausen C, Mentlein R, Schulte A, Berkhout TA, Broadway N, et al. The transmembrane CXC-chemokine ligand 16 is induced by IFN-γ and TNF-α and shed by the activity of the disintegrin-like metalloproteinase ADAM10. J Immunol. (2004) 172:6362–72. doi: 10.4049/jimmunol.172.10.6362
190. Shimaoka T, Kume N, Minami M, Hayashida K, Kataoka H, Kita T, et al. Molecular cloning of a novel scavenger receptor for oxidized low density lipoprotein, SR-PSOX, on macrophages. J Biol Chem. (2000) 275:40663–6. doi: 10.1074/jbc.C000761200
191. Abed M, Towhid ST, Mia S, Pakladok T, Alesutan I, Borst O, et al. Sphingomyelinase-induced adhesion of eryptotic erythrocytes to endothelial cells. Am J Physiol Cell Physiol. (2012) 303:C991–9. doi: 10.1152/ajpcell.00239.2012
192. Borst O, Abed M, Alesutan I, Towhid ST, Qadri SM, Föller M, et al. Dynamic adhesion of eryptotic erythrocytes to endothelial cells via CXCL16/SR-PSOX. Am J Physiol Cell Physiol. (2011) 302:C644–51. doi: 10.1152/ajpcell.00340.2011
193. Shimaoka T, Nakayama T, Kume N, Takahashi S, Yamaguchi J, Minami M, et al. Cutting edge: SR-PSOX/CXC chemokine ligand 16 mediates bacterial phagocytosis by APCs through its chemokine domain. J Immunol. (2003) 171:1647–51. doi: 10.4049/jimmunol.171.4.1647
194. Gursel M, Gursel I, Mostowski HS, Klinman DM. CXCL16 influences the nature and specificity of CpG-induced immune activation. J Immunol. (2006) 177:1575–80. doi: 10.4049/jimmunol.177.3.1575
195. Minami M, Kume N, Shimaoka T, Kataoka H, Hayashida K, Akiyama Y, et al. Expression of SR-PSOX, a novel cell-surface scavenger receptor for phosphatidylserine and oxidized LDL in human atherosclerotic lesions. Arterioscler Thromb Vasc Biol. (2001) 21:1796–800. doi: 10.1161/hq1001.096652
196. Tabata S, Kadowaki N, Kitawaki T, Shimaoka T, Yonehara S, Yoshie O, et al. Distribution and kinetics of SR-PSOX/CXCL16 and CXCR6 expression on human dendritic cell subsets and CD4+ T cells. J Leukoc Biol. (2005) 77:777–86. doi: 10.1189/jlb.1204733
197. Wågsäter D, Olofsson PS, Norgren L, Stenberg B, Sirsjö A. The chemokine and scavenger receptor CXCL16/SR-PSOX is expressed in human vascular smooth muscle cells and is induced by interferon γ. Biochem Biophys Res Commun. (2004) 325:1187–93. doi: 10.1016/j.bbrc.2004.10.160
198. Hofnagel O, Luechtenborg B, Plenz G, Robenek H. Expression of the novel scavenger receptor SR-PSOX in cultured aortic smooth muscle cells and umbilical endothelial cells. Arterioscler Thromb Vasc Biol. (2002) 22:710–1. doi: 10.1161/01.ATV.0000012402.85056.45
199. Geissmann F, Cameron TO, Sidobre S, Manlongat N, Kronenberg M, Briskin MJ, et al. Intravascular immune surveillance by CXCR6+ NKT cells patrolling liver sinusoids. PLoS Biol. (2005) 3:e113. doi: 10.1371/journal.pbio.0030113
200. Wilbanks A, Zondlo SC, Murphy K, Mak S, Soler D, Langdon P, et al. Expression cloning of the STRL33/BONZO/TYMSTR ligand reveals elements of CC, CXC, and CX3C chemokines. J Immunol. (2001) 166:5145–54. doi: 10.4049/jimmunol.166.8.5145
201. Matloubian M, David A, Engel S, Ryan JE, Cyster JG. A transmembrane CXC chemokine is a ligand for HIV-coreceptor Bonzo. Nat Immunol. (2000) 1:298. doi: 10.1038/79738
202. Shimaoka T, Nakayama T, Fukumoto N, Kume N, Takahashi S, Yamaguchi J, et al. Cell surface-anchored SR-PSOX/CXC chemokine ligand 16 mediates firm adhesion of CXC chemokine receptor 6-expressing cells. J Leukoc Biol. (2004) 75:267–74. doi: 10.1189/jlb.1003465
203. Yamauchi R, Tanaka M, Kume N, Minami M, Kawamoto T, Togi K, et al. Upregulation of SR-PSOX/CXCL16 and recruitment of CD8+ T cells in cardiac valves during inflammatory valvular heart disease. Arterioscler Thromb Vasc Biol. (2004) 24:282–7. doi: 10.1161/01.ATV.0000114565.42679.c6
204. Ruth JH, Haas CS, Park CC, Amin MA, Martinez RJ, Haines Iii GK, et al. CXCL16-mediated cell recruitment to rheumatoid arthritis synovial tissue and murine lymph nodes is dependent upon the MAPK pathway. Arthr Rheumat. (2006) 54:765–78. doi: 10.1002/art.21662
205. Jiang X, Shimaoka T, Kojo S, Harada M, Watarai H, Wakao H, et al. Cutting edge: critical role of CXCL16/CXCR6 in NKT cell trafficking in allograft tolerance. J Immunol. (2005) 175:2051–5. doi: 10.4049/jimmunol.175.4.2051
206. Heydtmann M, Lalor PF, Eksteen JA, Hübscher SG, Briskin M, Adams DH. CXC chemokine ligand 16 promotes integrin-mediated adhesion of liver-infiltrating lymphocytes to cholangiocytes and hepatocytes within the inflamed human liver. J Immunol. (2005) 174:1055–62. doi: 10.4049/jimmunol.174.2.1055
207. Wehr A, Tacke F. The Roles of CXCL16 and CXCR6 in Liver Inflammation and Fibrosis. Curr Pathobiol Rep. (2015) 3:283–90. doi: 10.1007/s40139-015-0090-2
208. Sato T, Thorlacius H, Johnston B, Staton TL, Xiang W, Littman DR, et al. Role for CXCR6 in recruitment of activated CD8+ lymphocytes to inflamed liver. J Immunol. (2005) 174:277–83. doi: 10.4049/jimmunol.174.1.277
209. Hudspeth K, Donadon M, Cimino M, Pontarini E, Tentorio P, Preti M, et al. Human liver-resident CD56bright/CD16neg NK cells are retained within hepatic sinusoids via the engagement of CCR5 and CXCR6 pathways. J Autoimmun. (2016) 66:40–50. doi: 10.1016/j.jaut.2015.08.011
210. Stegmann KA, Robertson F, Hansi N, Gill U, Pallant C, Christophides T, et al. CXCR6 marks a novel subset of T-bet lo Eomes hi natural killer cells residing in human liver. Sci Rep. (2016) 6:26157. doi: 10.1038/srep26157
211. Wang H, Shao Y, Zhang S, Xie A, Ye Y, Shi L, et al. CXCL16 deficiency attenuates acetaminophen-induced hepatotoxicity through decreasing hepatic oxidative stress and inflammation in mice. Acta Biochim Biophys Sin. (2017) 49:541–9. doi: 10.1093/abbs/gmx040
212. Xu H, Xu W, Chu Y, Gong Y, Jiang Z, Xiong S. Involvement of up-regulated CXC chemokine ligand 16/scavenger receptor that binds phosphatidylserine and oxidized lipoprotein in endotoxin-induced lethal liver injury via regulation of T-cell recruitment and adhesion. Infect Immun. (2005) 73:4007–16. doi: 10.1128/IAI.73.7.4007-4016.2005
213. Xu H-B, Gong Y-P, Cheng J, Chu Y-W, Xiong S-D. CXCL16 participates in pathogenesis of immunological liver injury by regulating T lymphocyte infiltration in liver tissue. World J Gastroenterol. (2005) 11:4979. doi: 10.3748/wjg.v11.i32.4979
214. Ma C, Han M, Heinrich B, Fu Q, Zhang Q, Sandhu M, et al. Gut microbiome–mediated bile acid metabolism regulates liver cancer via NKT cells. Science (2018) 360:eaan5931. doi: 10.1126/science.aan5931
215. Goerdt S, Walsh LJ, Murphy GF, Pober JS. Identification of a novel high molecular weight protein preferentially expressed by sinusoidal endothelial cells in normal human tissues. J Cell Biol. (1991) 113:1425–37. doi: 10.1083/jcb.113.6.1425
216. Politz O, Gratchev A, Mccourt PA, Schledzewski K, Guillot P, Johansson S, et al. Stabilin-1 and -2 constitute a novel family of fasciclin-like hyaluronan receptor homologues. Biochem J. (2002) 362:155–64. doi: 10.1042/bj3620155
217. Irjala H, Elima K, Johansson EL, Merinen M, Kontula K, Alanen K, et al. The same endothelial receptor controls lymphocyte traffic both in vascular and lymphatic vessels. Eur J Immunol. (2003) 33:815–24. doi: 10.1002/eji.200323859
218. Salmi M, Koskinen K, Henttinen T, Elima K, Jalkanen S. CLEVER-1 mediates lymphocyte transmigration through vascular and lymphatic endothelium. Blood (2004) 104:3849–57. doi: 10.1182/blood-2004-01-0222
219. Karikoski M, Irjala H, Maksimow M, Miiluniemi M, Granfors K, Hernesniemi S, et al. Clever-1/Stabilin-1 regulates lymphocyte migration within lymphatics and leukocyte entrance to sites of inflammation. Eur J Immunol. (2009) 39:3477–87. doi: 10.1002/eji.200939896
220. Shetty S, Weston CJ, Oo YH, Westerlund N, Stamataki Z, Youster J, et al. Common lymphatic endothelial and vascular endothelial receptor-1 mediates the transmigration of regulatory T cells across human hepatic sinusoidal endothelium. J Immunol. (2011) 186:4147–55. doi: 10.4049/jimmunol.1002961
221. Goerdt S, Bhardwaj R, Sorg C. Inducible expression of MS-1 high-molecular-weight protein by endothelial cells of continuous origin and by dendritic cells/macrophages in vivo and in vitro . Am J Pathol. (1993) 142:1409.
222. Kzhyshkowska J, Gratchev A, Brundiers H, Mamidi S, Krusell L, Goerdt S. Phosphatidylinositide 3-kinase activity is required for stabilin-1-mediated endosomal transport of acLDL. Immunobiology (2005) 210:161–73. doi: 10.1016/j.imbio.2005.05.022
223. Lee S-J, Park S-Y, Jung M-Y, Bae SM, Kim I-S. Mechanism for phosphatidylserine-dependent erythrophagocytosis in mouse liver. Blood (2011) 117:5215–23. doi: 10.1182/blood-2010-10-313239
224. Park S-Y, Bae D-J, Kim M-J, Piao ML, Kim I-S. Extracellular low pH modulates phosphatidylserine-dependent phagocytosis in macrophages by increasing stabilin-1 expression. J Biol Chem. (2012) 287:11261–71. doi: 10.1074/jbc.M111.310953
225. Park S-Y, Jung M-Y, Lee S-J, Kang K-B, Gratchev A, Riabov V, et al. Stabilin-1 mediates phosphatidylserine-dependent clearance of cell corpses in alternatively activated macrophages. J Cell Sci. (2009) 122:3365–73. doi: 10.1242/jcs.049569
226. Kzhyshkowska J, Workman G, Cardó-Vila M, Arap W, Pasqualini R, Gratchev A, et al. Novel function of alternatively activated macrophages: stabilin-1-mediated clearance of SPARC. J Immunol. (2006) 176:5825–32. doi: 10.4049/jimmunol.176.10.5825
227. Kzhyshkowska J, Gratchev A, Schmuttermaier C, Brundiers H, Krusell L, Mamidi S, et al. Alternatively activated macrophages regulate extracellular levels of the hormone placental lactogen via receptor-mediated uptake and transcytosis. J Immunol. (2008) 180:3028–37. doi: 10.4049/jimmunol.180.5.3028
228. Adachi H, Tsujimoto M. FEEL-1, a novel scavenger receptor with in vitro bacteria-binding and angiogenesis-modulating activities. J Biol Chem. (2002) 277:34264–70. doi: 10.1074/jbc.M204277200
229. Kzhyshkowska J. Multifunctional receptor stabilin-1 in homeostasis and disease. Sci World J. (2010) 10:2039–53. doi: 10.1100/tsw.2010.189
230. Kzhyshkowska J, Gratchev A, Goerdt S. Stabilin-1, a homeostatic scavenger receptor with multiple functions. J Cell Mol Med. (2006) 10:635–49. doi: 10.1111/j.1582-4934.2006.tb00425.x
231. Karikoski M, Marttila-Ichihara F, Elima K, Rantakari P, Hollmén M, Kelkka T, et al. Clever-1/stabilin-1 controls cancer growth and metastasis. Clin Cancer Res. (2014) 20:6452–64. doi: 10.1158/1078-0432.CCR-14-1236
232. Shetty S, Bruns T, Weston CJ, Stamataki Z, Oo YH, Long HM, et al. Recruitment mechanisms of primary and malignant B cells to the human liver. Hepatology (2012) 56:1521–31. doi: 10.1002/hep.25790
233. Cain O, Shetty S, Huebscher S. Expression of Common Lymphatic Endothelial and Vascular Endothelial Receptor-1 (CLEVER-1) by Peritumoural Endothelium is Associated With Adverse Histological Features in Hepatocellular Carcinoma. New York, NY:Virchows Archiv: Springer 233 Spring St (2018). p. S5–S5.
234. Times F. (2016). Available online at: https://markets.ft.com/data/announce/detail?dockey=1323-13194781-25T6IP1N5CIS7BQU5VED8QRPOJ
235. Falkowski M, Schledzewski K, Hansen B, Goerdt S. Expression of stabilin-2, a novel fasciclin-like hyaluronan receptor protein, in murine sinusoidal endothelia, avascular tissues, and at solid/liquid interfaces. Histochem Cell Biol. (2003) 120:361–9. doi: 10.1007/s00418-003-0585-5
236. Zhou B, Weigel JA, Fauss L, Weigel PH. Identification of the hyaluronan receptor for endocytosis (HARE). J Biol Chem. (2000) 275:37733–41. doi: 10.1074/jbc.M003030200
237. Harris EN, Weigel JA, Weigel PH. The human hyaluronan receptor for endocytosis (HARE/Stabilin-2) is a systemic clearance receptor for heparin. J Biol Chem. (2008) 283:17341–50. doi: 10.1074/jbc.M710360200
238. Kim S, Bae D-J, Hong M, Park S-Y, Kim I-S. The conserved histidine in epidermal growth factor-like domains of stabilin-2 modulates pH-dependent recognition of phosphatidylserine in apoptotic cells. Int J Biochem Cell Biol. (2010) 42:1154–63. doi: 10.1016/j.biocel.2010.03.024
239. Park S-Y, Kim S-Y, Jung M-Y, Bae D-J, Kim I-S. Epidermal growth factor-like domain repeat of stabilin-2 recognizes phosphatidylserine during cell corpse clearance. Mol Cell Biol. (2008) 28:5288–98. doi: 10.1128/MCB.01993-07
240. D'souza S, Park SY, Kim IS. Stabilin-2 acts as an engulfment receptor for the phosphatidylserine-dependent clearance of primary necrotic cells. Biochem Biophys Res Commun. (2013) 432:412–7. doi: 10.1016/j.bbrc.2013.01.133
241. Jung M-Y, Park S-Y, Kim I-S. Stabilin-2 is involved in lymphocyte adhesion to the hepatic sinusoidal endothelium via the interaction with αMβ2 integrin. J Leukoc Biol. (2007) 82:1156–65. doi: 10.1189/jlb.0107052
242. Géraud C, Koch P-S, Zierow J, Klapproth K, Busch K, Olsavszky V, et al. GATA4-dependent organ-specific endothelial differentiation controls liver development and embryonic hematopoiesis. J Clin Invest. (2017) 127:1099–114. doi: 10.1172/JCI90086
243. Schober JM, Chen N, Grzeszkiewicz TM, Jovanovic I, Emeson EE, Ugarova TP, et al. Identification of integrin αMβ2 as an adhesion receptor on peripheral blood monocytes for Cyr61 (CCN1) and connective tissue growth factor (CCN2): immediate-early gene products expressed in atherosclerotic lesions. Blood (2002) 99:4457–65. doi: 10.1182/blood.V99.12.4457
244. Zhang L. The α βα M β 2 integrin and its role in neutrophil function. Cell Res. (1999). 9:171–9. doi: 10.1038/sj.cr.7290015
245. Hare AK, Harris EN. Tissue-specific splice variants of HARE/Stabilin-2 are expressed in bone marrow, lymph node, and spleen. Biochem Biophys Res Commun. (2015) 456:257–61. doi: 10.1016/j.bbrc.2014.11.068
246. Prevo R, Banerji S, Ni J, Jackson DG. Rapid plasma membrane-endosomal trafficking of the lymph node sinus and high endothelial venule scavenger receptor/homing receptor stabilin-1 (FEEL-1/CLEVER-1). J Biol Chem. (2004) 279:52580–92. doi: 10.1074/jbc.M406897200
Keywords: leukocyte adhesion cascade, SR-AI, LOX-1, mannose receptor, SCARF1, SR-PSOX, stabilin-1, stabilin-2
Citation: Patten DA and Shetty S (2018) More Than Just a Removal Service: Scavenger Receptors in Leukocyte Trafficking. Front. Immunol. 9:2904. doi: 10.3389/fimmu.2018.02904
Received: 03 September 2018; Accepted: 27 November 2018;
Published: 12 December 2018.
Edited by:
Susanna Carola Fagerholm, University of Helsinki, FinlandReviewed by:
Dianne Cooper, Queen Mary University of London, United KingdomKlaus Ley, La Jolla Institute for Allergy and Immunology (LJI), United States
Copyright © 2018 Patten and Shetty. This is an open-access article distributed under the terms of the Creative Commons Attribution License (CC BY). The use, distribution or reproduction in other forums is permitted, provided the original author(s) and the copyright owner(s) are credited and that the original publication in this journal is cited, in accordance with accepted academic practice. No use, distribution or reproduction is permitted which does not comply with these terms.
*Correspondence: Daniel A. Patten, ZC5hLnBhdHRlbkBiaGFtLmFjLnVr